- 1AgriBio, Agriculture Victoria Research, Bundoora, VIC, Australia
- 2School of Applied Systems Biology, La Trobe University, Bundoora, VIC, Australia
- 3Ellinbank Centre, Agriculture Victoria Research, Ellinbank, VIC, Australia
- 4Centre for Agricultural Innovation, School of Agriculture and Food, Faculty of Veterinary and Agricultural Sciences, The University of Melbourne, Parkville, VIC, Australia
Milk and serum were collected from dairy cows before and during a 2-day heat challenge. The concentrations of free short-chain fatty acids (SCFAs), the fatty acid (FA) profile, and the abundance of the major species of phosphatidylcholine (PC), phosphatidylethanolamine (PE), and sphingomyelin (SM) were measured, and samples collected during heat exposure were compared with those collected prior to heat exposure. It was found that a 2-day heat challenge did not alter the global FA composition of milk fat nor the content of the major phospholipids. Although the concentration of SCFAs C3 and C4 and some lysophosphatidylcholine (LPC) species in milk was found to be associated with the forage type, neither of these lipid molecules can be used as an indicator of acute heat stress. While it is a positive finding that short-term heat stress has no detrimental effect on the FA composition or the nutritive quality of milk fat, this study highlights the complexity of validating a milk lipid biomarker for heat stress in dairy cows.
1 Introduction
Heat stress adversely affects the health and production of dairy cows and is commonly observed in temperate, tropical, and subtropical areas (West, 2003; Sunil Kumar et al., 2011; Hempel et al., 2019). Cows begin experiencing heat stress when the air temperature increases beyond 26°C (Kadzere et al., 2002) dependent on the relative humidity. The level of heat stress can be indicated by body temperature and the temperature–humidity index (THI): THI scores of ≤ 70, 75–78, and > 78 indicate no stress, stress, and extreme stress, respectively (Kadzere et al., 2002). It is expected that the frequency and severity of heat events will increase in the future, so the identification of methods to mitigate the adverse effects of heat stress is essential for supporting the dairy industry (Das et al., 2016; Garner et al., 2016).
Heat stress is a worldwide issue affecting the fertility, growth, immune function, rumen fermentation, metabolism, and overall milk production of dairy cows (Polsky and von Keyserlingk, 2017; Osei-Amponsah et al., 2020; Wang et al., 2020). Lipids are important nutrients in milk and originate from cow feed intake and rumen fermentation; they have a complex composition including free fatty acids (FFAs), triacylglycerol (TAG), and polar lipids (Jensen, 2002; Månsson, 2008). Lipid metabolism is one of the metabolic pathways affected by heat stress (Belhadj Slimen et al., 2016). Heat stress has been shown to influence lipogenesis and lipolysis in cow lipid cells (Faylon et al., 2015). Reduced lipid mobilization in fat tissue during a heat challenge has been linked to reduced milk production (Wheelock et al., 2010). The literature is equivocal regarding total milk fat concentration during heat stress. While some studies report a decline in total fat content (Joksimović-Todorović et al., 2011), some show no change (Knapp and Grummer, 1991) or even an increase in total fat concentration for cows under heat stress (Garner et al., 2022). The variation may be due to the length of exposure to heat. Joksimović-Todorović et al. (2011) tested milk from cows during a whole summer period, in contrast to the 15 days of Knapp and Grummer (1991) and the 2–4 days of Garner et al. (2017), Garner et al. (2022), and Williams et al. (2023). Thus, the overall impact of heat stress on milk composition, particularly lipid composition, remains unclear and may be influenced by the severity and duration of heat exposure in addition to nutrition.
While the total fat in milk may not be an accurate indicator of heat stress, there is the potential to identify lipid biomarkers of heat stress in milk or serum. In fact, concentrations of FFAs, TAG, and polar lipids in bovine milk were observed to change under heat stress at 30°C or with a THI of ≥80 for 4–24 days (Bandaranayaka and Holmes, 1976; Tian et al., 2016; Liu et al., 2017), while exposure to a high THI reduced the amount of short- and medium-chain FAs and increased the amount of long-chain FAs in milk (Hammami et al., 2015; Liu et al., 2017). The concentrations of glucosylceramide (GluCer), lysophosphatidylcholine (LPC), phosphatidylcholine (PC), phosphatidylserine (PS), and phosphatidylethanolamine (PE) have been shown to decrease due to heat exposure, with LPC undergoing the largest decrease (Liu et al., 2017). In bovine blood, lysophosphatidylethanolamine (LPE) 18:0 as well as LPC 16:0 and 18:0 increased, whereas PC 16:0/14:0, 14:1/18:3, 12:0/22:2, 15:1/18:2, 20:2/12:0, and 18:1/18:3 reduced during heat stress (Tian et al., 2015). In addition, biomarkers of heat stress in milk were found to correlate with the same biomarkers in blood, for example, linoleic acid, oleic acid, β-hydroxybutyric acid (BHBA), LPC 16:0, and PC 42:2 (Tian et al., 2015; Tian et al., 2016). A 4-day heat challenge was intensive enough to cause physiological and metabolic changes as well as a reduction in milk yield from dairy cows (Garner et al., 2017). The effect of heat exposure for fewer than 4 days is still unclear.
While most of the previous research has focused on the yield and composition of milk as influenced by heat stress, as well as genetic selection to improve the heat tolerance of dairy cows (Bandaranayaka and Holmes, 1976; Garner et al., 2016; Liu et al., 2017), this study aimed to search for potential lipid biomarkers for short-term heat stress. In addition, both milk and serum samples were analyzed for selected lipid classes including SCFAs, a group of active lipids produced by rumen bacterial activity (Shen et al., 2019; Giannuzzi et al., 2022). A targeted lipidomic analysis was adopted using gas chromatography–mass spectrometry (GC-MS) and liquid chromatography–mass spectrometry (LC-MS).
2 Materials and methods
2.1 Milk and serum samples
This study was part of a larger experiment investigating the impact of forage type and amount on dry matter intake (DMI), milk production, and physiological responses during a controlled heat challenge, as reported by Williams et al. (2023). Raw milk and serum were collected at the Department of Jobs, Precincts and Regions Research farm, located in Ellinbank, Victoria, Australia. The experiment received animal ethics approval from the Agricultural Research and Extension Animal Ethics Committee of the Department of Economic Development, Jobs, Transports and Resources, Victoria, Australia (protocol code: 2019-15; approved: 15 October 2019).
Full details of the experiment are described in Williams et al. (2023). In brief, 32 dairy cows were allocated to one of the four diets. Each diet consisted of the same grain mix offered at 5 kg dry matter (DM) per cow/day, consisting of cracked wheat grain, cracked lupins, and a standard mineral mix. The CH-L treatment included freshly cut chicory offered at 10 kg DM/cow/day, CH-H included freshly cut chicory offered at 13 kg DM/cow/day, PS-L included pasture silage offered at 10 kg DM/cow/day, and PS-H included pasture silage offered at 13 kg DM/cow/day. Following 14 days of adaptation to the treatment diets, the cows were placed in individual controlled-environment chambers where they experienced 1 day at thermoneutral conditions (baseline) before 2 days of heat challenge (Garner et al., 2016). The climate-controlled conditions of the individual chambers during the baseline period were set at 20°C and 60% RH (THI 67), and during the heat challenge period, at 30°C and 50% RH between 6:01 and 12:00 (THI 80.1), then at 33°C and 50% RH (THI 84.2) between 12:01 and 18:00, and finally, at 26.5°C and 60% RH (THI 74.5) overnight between 18:01 and 6:00. A total of 28 cows completed the heat event in the climate-controlled chambers in five cohorts, with sick cows (unrelated to the heat challenge) being excluded from the analysis.
Cows were milked twice daily, at approximately 06:00 and about 15:00, and milk yield was measured for each cow at each milking. Feed was offered in two equal portions immediately following the morning and afternoon milkings and refusals were collected and weighed. Milk yield measurements were made by collecting and weighing the milk from individual cows. Samples of milk from each cow were collected at each milking during the baseline period and on day 2 of the heat challenge period. Milk samples were analyzed for fat and protein using a mid-infrared milk analyzer (Bentley FTS, Bentley Instruments, Chaska, MN, USA). Further milk samples of 50 ml were collected and stored in 70-ml specimen containers and stored at −80°C until analysis.
Serum samples were obtained from each cow as described previously (Williams et al., 2023) during the baseline period and on day 2 of the heat challenge. They were stored at −80°C until analysis.
Milk and serum samples from 28 individual cows belonging to four different dietary treatments (six to eight cows per treatment) were analyzed to determine the concentration of selected lipid classes from cows in each dietary treatment, namely, FA profile of milk fat and free SCFA of milk and serum, as well as the major phospholipid and LPC species of milk at the pre-heat challenge period (baseline, THI < 70) and after a 2-day heat challenge (THI > 70).
2.2 Chemicals
The chemicals and solvents used were of chromatographic/analytical grade. SCFA standard mix (containing acetate, propionate, isobutyrate, butyrate, 2-methylbutyrate, isovalerate, and valerate) and SCFA derivatization reagents 3-nitrophenylhydrazine hydrochloride (3-NPH·HCl), 1-ethyl-3-(3-dimethylaminopropyl) carbodiimide (EDC), and pyridine, as well as nonadecane (used as an internal standard for FA analysis by GC-MS) and a standard mixture of 37 FAMEs were purchased from Sigma-Aldrich. The solvents (acetonitrile) used for SCFA sample preparation and LC-MS mobile phase (0.1% formic acid in water and 0.1% formic acid in acetonitrile) were from Fisher Scientific. The chemicals and solvents for FAME preparation were from Ajax FineChem (hexane and sulfuric acid) and Merck (methanol).
2.3 SCFA derivatization
The SCFA (acetate, propionate, butyrate, isovalerate, and valerate) derivatization for both the milk and serum samples was performed as described previously (Li et al., 2022).
2.4 Polar lipid preparation
PC, PE, SM, and LPC were prepared using the one-phase method for milk lipid extraction described by Liu et al. (2016). In brief, 1,000 µL of butanol/methanol/dichloromethane (v/v/v, 3:5:4) mixture was added to 100 µL of three-fold diluted (in Milli-Q H2O) raw milk followed by 20-s vortex, 20-min sonication, and 15-min centrifugation (15,000g) sequentially. The supernatant was then transferred to injection vials and analyzed by LC-MS.
2.5 FAME preparation
The FAMEs were prepared using the direct liquid milk acid-catalyzed methylation described by Liu et al. (2020). In brief, methylation was achieved by adding 2.8 mL of 2% H2SO4 in methanol to 50 µL of raw milk in a 5-mL glass vial tightly sealed with a Teflon-lined cap, then samples were incubated at 60°C for 3 h, with occasional vortexing. When methylation was complete and samples had cooled down to room temperature, 1 mL of Milli-Q water was added, followed by FAME extraction using 1 mL hexane containing 100 mg/L internal standard nonadecane. After phase separation, the upper phase was analyzed by GC-MS.
2.6 LC-MS conditions
Derivatized SCFAs of both milk and serum samples were quantified using the same LC-MS method as described previously (Shen et al., 2019).
PC, PE, SM, and LPC were separated by a Luna Omega C18 column (150 mm × 2.1 mm, 1.6 µm, Phenomenex) on a Vanquish UHPLC system (Thermo Fisher Scientific), with the column compartment and sample tray maintained at 55°C and 15°C, respectively. The mobile phase consisted of water/acetonitrile (40:60, v/v) containing 10 mM ammonium (A) and acetonitrile/isopropanol (10:90, v/v) containing 10 mM ammonium formate (B). The gradient elution was conducted by increasing mobile phase B from 5% to 100% in 25 min and maintaining 100% in the next 3 min. Flow rate and injection volume were 0.25 mL/min and 3 µL, respectively.
PC, PE, SM, and LPC were detected by a Q Exactive Plus mass spectrometer (Thermo Fisher Scientific) with a heated electrospray ionization (HESI) source. Capillary and source heater temperatures were 300°C, and the sheath, auxiliary, and sweep gases were at 30 units, 10 units, and 0 units, respectively. The mass spectrometer was operated in both positive (4.2 kV) and negative (3.6 kV) ionization modes with a full scan (120 m/z–1,800 m/z) at a resolution of 70,000. Phospholipid species were identified using Xcalibur (Thermo Fisher Scientific) based on retention time and accurate mass matching as reported previously (Liu et al., 2017).
2.7 GC-MS conditions
The FAME analysis was performed as described by Liu et al (Li et al., 2022). In brief, FAMEs were separated by an Rt-2560 column (100 m × 0.25 mm ID, 0.20 µm df, Restek) with a 2.25 mL/min flow of carrier gas helium and an oven temperature of 100°C was maintained for 4 min, which increased by 6°C/min to 170°C, then increased by 3°C/min to 240°C, and was maintained for 11 min. Injection inlet temperature and injection volume were 240°C and 1 µL, respectively (split ratio 20:1).
FAMEs were detected by an Agilent 7000 GC-MS Triple Quadrupole with a scanning mass range of 40 amu–500 amu, and transfer line, source, and quad temperatures 240°C, 280°C, and 150°C, respectively. Quantification of methylated FAs was achieved by using the FAME standard mixture containing 37 FAMEs.
2.8 Data analysis
All lipidomic analyses were conducted with six to eight biological replicates (individual cows) and no technical replicates. Unless otherwise indicated, the effect of heat challenge on milk and serum lipids was expressed as the ratio of day 2 heat challenge to the baseline level, and the raw results were subjected to a Student’s t-test (Excel, Microsoft 365) for statistical differences. As the main aim of this study was to investigate the lipidomic change of milk and serum caused by heat stress, the effect of different forage treatments on milk lipids was compared (by t-test) for only free C3 and C4 as well as LPC 16:0 and LPC 18:1 at the baseline level.
3 Results and discussion
3.1 Milk yield and composition after exposure to heat challenge
During the baseline period, the cows experienced a THI of 66 ± 0.6, and during the heat challenge period, they experienced a THI of 76 ± 4.1. The heat challenge induced heat stress symptoms in all cows, as evidenced by increased respiration rates and body temperatures (Williams et al., 2023). There was no overall effect of the 2-day heat challenge on dry matter intake (DMI) or milk yield. However, the feed type and amount affected a range of variables, which are outlined in Table 1. Notably, during the heat challenge, cows offered the pasture silage treatments had a lower milk yield but a greater concentration of total fat in milk during both the baseline period and the heat challenge than cows fed the chicory diets.
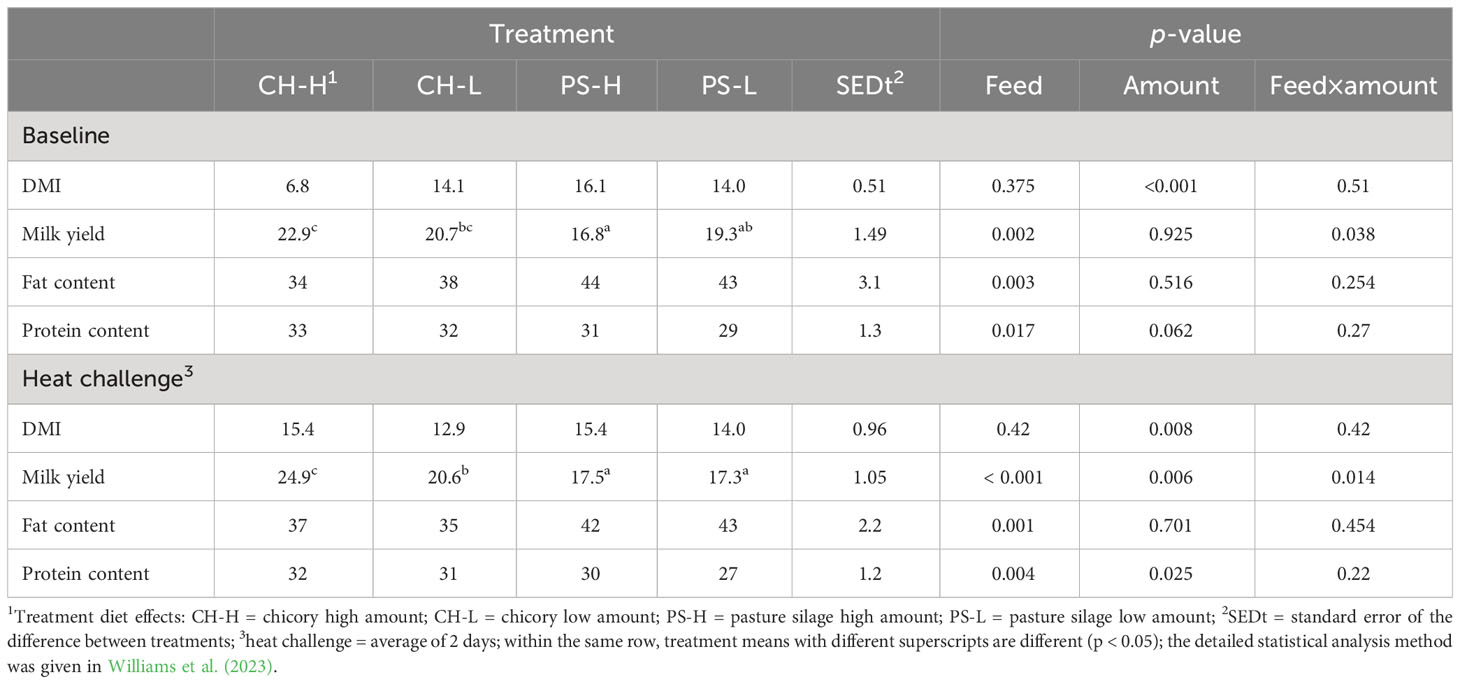
Table 1 DMI, milk yield (kg/d), and milk composition (g/kg) during the thermoneutral baseline period and the 2-day heat challenge period (adapted from Williams et al. (2023)).
3.2 Milk and serum short-chain fatty acid content after exposure to heat challenge
SCFAs are typically derived from bacterial activity in the rumen and may be used as an indicator of animal health status (Shen et al., 2019; Giannuzzi et al., 2022). Our previous study revealed that after a 4-day heat challenge, total butyric acid (C4) content in milk fat was significantly reduced (Liu et al., 2017). The present study found five free SCFAs (acetate, propionate, butyrate, iso-valerate, and valerate) in both milk and serum samples, and all of them displayed a huge variation at the baseline level across individual cows offered the same type and amount of forage, suggesting that free SCFAs are a highly dynamic trait (Figures 1A, B, only propionic acid (C3) and C4 in milk are shown). The fact that higher levels of C3 and C4 were observed with pasture silage also indicates that milk SCFAs are partially influenced by feedstuff. By contrast, neither milk C3 nor C4 was affected by the amount of forage offered within each forage type (p > 0.05).
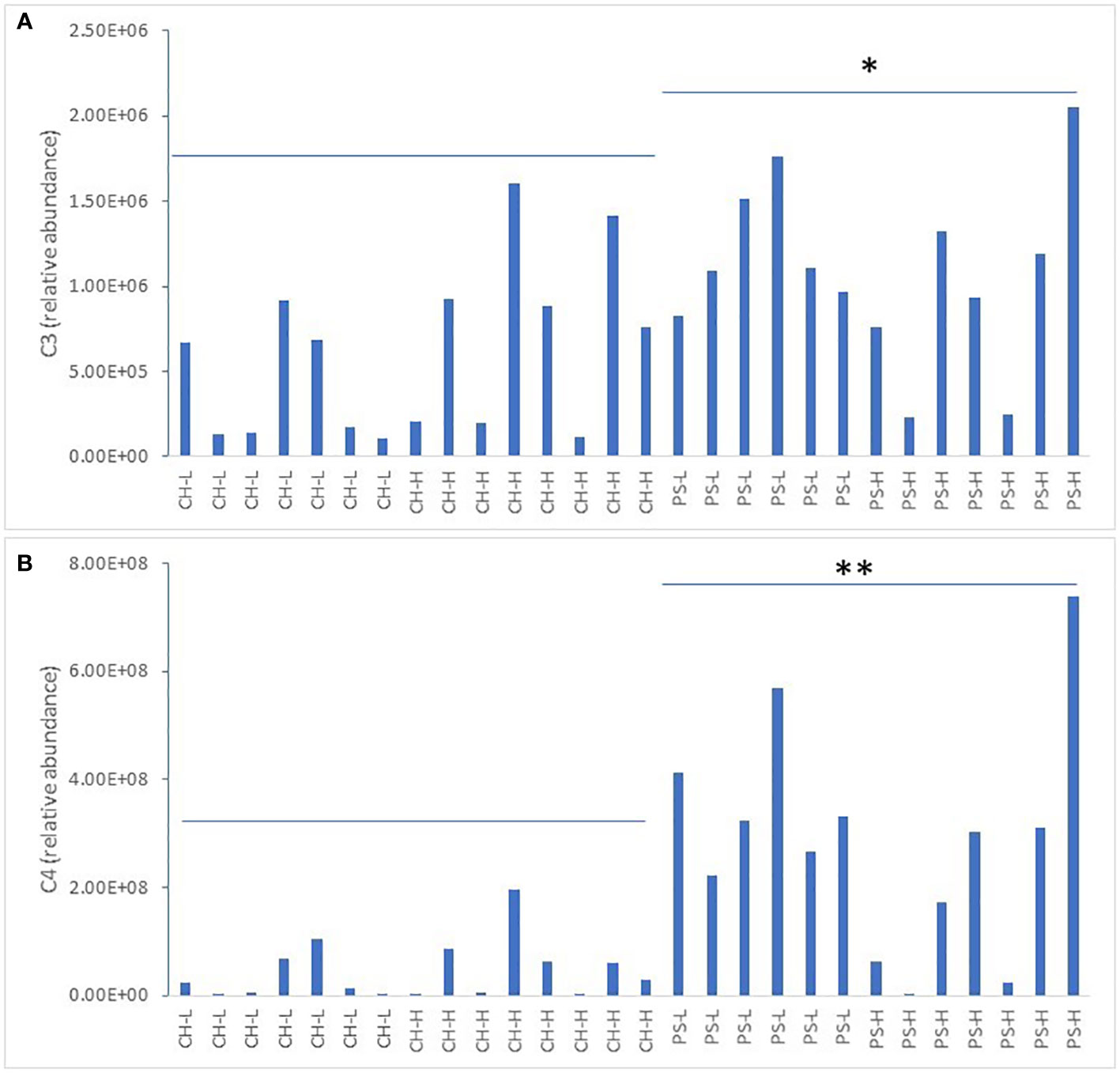
Figure 1 Relative abundance of C3 (A) and C4 (B) in milk at the baseline level. CH-L: chicory low amount; CH-H: chicory high amount; PS-L: pasture silage low amount; PS-H: pasture silage high amount. Note: the overall abundance of C4 is higher than C3 in milk samples. Note: higher abundance of both C3 (* denotes p < 0.05) and C4 (** denotes p < 0.01) with pasture silage compared to chicory.
Due to the large difference in abundance across the SCFAs, a ratio of 2-day heat challenge vs. baseline (2D vs. baseline) was used to estimate the effect of heat stress on milk and serum SCFA content (a ratio equal to 1 indicating no effect, a ratio > 1 indicating an increase of SCFAs by heat stress, and a ratio < 1 indicating a decrease of SCFAs by heat stress). Figures 2A, B show that none of the free SCFAs measured (acetate, propionate, butyrate, and valerate) displayed a consistent pattern after exposure to the heat challenge, regardless of the type and amount of forage offered; the large error bars are caused by the large variation in response among individual cows within each cohort (i.e., increase in some cows but decrease in others).
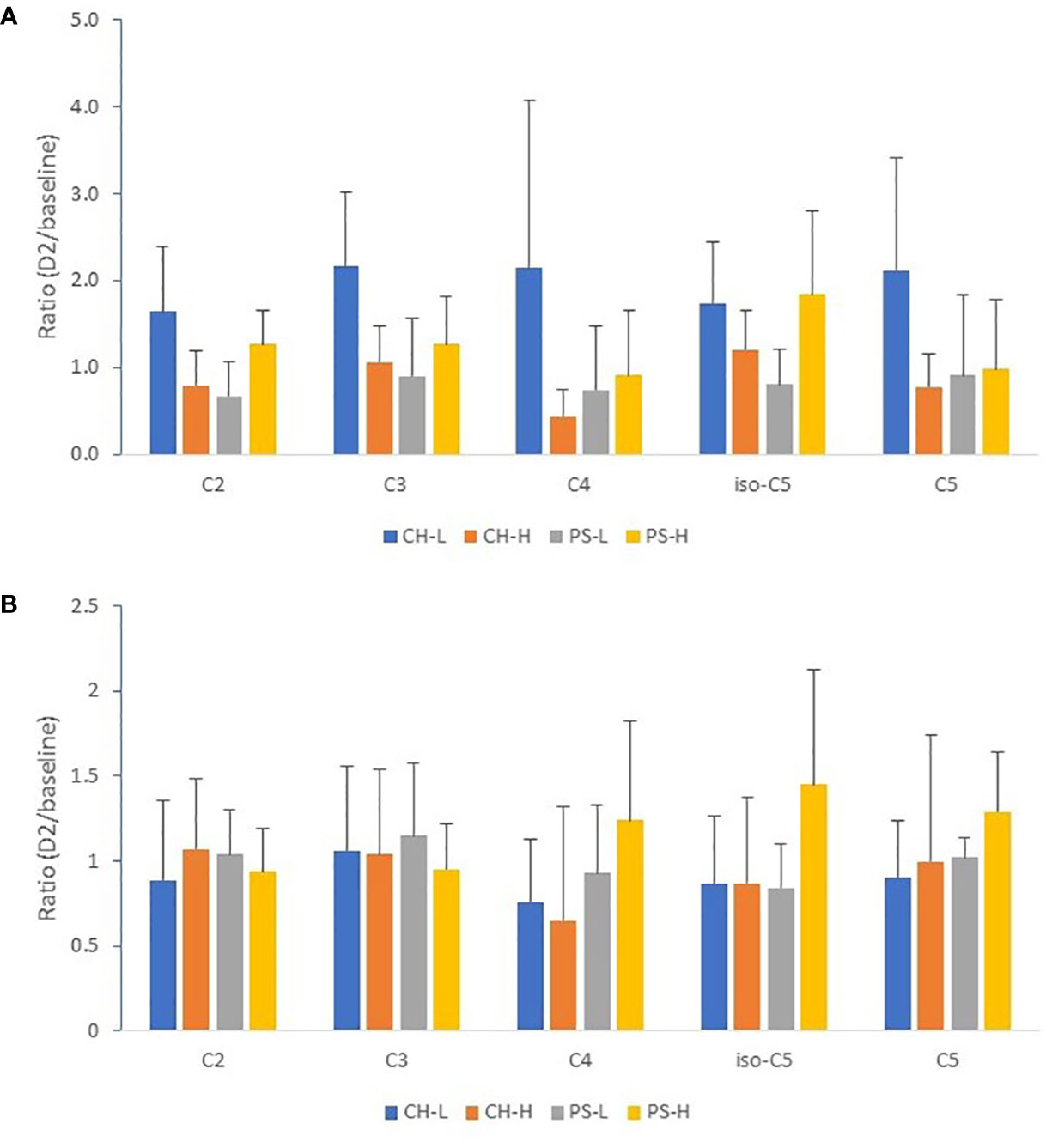
Figure 2 Effect of 2-day heat challenge on SCFA content of milk (A) and serum (B). CH-L: chicory low amount (n = 7); CH-H: chicory high amount (n = 8); PS-L: pasture silage low amount (n = 7); PS-H: pasture silage high amount (n = 6). C2: acetate; C3: propionate; C4: butyrate; iso-C5: isovalerate; C5: valerate. Error bars are standard deviation. Note: no significant difference between the baseline and the 2-day heat stress for all SCFA species and all forage regimes.
Free SCFAs have not been measured before in milk or serum samples collected from heat stress experiments, to our knowledge. The inconsistent response pattern after the heat challenge indicates that these variables are unlikely to be useful biomarkers for the early detection of heat stress in dairy cows. While the large natural between-cow variation of SCFAs in both milk and serum was already observed in our previous study (Li et al., 2022), the irregular response pattern between individual cows to heat challenge was unexpected. Clearly, both milk and serum SCFAs are sensitive indicators of ruminant physiology, but the differential response of experimental animals may be the result of complex interactions between animal genetics, rumen microbiota, and the feedstuff ingested.
3.3 Milk lysophosphatidylcholine content after exposure to heat challenge
LPC showed a much smaller intra-cohort variation between individual cows prior to the heat challenge than SCFAs. Figure 3 shows the relative abundance of LPC 16:0 and LPC 18:1 at the baseline level for all four treatments.
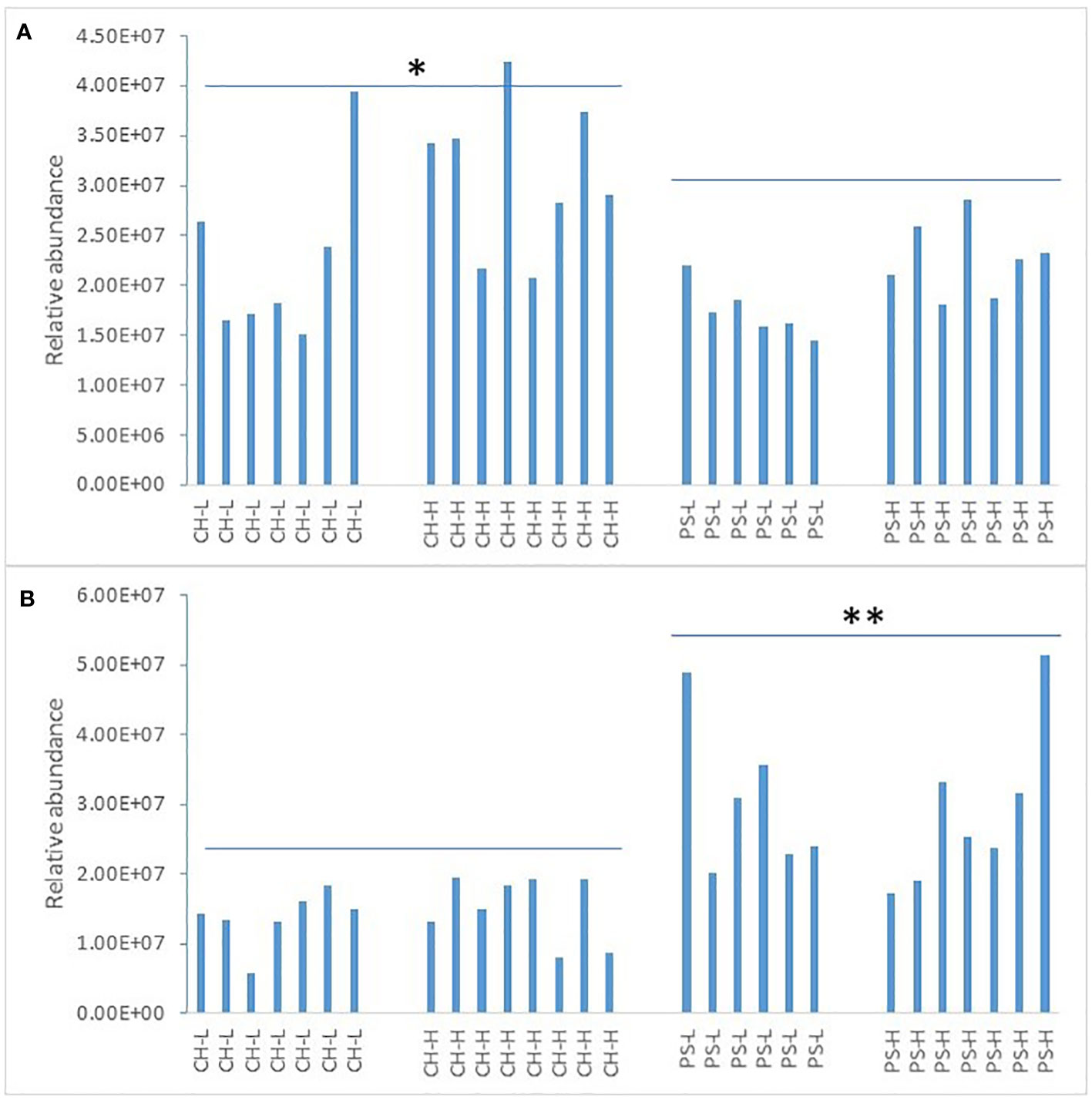
Figure 3 Relative abundance of LPC 16:0 (A) and LPC 18:1 (B) in milk at the baseline level. CH-L: chicory low amount; CH-H: chicory high amount; PS-L: pasture silage low amount; PS-H: pasture silage high amount. Note: higher abundance of LPC 16:0 with chicory (* denotes p < 0.05) and higher abundance of LPC 18:1 with pasture silage (** denotes p < 0.01).
It is interesting to note that a higher abundance of LPC 16:0 was observed for cows offered chicory than for those offered pasture silage (p < 0.05), whereas the opposite trend was found for LPC 18:1 (p < 0.05). It appears that the LPC content of the milk may be influenced by the forage type in the diet of lactating cows. Again, for both LPC species, no significant difference was found between the low and the high amount of forage offered within each forage type. Although the influence of forage type on milk lipid profile was not the focus of this study, these results add further evidence that milk bioactives can be altered by the feeding regime.
After the 2-day heat challenge, overall, no significant reduction was measured for any of the four forage treatments (Figure 4). However, one or two cows in each cohort did record a reduction in LPC content. Results concerning milk LPC content in the event of heat stress are not consistent. For example, our previous work found a substantial decrease in milk LPC after a 4-day heat challenge and a significant reduction even after 2 days of the heat challenge (Liu et al., 2017), whereas an increase in milk LPC 16:0 was observed by Tian et al. (2015) in heat-stressed cows. Consequently, the potential of using LPC as a biomarker for heat stress in dairy cows has not been confirmed in this study. It is known that the diets offered to animals during the current experiment (Williams et al., 2023) were different from those offered in our previous study, which consisted of ad libitum access to compressed cubes containing 75% lucerne hay and 25% crushed barley grain (Garner et al., 2016), and the experiments were conducted with different cows at a different time of the year. However, the main reasons for the inconsistent pattern in milk LPC observed in this study remain unknown.
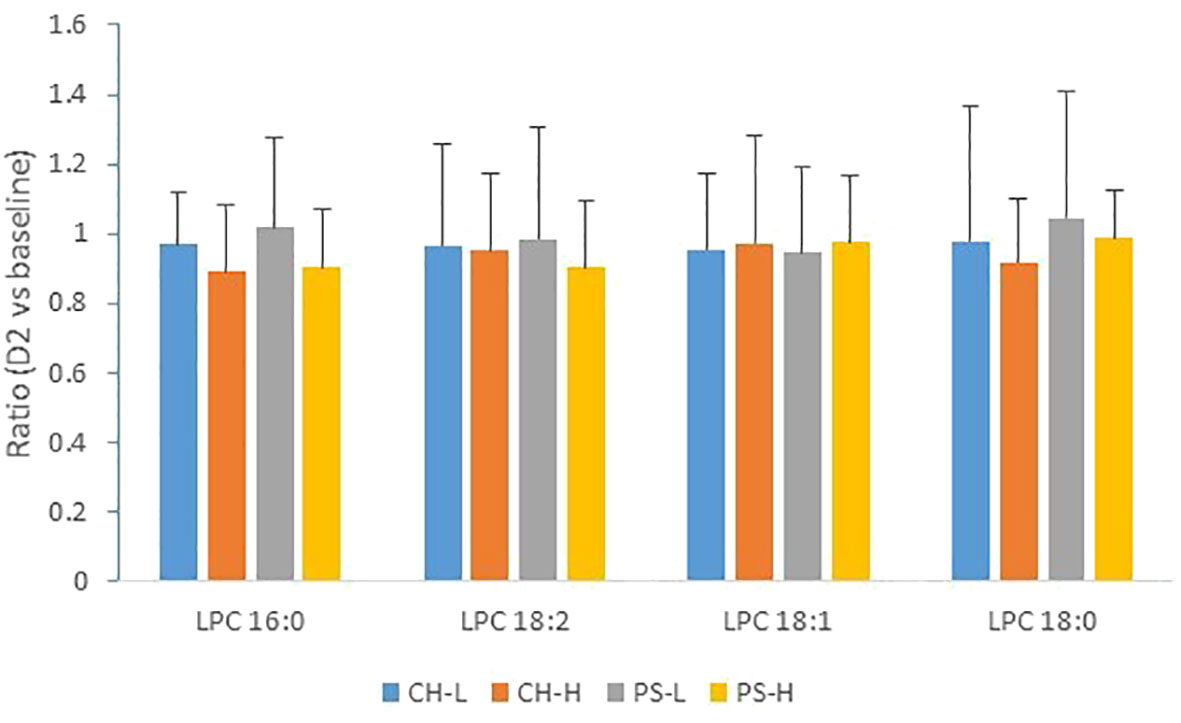
Figure 4 Effect of 2-day heat challenge on LPC content of milk. CH-L: chicory low amount (n = 7); CH-H: chicory high amount (n = 8); PS-L: pasture silage low amount (n = 7); PS-H: pasture silage high amount (n = 6). Error bars are standard deviation. Note: no significant difference between the baseline and the 2-day heat stress for all LPC species and all forage regimes.
3.4 Milk fatty acid profile after exposure to heat challenge
The FA composition of milk fat is the single most important indicator of milk lipid change. There was a significant reduction of C4 in milk on the PS-L diet after the 2-day heat challenge (Figure 5) compared with the baseline level. For the majority of milk FAs, no significant difference was observed between the baseline and 2-day heat stress concentrations, regardless of forage type or amount. It is worth noting that our previous study found a significant reduction of de novo FAs (C4:0 to C15:0) and a concomitant increase in pre-formed FA (C18:0, C18:1, and C18:2) after 4-day heat stress (Liu et al., 2017). Hammami et al. (2015) also proposed C18:1 as a feasible milk biomarker for heat stress. Overall, it appears that a short-term (up to 2 days) heat challenge has little impact on the FA profile of milk fat or the nutritive quality of milk lipids. Indeed, neither the milk yield nor the protein content were negatively affected by this short-term heat challenge (Table 1). All these results suggest that the profitability of dairy farmers in areas that experience short heat events is unlikely to be compromised by acute heat exposure.
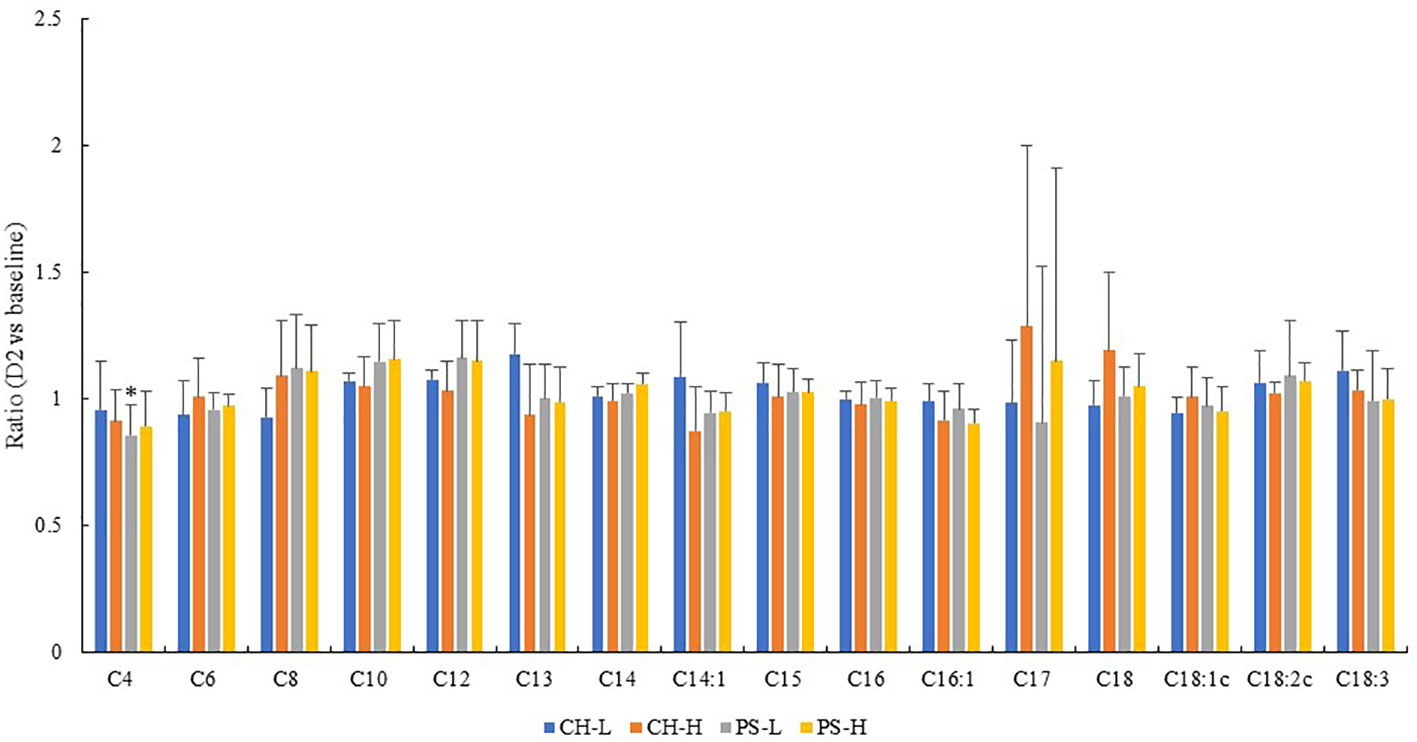
Figure 5 Effect of 2-day heat challenge on FA content of milk. CH-L: chicory low amount (n = 7); CH-H: chicory high amount (n = 8); PS-L: pasture silage low amount (n = 7); PS-H: pasture silage high amount (n = 6). Error bars are standard deviation. * Indicate significant difference between 2-day stress and the baseline (p < 0.05).
3.5 Milk phospholipid content after exposure to heat challenge
Phospholipids are one kind of polar lipid in bovine milk. The major phospholipids of milk PC, PE, and SM were surveyed in this study to determine the possible effect of short-term heat stress on these lipids. Figure 6 shows that, compared with the baseline level, 2-day heat stress did not cause any significant reduction in the abundance of any of the major species of PC, PE, or SM. However, a significant forage type effect (not the amount) on the abundance of some phospholipid species (e.g., PC 34:1 and PC 36:2) was observed. This suggests that short-term heat stress of up to 2 days has no negative impact on phospholipid accumulation in milk. By contrast, our previous study found that a 4-day heat stress test significantly reduced the PC and PE contents of milk (Liu et al., 2017), and a reduction of some PC species in milk was also reported by Tian et al. (2015) in heat-stressed cows.
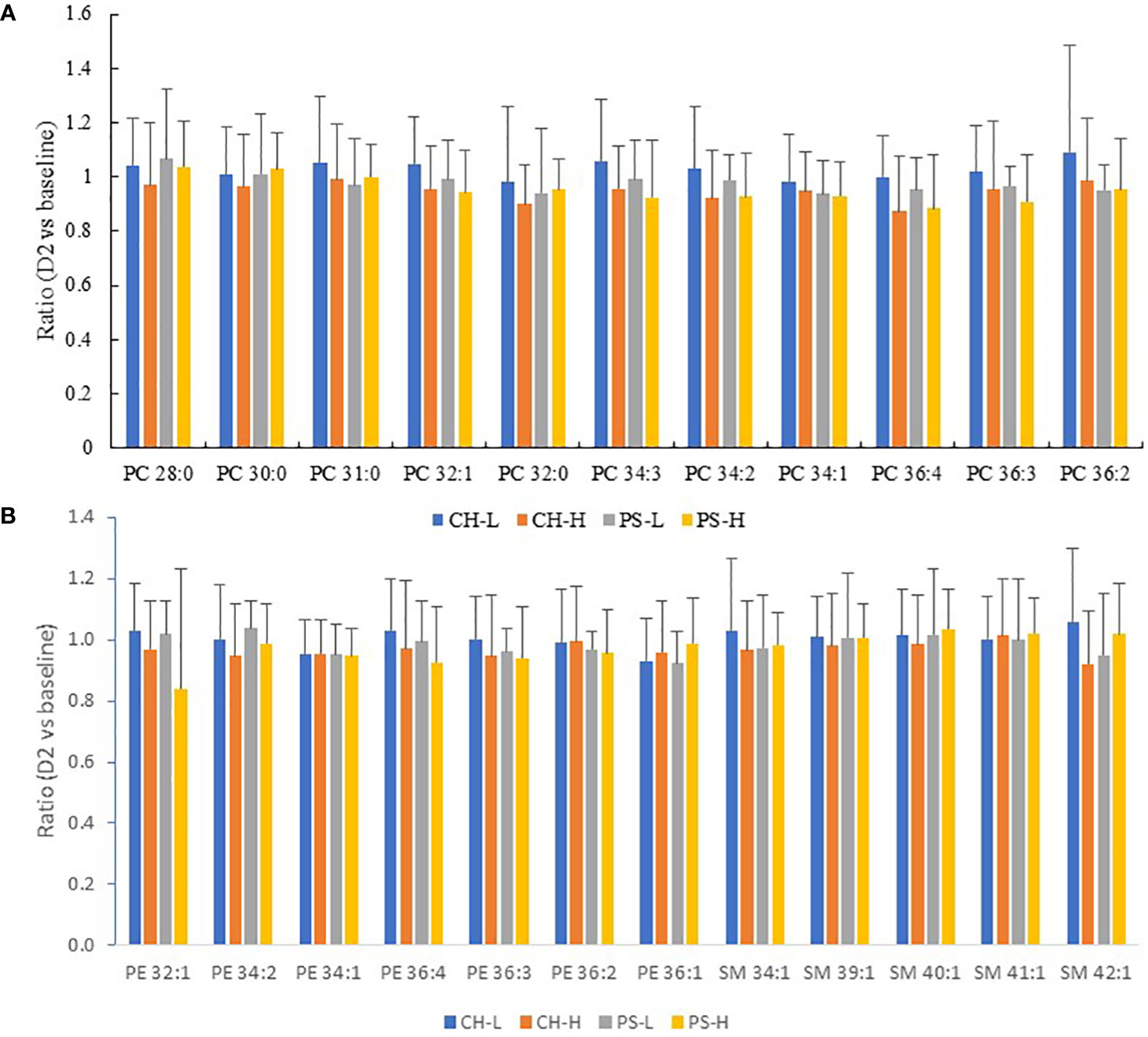
Figure 6 Effect of 2-day heat challenge on PC (A) and PE and SM (B) content of milk. CH-L: chicory low amount (n = 7); CH-H: chicory high amount (n = 8); PS-L: pasture silage low amount (n = 7); PS-H: pasture silage high amount (n = 6). Error bars are standard deviation. Note: no significant difference between the baseline and the 2-day heat stress test for all PC/PE/SM species and all forage regimes.
Phospholipids are bioactive molecules that have multiple beneficial functions for human health (Küllenberg et al., 2012; Contarini and Povolo, 2013; Rodríguez-Alcalá et al., 2017; Ortega-Anaya and Jiménez-Flores, 2019). The lack of change in the lipid composition of milk due to heat exposure indicates that there is likely no negative impact of heat stress on the human health outcomes of cow’s milk consumption.
Based on physiological parameters and milk yield, Williams et al. (2023) concluded that cows offered chicory were less affected by the 2-day heat challenge (greater milk yield and lower body temperature) than those offered pasture silage. However, our study found only small differences in the milk lipid composition in the milk and serum of cows offered chicory compared with those offered pasture silage during a 2-day heat challenge. There was no difference in milk or serum lipid profiles that could be attributed to the heat challenge or the amount of feed offered to cows.
4 Conclusion
With global warming, both acute and prolonged heat stress is expected to become more and more frequent, affecting animal welfare and production. Although a 2-day heat challenge caused some physiological stress in dairy cows, neither milk fat FA composition nor the content of phospholipids recorded any significant change due to the heat challenge. Therefore, 2-day heat stress is unlikely to have any adverse effects on the nutritional quality of milk for human consumption. Both SCFAs and LPC in milk appear to be associated with the forage type offered to dairy cows, but the potential application of these active lipid molecules as sensitive biomarkers for heat stress was not confirmed in this study. Further study should focus on untargeted lipidomic analysis to reveal the global lipidome change induced by acute heat stress.
Data availability statement
The original contributions presented in the study are included in the article/supplementary material. Further inquiries can be directed to the corresponding author.
Ethics statement
Cows were cared for in accordance with the Australian Code of Practice for the Care and Use of Animals for Scientific Purposes (NHMRC, 2013). The animal study protocol was approved by the DJPR Agricultural Research & Extension Animal Ethics Committee (Protocol code: 2019–15, Approved: 15 October 2019).
Author contributions
Conceptualization, ZL and SR; investigation, CL; animal management and sample collection, LM and CL; writing, CL and ZL; editing and reviewing, CB, LM, JP, and SR; funding acquisition, LM, JP, and SR. All authors contributed to the article and approved the submitted version.
Funding
This investigation was part of the Dairy Feedbase research program funded by Agriculture Victoria, Dairy Australia, and Gardiner Foundation.
Conflict of interest
The authors declare that the research was conducted in the absence of any commercial or financial relationships that could be construed as a potential conflict of interest.
Publisher’s note
All claims expressed in this article are solely those of the authors and do not necessarily represent those of their affiliated organizations, or those of the publisher, the editors and the reviewers. Any product that may be evaluated in this article, or claim that may be made by its manufacturer, is not guaranteed or endorsed by the publisher.
References
Bandaranayaka D. D., Holmes C. W. (1976). Changes in the composition of milk and rumen contents in cows exposed to a high ambient temperature with controlled feeding. Trop. Anim. Health Prod. 8, 38–46. doi: 10.1007/BF02383364
Belhadj Slimen I., Najar T., Ghram A., Abdrrabba M. (2016). Heat stress effects on livestock: Molecular, cellular and metabolic aspects, a review. J. Anim. Physiol. Anim. Nutr. 100, 401–412. doi: 10.1111/jpn.12379
Contarini G., Povolo M. (2013). Phospholipids in milk fat: Composition, biological and technological significance, and analytical strategies. Int. J. Mol. Sci. 14, 2808–2831. doi: 10.3390/ijms14022808
Das R., Sailo L., Verma N., Bharti P., Saikia J., Imtiwati, et al. (2016). Impact of heat stress on health and performance of dairy animals: A review. Vet. World 9, 260–268. doi: 10.14202/vetworld.2016.260-268
Faylon M. P., Baumgard L. H., Rhoads R. P., Spurlock D. M. (2015). Effects of acute heat stress on lipid metabolism of bovine primary adipocytes. J. Dairy Sci. 98, 8732–8740. doi: 10.3168/jds.2015-9692
Garner J. B., Douglas M., Williams S. R. O., Wales W. J., Marett L. C., DiGiacomo K., et al. (2017). Responses of dairy cows to short-term heat stress in controlled-climate chambers. Anim. Prod. Sci. 57, 1233–1241. doi: 10.1071/AN16472
Garner J. B., Douglas M. L., Williams S. R. O., Wales W. J., Marett L. C., Nguyen T. T. T., et al. (2016). Genomic selection improves heat tolerance in dairy cattle. Sci. Rep. 6, 34114. doi: 10.1038/srep34114
Garner J. B., Williams S. R. O., Moate P. J., Jacobs J. L., Hannah M. C., Morris G. L., et al. (2022). Effects of heat stress in dairy cows offered diets containing either wheat or corn grain during late lactation. Anim. (Basel) 12, 2031. doi: 10.3390/ani12162031
Giannuzzi D., Toscano A., Pegolo S., Gallo L., Tagliapietra F., Mele M., et al. (2022). Associations between milk fatty acid profile and body condition score, ultrasound hepatic measurements and blood metabolites in Holstein cows. Animals 12, 1202. doi: 10.3390/ani12091202
Hammami H., Vandenplas J., Vanrobays M. L., Rekik B., Bastin C., Gengler N. (2015). Genetic analysis of heat stress effects on yield traits, udder health, and fatty acids of walloon holstein cows. J. Dairy Sci. 98, 4956–4968. doi: 10.3168/jds.2014-9148
Hempel S., Menz C., Pinto S., Galán E., Janke D., Estellés F., et al. (2019). Heat stress risk in european dairy cattle husbandry under different climate change scenarios – uncertainties and potential impacts. Earth Syst. Dynam. 10, 859–884. doi: 10.5194/esd-10-859-2019
Jensen R. G. (2002). The composition of bovine milk lipids: January 1995 to december 2000. J. Dairy Sci. 85, 295–350. doi: 10.3168/jds.S0022-0302(02)74079-4
Joksimović-Todorović M., Davidović V., Hristov S., Stanković B. (2011). Effect of heat stress on milk production in dairy cows. Biotechnol. Anim. Husb. 27, 1017–1023. doi: 10.2298/BAH1103017J
Kadzere C. T., Murphy M. R., Silanikove N., Maltz E. (2002). Heat stress in lactating dairy cows: A review. Livest. Prod. Sci. 77, 59–91. doi: 10.1016/S0301-6226(01)00330-X
Knapp D. M., Grummer R. R. (1991). Response of lactating dairy cows to fat supplementation during heat stress. J. Dairy Sci. 74, 2573–2579. doi: 10.3168/jds.S0022-0302(91)78435-X
Küllenberg D., Taylor L. A., Schneider M. (2012). Massing, U. Health effects of dietary phospholipids. Lipids Health Dis. 11, 3. doi: 10.1186/1476-511X-11-3
Li C., Liu Z., Bath C., Marett L., Pryce J., Rochfort S. (2022). Optimised method for short-chain fatty acid profiling of bovine milk and serum. Molecules 27, 436. doi: 10.3390/molecules27020436
Liu Z., Ezernieks V., Wang J., Arachchillage N. W., Garner J. B., Wales W. J., et al. (2017). Heat stress in dairy cattle alters lipid composition of milk. Sci. Rep. 7, 961. doi: 10.1038/s41598-017-01120-9
Liu Z., Rochfort S., Cocks B. G. (2016). Optimization of a single phase method for lipid extraction from milk. J. Chromatogr. A 1458, 145–149. doi: 10.1016/j.chroma.2016.06.055
Liu Z., Wang J., Li C., Rochfort S. (2020). Development of one-step sample preparation methods for fatty acid profiling of milk fat. Food Chem. 315, 126281. doi: 10.1016/j.foodchem.2020.126281
Månsson H. L. (2008). Fatty acids in bovine milk fat. Food Nutr. Res. 52, 1821. doi: 10.3402/fnr.v52i0.1821
Ortega-Anaya J., Jiménez-Flores R. (2019). Symposium review: The relevance of bovine milk phospholipids in human nutrition—evidence of the effect on infant gut and brain development. J. Dairy Sci. 102, 2738–2748. doi: 10.3168/jds.2018-15342
Osei-Amponsah R., Dunshea F. R., Leury B. J., Cheng L., Cullen B., Joy A., et al. (2020). Heat stress impacts on lactating cows grazing Australian summer pastures on an automatic robotic dairy. Animals 10, 869. doi: 10.3390/ani10050869
Polsky L., von Keyserlingk M. A. G. (2017). Invited review: Effects of heat stress on dairy cattle welfare. J. Dairy Sci. 100, 8645–8657. doi: 10.3168/jds.2017-12651
Rodríguez-Alcalá L. M., Castro-Gómez M. P., Pimentel L. L., Fontecha J. (2017). Milk fat components with potential anticancer activity—a review. Biosci. Rep. 37, 37. doi: 10.1042/BSR20170705
Shen H., Xu Z., Shen Z., Lu Z. (2019). The regulation of ruminal short-chain fatty acids on the functions of rumen barriers. Front. Physiol. 10, 1305. doi: 10.3389/fphys.2019.01305
Sunil Kumar B. V., Kumar A., Kataria M. (2011). Effect of heat stress in tropical livestock and different strategies for its amelioration. J. Stress Physiol. Biochem. 7, 45–54.
Tian H., Wang W., Zheng N., Cheng J., Li S., Zhang Y., et al. (2015). Identification of diagnostic biomarkers and metabolic pathway shifts of heat-stressed lactating dairy cows. J. Proteomics 125, 17–28. doi: 10.1016/j.jprot.2015.04.014
Tian H., Zheng N., Wang W., Cheng J., Li S., Zhang Y., et al. (2016). Integrated metabolomics study of the milk of heat-stressed lactating dairy cows. Sci. Rep. 6, 24208. doi: 10.1038/srep24208
Wang J., Li J., Wang F., Xiao J., Wang Y., Yang H., et al. (2020). Heat stress on calves and heifers: A review. J. Anim. Sci. Biotechnol. 11, 1–79. doi: 10.1186/s40104-020-00485-8
West J. W. (2003). Effects of heat-stress on production in dairy cattle. J. Dairy Sci. 86, 2131–2144. doi: 10.3168/jds.S0022-0302(03)73803-X
Wheelock J. B., Rhoads R. P., Vanbaale M. J., Sanders S. R., Baumgard L. H. (2010). Effects of heat stress on energetic metabolism in lactating holstein cows. J. Dairy Sci. 93, 644–655. doi: 10.3168/jds.2009-2295
Keywords: milk, serum, fatty acids, short-chain fatty acids, phospholipids, heat stress
Citation: Li C, Liu Z, Bath C, Marett LC, Pryce J and Rochfort S (2023) The effect of 2-day heat stress on the lipid composition of bovine milk and serum. Front. Anim. Sci. 4:1212904. doi: 10.3389/fanim.2023.1212904
Received: 27 April 2023; Accepted: 23 August 2023;
Published: 14 September 2023.
Edited by:
Pasquale De Palo, University of Bari Aldo Moro, ItalyCopyright © 2023 Li, Liu, Bath, Marett, Pryce and Rochfort. This is an open-access article distributed under the terms of the Creative Commons Attribution License (CC BY). The use, distribution or reproduction in other forums is permitted, provided the original author(s) and the copyright owner(s) are credited and that the original publication in this journal is cited, in accordance with accepted academic practice. No use, distribution or reproduction is permitted which does not comply with these terms.
*Correspondence: Zhiqian Liu, WmhpcWlhbi5saXVAYWdyaWN1bHR1cmUudmljLmdvdi5hdQ==