- Lethbridge Research and Development Centre, Agriculture and Agri-Food Canada, Lethbridge, Canada
Supplementing ruminant diets with macroalgae is gaining interest globally because bromoform-containing seaweeds (e.g., Asparagopsis spp.) have been shown to be highly effective enteric methane (CH4) inhibitors. Some alternative seaweeds decrease in vitro CH4 production, but few have been evaluated in animals. This study examined the effects of including the red seaweed Mazzaella japonica in the diet of beef cattle on dry matter intake (DMI), rumen fermentation, digestibility, nitrogen (N) utilization, and enteric CH4 production. Six ruminally cannulated, mature beef heifers (824 ± 47.1 kg) were used in a double 3 × 3 Latin square with 35-d periods. The basal diet consisted of 52% barley silage, 44% barley straw, and 4% vitamin and mineral supplement [dry matter (DM) basis]. The treatments were (DM basis): 0% (control), 1%, and 2% M. japonica. The DMI increased quadratically (P = 0.025) with the inclusion of M. japonica, such that the DMI of heifers consuming 1% was greater (P < 0.05) than that of control heifers. The apparent total-tract digestibility of DM decreased linearly (P = 0.002) with the inclusion of M. japonica, but there were no treatment differences in the digestibility of organic matter, crude protein (CP), neutral detergent fiber, or starch. The level of M. japonica linearly (P < 0.001) increased the N intake of the heifers. Fecal N excretion linearly increased (P = 0.020) with M. japonica, but there were no differences in total urinary N excretion, N fractions (allantoin, uric acid), total purine derivatives, microbial purine derivatives absorbed, microbial N flow, or retained N. There were no treatment effects on rumen pH or total volatile fatty acids (VFAs); however, adding M. japonica to the diet quadratically (P = 0.023) decreased the proportion of acetate, whereas 1% inclusion decreased the acetate proportion. Methane production (g/day) decreased quadratically (P = 0.037), such that the heifers receiving 2% M. japonica produced 9.2% less CH4 than control animals; however, CH4 yield (g/kg DMI) did not differ among treatments. We conclude that supplementing a forage-based diet with up to 2% M. japonica failed to lower the enteric CH4 yield of beef heifers. M. japonica can be used in diets to help meet the CP requirements of cattle, but inclusion rates may be limited by high inorganic matter proportions. When comprising up to 2% of the diet, M. japonica cannot be recommended as a CH4 inhibitor for beef cattle fed on high-forage diets.
1 Introduction
The International Panel on Climate Change (IPCC) estimated that in 2010 enteric methane (CH4) from ruminant livestock [1.6 to 2.7 Gt of carbon dioxide equivalent (CO2e) per year] accounted for 3% to 5% of the total global anthropogenic greenhouse gas (GHG) emissions by all sectors (49 Gt total; Smith et al., 2014). Gerber et al. (2013) estimated a slightly greater contribution of enteric CH4 at 6% of global GHG emissions, representing 40% of all livestock emissions. Given the ambitious GHG reduction commitments made by many countries, mitigation of enteric CH4 has become a subject of intense investigation by the global research community. Numerous mitigation approaches have been proposed, as summarized in recent reviews (e.g., Beauchemin et al., 2020; Ku-Vera et al., 2020; Arndt et al., 2022; Beauchemin et al., 2022). Some strategies include improving animal efficiency, diet reformulation (e.g., decreasing fiber concentration), lipid supplementation of diets, forage management to improve digestibility, improved grazing management, tannin-containing forages, seaweed (macroalgae), CH4 inhibitors (e.g., 3-nitrooxypropanol), nitrates, essential oils, other secondary plant compounds, genetic selection of low-emitting animals, vaccines, and early-life microbiome programming.
Among the mitigation approaches, the use of seaweeds has gained wide-scale interest because seaweeds are “naturally” sourced and widely abundant, livestock has a long history of grazing seaweed along shorelines in coastal areas, and some seaweeds are highly effective CH4 inhibitors (Abbott et al., 2020). Specifically, the red seaweed Asparagopsis spp. (e.g., A. taxiformis and A. armata) has been shown to consistently decrease CH4 emissions from dairy and beef cattle production (Lean et al., 2021), with reductions of up to 98% reported in beef cattle fed a high-grain diet supplemented with A. taxiformis (Kinley et al., 2020). Asparagopsis spp. accumulates halogenated compounds (mainly bromoform and dibromochloromethane) that reduce the efficiency of methyltransferase during methanogenesis (Machado et al., 2016). A recent study examining the impact of A. taxiformis on the rumen microbiome found that it rapidly reduced the abundance of rumen methanogens and led to a total collapse of the methanogen community (O’Hara et al., 2022). However, bromoform-containing seaweeds are not yet widely available to farmers because of regulatory issues, high production costs, and uncertainty as to whether or not bromoform and iodine accumulate in milk and meat. There is also a need for more efficient production practices to lower the CO2 emissions from growing, harvesting, processing, extracting, and transporting seaweeds on a large scale.
Given the current limitations to the wide-scale use of Asparagopsis spp., other red, brown, and green seaweeds have garnered interest for CH4 mitigation because they contain specific carbohydrates, lipids, peptides, saponins, and phlorotannins that have the potential to inhibit methanogens, albeit to a lesser extent than bromoform-containing seaweeds (Vijn et al., 2020; Abbott et al., 2020). To date, most of the research on these alternative seaweeds for ruminants has been conducted in vitro. As summarized by Abbott et al. (2020), decreases in in vitro CH4 production have been reported for Cladophora patentiramea (green seaweed), Cystoseira trinodis (brown seaweed), Dictyota bartayresii (brown seaweed), Gigartina spp. (red seaweed), Padina australis (brown seaweed), and Ulva spp. (green seaweed), but efficacy has not been confirmed in vivo. In fact, few in vivo studies have evaluated non-bromoform-containing seaweeds for their ability to inhibit CH4 production. Lean et al. (2021) searched the literature prior to 2021 to conduct a meta-analysis and identified only one study (Antaya et al., 2019) that evaluated a non-bromoform-containing seaweed and CH4 production in ruminants. The study by Antaya et al. (2019), which provided grazing dairy cows with Ascophyllum nodosum (kelp meal) at approximately 0.7% of dry matter (DM) intake (DMI), reported a 10.5% decrease in CH4 production (g/day) in the first month of feeding, with no effects thereafter. However, CH4 yield (g/kg DMI) was not affected during that study. A more recent meta-analysis by Sofyan et al. (2022) included an additional publication (Katwal et al., 2021) in which the feeding of Sargassum johnstonii, at 8% of dietary DM, had no effect on CH4 yields of lactating cows (Katwal et al., 2021). Thus, there is a paucity of support for the use of non-bromoform-containing seaweeds for CH4 mitigation, and further in vivo research is required.
The red seaweed Mazzaella japonica is native to Republic of Korea, Japan, and Russia, and it is a non-indigenous species in other areas, including the coastal waters of western Canada where it is licensed for beach harvest (Holden et al., 2018). Once harvested, the material is sun-dried and marketed primarily as a source of carrageenan, a thickening agent used in processed foods, cosmetics, and pharmaceuticals. It is also used by local farmers as a roughage source for beef cattle, which has prompted interest in its potential for CH4 mitigation. The objective of this study was to evaluate the effects of including M. japonica in the diet of beef cattle on DMI, rumen fermentation, digestibility, nitrogen (N) utilization, and enteric CH4 production. The hypothesis was that incorporating M. japonica into the diet of beef cattle would decrease CH4 production without incurring any negative effects on DMI and digestibility.
2 Methods
2.1 Experimental design, animals, and diet
The experiment was conducted at the Beef Cattle Metabolism Facility of Agriculture and Agri-Food Canada, Lethbridge Research and Development Centre (Lethbridge, AB) in accordance with guidelines from the Canadian Council on Animal Care (2009) and was pre-approved (protocol #ACC1935) by the Institutional Animal Care and Use Committee. Six ruminally cannulated, mature beef heifers [Angus cross; mean initial body weight (BW) ± SD, 824 ± 47.1 kg] were used in a double 3 × 3 Latin square, with three animals per square. The three periods consisted of 14 days of adaption and 14 days of measurements and the two periods were separated by a washout phase of at least 7 days, during which the animals were fed the basal diet.
The basal diet consisted of 52% barley silage, 44% barley straw, and 4% vitamin and mineral supplements (DM basis; Table 1) and was formulated to meet the nutrient requirements of the animals according to the National Academies of Sciences, Engineering, and Medicine (NASEM) (2016). To provide three dietary treatments, the basal diet was supplemented with air-dried M. japonica at 0% (control), 1%, and 2% of DM. M. japonica was incorporated into the diet by replacing equal portions of silage and straw on a DM basis. The diets were prepared daily as total mixed rations (TMRs) using a Data Ranger (American Calan Inc., Northwood, NH, USA). The rations were offered once daily at 09:00 h for ad libitum intake (5% refusals), except during total-tract digestibility measurements (95% of ad libitum intake). Refusals were removed daily prior to feeding. The cattle had free access to water and animal health was monitored daily. The heifers were housed in individual tie stalls that contained rubber mattresses bedded with wood shavings, except during total-tract digestibility and gas emission measurements. When weather and sampling permitted, the heifers were released into an outdoor pen for 2–3 hours of daily exercise.
2.2 Measurements
2.2.1 Body weight, dry matter intake, and eating rate
The heifers were weighed prior to feeding (non-fasted) at the beginning and end of each period. The DMI was calculated as the daily amount of TMRs offered minus the daily refusal, and corrected for the DM concentration of each. To determine the DM concentration, samples of TMRs were collected weekly and daily refusals were pooled by animal and week, and then dried in a forced air oven at 55°C for 48 hours. The weekly samples were then pooled by period and stored at −20°C until they were analyzed. The eating rate was measured on days 1, 4, and 11 by weighing the feed bunk to determine feed disappearance at 3, 6, 9, 12, and 24 hours after feeding, as described by Kim et al. (2019). The repeated measurement was done to determine potential palatability effects due to the seaweed additions.
2.2.2 Rumen pH and fermentation
Indwelling pH meters (LRCpH Data Loggers; Dascor, Inc., Escondido, CA, USA) were inserted through the rumen fistula of each animal on day 15 to record ruminal pH at 1-minute intervals for a total of 72 hours. Prior to insertion into the rumen and following removal, the pH systems were standardized in pH buffers 7 and 4 at 39°C. On day 18, approximately 250 mL of content was collected from four different sites within the rumen at 0, 2, 4, 6, 9, 12, and 24 hours after feeding. Samples were immediately filtered through a polyester monofilament fabric (355-µm mesh opening; B & S H Thompson, Ville Mont-Royal, QC, Canada) and 5-mL subsamples of filtrate were preserved with either 1 mL of 25% (w/v) metaphosphoric acid for volatile fatty acid (VFA) concentrations or 1 mL of 1% (v/v) sulfuric acid (H2SO4) for ammonia (NH3) analysis. Samples were then stored at −20°C until they were analyzed. For protozoa enumeration, 5 mL of filtrate was mixed with 5 mL of methyl green–formalin–saline solution and stored in the dark at room temperature until analyzed. Ruminal protozoa were enumerated under a light microscope using a counting chamber (Neubauer Improved Bright-Line counting cell, 0.1-mm depth; Hausser Scientific, Horsham, PA, USA).
2.2.3 Apparent total tract digestibility and nitrogen metabolism
An indwelling catheter (26 French, 75-cc balloon; C. R. Bard, Inc., Covington, GA, USA) was inserted into the bladder of each animal on day 19: tubing was attached, and urine was collected into a closed 20-L collection container for 96 hours. To prevent microbial activity and volatilization of NH3, the pH of the urine was maintained at < 2.5 by adding 500 mL of 2 M H2SO4 to the collection container daily. The volume of urine was recorded daily, and a 20-mL sample of the acidified urine for each day was diluted to 100 mL with deionized water to avoid precipitation of the uric acid (Chen and Gomes, 1992), and samples were stored frozen (−20°C) until analysis for total N, urea, NH3, and purine derivatives (PDs; i.e., allantoin and uric acid). Apparent total-tract digestibility was measured by collecting the total amount of feces excreted from each animal. The feces were weighed, mixed, sampled (5% of total wet weight), and stored at −20°C until they were analyzed.
2.2.4 Methane emissions
Enteric CH4 emissions were measured using three open-circuit calorimetry chambers (4.4 m wide × 3.7 m deep × 3. m tall; Conviron Inc., Winnipeg, MB, Canada) according to the methods described by Beauchemin and McGinn (2006). The chambers were calibrated before and after every period by sequentially releasing known quantities of CH4 and CO2 into each chamber using a mass flow meter (Omega Engineering, Stamford, CT, USA). Best-fit calibration regressions were generated for each gas and the slopes were used to correct the emissions (McGinn et al., 2004). Animals were trained for entry into the chambers prior to starting the study to minimize stress. As there were only three chambers, the two squares (groups) were staggered by 1 week. Gas concentrations were measured using infrared gas analyzers (CH4: model Ultramat 5E—Siemens Inc., Karlsruhe, Germany; CO2/H2O: model LI-7000—LICOR Environmental, Lincoln, NE). Gas concentrations in the inlet and outlet air ducts of each chamber were monitored sequentially for approximately 3 consecutive minutes (total 6–7 minutes/chamber). All chambers were sampled within 27 minutes, with an additional 3 minutes used to measure the zero reference gas (pure N). The gas sampling procedure was repeated every 30 minutes, for a total of 48 times per chamber per day.
2.3 Chemical analysis
The period composites of TMRs and refusals were thawed and split using a riffle splitter to ≈ 1 L, and the DM was determined at 55°C in a forced draft oven for 48 hours. The dried samples were ground in a Wiley mill (Thomas Scientific, Swedesboro, NJ, USA) through a 4-mm diameter screen and then through a 1-mm screen. All chemical analyses were performed on each sample in duplicate and reported on a DM basis. The daily fecal samples were thawed, dried at 55°C in an oven for 120 hours, and ground through a 1-mm sieve (standard model 4; Arthur Thomas Co., Philadelphia, PA, USA).
To correct the chemical results to a DM basis, the analytical DM was determined by weighing 0.5 g of sample in a crucible, which was then placed in a forced air oven at 135°C for 2 hours (AOAC, 2016; method no 930.15) and hot weighed. The organic matter (OM) content was determined from the same sample as the difference between 100 and the percentage ash (AOAC, 2016; method no. 942.05). Crude fat (EE) was determined by ether extraction for 6 hours (AOAC, 2016; method 920.39; E-816 Hot Extraction Unit, Buchi Labortechnik AG, Flawil, Switzerland). The NDF and ADF concentrations were determined sequentially using an ANKOM A200 fiber analyzer (Ankom Technology, Macedon, NY), with heat-stable amylase and sodium sulfite used for the NDF analysis. Samples were ground to a fine powder using a ball grinder (Mixer Mill MM2000; Retsch GmbH, Haan, Germany) before determining nitrogen (N) and starch concentrations. The crude protein (CP) concentration (CP = N × 6.25) was determined by flash combustion, gas chromatographic separation, and thermal conductivity detection (AOAC, 2016, method 990.03; Carlo Erba Instruments, Milan, Italy). Starch concentration was determined by enzymatic hydrolysis and colorimetric detection of glucose. Uric acid in urine was determined using a colorimetric procedure (uric acid; Pointe Scientific, Inc., Canton, MI, USA), with volumes adjusted accordingly for a microplate reader (Appliskan; Thermo Electron Corporation). Allantoin was measured in urine according to the procedure of Chen and Gomes (1992) and adjusted for a microplate reader. Ruminal VFAs were quantified by gas chromatography (model 5890; Hewlett Packard, Little Falls, DE, USA), with a capillary column (30 m × 0.25 mm I.D.; 1-µm phase thickness; bonded PEG; Supelco Nukol; Sigma-Aldrich Canada, Oakville, ON, Canada) and flame ionization detection. The ruminal NH3 concentration was determined by the salicylate–nitroprusside–hypochlorite method using a flow injection analyzer (Sims et al., 1995).
2.4 Calculations and statistical analysis
Ad libitum intake was averaged by the animal from days 7 to 14 (i.e., not including total-tract digestibility and CH4 measurements). Apparent total-tract nutrient digestibility was calculated as the difference between the daily nutrient (or fraction) intake and the amount of the corresponding nutrient appearing in the feces. Rumen microbial N synthesis was estimated by the urinary PD method using the equations of Chen and Gomes (1992). For rumen pH, the raw data from the loggers were downloaded (model M5-version 755 Dascor Data Logger Software; Dascor Inc., Escondido, CA, USA) and transformed from mV recordings to pH using beginning and ending linear regressions derived from the starting and ending standardizations. The conversion of mV to pH was applied to linearly account for drift in the pH sensors over time (Penner et al., 2006). The data were summarized by 24-hour days, and mean, minimum, and maximum pH were reported. Daily CH4 emissions were calculated as described by McGinn et al. (2004) and reported as production (g/day), yield (g/kg DMI, using the DMI measured while the animals were in the chambers), and as a proportion of GE intake (Ym) according to the IPCC (2019; assuming 4.41 Mcal/kg DMI and 13.30 Mcal/kg CH4).
The normality of distribution and homogeneity of variance of the data were determined using the UNIVARIATE procedure of SAS (SAS Inst. Inc., Cary, NC). The data were analyzed using the MIXED procedure of SAS using a model that included the random effects of the square (1 and 2), the animal within the square (1, 2, and 3), the period (1, 2, and 3) within the square, the fixed effects of treatment (0, 10, and 20 g M. japonica/kg DM), and all interactions. Day (or sampling time) was considered as a repeated measure for feeding behavior, rumen pH, and chamber measurements, and the best time-series covariance structure was selected on the basis of the lowest Akaike and Bayesian information criterion. Statistical differences were declared at a P-value < 0.05 and differences between treatments with 0.05 < P < 0.10 were considered to have a tendency toward significance. Linear and quadratic effects of treatment were tested using orthogonal contrasts, and when the main effect of treatment was significant the treatment means were separated using the pdiff option of PROC MIXED (pairwise t-test). All data are presented as least squares means.
3 Results
3.1 Body weight, dry matter intake, digestibility, and eating rate
There was no effect of treatment on body weight (BW) (P = 0.35; Table 2). However, ad libitum DMI increased quadratically (P = 0.025) with the inclusion level of M. japonica such that the DMI of heifers consuming 1% was greater (P < 0.05) than that of control heifers, with those consuming 2% being intermediate and not different (P > 0.05) from the other treatments. Apparent total-tract digestibility of DM decreased linearly (P = 0.002) with the level of M. japonica added to the diet, but there were no treatment differences (P ≥ 0.36) in the digestibility of OM, CP, NDF, ADF, or starch. There was no interaction effect of treatment and day on feeding rates. The number of TMRs consumed by the heifers was similar between 0 and 9 hours post feeding (P > 0.21), but adding 1% or 2% M. japonica to the diet tended (P = 0.115) to decrease the amount consumed 9–12 hours post feeding and quadratically (P = 0.051) increased (P = 0.010) the amount consumed 12–24 hours post feeding (Table 3). Consequently, a lesser proportion of the daily intake occurred between 9 and 12 hours post feeding for heifers consuming 1% M. japonica than for control heifers (P = 0.041), with heifers consuming 2% M. japonica being intermediate and not different from the other treatment groups. A greater (P = 0.047) proportion of intake occurred 12–24 hours post feeding for heifers fed 1% or 2% M. japonica than for control heifers.
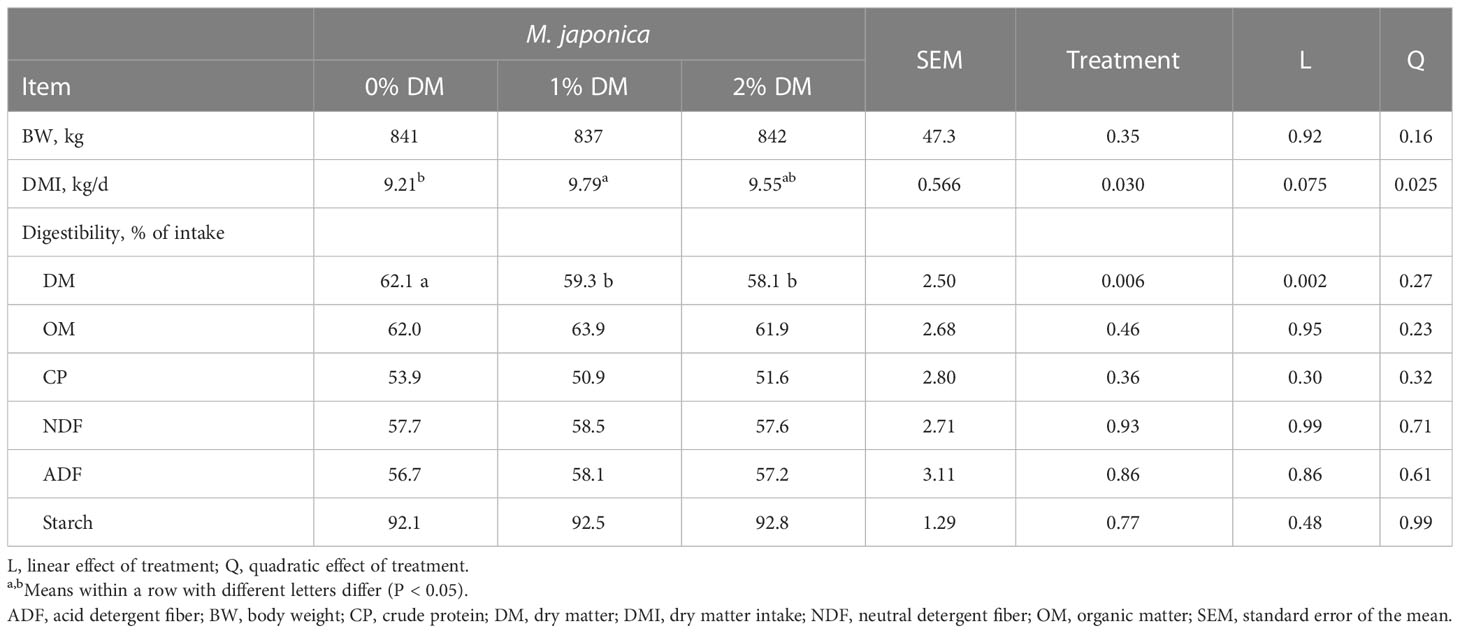
Table 2 Dry matter intake, body weight, and apparent total-tract digestibility of beef heifers receiving a forage diet supplemented with Mazzaella japonica.
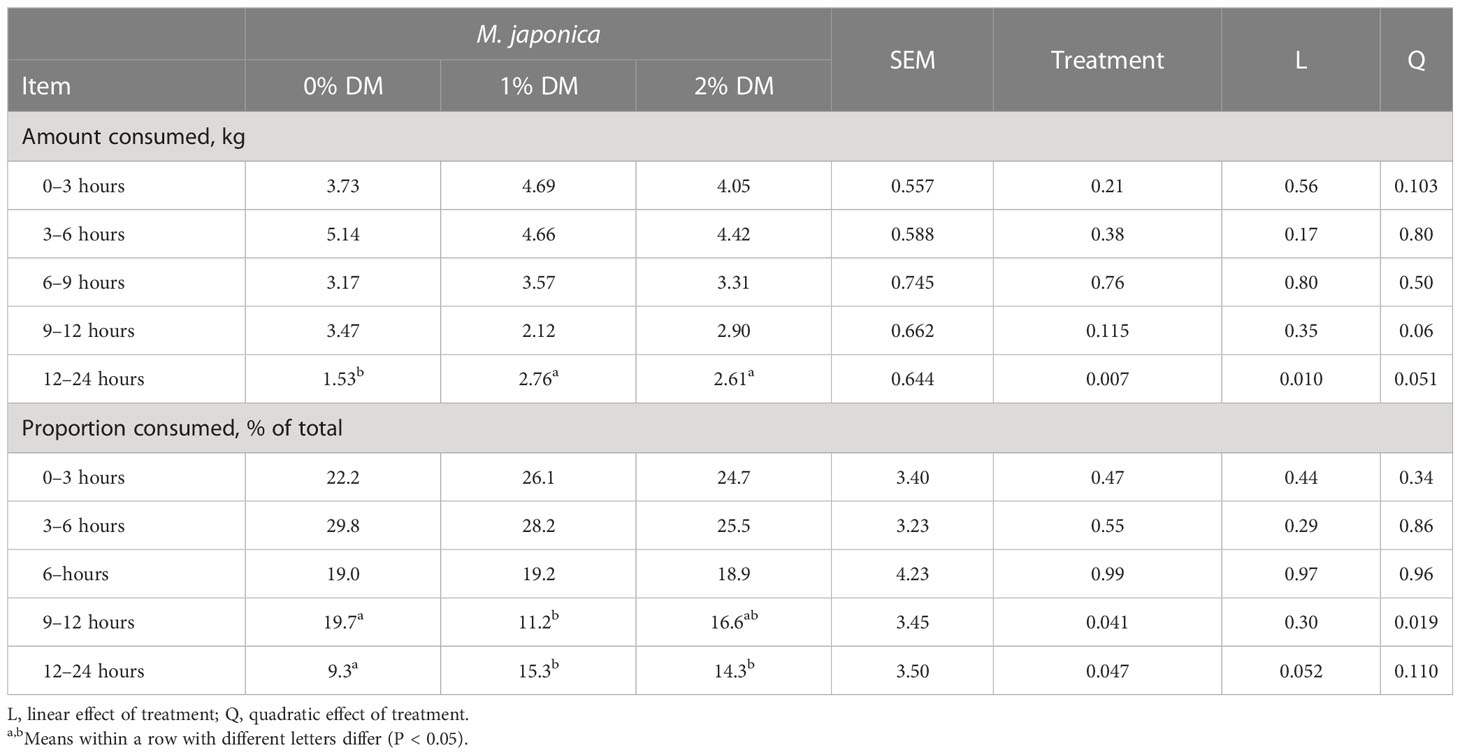
Table 3 Total mixed ration (TMR, as-is) consumption rate post feeding of beef heifers receiving a forage diet supplemented with Mazzaella japonica.
3.2 Nitrogen metabolism
Adding 1% or 2% M. japonica increased (P < 0.032) the N intake of the heifers, but there were no differences (P > 0.05) between the two inclusion levels (Table 4). Heifers fed M. japonica also had greater daily fecal output than that of control heifers (P = 0.001), with no difference between 1% and 2% M. japonica. There were no treatment differences (P = 0.95) for urinary output. Fecal N excretion linearly increased (P = 0.020), with a tendency (P = 0.076) for a quadratic response with the proportion of seaweed in the TMRs, but there were no differences in urinary N excretion (P = 0.53). The result was a tendency for a linear increase (P = 0.07) in total N excretion with increasing levels of M. japonica. Approximately 50% of the N excreted was in the feces and 50% in the urine, with no differences due to treatment (P ≥ 0.15). There were no treatment effects (P ≥ 0.82) on urinary N fractions (allantoin, uric acid), total PD, microbial PD absorbed, microbial N flow, or retained N (g/day or % of N intake).
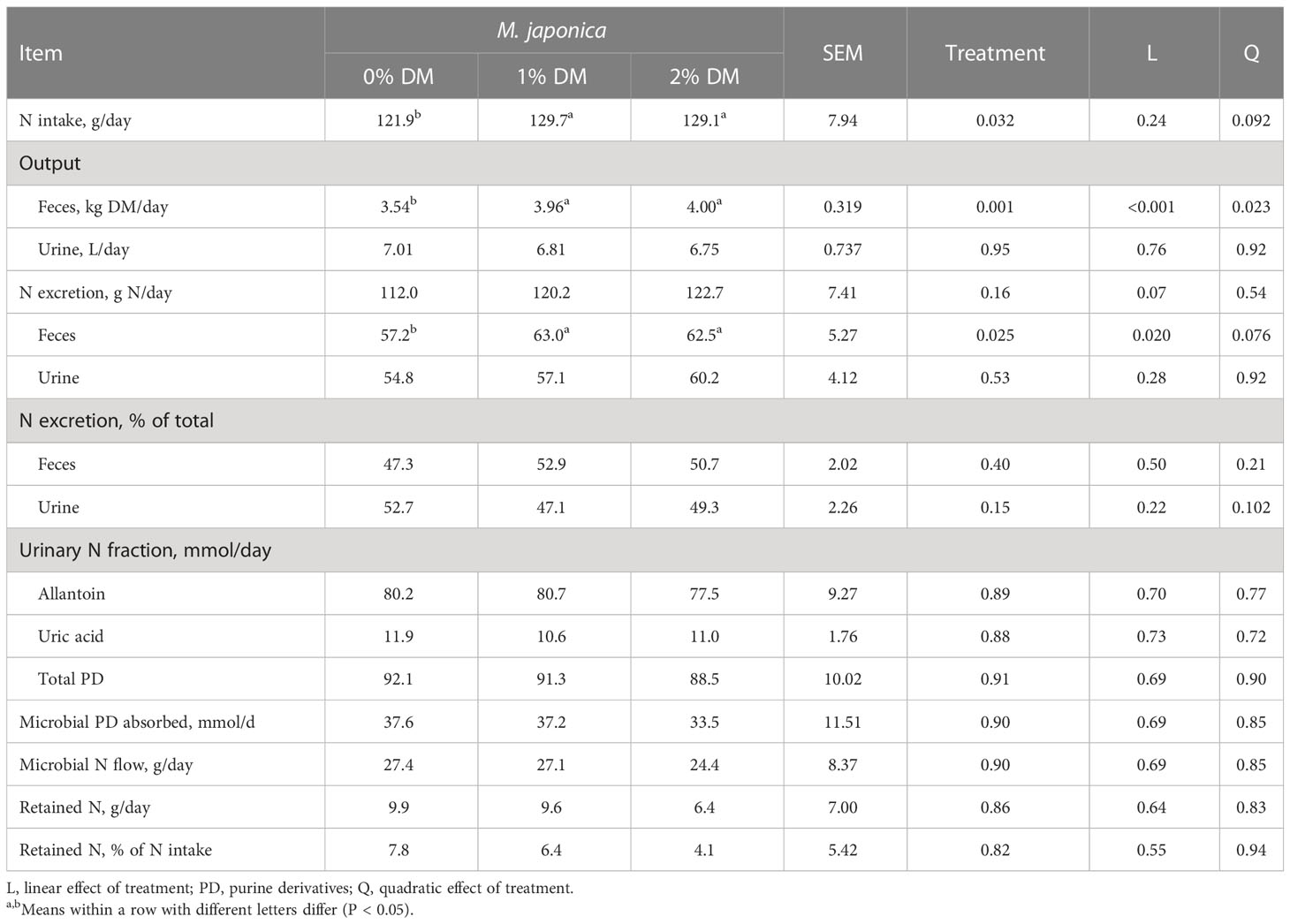
Table 4 Nitrogen intake and excretion of beef heifers receiving a forage diet supplemented with Mazzaella japonica.
3.3 Rumen pH, fermentation, and protozoa
There were no treatment effects on the minimum, mean, or maximum rumen pH (P ≥ 0.20; Table 5). The total VFA was not affected (P = 0.65) by the addition of M. japonica; however, adding M. japonica to the diet quadratically (P = 0.023) decreased the proportion of acetate at 1% inclusion. There was no combined treatment and time effect (P ≤ 0.05) on all proportions of individual and total VFA, so data are not presented by hour. The remaining individual VFA proportions were not affected by treatment (P ≥ 0.16). Adding increasing levels of M. japonica to the diet linearly (P = 0.035) increased rumen NH3-N. Protozoa counts were not affected by treatment (P = 0.70). Sampling time was significant (P < 0.001) for all VFA variables and NH3-N, except the butyrate proportion (P = 0.069), but there were no treatment × sampling time interactions (P ≥ 0.50), and, therefore, the data are not presented.
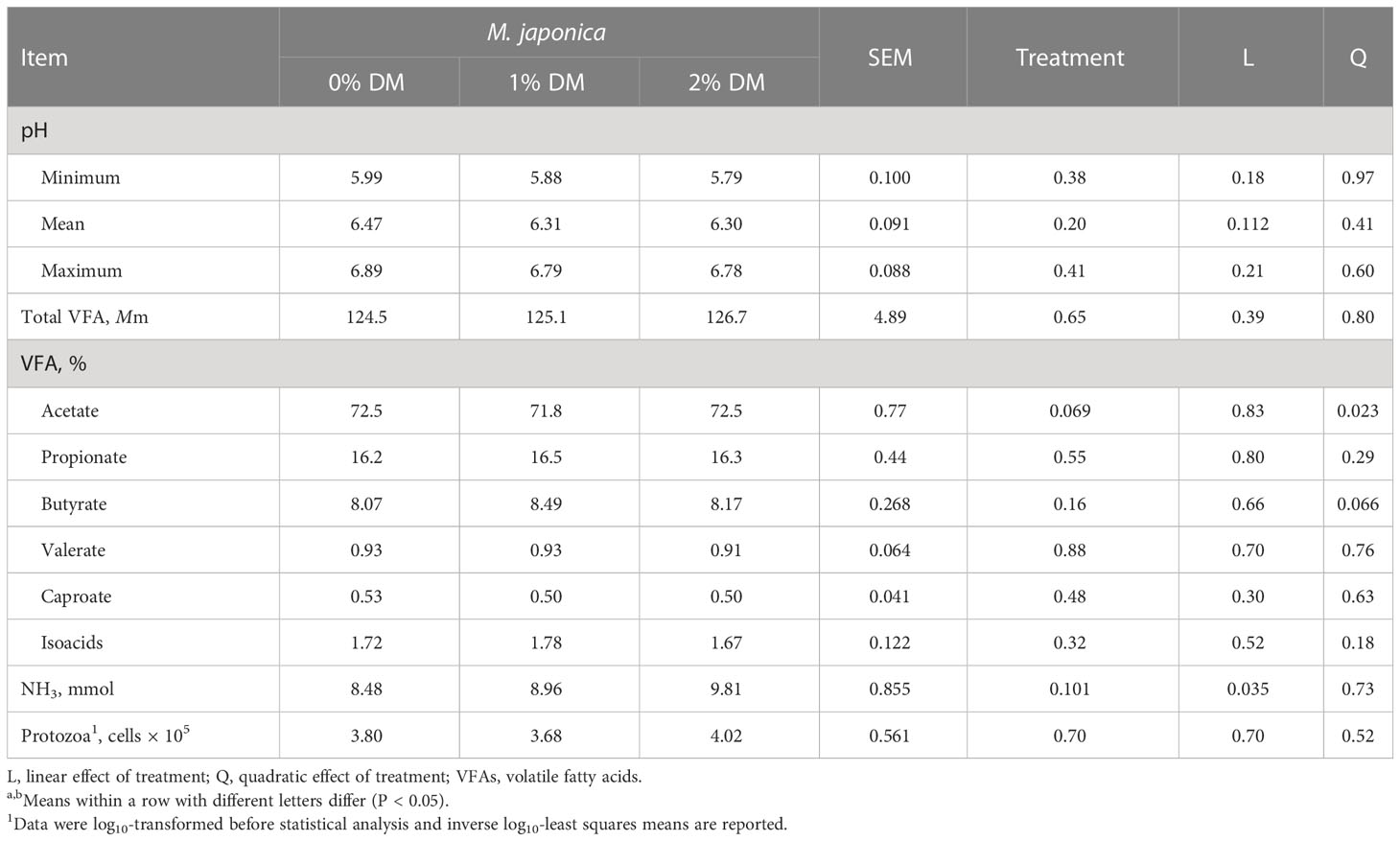
Table 5 Rumen pH, total and individual volatile fatty acids (VFAs), and protozoa enumeration for beef heifers receiving a forage diet supplemented with Mazzaella japonica.
3.4 Methane emissions
The DMI of the heifers while in the respiratory chambers was approximately 85% of the ad libitum intake, and quadratically tended (P = 0.085) to be greater in animals receiving 1% M. japonica, than in those receiving either 0% or 2% M. japonica (Table 6). Methane production (g/day) was quadratically (P = 0.041) decreased, such that the heifers receiving 2% M. japonica produced 9.2% less CH4 than the control animals. However, correcting CH4 emissions for DMI (i.e., CH4 yield) eliminated the treatment differences (P = 0.83).
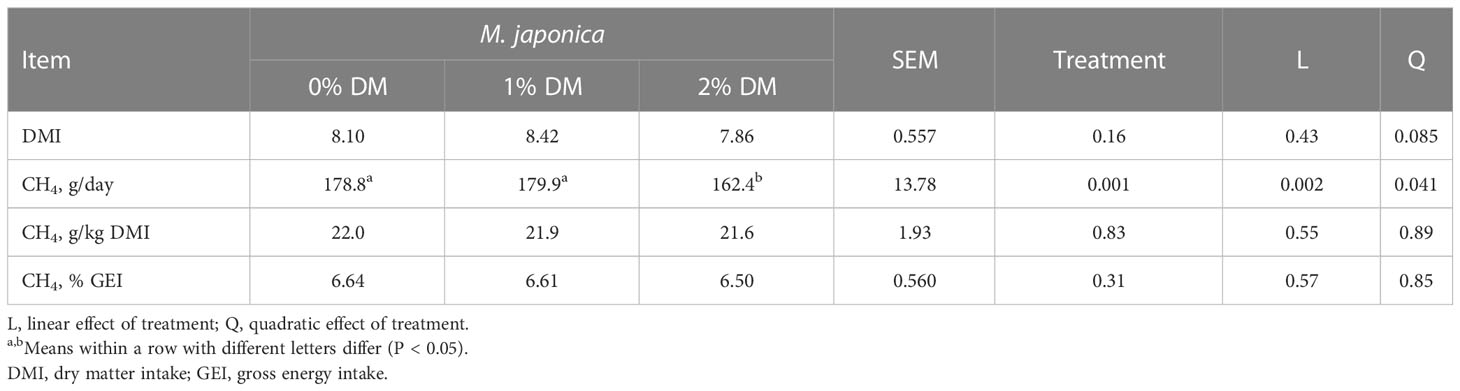
Table 6 Chamber dry matter intake (DMI) and methane (CH4) production of beef heifers receiving a forage diet supplemented with Mazzaella japonica.
4 Discussion
There is tremendous global interest in the use of seaweed (macroalgae) as a means of mitigating enteric CH4 emissions from ruminant livestock (Beauchemin et al., 2022). Most animal feeding studies have evaluated the red seaweed Asparagopsis spp. because it contains halogenated compounds that are highly effective at disrupting methanogenesis when fed in small quantities (≤ 5% of DM) to ruminant livestock (as reviewed by Lean et al., 2021). However, very few in vivo studies have examined the mitigation potential of other seaweed species, despite a number of in vitro studies showing reductions in CH4 production for a range of red, brown, and green seaweeds (Abbott et al., 2020). The CH4 mitigation effect of non-bromoform-containing seaweeds is thought to be related to the numerous secondary plant compounds they contain (Vijn et al., 2020; Abbott et al., 2020). The present study evaluated M. japonica because it is available in western Canada, and some farmers claim that feeding this seaweed to their beef cattle improves weight gain and decreases CH4 emissions. These claims are based on anecdotal evidence because controlled studies are not available to confirm or refute these assertions. M. japonica was inadvertently introduced to the waters of coastal British Columbia in the early 1900s, and in the past decade, the government of British Columbia has implemented a licensed harvest with no apparent negative environmental impacts (British Columbia Government News, 2015).
Seaweeds have been used as animal feeds for decades, especially in countries in close proximity to the sea. However, when fed at high levels, many seaweeds are known to reduce animal growth due to a lack of palatability, low digestibility of complex carbohydrates, and high inorganic matter proportion (Abbott et al., 2020). Rather than using seaweed as a major source of energy in the diet, low inclusion rates (1% and 2%) of M. japonica were used in the current study to evaluate the effects on CH4 production. These low inclusion levels had no adverse effects on the palatability or DMI of the heifers. In fact, the cattle receiving M. japonica had a slightly greater intake than that of the control cattle, which was not due to enhanced palatability given that the rates of TMR intake in the early hours post feeding were not different across the treatments. Rather, the heifers appeared to compensate for the slightly lower DM digestibility of the TMR supplemented with M. japonica by increasing DMI in the later hours post-feeding, such that intake of digestible DM was similar for all treatments (data not shown). The decreased digestibility of diets containing M. japonica was due to a less digestible inorganic fraction, as there were no treatment effects on the digestibility of the organic fraction.
As is the case with most seaweeds, M. japonica contains a relatively high inorganic fraction (ash, 28.7% DM), thus adding 1% or 2% to the TMR decreased the OM concentration of the TMR by about 1 percentage unit. M. japonica is relatively high in macrominerals: 0.68% calcium, 1.71% potassium, 0.92% magnesium, 4.09% sodium, 0.16% phosphorus, and 6.46% sulfur (Terry et al., 2022). Most ruminants require 0.18% to 0.24% DM of dietary sulfur (NASEM, 2016); thus, the high sulfur concentration of M. japonica could be a concern with inclusion rates of > 2%, especially in cases where sulfur concentration in water is high or when diets include other feed ingredients that contain high sulfur concentration (e.g., distillers’ grains). The iodine concentration in M. japonica is low, unlike that of many bromoform-containing seaweeds. Terry et al. (2022) reported that the iodine concentration of M. japonica (15.7 mg/kg DM) was substantially less than that of A. taxiformis (2,580 mg/kg DM). Elevated iodine concentration in bromoform-containing seaweeds can exceed the daily iodine intake recommendations for cattle (Roque et al., 2021) – a major limitation to the inclusion of A. taxiformis in ruminant diets.
The finding that supplementing a forage-based TMR with 1% or 2% M. japonica had no mitigating effect on methanogenesis, when CH4 was expressed relative to DMI (i.e., CH4 yield), is consistent with the lack of effects on propionate proportion, rumen pH, and protozoa numbers. It is well established that inhibiting methanogenesis shifts rumen fermentation from acetate to propionate (Ungerfeld et al., 2022), and a decrease in rumen pH lowers CH4 production (Luna et al., 1998). Ciliate protozoa produce H2, the main substrate for methanogenesis in the rumen, and, therefore, treatments that decrease protozoa numbers result in less CH4 produced (Tapio et al., 2017). The observed 9.2% decrease in daily CH4 production (g/day) observed for the 2% M. japonica treatment was attributed to the slightly lower DMI of these animals during the CH4 measurements. This finding is important because numerous in vitro studies have shown a decrease in CH4 production with various non- and low-bromoform-containing seaweeds (Abbott et al., 2020; Sofyan et al., 2022). However, in many cases, the decrease in in vitro CH4 production can be explained by lower substrate fermentability and lower gas production. Our results emphasize the need to express CH4 production on the basis of OM fermented when conducting in vitro studies, or as CH4 yield for in vivo studies, rather than as the total amount of CH4 produced. Similar to our finding, Antaya et al. (2019) reported a 10.5% decrease in CH4 production (g/day) in grazing dairy cows receiving A. nodosum, with no differences when CH4 was expressed as g/kg DMI. The lack of effect of M. japonica on CH4 yield in the current study confirms a recent in vitro rumen simulation study by Terry et al. (2022) that reported that adding 2% M. japonica to a forage-based diet had no effect on CH4, expressed on the basis of mL/day, mg/g DM disappeared, or mg/g DM incubated. Similarly, supplementing M. japonica at 2% in an in vitro RUSITEC system had no significant effects on the composition of bacterial and archaeal communities (O’Hara et al., 2022).
Compared with barley silage and straw, M. japonica is relatively high in CP concentration (20.7% DM). Owing to the relatively low CP concentration (8.3% ± 0.26) of the basal diet, adding 1% and 2% M. japonica increased the N intake of the cattle. However, fecal N excretion was also increased for heifers consuming M. japonica because the CP digestibility did not differ among the diets. A portion of dietary CP is degraded in the rumen by ruminal microorganisms to NH3-N, amino acids, and peptides, with NH3-N being the primary N source for ruminal microbial protein synthesis. The increase in NH3-N concentration in the rumen fluid with increasing supplementation of M. japonica indicates that the CP in this seaweed was rumen degradable. However, the lack of treatment effects on microbial N flow indicates that the degradable N supply was already met by the basal diet (8.48 mmol NH3-N/L). Schwab et al. (2005) suggested that bacteria require 5–11 mmol/L of ammonia-N for optimal microbial growth depending on fermentation conditions. Ammonia that is not incorporated into bacterial protein is absorbed, enters the portal vein, is transported to the liver, and is converted to urea for excretion in urine. The linear increase in urinary N excretion with increasing M. japonica is consistent with the increase in NH3-N concentration in the rumen fluid. Therefore, despite the high CP concentration of M. japonica and the relatively low CP concentration of the basal diet, supplementing with M. japonica did not improve the N utilization of the cattle, probably because the N requirement of the animals was sufficiently met by the basal diet. The cattle used in the study were at physiological BW (mean BW 840 kg) and CP requirements were low (8% CP), and, therefore, the mean N retention (mean, 6%) was low compared with that expected for younger cattle (26%, Angelidis et al., 2019; 30%, Schuba et al., 2017). It should be emphasized that N retention, calculated by subtracting N losses in refusals, feces, and urine from N intake, is considered an overestimate as other potential losses are not accounted for (e.g., epithelial cells, hair, nails, and N gas from the rumen; NASEM, 2016).
5 Conclusions
Supplementing a forage-based diet with the red seaweed M. japonica at 1% or 2% of DM failed to lower the enteric CH4 yield (g/kg DMI) of mature beef heifers. A 9.2% decrease in daily CH4 production (g/day) of cattle fed 2% M. japonica was observed, but this decrease was attributed to the slightly lower intake of these animals during the CH4 measurements. Diets containing M. japonica were slightly less digestible than the control diet because the seaweed replaced forage when incorporated into the basal diet and the M. japonica contained a relatively large inorganic fraction (28.7% of DM). The sun-dried M. japonica used in the study was highly palatable, and thus the slightly lower digestibility of diets containing seaweed was offset by the increased DMI of the cattle, such that intake of digestible DM was not different among treatments. The high CP concentration (20.7% of DM) of M. japonica increased the N intake of cattle. The CP was rumen degradable, resulting in increased NH3-N concentrations in the rumen fluid with increasing supplementation of M. japonica. Thus, we conclude that M. japonica can be used in diets to help meet the CP requirements of cattle. However, its high inorganic fraction, particularly its high sulfur concentration, may limit inclusion rates. When comprising up to 2% of the diet, M. japonica cannot be recommended as a CH4 inhibitor for beef cattle fed high-forage diets.
Data availability statement
The raw data supporting the conclusions of this article will be made available by the authors, without undue reservation.
Ethics statement
The experiment was conducted at the Beef Cattle Metabolism Facility of Agriculture and Agri-Food Canada, Lethbridge Research and Development Centre (Lethbridge, AB) in accordance with guidelines of the Canadian Council on Animal Care (2009) and was pre-approved (protocol #ACC1935) by the Institutional Animal Care and Use Committee.
Author contributions
ST conducted the statistical analyses, oversaw the study, and contributed to manuscript writing. TC conducted the respiration chamber component and reviewed the manuscript. RG contributed to the study design and reviewed the manuscript. DA contributed to the study design and reviewed the manuscript. KB procured the funding, conceptualized the study, prepared the manuscript draft, and reviewed the manuscript. All authors contributed to the article and approved the submitted version.
Funding
The study was funded by Agriculture and Agri-Food Canada.
Acknowledgments
The authors thank C. Sapsford and J.-F. Coulombe for their technical expertise, and the staff of the Beef Metabolism and Controlled Environment Facilities for caring for the cattle. Special thanks to Spencer Serin and Edgar Smith for providing the seaweed and for their macroalgae expertise.
Conflict of interest
The authors declare that the research was conducted in the absence of any commercial or financial relationships that could be construed as a potential conflict of interest.
Publisher’s note
All claims expressed in this article are solely those of the authors and do not necessarily represent those of their affiliated organizations, or those of the publisher, the editors and the reviewers. Any product that may be evaluated in this article, or claim that may be made by its manufacturer, is not guaranteed or endorsed by the publisher.
References
Abbott D. W., Aasen I. M., Beauchemin K. A., Grondahl F., Gruninger R., Hayes M., et al. (2020). Seaweed and seaweed bioactives for mitigation of enteric methane: challenges and opportunities. Animals 10, 2432. doi: 10.3390/ani10122432
Angelidis A., Crompton L., Misselbrook T., Yan T., Reynolds C. K., Stergiadis S. (2019). Evaluation and prediction of nitrogen use efficiency and outputs in faeces and urine in beef cattle. Agric. Ecosyst. Envir. 280, 1–15. doi: 10.1016/j.agee.2019.04.013
Antaya N. T., Ghelichkhan M., Pereira A. B. D., Soder K. J., Brito A. F. (2019). Production, milk iodine, and nutrient utilization in Jersey cows supplemented with the brown seaweed Ascophyllum nodosum (kelp meal) during the grazing season. J. Dairy Sci. 102, 8040–8058. doi: 10.3168/jds.2019-16478
Arndt C., Hristov A. N., Price W. J., McClelland S. C., Pelaez A. M., Cueva S. F., et al. (2022). Full adoption of the most effective strategies to mitigate methane emissions by ruminants can help meet the 1.5°C target by 2030 but not 2050. Proc. Natl. Acad. Sci. U.S.A. 119, e2111294119. doi: 10.1073/pnas.2111294119
Beauchemin K. A., McGinn S. M. (2006). Methane emissions from beef cattle: effects of fumaric acid, essential oil, and canola oil. J. Anim. Sci. 84, 1489–1496. doi: 10.2527/2006.8461489x
Beauchemin K. A., Ungerfeld E. M., Abdalla A. L., Álvarez C., Arndt C., Becquet P., et al. (2022). Invited review: current enteric methane mitigation options. J. Dairy Sci. 105, 9297–9326. doi: 10.3168/jds.2022-22091
Beauchemin K. A., Ungerfeld E. M., Eckard R., Wang M. (2020). REVIEW: fifty years of research on rumen methanogenesis - lessons learned and future challenges for mitigation. Animal 14, S1, s2–s16. doi: 10.1017/S1751731119003100
British Columbia Government News (2015) Agriculture ministry receives report on mazzaella japonica harvest. Available at: https://news.gov.bc.ca/releases/2015AGRI0032-000847 (Accessed December 1 2022).
Canadian Council on Animal Care (2009). CCAC guidelines on: the care and use of farm animals in research, teaching and testing (Ottawa, ON: Canadian Council on Animal Care).
Chen X. B., Gomes M. J. (1992). Estimation of microbial protein supply to sheep and cattle based on urinary excretion of purine derivatives – an overview of the technical details (Aberdeen, Scotland: International Feed Resources Unit, Rowett Research Institute).
Gerber P. J., Steinfeld H., Henderson B., Mottet A., Opio C., Dijkman J., et al. (2013) Tackling climate change through livestock: a global assessment of emissions and mitigation opportunities (Rome, Italy: Food and Agriculture Organization of the United Nations (FAO). Available at: http://www.fao.org/3/a-i3437e.pdf (Accessed November 21 2022).
Holden J. J., Kingzett B. C., MacNeill S., Smith W., Juanes F., Dudas S. E. (2018). Beach-cast biomass and commercial harvesting of a non-indigenous seaweed, Mazzaella japonica, on the east coast of Vancouver island, British Columbia. J. Appl. Phycol. 30, 1175–1184. doi: 10.1007/s10811-017-1321-1
Intergovernmental Panel on Climate Change (2019) Refinement to the 2006 IPCC guidelines for national greenhouse gas inventories (Geneva, Switzerland: Agriculture, Forestry And Other Land Use). IPCC). Available at: https://www.ipcc-nggip.iges.or.jp/public/2019rf/vol4.html (Accessed Novemeber 23 2022).
Katwal S., Pandya R. R., Trivedi M. M., Sorathiya K. K., Shah S. V. (2021). Antimethanogenic effects of soybean straw and seaweed (Sargassum johnstonii) based total mixed ration in crossbred cows. Indian J. Dairy Sci. 74, 498–503. doi: 10.33785/IJDS.2021.v74i06.005
Kim S. H., Lee C., Pechtl H. A., Hettick J. M., Campler M. R., Pairis-Garcia M. D., et al. (2019). Effects of 3-nitrooxypropanol on enteric methane production, rumen fermentation, and feeding behavior in beef cattle fed a high-forage or high-grain diet. J. Anim. Sci. 97, 2687–2699. doi: 10.1093/jas/skz140
Kinley R. D., Martinez-Fernandez G., Matthews M. K., de Nys R., Magnusson M., Tomkins N. W. (2020). Mitigating the carbon footprint and improving productivity of ruminant livestock agriculture using a red seaweed. J. Cleaner Prod. 259, 120836. doi: 10.1016/j.jclepro.2020.120836
Ku-Vera J. C., Castelán-Ortega O. A., Galindo-Maldonado F. A., Arango J., Chirinda N., Jiménez-Ocampo R., et al. (2020). Review: strategies for enteric methane mitigation in cattle fed tropical forages. Animal 14, S3, s453–s463. doi: 10.1017/S1751731120001780
Lean I. J., Golder H. M., Grant T. M. D., Moate P. J. (2021). A meta-analysis of effects of dietary seaweed on beef and dairy cattle performance and methane yield. PloS One 16 (7), e024905. doi: 10.1371/journal.pone.0249053
Luna R. P., Russell J. B., Van Amburgh M. E. (1998). The role of pH in regulating ruminal methane and ammonia production. J. Anim. Sci. 76, 2190–2196. doi: 10.2527/1998.7682190x
Machado L., Magnusson M., Paul N. A., Kinley R., de Nys R., Tomkins N. (2016). Identification of bioactives from the red seaweed asparagopsis taxiformis that promote antimethanogenic activity in vitro. J. Appl. Phycol. 28 (5), 3117–3126. doi: 10.1007/s10811-016-0830-7
McGinn S. M., Beauchemin K. A., Coates T., Colombatto D. (2004). Methane emissions from beef cattle: effects of monensin, sunflower oil, enzymes, yeast, and furmaric acid. J. Anim. Sci. 82, 3346–3356. doi: 10.2527/2004.82113346x
National Academies of Sciences, Engineering, and Medicine (NASEM) (2016). Nutrient requirements 598 of beef cattle. 8th (Washington, DC: The National Academies Press).
O’Hara E., Moote P., Terry S., Beauchemin K., McAllister T., Abbott D., et al. (2022). Comparative analysis of macroalgae supplementation on the rumen microbial community: asparagopsis taxiformis inhibits major ruminal methanogenic, fibrolytic, and volatile fatty acid-producing microbes in vitro. Front. Microbiol 14. doi: 10.1101/2022.09.08.507231
Penner G. B., Beauchemin K. A., Mutsvangwa T. (2006). An evaluation of the accuracy and precision of a stand-alone submersible continuous ruminal pH measurement system. J. Dairy Sci. 89, 2132–2140. doi: 10.3168/JDS.S0022-0302(06)72284-6
Roque B. M., Venegas M., Kinley R. D., de Nys R., Duarte T. L., Yang X., et al. (2021). Red seaweed (Asparagopsis taxiformis) supplementation reduces enteric methane by over 80 percent in beef steers. PloS One 16, e0247820. doi: 10.1371/journal.pone.0247820
Schuba J., Südekum K.-H., Pfeffer. E., Jayanegara A. (2017). Excretion of faecal, urinary urea and urinary non-urea nitrogen by four ruminant species as influenced by dietary nitrogen intake: a meta-analysis. Livest. Sci. 198, 82–88. doi: 10.1016/j.livsci.2017.01.017
Schwab C. G., Huhtanen P., Hunt C. W., Hvelplund T. (2005)Nitrogen requirements of cattle. In: Nitrogen and phosphorus nutrition of cattle: reducing the environmental impact of cattle operations (Wallingford, UK: CABI Digital Library). Available at: https://www.cabidigitallibrary.org/doi/book/10.1079/9780851990132.0000 (Accessed December 12 2022).
Sims G. K., Ellsworth T. R., Mulvaney R. L. (1995). Microscale determination of inorganic nitrogen in water and soil extracts. Commun. Soil Sci. Plant Anal. 26, 303–316. doi: 10.1080/00103629509369298
Smith P., Bustamante M., Ahammad H., Clark H., Dong H., Elsiddig E. A., et al. (2014). “Agriculture, forestry and other land use (AFOLU,” in Climate change 2014: mitigation of climate change: contribution of working group III to the fifth assessment report of the intergovernmental panel on climate change. Eds. Edenhofer O., PichsMadruga R., Sokona Y., Minx J. C., Farahani E., Kadner S., et al, Cambridge University Press: Cambridge, United Kingdom and New York, NY, USA. 811–922.
Sofyan A., Irawan A., Herdian H., Jasmadi, Ainsyar Harahap M., Angger Sakti A., et al. (2022). Effects of various macroalgae species on methane production, rumen fermentation, and ruminant production: a meta-analysis from in vitro and in vivo experiments. Anim. Feed Sci. Technol. 294, 115503. doi: 10.1016/j.anifeedsci.2022.115503
Tapio I., Snelling T. J., Strozzi F., Wallace R. J. (2017). The ruminal microbiome associated with methane emissions from ruminant livestock. J. Anim. Sci. Biotechnol. 8, 7. doi: 10.1186/s40104-017-0141-0
Terry S. A., Krüger A. M., Lim P. M. T., Gruninger R. J., Abbott D. W., Beauchemin K. A. (2022). Evaluation of rumen fermentation and microbial adaptation to three red seaweeds using the rumen simulation technique. Preprints.org 2022080186. doi: 10.20944/preprints202208.0186.v1
Ungerfeld E. M., Beauchemin K. A., Muñoz C. (2022). Current perspectives on achieving pronounced enteric methane mitigation from ruminant production. hypothesis and theory article. Front. Anim. Sci 2. doi: 10.3389/fanim.2021.79520
Keywords: digestibility, greenhouse gas, macroalgae, nitrogen metabolism, rumen fermentation
Citation: Terry SA, Coates T, Gruninger R, Abbott DW and Beauchemin KA (2023) Evaluation of the red seaweed Mazzaella japonica as a feed additive for beef cattle. Front. Anim. Sci. 4:1181768. doi: 10.3389/fanim.2023.1181768
Received: 07 March 2023; Accepted: 26 April 2023;
Published: 20 June 2023.
Edited by:
Rodolpho Martin do Prado, Laval University, CanadaReviewed by:
Valiollah Palangi, Ege University, TürkiyeCristina Saro, Universidad de León, Spain
Jon Schoonmaker, Purdue University, United States
Copyright © 2023 His Majesty the King in Right of Canada, as represented by the Minister of Agriculture and Agri-Food Canada for the contribution of Stephanie A Terry, Trevor Coates, Robert Gruninger, Wade Abbott, and Karen Beauchemin. This is an open-access article distributed under the terms of the Creative Commons Attribution License (CC BY). The use, distribution or reproduction in other forums is permitted, provided the original author(s) and the copyright owner(s) are credited and that the original publication in this journal is cited, in accordance with accepted academic practice. No use, distribution or reproduction is permitted which does not comply with these terms.
*Correspondence: Stephanie A. Terry, c3RlcGhhbmllLnRlcnJ5QGFnci5nYy5jYQ==
†Present address: Karen A. Beauchemin, Retired, Lethbridge, AB, Canada