- 1Agroecosystems Management Research Unit, USDA-ARS National Laboratory for Agriculture and the Environment, Ames, IA, United States
- 2Department of Animal Science, Iowa State University, Ames, IA, United States
Heat stress (HS) negatively impacts human health, as well as animal agriculture. The mechanisms underlying HS-induced intestinal dysfunction in vivo are still not fully elucidated. However, HS has been shown to cause intestinal ischemia/hypoxia, which contributes to reduced barrier integrity. The objective of this study was to examine hypoxia alone, HS alone, and a combination using IPEC-J2 cells. We hypothesized that hypoxia is a critical factor and important step in the pathway to HS-induced barrier dysfunction. Porcine IPEC-J2 cells were grown in Transwell™ plates and then treated either under thermal neutral (TN; 38°C) or heat stress (HS; 42°C) and either normoxia (NX; ~21% O2) or hypoxia (HX; 1% O2) conditions for 24 h. Transepithelial electrical resistance, paracellular permeability marker, FITC-dextran, media interleukin 8, cell HSP70 and 90, CLDN4, ZO-1, and EEA1 were all analyzed. Results showed that HS did not increase intestinal permeability in this model and elicited a reduction in IL-8 while still exhibiting a robust HSP response. In this model, hypoxia was required to induce intestinal barrier dysfunction and TJ redistribution. The combination of HS and hypoxia caused even more severe tight junction disruption. This was accompanied by the absence of an IL-8 response under HS.
Introduction
Heat stress negatively impacts human health, as well as animal agriculture (Pearce et al., 2013b; Ghulam Mohyuddin et al., 2022; Rosinger, 2023). Heatwaves and heat-stroke can kill humans and animals, and there are still no standard treatments available aside from cooling and hydration therapies (Hutchins et al., 2022; Wood et al., 2022). Combined with reduced feed intake, HS markedly impacts animal gastrointestinal tract integrity. Previous studies have shown that HS reduces intestinal integrity (Pearce et al., 2013a; Vásquez et al., 2022) in pigs, however the mechanisms underlying the cause are still not fully elucidated. Heat stress has been shown to cause intestinal ischemia/hypoxia as blood is partitioned to the periphery to aid in cooling. The result of this redistribution of blood flow is a lack of blood flow to internal organs, including the gastrointestinal tract. Decrements in intestinal integrity under HS have been observed previously by our group when core temperatures reach 41–41.8°C (Pearce et al., 2013a; Pearce et al., 2014; Pearce et al., 2015) for between 6 and 24 hours. Evidence of hypoxia has also been shown in cases of environmental hyperthermia (Pearce et al., 2013a). The gastrointestinal tract requires an extremely balanced gradient of oxygen from the luminal to the mucosal surface, thus the GI tract is one of the most sensitive organs to changes in oxygenation (Konjar et al., 2021; Lian et al., 2021). Heat shock protein expression has been shown to be a key regulator of the hyperthermic response (Hu et al., 2022). Hypoxia and oxidative stress have been separately proven to induce alterations in tight junction complexes, which results in epithelial dysregulation and increased permeability. There is a clear link between environmental hyperthermia and intestinal permeability, although the role of hypoxia is still not fully understood. However, it is known that the main transcription factor involved in the hypoxic response (hypoxia-inducible factor 1, or HIF-1α) can interact with tight junction proteins including claudins (Della Rocca et al., 2022).
Few studies have examined the combined effects of HS and hypoxia in vitro, and these have been done in human cell lines under acute conditions and normal human body temperatures for controls (Lian et al., 2021; Lian et al., 2023). The current knowledge base is still quite new. The IPEC-J2 cell line, which is a non-transformed small intestinal cell line derived from neonatal pigs, has been shown to be a good model to study nutrition (Rhoads et al., 1994; Yan and Ajuwon, 2017), bacterial invasion (Duan et al., 2022), viral infection (Guo et al., 2022), toxins (Li et al., 2022), and environmental factors such as HS (Cao et al., 2016) and hypoxia (Chapman et al., 2012).
Mechanisms underlying barrier dysfunction and the reduced integrity of tight junctional complexes due to HS are not fully understood; however, several pathways have been implicated. It is also known that hypoxia and HS-related transcription factors regulate the intestinal barrier and inflammatory signaling pathway. Therefore, our objective was to use an agriculturally relevant in vitro intestinal epithelial model to examine the effects of hypoxia and high ambient heat (i.e., HS) on barrier integrity, as well as tight junction protein localization and internalization. We hypothesized that hypoxia is a critical factor and important step in the pathway and hypoxia alone would result in tight junction protein remodeling.
Materials and methods
Cell lines
IPEC-J2 cells were obtained from the Leibniz Institute (DSMZ; Braunschweig, Germany). Cells were cultured in a humidified incubator at 38°C under 5% CO2 in a 25-cm2 cell culture flask (Corning Inc., Corning, NY). Cells were grown at 38°C, as this more closely mimics pigs’ normal body temperatures in vivo but is not far from the standard culture condition of 37°C. Growth media consisted of Dulbecco’s modified Eagle’s medium: Nutrient Mixture F-12 (DMEM/F12; Sigma-Aldrich, St. Louis, MO) with 5% fetal bovine serum (FBS; Sigma-Aldrich, St. Louis, MO) supplemented with 1% insulin–transferrin–selenium premix (ITS premix: human recombinant insulin 1 mg/mL, transferrin 0.6 mg/mL, and selenium 0.6 μg/mL; Corning Inc., Corning, NY), 5 ng/mL epidermal growth factor (EGF: Sigma-Aldrich, St. Louis, MO), and 1% penicillin–streptomycin mixture (Sigma-Aldrich, St. Louis, MO). Cells were initially cultured in 75-cm2 flasks until enough cells were obtained to transfer to 12-well Transwell™ plates (Corning, Corning, NY). Transepithelial electrical resistance (TEER) was measured daily until cells became confluent and stabilized (after approximately 14 days) prior to treating.
Treatments
Cells were treated under thermal neutral (TN; 38°C) or heat stress (HS; 42°C) conditions and subjected to either normoxia (NX; approximately 21% O2) or hypoxia (HX; 1% O2) for 24 hours. The treatment set-up is labelled as follows for figures: thermal neutral in normoxia (TN NX), thermal neutral in hypoxia (TN HX), heat stress in normoxia (HS NX), and heat stress in hypoxia (HS HX). Hypoxia treatments were conducted using a hypoxia incubator chamber (Stem Cell Technologies, Cambridge, MA) with a mixed gas tank consisting of 1% O2, 5% CO2, and 94% N2). To minimize exposure to ambient O2 during sampling, samples were collected as quickly as possible. Transepithelial electrical resistance (TEER) was measured every 6 hours, whereas all other measures were end-point assays at 24 hours. Cell lysate was collected for Western blot analysis, and basolateral media was collected for cytokine and FITC-dextran assays. Immunofluorescence was conducted using the Transwell™ membranes.
Functional assays
TEER was measured every 6 hours using an epithelial Volt/Ohm meter (EVOM2; World Prevision Instruments, Sarasota, FL). At the end of TEER measurement, 0.2 mg/mL of 4-kDa fluorescein isothiocyanate-dextran (FITC-dextran) was applied to the apical side of Transwell™ inserts to measure the paracellular permeability. The concentration of FITC-dextran at the basolateral side was measured using a fluorescent plate reader (BioTek Instruments, Santa Clara, CA) with excitation and emission wavelengths of 485 and 530 nm, respectively.
Immunofluorescence
IPEC-J2 membranes were permeabilized with 0.5% Triton for 10 minutes, blocked with 10% bovine serum albumin for 2 hours, and incubated with primary antibodies at a 1:200 dilution at 4°C overnight. Secondary antibodies were incubated for 2 hours, and DAPI was also applied for 10 mins at room temperature. Early endosomal antigen-1 (EEA1; Santa Cruz Biotechnology), zonula occluden 1 (ZO-1; Invitrogen), and claudin-4 (CLDN4; Invitrogen) were analyzed. Images were obtained with a fluorescent microscope (Olympus, Waltham, MA) and processed in FIJI (NIH). Corrected total cell fluorescence (CTCF) was used as a quantitative output for fluorescence images and calculated as follows: CTCF = integrated density – (area of selected cell × mean fluorescence of background readings). To examine the co-localization of CLDN4 and EEA1, Pearson’s coefficients were generated using the Coloc 2 Plug-in in FIJI.
Western blotting
Protein from cells was extracted in a PBS +1% Triton X-100 buffer with protease and phosphatase inhibitors. Cell homogenates were separated by SDS (10%–15%) polyacrylamide gel electrophoresis (SDS-PAGE). Gels were run under reducing conditions and transferred to nitrocellulose membranes. Membranes were blocked for 1 hour in 5% non-fat dry milk (NFDM) in TBST (1× TBS, 0.1% Tween-20). Membranes were then blocked in primary antibody with 5% NFDM in TBST overnight. After blocking in primary antibody (HSP70 and HSP90) membranes were incubated in secondary antibody for 1 hour. For detection, Supersignal® West Pico Chemiluminescent Substrate was used (Thermoscientific, Waltham, MA). Membranes were imaged using FOTO Analyst® Luminary/FX® (Fotodyne Inc, Hartland, WI). Band densities were quantified by densitometry using TotalLab Quant (Total Lab®, Newcastle Upon Tyne, UK). Bands were standardized to the density of GAPDH and represented as a ratio of each protein to GAPDH.
ELISA
Interleukin-8 was measured using a commercially available porcine kit (Quantikine; R&D Systems, Minneapolis, MN).
Statistical analysis
Experiments were analyzed in JMP SAS (Version 9.2, SAS institute). Individual wells were used as technical replicates. Data were analyzed using one-way ANOVA with the Tukey–Kramer adjustment for pairwise comparisons. TEER was analyzed as repeated measures. Data are presented as least square means ± standard error of the mean. Means not sharing any letter are significantly different by the Tukey–Kramer test p < 0.05. Image J was used to calculate Pearson’s r value for co-localization of CLDN4 and EEA1.
Results
To assess changes in IPEC barrier integrity, Transwell™ TEER was analyzed as a measure of paracellular permeability between adjacent epithelial cells. It was measured as the change from the baseline of the control (TN NX), which was set to 100%, with a higher number indicating better barrier integrity and lower permeability. Unexpectedly, HS alone (HS NX) increased TEER by more than 100% over 24 hours, whereas hypoxia alone (TN HX) and HS combined with hypoxia (HS HX) reduced TEER by approximately 50% (p<0.05; Figure 1A). Substantial changes in TEER were observed starting after approximately 12 hours of treatment. These results indicate that hypoxia was required to reduce TEER. FITC-dextran, another marker for paracellular permeability was increased by 133% in the TN HX treatment compared with TN NX control and HS NX treatment (p<0.05; Figure 1B). FITC-dextran was highest in the HS HX group, increasing by more than 500% compared with the TN NX controls and by nearly 200% compared with the TN HX treatment (p<0.05; Figure 1B). Similar to the findings concerning TEER, hypoxia was required to increase permeability using the FITC-dextran marker. Interestingly, one of the major mediators of the intestinal inflammatory response, basolateral chemokine IL-8, was reduced by more than 70% in both HS treatments (p<0.05; Figure 1C). There was a tendency for IL-8 to be increased due to hypoxia alone (p<0.10; Figure 1C). These results indicate a dampened IL-8 response to HS.
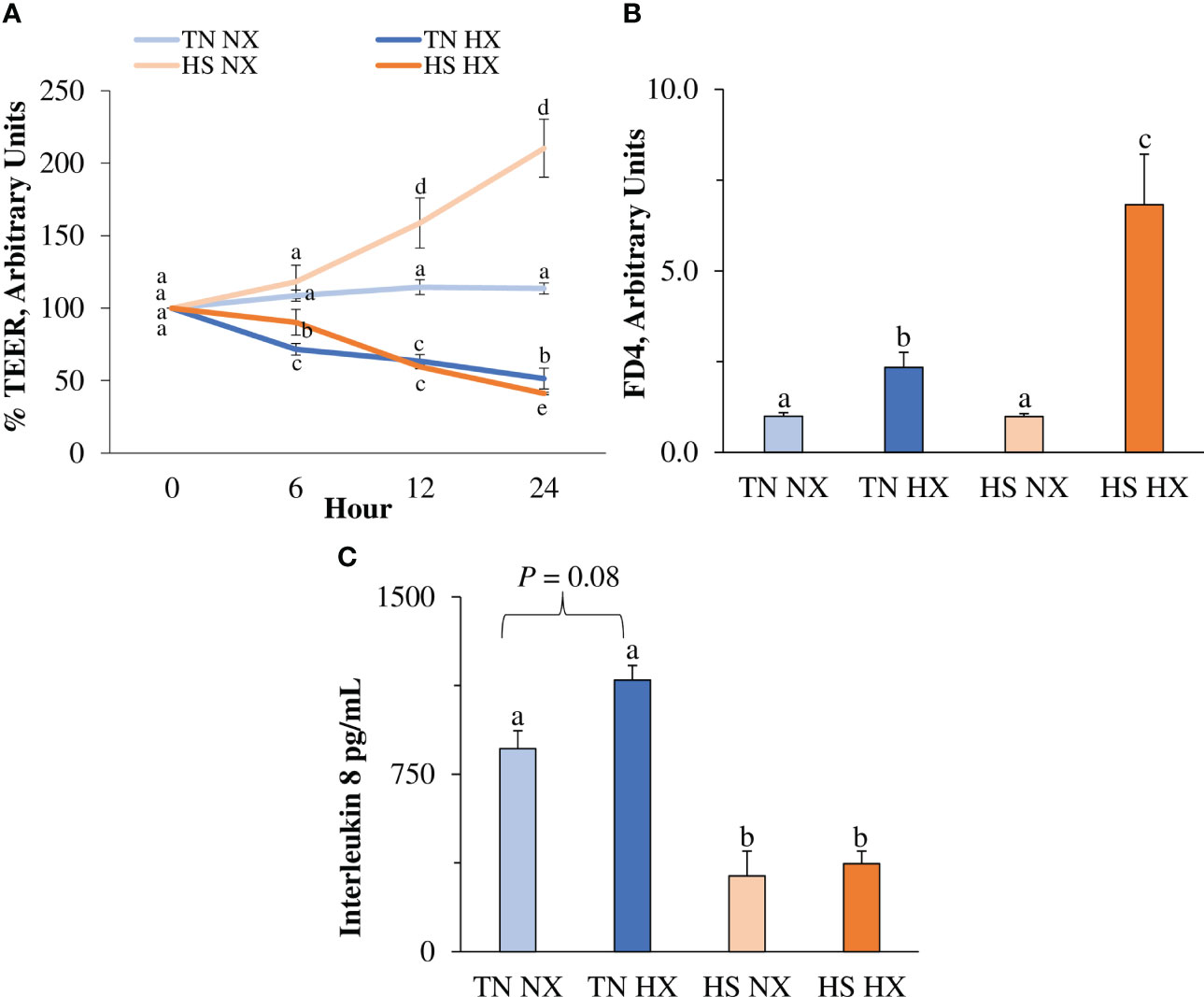
Figure 1 The effects of 24 hours of either constant thermal neutral conditions under normoxia (TN NX; 21°C), constant thermal neutral conditions under 1% hypoxia (TN HX), heat stress conditions under normoxia (HS NX; 42°C), or heat stress conditions under 1% hypoxia (HS HX) on (A) transepithelial electrical resistance (TEER), (B) FITC-dextran (FD4) transport, and (C) basolateral media interleukin 8 concentrations. a,b,c,dp<0.05, n = 12/trt.
The immunofluorescence distribution and structure of CLDN4 were significantly affected by the heat stress and hypoxia treatments. This included areas of redistribution of CLDN4 (white arrows; Figure 2A). CLDN4 appeared to redistribute from the membrane to the cytosol and formed cluster-like structures. In addition, total relative fluorescence (CTCF) was only significantly increased by 34% in the HS HX group relative to the CON group (p<0.05; Figure 2B). Although not different from the CON group, the TN HX group had significantly less CLDN4 than the HS NX group (30% decrease; p<0.05). The distribution and structure of the tight junction protein ZO-1 was largely unchanged due to treatment; however, there were some areas in which ZO-1 appears to cluster like CLDN4 (white arrows; Figure 2A). However, the total amount of ZO-1 was significantly altered. Compared with the CON group, TN HX-treated cells experienced a 31% decrease in ZO-1 fluorescence (p<0.05; Figure 2B). ZO-1 in both HS groups was significantly increased by nearly 20% compared with the CON group (p<0.05; Figure 2B). EEA1 was largely not present in the CON group but was present in significant amounts in the HS HX treatment (Figure 2A). However, total fluorescence was increased in both HS-treated groups compared with the CON and TN HX groups (p<0.05; Figure 2B). Total fluorescence of DAPI was not different between treatments (p>0.05; data not shown). EEA1 demonstrated high co-localization with CLDN4 with a Pearson’s r-value of 0.74, which was significant (p < 0.05; Supplemental Figure 1).
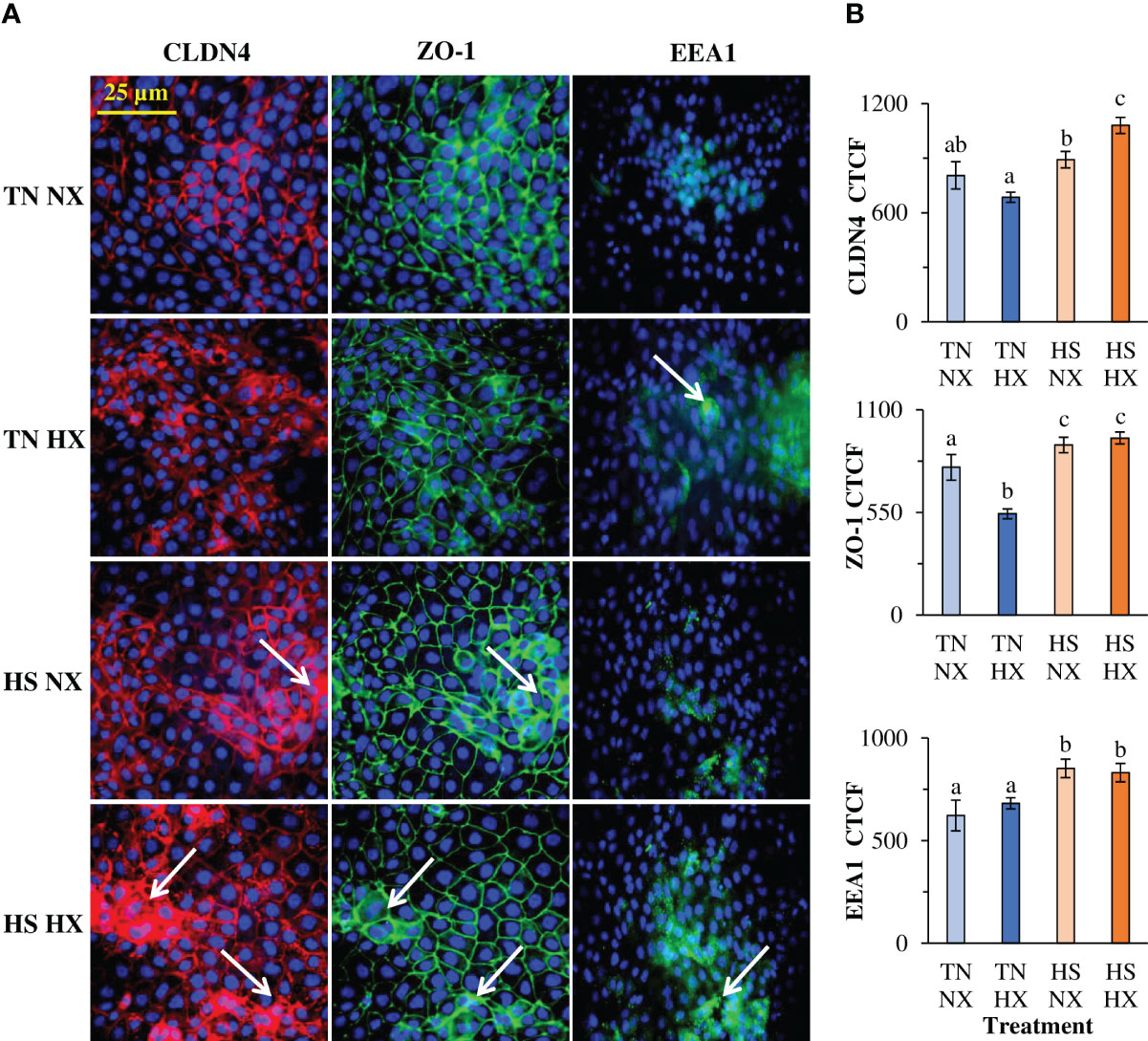
Figure 2 The effects of 24 hours of either constant thermal neutral conditions under normoxia (TN NX; 21°C), constant thermal neutral conditions under 1% hypoxia (TN HX), heat stress conditions under normoxia (HS NX; 42°C), or heat stress conditions under 1% hypoxia (HS HX) on (A) immunofluorescence images of claudin 4 (CLDN4; red), zonula-occluden 1 (ZO1; green), or early endosomal antigen 1 (EEA1; green) and (B) corrected total cell fluorescence measurements (CTCF). DAPI (blue) is marking the nuclei. Six fields of view from six separate wells were used for calculations. DAPI CTCF was not different between treatments. a,b,cp<0.05, n=6/trt.
Protein expression of two major heat shock proteins and cell chaperones, HSP70 and HSP90, was measured in cell lysate to confirm heat shock response in treated cells. HSP70 levels decreased by 40% (p<0.05; Figure 3A) due to hypoxia treatment but, as expected, increased by more than 150% due to heat exposure. HSP90 levels were not decreased due to hypoxia treatment alone (p>0.05; Figure 3B) but increased by more than 200% due to heat exposure. Representative blot images are shown in Figure 3C.
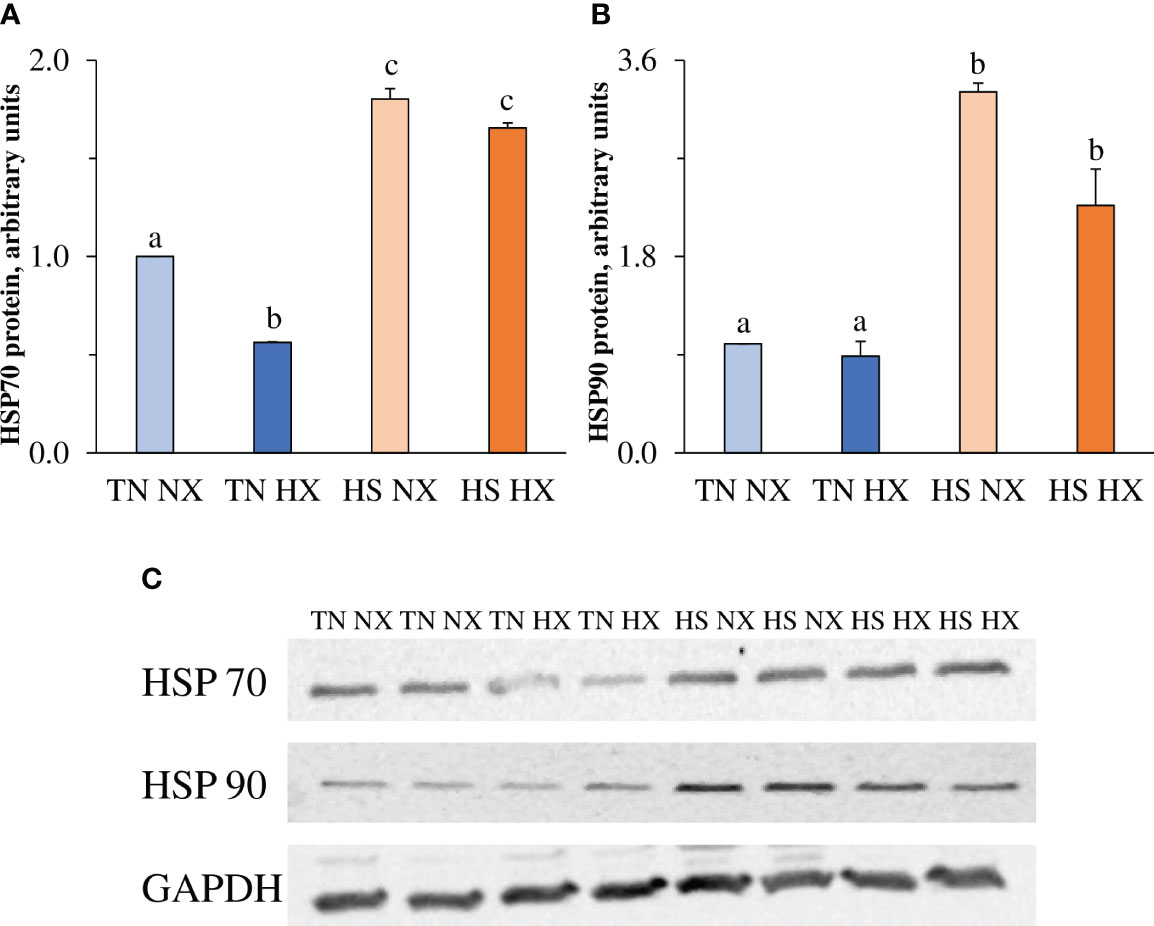
Figure 3 The effects of 24 hours of either constant thermal neutral conditions under normoxia (TN NX; 21°C), constant thermal neutral conditions under 1% hypoxia (TN HX), heat stress conditions under normoxia (HS NX; 42°C), or heat stress conditions under 1% hypoxia (HS HX) on (A) heat shock protein 70 (HSP70) relative expression, (B) heat shock protein 90 (HSP90) relative expression, and (C) representative blot images of each along with housekeeping protein GAPDH. a,b,cp<0.05, n = 6/trt.
Discussion
Changes in intestinal integrity can occur due to alterations in the amount and localization of tight junction protein at the plasma membrane of intestinal epithelial cells. The reduction of membrane-associated tight junction proteins and the consequential internalization of these proteins into the early endosome may result in the decreased polarization and integrity of the epithelial barrier. However, few studies have assessed this under heat stress or hypoxia conditions in pig models. Previous studies have shown that HS reduces intestinal integrity (Pearce et al., 2013a); however, it is largely unknown if the ambient heat or the associated intestinal hypoxia results in tight junction protein remodeling. Heat stress causes intestinal ischemia/hypoxia as blood is partitioned to the periphery, and there has been evidence of hypoxia in cases of environmental hyperthermia (Pearce et al., 2013a). Thus, in this agriculturally relevant in vitro model, our data suggest that hypoxia may be a driving factor in HS-induced barrier dysfunction. The IPEC-J2 cell line used herein is a non-transformed small intestinal cell line derived from neonatal pigs and has been shown to be a good model for studying nutrition (Rhoads et al., 1994; Yan and Ajuwon, 2017), bacterial invasion (Duan et al., 2022), viral infection (Guo et al., 2022), toxins (Li et al., 2022), and environmental factors such as HS (Cao et al., 2016) and hypoxia (Chapman et al., 2012). Under standard cell culture conditions, cells are typically grown at 37°C. However, pigs’ normal body temperature ranges from approximately 38.5°C to 39.7°C, which is higher than that of humans (Morales et al., 2016). Therefore, we propagated the IPEC at 38°C for our thermal neutral condition to mimic the source tissue. Cell viability and polarization was not affected by this warmer thermoneutral culturing condition (data not shown).
Under in vitro conditions, HS has been shown to upregulate the tight junction protein zonula-occluden-1 (ZO-1), as well as inflammatory genes IL-6, ICAM-1, and TGF-β; however, functional integrity such as macromolecular transport was not measured (Cao et al., 2016). Separately, Chapman et al. (2012) reported that hypoxia treatment of IPEC-J2 cells caused a large reduction in TEER, a functional measure of monolayer barrier function, after 12 hours. At the same time, inulin flux (a marker of paracellular macromolecular flux) was greatly increased by hypoxia treatment alone. These hypoxia functional data were also accompanied by changes in the tight junction protein distribution of ZO-1 (Chapman et al., 2012). In the current study, we reported large reductions in IPEC-J2 intestinal barrier function, as observed by TEER and FITC-dextran transport, in a short period of time in both the hypoxia alone and HS HX treatments. Intriguingly, HS alone increased TEER in IPEC-J2 cells, and this result was similar across multiple time points and experimental replications. Other cell culture models have been utilized to study the effects of HS, including IEC-6 (He et al., 2016), SW480 (Yi et al., 2017), Caco2 (Dokladny et al., 2008), and HT-29 (Lian et al., 2021). Of these models, all have reported decreases in intestinal integrity due to elevated culturing temperature conditions, which is in contrast to our current model. The discrepancies in barrier TEER under elevated culturing temperatures could also be attributed to our TN controls being cultured at a starting temperature 1–2°C higher than the standard to mimic normal porcine physiology. It is possible that this may have caused some adaptation or acclimation to heat in the cells; however, a robust HSP response was still observed.
The epithelial barrier between cells is created by a series of junctional proteins, including tight junctions (TJs), gap junctions, adherens junctions, and desmosomes. Epithelial TJs regulate the polarity and movement of molecules between adjacent cells. Claudins are a family of transmembrane TJ proteins that can be classified as either “tight” or “leaky” based on what they allow through. Claudin 4 is considered a tight claudin and is involved in regulating barrier function. Zonula occludens are another type of TJ protein that are cytoplasmic. ZO-1 helps to stabilize claudin strands and the actin cytoskeleton (Garcia-Hernandez et al., 2017).
Polarized epithelial cells are capable of rapidly remodeling and reshaping paracellular pore spaces through diffusion, endocytosis, and the internalization of tight junction proteins (Stamatovic et al., 2017). This internalization of tight junction proteins is interconnected to membrane-bound endosome compartments. Protein expression via immunofluorescence of tight junction proteins also appeared to redistribute to the cytosol from the membrane, especially CLDN4. This occurred under all treatments; however, the most noticeable changes were in the HS HX cells where large, internalized clusters of CLDN4 were found. This contrasts with the controls, which exhibited the typical honeycomb structure of tight junctions. Interestingly, total fluorescence did not correlate entirely with functional data or redistribution observations. Specifically, total fluorescence was decreased under hypoxia alone but increased under HS alone and HS HX, although functional data were similar between the hypoxia and HS HX treatments. This could be due to several factors, including the methods utilized, timing of protein translation and function, and proteins analyzed, as functional data is representative of the entire junctional complex. CLDN4 also appeared to also co-localize with EEA1. Endocytosis is a process by which substances are taken in by a cell, and during this process vesicles are formed containing the ingested material (Nighot and Ma, 2021). Early endosome antigen 1 (EEA1) localizes specifically to early endosomes; it is involved in endosomal trafficking and has been described as a central sorting station (Barone and Zimmer, 2016). Endocytosis is important for the regulation of tight junction complexes and allows cells to adapt to various stressors. Specifically, it has emerged as an important process for tight junction remodeling and endocytic removal (Li and Ajuwon, 2021; Nighot and Ma, 2021). EEA1 has previously been shown to co-localize with TJ proteins (Matsuda et al., 2004; Li et al., 2022). Although the HS HX treatment had the highest TEER, it also had increased TJ protein fluorescence and higher remodeling. This could be unrelated to endosomal recycling or may be due to effects on other junctional complexes that were not measured within the scope of the current study.
The changes in the amount of tight junction proteins of heat shock proteins. The levels of heat shock proteins, such as HSP70, are increased during HS, and they act as molecular chaperones and protect protein function (Alfieri et al., 2004; Chen et al., 2009). In pig intestinal tissues, we have reported that HS increases levels of SP70, in addition to levels of HIF1α (Pearce et al., 2013a). However, hypoxia alone may not increase the amount of heat shock proteins. Heat shock proteins act as molecular chaperones that assist in protein folding and are regulated by heat shock factors (HSFs). Heat shock protein 70 is one of the most inducible HSPs and is a chaperone for nascent polypeptide chains (Wang et al., 2018). It is also thought that HSPs act as intestinal gatekeepers (Liu et al., 2014). Following HS, intestinal epithelial cells produce heat shock proteins as a protective mechanism (Malago et al., 2002). HSP90 has been shown to be involved in several survival signaling pathways, including those involved in antioxidant response and apoptosis (Zhang et al., 2020). As expected, levels of HSP70 and HSP90 were increased in both HS-treated groups, indicating that both groups experienced HS. There was no difference between the normoxia- and hypoxia-treated groups; therefore hypoxia did not alter the HSP response. The total fluorescence of TJ proteins was increased in treatment groups where HSP activation was also observed.
Interleukin 8 (IL-8/CXCL-8) is one of the most abundant inflammatory cytokines/chemokines produced by the intestinal epithelium and epithelial cells. Its main functions are to induce chemotaxis in neutrophils, induce phagocytosis, and protect cells against damage (Maheshwari et al., 2002). Interestingly, our results show a decrease in IL-8 protein secretion in HS-treated cells, which would mimic systemic IL-8 concentrations in the blood. This is counter-intuitive to a potential inflammatory response to environmental hyperthermia; however, this phenomenon has also been observed in vivo (Pearce et al., 2013a; Varasteh et al., 2015) and may be a species-specific or timing-specific response. In our in vitro model, there is no immune cell component, thus the IL-8 response must be due solely to intestinal epithelial cell production. Previous research has shown that the induction of HSPs may inhibit IL-8 production or secretion. Interestingly, heat shock has been reported to co-activate interleukin-8 gene transcription (Singh et al., 2008; Chung et al., 2009; Zhong et al., 2012).
We believe hypoxia is a driving factor in barrier dysfunction, as HS alone in our model was not enough to disrupt the intestinal barrier at a functional level but was able to cause changes at a molecular level. This may be partially due to an increase in HSP70, which is a molecular chaperone and protectant during HS that is not observed in hypoxia. It may also be due to a slightly higher starting temperature to mimic normal porcine body temperatures. There are likely other factors or proteins involved in this process that were not measured in the current study. In conclusion, this short research communication provides new insights into how HS and hypoxia modulate intestinal barrier integrity. These data show for the first time in IPEC-J2 cells that hypoxia appears to cause a large majority of the epithelial damage and dysfunction during a bout of high ambient heat, including increased intestinal permeability, tight junction remodeling, and early endosome disruption. Interestingly, these changes are in the absence of an IL-8 response. Future studies should elucidate mechanisms and provide potential nutritional mitigation strategies to prevent HS and hypoxia-induced intestinal dysfunction.
Data availability statement
The original contributions presented in the study are included in the article/Supplementary Material. Further inquiries can be directed to the corresponding author.
Author contributions
All authors contributed to the design of the study, editing the manuscript, and the interpretation of results. SP conducted the experiments, collected the samples and wrote the manuscript. All authors contributed to the article and approved the submitted version.
Conflict of interest
The authors declare that the research was conducted in the absence of any commercial or financial relationships that could be construed as a potential conflict of interest.
Publisher’s note
All claims expressed in this article are solely those of the authors and do not necessarily represent those of their affiliated organizations, or those of the publisher, the editors and the reviewers. Any product that may be evaluated in this article, or claim that may be made by its manufacturer, is not guaranteed or endorsed by the publisher.
Supplementary material
The Supplementary Material for this article can be found online at: https://www.frontiersin.org/articles/10.3389/fanim.2023.1204152/full#supplementary-material
Supplementary Figure 1 | Merged images of CLDN4 (red) and EEA1 (green) along with DAPI (blue). Areas of co-localization shown with white arrows. Pearson’s r-value for co-localization between CLDN4 and EEA1 was calculated using a total of 12 fields of view.
References
Alfieri R. R., Petronini P. G., Bonelli M. A., Desenzani S., Cavazzoni A., Borghetti A. F., et al. (2004). Roles of compatible osmolytes and heat shock protein 70 in the induction of tolerance to stresses in porcine endothelial cells. J. Physiol. 555 (Pt 3), 757–767. doi: 10.1113/jphysiol.2003.058412
Barone M. V., Zimmer K. P. (2016). Endocytosis and transcytosis of gliadin peptides. Mol. Cell Pediatr. 3 (1), 8. doi: 10.1186/s40348-015-0029-z
Cao L., Tang J., Li Q., Xu J., Jia G., Liu G., et al. (2016). Expression of selenoprotein genes is affected by heat stress in IPEC-J2 cells. Biol. Trace Elem Res. 172 (2), 354–360. doi: 10.1007/s12011-015-0604-0
Chapman J. C., Liu Y., Zhu L., Rhoads J. M. (2012). Arginine and citrulline protect intestinal cell monolayer tight junctions from hypoxia-induced injury in piglets. Pediatr. Res. 72 (6), 576–582. doi: 10.1038/pr.2012.137
Chen Z. C., Wu W. S., Lin M. T., Hsu C. C. (2009). Protective effect of transgenic expression of porcine heat shock protein 70 on hypothalamic ischemic and oxidative damage in a mouse model of heatstroke. BMC Neurosci. 10, 111. doi: 10.1186/1471-2202-10-111
Chung S. W., Lee J. H., Choi K. H., Park Y. C., Eo S. K., Rhim B. Y., et al. (2009). Extracellular heat shock protein 90 induces interleukin-8 in vascular smooth muscle cells. Biochem. Biophys. Res. Commun. 378 (3), 444–449. doi: 10.1016/j.bbrc.2008.11.063
Della Rocca Y., Fonticoli L., Rajan T. S., Trubiani O., Caputi S., Diomede F., et al. (2022). Hypoxia: molecular pathophysiological mechanisms in human diseases. J. Physiol. Biochem. 78 (4), 739–752. doi: 10.1007/s13105-022-00912-6
Dokladny K., Ye D., Kennedy J. C., Moseley P. L., Ma T. Y. (2008). Cellular and molecular mechanisms of heat stress-induced up-regulation of occludin protein expression: regulatory role of heat shock factor-1. Am. J. Pathol. 172 (3), 659–670. doi: 10.2353/ajpath.2008.070522
Duan Q., Pang S., Feng L., Liu J., Lv L., Li B., et al. (2022). Heat-labile enterotoxin enhances F4-producing enterotoxigenic e. coli adhesion to porcine intestinal epithelial cells by upregulating bacterial adhesins and STb enterotoxin. Veterinary Res. 53 (1), 88. doi: 10.1186/s13567-022-01110-4
Garcia-Hernandez V., Quiros M., Nusrat A. (2017). Intestinal epithelial claudins: expression and regulation in homeostasis and inflammation. Ann. N Y Acad. Sci. 1397 (1), 66–79. doi: 10.1111/nyas.13360
Ghulam Mohyuddin S., Khan I., Zada A., Qamar A., Arbab A. A. I., Ma X. B., et al. (2022). Influence of heat stress on intestinal epithelial barrier function, tight junction protein, and immune and reproductive physiology. BioMed. Res. Int. 2022, 8547379. doi: 10.1155/2022/8547379
Guo Z., Zhang C., Dong J., Wang Y., Hu H., Chen L. (2022). Persistence infection of TGEV promotes enterococcus faecalis infection on IPEC-J2 cells. Int. J. Mol. Sci. 24 (1). doi: 10.3390/ijms24010450
He S., Liu F., Xu L., Yin P., Li D., Mei C., et al. (2016). Protective effects of ferulic acid against heat stress-induced intestinal epithelial barrier dysfunction In vitro and In vivo. PloS One 11 (2), e0145236. doi: 10.1371/journal.pone.0145236
Hu C., Yang J., Qi Z., Wu H., Wang B., Zou F., et al. (2022). Heat shock proteins: biological functions, pathological roles, and therapeutic opportunities. MedComm 2020 3 (3), e161. doi: 10.1002/mco2.161
Hutchins K. P., Minett G. M., Stewart I. B. (2022). Treating exertional heat stroke: limited understanding of the female response to cold water immersion. Front. Physiol. 13. doi: 10.3389/fphys.2022.1055810
Konjar Š., Pavšič M., Veldhoen M. (2021). Regulation of oxygen homeostasis at the intestinal epithelial barrier site. Int. J. Mol. Sci. 22 (17), 9170. doi: 10.3390/ijms22179170
Li E., Ajuwon K. M. (2021). Mechanism of endocytic regulation of intestinal tight junction remodeling during nutrient starvation in jejunal IPEC-J2 cells. FASEB J. Off. Publ. Fed. Am. Societies Exp. Biol. 35 (2), e21356. doi: 10.1096/fj.202002098R
Li E., Horn N., Ajuwon K. M. (2022). EPA And DHA inhibit endocytosis of claudin-4 and protect against deoxynivalenol-induced intestinal barrier dysfunction through PPARγ dependent and independent pathways in jejunal IPEC-J2 cells. Food Res. Int. 157, 111420. doi: 10.1016/j.foodres.2022.111420
Lian P., Braber S., Varasteh S., Wichers H. J., Folkerts G. (2021). Hypoxia and heat stress affect epithelial integrity in a caco-2/HT-29 co-culture. Sci. Rep. 11 (1), 13186. doi: 10.1038/s41598-021-92574-5
Lian P., Henricks P. A. J., Wichers H. J., Folkerts G., Braber S. (2023). Differential effects of oligosaccharides, antioxidants, amino acids and PUFAs on Heat/Hypoxia-induced epithelial injury in a caco-2/HT-29 Co-culture model. Int. J. Mol. Sci. 24 (2), 1111. doi: 10.3390/ijms24021111
Liu H., Dicksved J., Lundh T., Lindberg J. E. (2014). Heat shock proteins: intestinal gatekeepers that are influenced by dietary components and the gut microbiota. Pathogens 3 (1), 187–210. doi: 10.3390/pathogens3010187
Maheshwari A., Lu W., Lacson A., Barleycorn A. A., Nolan S., Christensen R. D., et al. (2002). Effects of interleukin-8 on the developing human intestine. Cytokine 20 (6), 256–267. doi: 10.1006/cyto.2002.1996
Malago J. J., Koninkx J. F., van Dijk J. E. (2002). The heat shock response and cytoprotection of the intestinal epithelium. Cell Stress Chaperones 7 (2), 191–199. doi: 10.1379/1466-1268(2002)007<0191:THSRAC>2.0.CO;2
Matsuda M., Kubo A., Furuse M., Tsukita S. (2004). A peculiar internalization of claudins, tight junction-specific adhesion molecules, during the intercellular movement of epithelial cells. J. Cell Sci. 117 (7), 1247–1257. doi: 10.1242/jcs.00972
Morales A., Hernández L., Buenabad L., Avelar E., Bernal H., Baumgard L. H., et al. (2016). Effect of heat stress on the endogenous intestinal loss of amino acids in growing pigs. J. Anim. Sci. 94 (1), 165–172. doi: 10.2527/jas.2015-9393
Nighot P., Ma T. (2021). Endocytosis of intestinal tight junction proteins: in time and space. Inflammation Bowel Dis. 27 (2), 283–290. doi: 10.1093/ibd/izaa141
Pearce S. C., Mani V., Boddicker R. L., Johnson J. S., Weber T. E., Ross J. W., et al. (2013a). Heat stress reduces intestinal barrier integrity and favors intestinal glucose transport in growing pigs. PloS One 8 (8), e70215. doi: 10.1371/journal.pone.0070215
Pearce S. C., Mani V., Weber T. E., Rhoads R. P., Patience J. F., Baumgard L. H., et al. (2013b). Heat stress and reduced plane of nutrition decreases intestinal integrity and function in pigs. J. Anim. Sci. 91 (11), 5183–5193. doi: 10.2527/jas.2013-6759
Pearce S. C., Sanz-Fernandez M. V., Hollis J. H., Baumgard L. H., Gabler N. K. (2014). Short-term exposure to heat stress attenuates appetite and intestinal integrity in growing pigs. J. Anim. Sci. 92 (12), 5444–5454. doi: 10.2527/jas.2014-8407
Pearce S. C., Sanz Fernandez M. V., Torrison J., Wilson M. E., Baumgard L. H., Gabler N. K. (2015). Dietary organic zinc attenuates heat stress-induced changes in pig intestinal integrity and metabolism. J. Anim. Sci. 93 (10), 4702–4713. doi: 10.2527/jas.2015-9018
Rhoads J. M., Chen W., Chu P., Berschneider H. M., Argenzio R. A., Paradiso A. M. (1994). L-glutamine and l-asparagine stimulate na+ -h+ exchange in porcine jejunal enterocytes. Am. J. Physiol. 266 (5 Pt 1), G828–G838. doi: 10.1152/ajpgi.1994.266.5.G828
Rosinger A. Y. (2023). Extreme climatic events and human biology and health: a primer and opportunities for future research. Am. J. Hum. Biol. 35 (1), e23843. doi: 10.1002/ajhb.23843
Singh I. S., Gupta A., Nagarsekar A., Cooper Z., Manka C., Hester L., et al. (2008). Heat shock co-activates interleukin-8 transcription. Am. J. Respir. Cell Mol. Biol. 39 (2), 235–242. doi: 10.1165/rcmb.2007-0294OC
Stamatovic S. M., Johnson A. M., Sladojevic N., Keep R. F., Andjelkovic A. V. (2017). Endocytosis of tight junction proteins and the regulation of degradation and recycling. Ann. N Y Acad. Sci. 1397 (1), 54–65. doi: 10.1111/nyas.13346
Varasteh S., Braber S., Akbari P., Garssen J., Fink-Gremmels J. (2015). Differences in susceptibility to heat stress along the chicken intestine and the protective effects of galacto-oligosaccharides. PloS One 10 (9), e0138975. doi: 10.1371/journal.pone.0138975
Vásquez N., Cervantes M., Bernal-Barragán H., Rodríguez-Tovar L. E., Morales A. (2022). Short- and long-term exposure to heat stress differently affect performance, blood parameters, and integrity of intestinal epithelia of growing pigs. Anim. an Open Access J. MDPI 12 (19). doi: 10.3390/ani12192529
Wang Y., Lin F., Zhu X., Leone V. A., Dalal S., Tao Y., et al. (2018). Distinct roles of intracellular heat shock protein 70 in maintaining gastrointestinal homeostasis. Am. J. Physiol. Gastrointestinal liver Physiol. 314 (2), G164–g178. doi: 10.1152/ajpgi.00208.2017
Wood F., Roiz-de-Sa D., Pynn H., Smith J. E., Bishop J., Hemingway R. (2022). Outcomes of UK military personnel treated with ice cold water immersion for exertional heat stroke. BMJ Mil Health. doi: 10.1136/military-2022-002133
Yan H., Ajuwon K. M. (2017). Butyrate modifies intestinal barrier function in IPEC-J2 cells through a selective upregulation of tight junction proteins and activation of the akt signaling pathway. PloS One 12 (6), e0179586. doi: 10.1371/journal.pone.0179586
Yi G., Li L., Luo M., He X., Zou Z., Gu Z., et al. (2017). Heat stress induces intestinal injury through lysosome- and mitochondria-dependent pathway in vivo and in vitro. Oncotarget 8 (25), 40741–40755. doi: 10.18632/oncotarget.16580
Zhang X. H., Wu J. X., Sha J. Z., Yang B., Sun J. R., Bao E. D. (2020). Heat shock protein 90 relieves heat stress damage of myocardial cells by regulating akt and PKM2 signaling in vivo. Int. J. Mol. Med. 45 (6), 1888–1908. doi: 10.3892/ijmm.2020.4560
Keywords: heat stress, hypoxia, intestinal, cell culture, pig, stress
Citation: Pearce SC and Gabler NK (2023) Hypoxia exacerbates heat stress effects on the porcine intestinal epithelium in vitro. Front. Anim. Sci. 4:1204152. doi: 10.3389/fanim.2023.1204152
Received: 11 April 2023; Accepted: 23 June 2023;
Published: 18 July 2023.
Edited by:
Erin E. Connor, University of Delaware, United StatesReviewed by:
Yihang Li, University of Delaware, United StatesWeinan Zhou, University of Illinois at Urbana-Champaign, United States
Copyright © 2023 Pearce and Gabler. This is an open-access article distributed under the terms of the Creative Commons Attribution License (CC BY). The use, distribution or reproduction in other forums is permitted, provided the original author(s) and the copyright owner(s) are credited and that the original publication in this journal is cited, in accordance with accepted academic practice. No use, distribution or reproduction is permitted which does not comply with these terms.
*Correspondence: S. C. Pearce, Sarah.Pearce@usda.gov