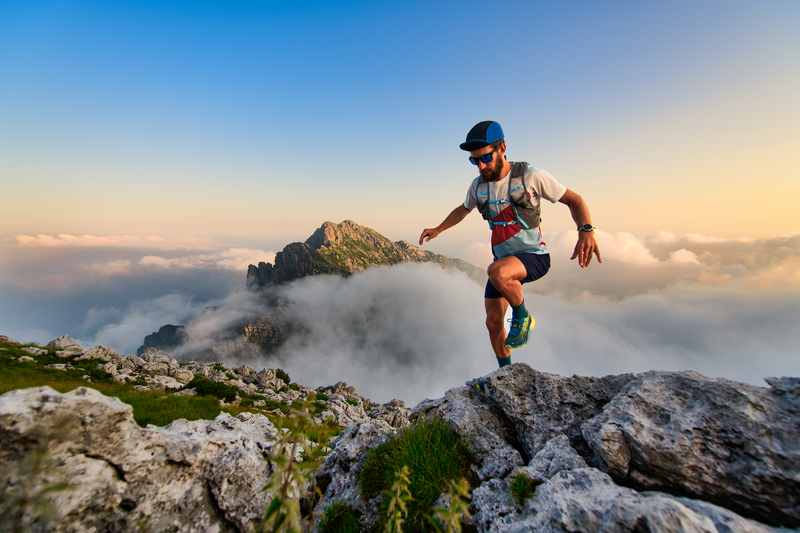
94% of researchers rate our articles as excellent or good
Learn more about the work of our research integrity team to safeguard the quality of each article we publish.
Find out more
REVIEW article
Front. Anim. Sci. , 25 August 2023
Sec. Animal Physiology and Management
Volume 4 - 2023 | https://doi.org/10.3389/fanim.2023.1169743
An expansive body of literature is dedicated to addressing the multi-faceted issue of bovine heat stress. This review firstly evaluated existing knowledge about the acute bovine heat stress response. Despite these papers being comprehensive, minor research gaps and biases somewhat limit their applicability. Categorising current management strategies under either a ‘reactive’ or ‘preventative’ approach, the review also evaluated the utility of strategies within each approach. Strategies that achieve individualised symptom detection and treatment were found to be the most practically effective reactive strategies. Genotypes that functionally increase the stability of proteins involved in the heat stress response and therefore increase an animal’s capacity for heat tolerance were identified as being among the most promising preventative strategies. Both approaches are generally limited by significant implementation costs for farmers and a lack of literature comparison between strategies for the best management outcome. A strong case is provided for further investigation into the role of mitochondrial genotypes for improved cattle heat tolerance. Overall, the review provides a balanced assessment of progress within heat stress scholarship and tenders a novel perspective that will inform further original work.
Heat stress (HS) is a complex condition caused by exposure to extreme thermal stimuli (Ames, 1980). As a condition that negatively affects cattle health, HS infringes upon the welfare status of individual animals and the herd collectively (Polsky and von Keyserlingk, 2017). Annual production losses from HS are estimated to cumulatively cost the beef and dairy industries at least $16.5mill in Australia (Sackett et al., 2006; Lees et al., 2019). Current modelling shows that increases in global temperature and heatwave extremity (Intergovernmental Panel on Climate Change, 2021) will increase the incidence of HS, with ~48% of the global cattle population predicted to be severely affected by 2100 (Carvajal et al., 2021; Thornton et al., 2021). Exacerbated by consistent production demand for an exponentially growing global population (Fróna et al., 2019; United Nations, 2019), these welfare, economic and sustainability challenges qualify cattle HS as a significant agricultural problem for beef and dairy herds worldwide (Summer et al., 2018; Lees et al., 2019).
Reflecting concern from farmers, scientists and consumers alike (Carvajal et al., 2021; Banik, 2022; Hendricks et al., 2022), HS is the subject of a large and complex body of literature. This literature aims to firstly understand the acute bovine heat stress response (HSR) that underpins HS and secondly utilise this knowledge to create and improve HS management strategies. Although both research objectives are well-established, their respective bodies of work are rarely subject to practical scrutiny. Such scrutiny is necessary to ensure that the research community continues to effectively address the issue of cattle HS. Here, we will critically assess the literature on both research objectives, with especial emphasis on practically evaluating the ‘reactive’ and ‘preventative’ management approaches that have formed in modern academia. As will be elaborated, reactive management approaches attempt to mitigate HS symptoms as they arise whereas preventative management approaches aim to pre-emptively reduce the presentation of HS symptoms. After establishing the importance of emerging preventative strategies that increase an animal’s genetic capacity for heat tolerance (HT), the rationale for a mitogenomic breeding strategy is also explored.
Cattle are homeothermic, meaning that they can only maintain normal homeostatic function within a finite range of core body temperatures (~38.0-39.0°C) (Lees et al., 2019; Liu et al., 2019). Exposure to ambient heat, humidity and/or radiation that exceeds this ‘thermoneutral zone’ (TNZ) induces a state of hyperthermia, or heightened internal temperature (Ames, 1980; Lees et al., 2019; Wang et al., 2020). The animal’s central nervous system (CNS) reacts to this stimulus by enacting a cascade of control pathways to regain euthermic balance (Collier and Gebremedhin, 2015; Collier et al., 2018). These pathways are collectively known as the HSR. Aside from structural adaptations, the acute bovine HSR – occurring 4-12h after stimulus exposure – is the foundation of an animal’s capacity to cope with HS (Horowitz, 2002; Collier and Gebremedhin, 2015; Collier et al., 2018). Within contemporary literature, the acute HSR has four progressive levels – the genomic, cellular, physiological and behavioural responses.
Upon induction of hyperthermia, the CNS activates a programmed alteration of genetic transcription patterns for various cell proteins and pathways. Most documented is the upregulation of the heat shock protein (HSP) pathway, which has been identified via RNA-sequencing of bovine transcriptomes under HS conditions (Collier et al., 2008). Included in this pathway are key transcription factors heat shock factor 1 (HSF1) and ‘activator of HSP90 ATPase activity 1’ (AHSA1), HSPs of varying size and their cochaperones including HSP40 member A1 (DNAJA1) (Srikanth et al., 2016; Sigdel et al., 2019; Garner et al., 2020; Khan et al., 2020; Fang et al., 2021). Especially noteworthy is heat shock protein 70 (HSP70), which is consistently identified as a significantly upregulated gene (Hassan et al., 2019; Kim et al., 2020). Other differentially expressed genes and therefore optimal candidates for further study include mitogen-activated protein kinase 8 interacting protein 1 (MAPK8IP1) and bradykinin receptor B1 (BDKRB1). These genes and others are related to oxidative stress, apoptosis, metabolism and signaling pathways (Sigdel et al., 2019; Garner et al., 2020). However, these studies show minimal variety in their use of cell type and cattle breeds. By limiting their scope to somatic granulosa or white blood cells, the studies further fail to capture tissue-specific expression trends that may occur in different cell types. Addressing this limitation with the investigation of various cell types allowed new insights into HS-induced differential expression (Dorji et al., 2020). Yet, the cells used were mostly sourced from lactating Holstein-Friesian (B. taurus) cows (Dorji et al., 2020). Whilst Holsteins are the most common and thus applicable dairy breed, this focus somewhat restricts conclusions by excluding more tropically adapted indicus and beef breeds. Indeed, the inclusion of both taurus and indicus beef breeds within the same expression study conducted by Morenikeji et al. (2020) supported findings that were applicable to a wider range of breeds. Such general bias towards the Holstein population, although arguably commercially justified, also prevents the discovery of potentially different and more in-depth findings in genetically different breeds. Further studies would therefore benefit from prioritising diversity of breed samples and cell types within their experimental design.
Hyperthermic onset has two main effects on bovine cells – a) the thermal inactivation and denaturation of thermosensitive proteins, and b) the overproduction of reactive oxygen species (ROS) like superoxide anions (Bernabucci et al., 2002; England et al., 2004; Jackson et al., 2006; Ganaie et al., 2013; Khan et al., 2020). These effects exacerbate each other, as antioxidant enzymes such as catalase are denatured and are unable to neutralise accumulating ROS (Oshino & Chance, 1977; Lallawmkimi, 2009; Slimen et al., 2015). In turn, ROS work to oxidise and denature more proteins.
There are multiple flow-on effects from these two immediate cellular changes. Accumulation of ROS is known to damage nuclear DNA and mitochondrial proteins within the inner mitochondrial membrane, causing mitochondrial dysfunction and oxidative stress (Higashikubo et al., 1993; Rhoads et al., 2006; Slimen et al., 2014; Mishra, 2021). Ion retention aims to preserve elements needed for cell function, as ROS-induced membrane lipid peroxidation perforates the semi-permeable lipid membrane that maintains cell balance (Mujahid et al., 2007; Baumgard and Rhoads, 2013). As evidenced by their upregulation, increased HSP production is also a key cellular response that aims to counteract the ‘leaks’ that are hindering normal cell function. Different HSPs have been found to serve different functions such as stabilising essential enzymes and membrane lipids, and neutralising ROS (Sreedhar et al., 2002; Shan et al., 2019; Mishra, 2021). Yet, the cell requires significant amounts of extra available ATP energy to maintain this altered state of function, which cannot be supplied by dysfunctional mitochondria. Ultimately, this metabolic strain activates apoptotic pathways (Katschinski et al., 2000; Bhatti et al., 2017). Although breakthrough progress has been made in identifying DNA methylation as an epigenetic factor that plays a role in the efficacy of the overarching HSR (Del Corvo et al., 2021), the specific impact of cellular epigenetic factors as another part of the cellular HSR is yet to be addressed – a minor yet important gap in knowledge that should be covered in further studies. Balancing these limitations against the depth of cellular HSR understanding, modern literature in this field is nevertheless effective.
Cellular death induced by HS manifests a whole-body need to generate more ATP energy and get rid of excess heat, thereby catalysing several physiological responses. Historically, knowledge of these responses has been subject to a blurred distinction between the acute HSR and chronic acclimation as identified by Collier et al. (2018). However, recent studies have confirmed that increased respiration rate (RR), heart rate (HR), sweat rate (SR) and core body temperature (CBT) are the main acute HS effects on cattle physiology (Brown-Brandl et al., 2005a; Wijffels et al., 2013; Galán et al., 2018; Thulani et al., 2019). Increased RR and HR aid quicker oxygen supply for aerobic respiration to cells (Thulani et al., 2019), whereas increased SR is associated with better heat evaporation from the body (Nardone et al., 2010; Islam et al., 2021). Correa-Calderon et al. also observed that blood flow is diverted to extremities such as ears to help physically evaporate heat in dairy cows (Correa-Calderon et al., 2004; Galán et al., 2018). It is generally discussed that these physiological responses are catalysed by neuro-endocrinal signals activated by cellular HS (Slimen et al., 2015; Thulani et al., 2019). Yet, knowledge of these hormonal connectors is limited to production-related responses such as increased disease susceptibility from decreased cytokine production (Steele, 2016) and redirection of nutrients from fat stores via increased leptin secretion (Morera et al., 2012). As this bias towards production indicators limits understanding of hormonal signaling pathways, more holistic research in this area is needed. Collectively, these physiological impacts were estimated to increase maintenance energy requirements by 7-25% per animal (Rezayazdi, 2001; Collier et al., 2018). However. Lees et al. (2019) highlights that the actual energy cost inclusive of responses involved in blood flow and protein synthesis is even greater. This finding reinforces the physical toll of the physiological HSR. Overall, despite minor flaws in the literature on the physiological HSR, there has been clear progress towards a more well-rounded research space.
To meet cellular and physiological metabolic demand to disperse bodily heat, cattle then exhibit coping behaviours. Many coping behaviours have been associated with the bovine HSR (Cook et al., 2007; Wijffels et al., 2013; Slimen et al., 2015; Thulani et al., 2019; Becker et al., 2020). Yet, several external variables such as ambient humidity (Becker et al., 2020) and system type (pasture, barn, feedlot etc.) (Smid et al., 2020) have been identified that affect the observed extent of such behaviours. These factors are often not incorporated into experimental design, but underpin a range of minor conflicts in research findings. For example, both decreased and increased aggressive behaviour have been reported in heat stressed cattle due to method differences in sample size, breed and location humidity (Little et al., 1980; Brown-Brandl et al., 2006; Becker et al., 2020). A review conducted by Herbut et al. (2021) effectively found that the most consistent behavioural changes in the HSR are increased standing time, panting behaviour, increased drinking, shade-seeking and reduced feed intake. Increased standing time provides more surface area for heat evaporation, whilst panting behaviour increases oxygen supply to cope with high metabolic demand (Ratnakaran et al., 2017; Galán et al., 2018). Increased drinking is suggested to help with internal cooling, shade-seeking reduces further heat exposure, and less feed intake has been found to prevent the additional heat increment of digestion (Kamal et al., 2018; Becker et al., 2020). Thus, the final symptoms of the acute HSR are well-documented.
The altered state of bodily function caused by the acute bovine HSR, and the extra energy it expends, continues until cells are able to metabolically process and disperse all excess heat for a normal internal temperature to be achieved (Collier et al., 2018). The stress upon the animal during this period can cause health, welfare and production losses and ultimately, mortality (Slimen et al., 2015). Alternatively, continued exposure to thermal stimuli can result in the activation of homeorhetic pathways indicative of a chronic heat stress response (Horowitz, 2002; Collier et al., 2018). These pathways can help ‘acclimatise’ the animal to heat exposure.
In addition to its progressive nature, the acute bovine HSR is also complicated by the range of external variables that affect response efficacy. These include not only the length of heat exposure, but also exposure intensity, age, bodyweight, condition, breed, species – B. taurus/B. indicus – and specific genotypes (Blackshaw and Blackshaw, 1994; Summer et al., 2018; Hassan et al., 2019; Lees et al., 2019; Wang et al., 2020). Generally, existing literature in this area is thorough.
There are also two overarching inconsistencies to note about existing research on the bovine HSR. Incorrect conclusions about HS impacts have been historically made due to limited access to technology and the heavy use of production indicators as measures of HS (Collier et al., 2018; Becker et al., 2020). The latter are easily confounded by animals that maintain production outputs despite HS-induced impacts on their health and welfare.
The second limitation is the overuse of the temperature-humidity index (THI) as a threshold for HS (THI > 72) and a nexus of correlation with HS indicators. This highly generalised measure of ambient conditions is known to neglect the unique conditions of the relevant herd in terms of breed, age, season etc. and similarly result in invalid conclusions (Habeeb et al., 2018; Thulani et al., 2019; Lewis Baida et al., 2021). Both limitations are notable research biases within the collective body of literature.
Overall, despite some research gaps and biases, modern literature effectively fulfills the first research goal of greater bovine HSR understanding and serves as a plausible foundation for the development of HS management strategies.
The second aim of HS research is to improve on-farm HS management. Existing strategies typically monitor a single level of the bovine HSR and reactively mitigate the respective HS symptoms. The merits of these strategies can be assessed under behavioural, physiological and cellular tiers.
Monitoring key behavioural responses to HS enables a non-invasive focus on the animal rather than their production quotas (Islam et al., 2021). Behavioural HS detection can be achieved by visual observation, using scoring systems such as the cow stress index (Ji et al., 2020). Alternatively, accelerometers can be fitted to the legs of cattle to monitor panting, standing, drinking and feed-related behavioural changes (Molfino et al., 2017; Islam et al., 2021; Nguyen, 2021). Both measures are limited by the cost and time required to either manually observe behaviours or maintain recording devices. The cost of novel digitised solutions like aerial drone and ear tag monitoring are yet to be addressed (Mufford et al., 2021). As such, the on-farm applicability of behavioural HS detection is somewhat limited by practical considerations.
Knowledge of the behavioural HSR has also been incorporated into HS amelioration strategies. As cattle seek shade to prevent heat exposure and further heat gain (Thulani et al., 2019), provision of shade structures have proved highly effective at reducing behavioural indicators of HS in animals of various ages and breeds (Blackshaw and Blackshaw, 1994; Thulani et al., 2019). Fans and sprinklers have also been found to aid evaporative heat loss (Brown-Brandl et al., 2006; Collier et al., 2006), although they have high management costs in larger operations (West, 2003; Renaudeau et al., 2011). Whilst these mitigation strategies demonstrate efficacy at reducing behavioural HS indicators, their benefit is admittedly more proven in arid rather than humid regions (Ames and Ray, 1983; Renaudeau et al., 2011; Nguyen, 2021). Other available strategies include cooling beds and misters (Yadav et al., 2016). Despite the differences in efficacy, no study has attempted to determine the best behavioural strategy or strategies for each climatic circumstance – a point that diminishes strategy applicability for farmers. More information is needed to improve the applicability of behavioural mitigation strategies.
Since manual measurement of responses such as RR, CBT and SR are difficult and time-exhaustive (Gebremedhin et al., 2008; Wijffels et al., 2013), novel technologies are being employed to optimise physiological data collection. These include attachable individual monitors for RR, HR and SR as discussed in Gebremedhin et al., 2008 and Idris et al., 2021, as well as infrared thermography drones that scan herds for elevated CBTs to indicate HS (Idris et al., 2021). Yet, these strategies require large investment in equipment and technical operation costs (Ji et al., 2020). Whether individual farms are willing to invest in such technologies is an internal decision. Several other techniques have been tested with minimal success, including the detection of hormonal changes which are confounded by multiple other stressors (Mishra, 2021). Models that attempt to predict either the risk or presence of HS in cattle from physiological responses are similarly unreliable due to variable success (Brown-Brandl et al., 2005b; Wijffels et al., 2013). Therefore, despite their cost, novel monitoring technologies are currently the most viable physiological monitoring strategy.
Various strategies can be used to ameliorate physiological HS symptoms. Technically, behavioural mitigation strategies also address the physiological HSR level. For example, shade-seeking has been found to prevent radiation exposure and further increases in CBT (Schütz et al., 2009; Lees et al., 2019). However, physiological amelioration as described in literature primarily focuses on nutritional strategies such as increasing ration energy content to accommodate higher maintenance energy costs (Mertens, 1997; Wijffels et al., 2013). Since evidence is divided over whether neutral detergent fibre content detrimentally contributes a high heat increment upon digestion that actually exacerbates physiological the HSR (Gaughan and Mader, 2009; Wijffels et al., 2013; Conte et al., 2018), more research is required in this area. Mineral salt supplements have been found to prevent the respiratory alkalosis that comes from increased RR, to varying degrees of efficacy (Sanchez et al., 1994; Wijffels et al., 2013). Although these nutritional changes can be integrated into an on-farm routine, they also neglect the inherent basal differences between individual animals and their presentation of HS symptoms. From this limitation, more individualised mitigation strategies such as specialised treatment areas (Islam et al., 2021) and individual feeding regimes (Schneider et al., 2020) are proving more effective and potentially more cost-efficient for some farming operations. Thus, whilst physiological mitigation is practical, the move towards more individualised HS treatment is of merit.
Cellular HS detection involves monitoring for cellular changes with established biomarkers linked to cellular HS. As an earlier stage of the HSR, the technique would allow for faster mitigation before larger physiological and behavioural impacts occur. This management option does not currently exist on a commercial scale, but is discussed in literature. Table 1 below outlines the key candidate biomarkers found to effectively identify cellular HS (Ganaie et al., 2013; Ganesan et al., 2017; Habashy et al., 2019).
Most potential biomarkers have only been tested on non-bovine species (Habashy et al., 2019), which highlights the need for further research into the most reliable biomarkers for cattle herds. Increased HSP activity can be confounded with multiple stressors other than cell hyperthermia (Mishra, 2021), and assaying redox potential (GSH : GSSH ratio) is cautioned against due to its lack of a consistent baseline (Flohé, 2013). Regardless, the main challenge going forward is improving the accessibility of cellular detection. Current protocol requires a precise, time-intensive and equipment-heavy process, whereas portable kits and easily interpretable data would be significantly more valuable to the everyday farmer (Habashy et al., 2019). In this regard, new innovations such as portable bioluminescent imaging (Yevtodiyenko et al., 2021) may greatly assist such development of cellular HS detection methods.
Nutritional content and supplement strategies that address various facets of the cellular HSR are at the forefront of the investigation into HS mitigation on a cellular level. To counter decreased availability of antioxidants resulting from ROS accumulation, antioxidant supplementation with Vitamins C and E have been found to successfully decrease cellular strain and therefore the scale of HS effects (Kumar et al, 2011; Wijffels et al., 2013). Studies have additionally identified chromium (Soltan, 2010), niacin (Zimbelman et al., 2010) and thiazolidinedione (Rhoads et al., 2013) as supplements that target cellular symptoms of HS. Whilst these findings are individually valuable, the literature is limited by its lack of comparison between the efficacy of the tested supplements either singularly or in combination. The studies are also somewhat limited by the lack of trial uniformity on level of heat exposure. Given that boosting bodily vitamins before HS exposure has also been found to decrease HS symptoms (Cronjé, 2005; Wijffels et al., 2013), the timing of nutrient delivery should also be investigated. Such research would ensure that feed supplementation is a precisely effective way to mitigate the cellular HSR and its bodily effects.
Overall, the reactive HS management approach is spearheading progress for on-farm HS detection and amelioration. The most promising strategies include individualised approaches and earlier targeting of the cellular HSR. Some options are limited by expense, equipment portability and/or farm system type. Further studies need to focus on overcoming these barriers and clarifying which management strategy or combination of strategies are most effective within different farm contexts. The management strategies within the reactive approach are also collectively limited by their non-addressal of the genotypes involved in the genomic HSR that affect an individual animal’s susceptibility to HS impacts. Furthermore, the reactive approach fails to provide strategies that actively prevent HS onset and progression.
The reactive approach to HS mitigation is confounded by the variable genetic status of HS susceptibility within herds and ultimately, the inability to prevent HS onset and progression (Carabaño et al., 2019; Pryce et al., 2022). Given the increasing HS susceptibility of livestock due to both climate change and selective breeding that prioritises production output (Gantner et al., 2017; Carabaño et al., 2019), academic consensus has highlighted a clear need for research into strategies enabling the prevention of cattle HS (Carabaño, 2016; Pryce et al., 2022). Thus, a preventative rationale has emerged in modern literature that aims to improve cattle tolerance to heat and HS through genomic selection of advantageous genotypes. By genotyping and breeding for inherently more heat tolerant animals in conjunction with appropriate HS detection and amelioration strategies, the negative impacts on cattle health, welfare and production may be minimised.
A heat tolerant animal is generally defined as one that has “the ability to maintain thermal stability at high temperatures and humidity” (Carabaño et al., 2019; Pryce et al., 2022) – a functional scope in principle. However, the specific definition of HT varies per different experimental purposes. Some papers define HT as coping capacity relative to the phenotypic rate of production decline (Nguyen et al., 2016; Cheruiyot et al., 2021). Notably, with recent increases in genomic HSR understanding, more papers are defining a heat tolerant animal as one with the genetic capacity to maintain thermal stability under HS stimuli (Carabaño et al., 2019; Thulani et al., 2019). This genetic scope is highly appropriate for the purposes of this review.
The first step towards breeding for cattle HT and therefore genetically preventing negative HS impacts is to identify the relevant genotypes that should be selected for. There are four interpretations of this goal identified in existing literature, discussed below.
The established genetic perspective on improving cattle HT is to prioritise breeding for structural traits that decrease the animal’s exposure to high thermal loads and therefore HS onset and progression. Given the somewhat inconsistent results from traditional crossbreeding strategies, recent literature has turned to the isolation and genetic modification of relevant genes. A prominent example is the introgression of the ‘slick’ genotype to Holstein dairy cattle, which is caused by mutations in the prolactin receptor gene and allows an animal to express shorter coat hair (Littlejohn et al., 2014; Porto-Neto et al., 2018). This short, slick coat is suggested to trap less heat (Olson et al., 2003; Dikmen et al., 2014). Similarly, alteration of a premelanosome protein gene in Holstein calves to introduce a lighter grey coat colour helps reflect solar radiation and similarly reduce the animal’s thermal exposure (Laible et al., 2021). Both coat strategies generate a desirable HT benefit by prolonging hyperthermia onset and consequent HS indicators for modified calves. However, the practical drawback left undiscussed is that lighter and/or shorter coats are conversely more susceptible to sunburn (Hodnik et al., 2021). The impacts of this trade-off, especially in high-thermal areas with minimal shade, should be investigated before implementing this genetic strategy. Moreover, improved resistance to HS stimuli cannot wholly prevent HS onset under consistent high-thermal conditions despite actively reducing heat load throughout the HSR. Therefore, there is need for the inherent efficacy of genomic HSR mechanisms to be improved. Whilst this initial interpretation towards HT has yielded advantageous genotypes, there is still scope for further research.
Another perspective of HT advantage is to breed for animals with nuclear SNP genotypes associated with greater heat tolerance. This result is achieved via genome-wide association studies (GWAS), which identify SNPs that correlate with greater levels of ‘HT’ (Luo et al., 2021). Whilst this perspective can theoretically identify SNPs associated with lower HS indicators (Halli et al., 2021), the use of easily confounded indicators such as blood cortisol concentration can mean that correlations with ‘HT’ are not necessarily significant (Idris et al., 2021). Exclusive study of the nuclear genome also neglects the potential impact of other genetic architecture such as mitochondrial DNA (mtDNA), micro RNAs and epigenetic interactions (Lee et al., 2020; Mishra, 2021). The lack of specificity around SNPs – regarding the actual genetic impact they have on the HSR and trade-off impacts on other phenotypic traits not investigated in the GWAS – is another crucial limiting factor. These barriers complicate this genotypic perspective.
An advantageous genotype has also been specifically defined as one that increases activity of key proteins and complexes within the genomic HSR for a more efficient return to euthermia upon hyperthermic onset. Various coding mutations in heat shock protein 70 (HSP70), heat shock protein 90 (HSP90) and ATPase Na+/K+ subunit alpha 1 (ATP1A1) have been predicted to do so, allowing faster deactivation of negative cellular impacts and prevention of apoptotic pathways (Liu et al., 2010; Hansen, 2013; Bhat et al., 2016; Hassan et al., 2019; Halli et al., 2021; Onasanya et al., 2021). Polymorphisms have also been found in non-coding promoter and 3’UTR regions, such as a HSP90 promoter mutation found to increase higher transcription activity upon HSP activation (Deb et al., 2013; Badri et al., 2018). Associations with other genes including HSP regulators like heat shock factor 1 and neural ligand-receptors have also been marked as potentially advantageous genotypes (Li et al., 2011; Rong et al., 2019; Cheruiyot et al., 2021; Onasanya et al., 2021). Altogether, these findings comprise a highly promising area of research. However, there is a clear bias towards HSP investigation. On the one hand, HSPs are highly crucial HSR components whereby maximising their capacity is significantly aiding the goal of HSR efficacy and thus HT capacity. On the other hand, this bias means that equally important drivers like mitochondria and neural communicators, are overlooked. Hence, despite the value of this HT interpretation, a more holistic research approach that includes other relevant HSR proteins would be appropriate.
The final known interpretation of genotypic advantage for HT advocates for greater conformational stability of proteins involved in the HSR, higher resistance to thermal inactivation and ROS oxidation and therefore greater cellular capacity to sustain function in a HS environment (van den Burg and Eijsink, 2002; Basiricò et al., 2011). Although agrigenomic research for such improved protein stability is more developed in crop species (Bita and Gerats, 2013; Fragkostefanakis et al., 2014; Wang et al., 2018), there are several mutations that have been found to affect protein stability in animal species (Basiricò et al., 2011; Saadeldin et al., 2020). This work positively identifies specific genotypic impacts on corresponding proteins. For example, a mutant Mge1 cochaperone to HSP70 was found to possess higher thermal stability and catalyse longer HSP70 refolding activity (Marada et al., 2016). However, an emphasis on HSPs is again observed. These papers also tend towards investigating thermal stability as opposed to ROS resistance – an understandable bias since knowledge surrounding ROS mechanisms has only recently been clarified (Girod et al., 2014; Chang et al., 2020). It is also important that any potential trade-offs between thermal stability and ROS resistance, or broader protein function and interactions, are identified and assessed. Overall, the rationale yields clearly beneficial genotypes with increased protein stability and function under cellular HS. Such benefits indicate the rationale’s significance to cattle HT research.
Once advantageous HT genotypes have been identified, reliable detection of these genotypes within cattle herds can be achieved via multiple techniques. These include SNP microarrays (Ventura et al., 2020), sequencing of HT-relevant genes (Koopaee and Koshkoiyeh, 2014), and whole genome sequencing (WGS) of individual samples (Liao et al., 2013). Variable cost, equipment portability and the nature of HT-relevant genotype being identified are key factors that affect the suitability of each technique (Liao et al., 2013; Ventura et al., 2020). Thus, the most effective genotyping strategy is dependent on case-by-case assessment and clarification of relevant HT genotypes.
Once HT genotypes have been profiled within a herd, their facilitation into selective breeding programs is a further point of management within the preventative approach. Notably, this is achievable via selection based on genomic estimated breeding values (GEBVs), which is a calculation of an animal’s ‘value’ based on its possession and the heritability of chosen HT genotypes (Li et al., 2018; Thulani et al., 2019). Although knowledge of HT genotypes is in its infancy, a HT GEBV is defined in literature (Nguyen et al., 2016). Facilitating Interpretation 2, the GEBV assigns HT genotypes based on SNP discrepancies associated with lesser rates of production decline during HS (Nguyen et al., 2016). Although phenotypic data is a good nexus for genetic data (Vanderweele et al., 2013), the susceptibility of production data to genetic and environmental confounders (Hayes et al., 2009) prevents a tangible link between those SNPs and their HSR benefit. As the algorithm is trained on a reference population containing only Holstein-Friesian and Jersey breed samples, it also isn’t validly applicable to beef or other dairy breeds (Nguyen et al., 2016). Further work is needed to ensure GEBVs are a robust strategy for selection of more heat tolerant breeding animals.
Overall, literature within the emerging preventative approach is effectively identifying advantageous genotypes that can prevent HS onset and prevent its detrimental impacts. The investigation of genotypes that increase protein stability and function is especially poignant. However, before consequent genotyping and breeding strategies are formulated, it is necessary to examine non-HSP players within the bovine HSR and their specific – rather than vague – impact on cattle HT. Reflecting upon the literature thus far, cellular mitochondria are arguably the most significant uninvestigated HSR component.
Mitochondria are the cellular organelles responsible for converting adenosine diphosphate (ADP) to adenosine triphosphate (ATP), the energy source that fuels all cell operations (Krebs and Johnson, 1937; Tager et al., 1983; Spinelli and Haigis, 2018; Mishra, 2021). The generation of ATP is achieved via the process of oxidative phosphorylation (OXPHOS), which involves the accumulation of a H+ proton gradient in the mitochondrial intermembrane space by enzymatic Complexes I-IV situated in the inner mitochondrial membrane (Spinelli and Haigis, 2018). Complex V uses this proton gradient to attach free phosphate groups (Pi) to ADP molecules and thus synthesise ATP for distribution and cellular use. The five complexes (comprising the electron transport chain (ETC)) and by extension the OXPHOS process is the foundation of cellular metabolism (Tager et al., 1983; Spinelli and Haigis, 2018). This function is especially important during mammalian HS, as there is greater demand for ATP energy to support the cellular HSR and general cell function in a hyperthermic environment (Zhao et al., 2002).
Upon the onset of hyperthermia, the genomic HSR enacts differential transcription of mitochondrial-related proteins, including uncoupling proteins, antioxidants such as catalase, and HSPs 70 and 27 (Bernabucci et al., 2002; Lallawmkimi, 2009; Picard et al., 2018). Collectively, these altered transcription patterns work to counter the two key HS-induced impacts of thermal protein inactivation and increased ROS production within mammalian mitochondria. Consistent exposure to these two impacts result in major effects on mitochondrial function. Mitochondrial proteins (mt-proteins) are inactivated and thereby denatured by heightened cellular temperatures (Mujahid et al., 2007; Slimen et al., 2014). ROS accumulation, exacerbated by decreased antioxidant activity, oxidises and hence damages mtDNA (Zhao et al., 2002; Bhatti et al., 2017). These detrimental effects ultimately hinder the OXPHOS efficiency of each mitochondrion. Also notable is the increased release of Ca2+ ions into the cytoplasm, which are intimately involved in intracellular communication (Ermak and Davies, 2002). Cumulatively, this complex mitochondrial dysfunction triggers the solubilisation of Cytochrome C proteins from the inner mitochondrial membrane and its BAX-mediated release into the cytoplasm (Du et al., 2008). With many mitochondria per cell, this results in cellular accumulation of Cytochrome C (Du et al., 2008; Slimen et al., 2014). Once bound to apoptotic protease-activating factors, these proteins further activate the caspases responsible for catalysing cellular death (apoptosis) (Du et al., 2008; Vakifahmetoglu-Norberg et al., 2017; Chen et al., 2020). On a whole-body scale, this action advances HS to higher-order physiological symptoms (Chen et al., 2020). See Figure 1 for a visual demonstration.
Figure 1 The effects of elevated cellular temperature on mammalian mitochondria. Created with BioRender.com.
Given the comprehensive nature of existing literature, it is clear that mitochondria are critical HSR components not only as suppliers of increased ATP demand but also as catalysts of further HS progression through HS-induced dysfunction. As mammals, this knowledge inherently applies to bovine species.
Considering the importance of mitochondrial function within acute bovine HS, it stands to reason that prolonged and/or enhanced mitochondrial function under cellular HS conditions would assist a more efficient HSR and prevent the catalysation of HS progression. It follows that animals with the genetic capacity for such sustained mitochondrial function would be able to exhibit a faster return to euthermic balance and less detrimental HS symptoms. Although this rationale is not explicitly proven, there are several circumstantial facts that point to its legitimacy. The first is that the more heat-adapted indicine cattle breeds tend to have higher levels of mitochondrial function and therefore metabolic efficiency (Hansen, 2004; Bhat et al., 2016; Collier et al., 2018). This correlation suggests a positive, potentially selection-driven connection between sustained mitochondrial function and cattle HT. The second is that increased mitochondrial function of feedlot bovines under HS conditions (via beta-adrenergic agonist supplementation) was recently found to allow supplemented animals to maintain muscle growth more efficiently than non-supplemented animals (Sieck et al., 2021). These findings are further indicative of a clear link between sustained mitochondrial function, lesser HS impacts and ultimately, greater cattle HT. These facts support an arguably strong theoretical case that the genetic capacity for sustained mitochondrial function during cellular HS is a desirable selective trait for improving cattle HT.
Due to the inherent complexity of mammalian mitochondria, it is recognised that this trait is most likely polygenic. As such, in assessing the viability of a breeding strategy centred around mitochondrial function, it is necessary to identify the multiple genotypes that most significantly contribute to the presentation of mitochondrial units that can maintain longer or better function in cellular HS conditions. Identifying the specific mitochondrial genotypes (mt-genotypes) that underpin this trait may exist in either nuclear-coded genes or genes separately encoded in the bovine mitogenome (Chinnery and Hudson, 2013). As an indicator of their relevance, both types of mitochondrial gene (mt-gene) are differentially expressed in cattle under HS conditions and/or high metabolic demand (Dorji et al., 2020). Nuclear mt-genes associated with regulatory ETC complex function have already been profiled via existing GWAS research, with some identified SNPs hypothesised to assist OXPHOS and thus mitochondrial efficiency during HS (Liu et al., 2010; Collier et al., 2017).
However, there is paucity of data about the bovine mitogenome and its genotypic impact on the configuration of mitochondrial proteins (mt-proteins), mitochondrial efficiency under HS and ultimately HT. The paucity of research effort in this space is despite multiple studies in smaller non-cattle species like songbirds that have linked mitogenomic mt-genotypes and mitochondrial HS efficiency (Lamb et al., 2018; Baker et al., 2019; Li et al., 2019). Given the hypothesised significance of mitochondrial genetics in HT, the bovine mitogenome represents a large gap in knowledge.
The bovine mitogenome was first sequenced in 1982 and is known to possess all regular features of a mammalian mitogenome (Anderson et al., 1982). These include a circular haploid structure with a heavy (H-strand) and light strand (L-strand), a highly variable D-loop region for bidirectional transcription and replication, and the mechanisms required for solely maternal mtDNA inheritance. The mitogenome codes for 13 protein-coding genes and 24 regulatory RNAs. These 13 mt-genes are ATP synthase subunit A (ATP6), ATP synthase subunit A6L (ATP8), Cytochrome C oxidase subunit 1 (COX1), Cytochrome C oxidase subunit 2 (COX2), Cytochrome C oxidase subunit 3 (COX3), Cytochrome B (CYTB), NADH-ubiquinone dehydrogenase subunit 1 (ND1), NADH-ubiquinone dehydrogenase subunit 2 (ND2), NADH-ubiquinone dehydrogenase subunit 3 (ND3), NADH-ubiquinone dehydrogenase subunit 4 (ND4), NADH-ubiquinone dehydrogenase subunit 4L (ND4L), NADH-ubiquinone dehydrogenase subunit 5 (ND5) and NADH-ubiquinone dehydrogenase subunit 6 (ND6) (Anderson et al., 1982; Chinnery and Hudson, 2013). Cumulatively, they code for structurally integral protein subunits in Complexes I, III, IV and V of the ETC (Anderson et al., 1982).
As the structural and regulatory basis of each mitochondrial unit, the mt-proteins encoded by the bovine mitogenome represent great scope for mt-genotypes that positively affect mitochondrial function under cellular HS. Yet, despite such documentation of structural features, modern literature has evolving yet relatively minimal understanding of genotypic features within the bovine mitogenome. Based on D-loop derivation of maternal lineages, there is an assumed inter-species distinction between coding B. taurus and B. indicus mtDNA (Achilli et al., 2008; Bonfiglio et al., 2010). Recently, Dorji et al. (2022) thoroughly characterised the existence and distribution of polymorphic diversity across the cattle mitogenome using 1883 animals from the 1000 Bulls Project. This paper, although highly valuable, did not frame and compare differences in polymorphic diversity between the 13 mt-genes. This information could have contributed needed understanding of which mt-genes are most relevant and perhaps under selection. Apart from the generally conserved nature of mammalian mitochondria (Chinnery and Hudson, 2013), there is similarly minimal information on past and present trends of selection within bovine mtDNA. Knowledge of such trends allow for conclusions about favoured mutations and the broader direction of evolution within the global cattle population. Moreover, understanding of these genotypic features would allow the identification of conserved non-synonymous polymorphisms that cause amino acid (AA) replacement and thus a clear impact on mt-protein structure.
There is a further gap in knowledge as to the resulting mt-protein variants that can positively impact mitochondrial function during HS. As structurally integral mitochondrial components, mt-genotypes with enhanced structure, stability and function – especially against the threat of thermal inactivation and ROS accumulation – would clearly be advantageous. These mt-genotypes may be comprised of sole SNPs or combinations of polymorphisms across multiple mt-proteins. Although non-coding regulatory regions may be equally significant in promoting greater transcription activity – akin to the third interpretation of genotypic advantage – the lack of knowledge about these mitochondrial mechanisms inhibits such investigation. Other studies have attempted to investigate mitogenomic impact on phenotypic traits with little success (Schutz et al., 1994; Srirattana et al., 2017; Alves et al., 2021). However, their use of haplotypes or ‘mtDNA profiles’ formulated from the non-coding D-loop region means that these conclusions are an indicator of correlations between phylogenetic animal divergence as opposed to the coding polymorphisms that have a tangible impact on mt-protein configuration – a barrier recognised by the papers themselves (Schutz et al., 1994; Alves et al., 2021). This research reinforces the need to bioinformatically investigate coding bovine mt-genotypes and the further mt-genotypic influence on mt-proteins, HS and HT. If certain coding mt-genotypes can be identified to exhibit a positive impact on mt-protein configuration and consequent mitochondrial function under HS conditions, they would be highly desirable selective traits for cattle HT.
Overall, mitochondria play a pivotal role within cellular HS. Breeding for mt-genotypes that can sustain mitochondrial efficiency during HS is a novel but feasible preventative strategy. Assessment of strategy viability crucially requires bioinformatic understanding of cattle mt-genotypes and investigation of their impact on the stability and function of the 13 mt-protein variants.
The acute bovine HSR is a multi-faceted pathway that underpins HS and its negative impact on cattle. Aside from a few consistent research flaws, the literature dedicated to understanding the acute bovine heat stress response is fairly comprehensive.
Both reactive and preventative strategies have a role in bovine HS management. The established ‘reactive’ approach to the goal of HS management encompasses strategies that work to detect and mitigate HS symptoms as they arise. Investigation into the efficacy of various strategy combinations and developing technology accessibility would bolster this approach. Despite its value, the reactive approach has limited scope in preventing and/or prolonging HS onset - a clear need of the field going forward.
Borne from this need, the emerging ‘preventative’ approach to HS management aims to harness the genomic HSR to breed cattle with greater capacity to prolong HS onset and improved HSR execution. Related papers are effectively exploring the existence of various advantageous genotypes. Yet, the underlying bias towards HSP research neglects the potential genetic significance of other key HSR components which should be assessed under the preventative HT rationale.
Arguably the most important unexplored HSR Components* are cellular mitochondria. The bovine mitogenome – as the genetic basis for mt-protein stability and function – is especially significant to the needed study of mitochondrial-based genotypic HT advantages. However, not only is there minimal understanding of the genotypic features that comprise mt-gene regions, but it is also unknown whether any mt-genotypes can be tangibly linked to advantages in mt-protein stability, function, mitochondrial efficiency under HS and ultimately greater HT. Such paucity of data yields opportunity for further original research.
TS – Conceptualisation, writing of the manuscript draft and creation of figures. CC and SL – Review and editing of the manuscript. All authors contributed to the article and approved the submitted version.
The authors declare that the research was conducted in the absence of any commercial or financial relationships that could be construed as a potential conflict of interest.
All claims expressed in this article are solely those of the authors and do not necessarily represent those of their affiliated organizations, or those of the publisher, the editors and the reviewers. Any product that may be evaluated in this article, or claim that may be made by its manufacturer, is not guaranteed or endorsed by the publisher.
Achilli A., Olivieri A., Pellecchia M., Uboldi C., Colli L., Al-Zahery N., et al. (2008). Mitochondrial genomes of extinct aurochs survive in domestic cattle. Curr. Biol. 18, 157–158. doi: 10.1016/j.cub.2008.01.019
Alves J. S., Diaz I. D. P. S., da Cruz V. A. R., Bastos M. S., de Oliveira L. S. M., de Albuquerque L. G., et al. (2021). The effect of mitochondrial DNA polymorphisms on cattle reproduction. Mol. Biol. Rep. 48, 1005–1008. doi: 10.1007/s11033-020-06068-0
Ames D. (1980). Thermal environment affects production efficiency of livestock. BioScience 30, 457–460. doi: 10.2307/1307947
Ames D. R., Ray D. E. (1983). Environmental manipulation to improve animal productivity. J. Anim. Sci. 57, 209–220.
Anderson S., de Bruijn M. H. L., Coulson A. R., Eperon I. C., Sanger F., Young I. G. (1982). Complete sequence of bovine mitochondrial DNA conserved features of the mamMalian mitochondrial genome. J. Mol. Biol. 156, 683–717. doi: 10.1016/0022-2836(82)90137-1
Badri T. M., Chen K. L., Alsiddig M. A., Li L., Cai Y., Wang G. L. (2018). Genetic polymorphism in hsp90AA1 gene is associated with the thermotolerance in Chinese holstein cows. Cell Stress Chaperones 23, 639–651. doi: 10.1007/s12192-017-0873-y
Baker E. P., Peris D., Moriarty R. V., Li X. C., Fay J. C., Hittinger C. T. (2019). Mitochondrial DNA and temperature tolerance in lager yeasts. Sci. Adv. 5, eaav1869. doi: 10.1126/sciadv.aav1869
Banik S. (2022). Farmer’s attitude, perception and management of heat stressed cows in selected dairy farms (Bangladesh: Faculty of Veterinary Medicine, Chattogram Veterinary and Animal Sciences University).
Basiricò L., Morera P., Primi V., Lacetera N., Nardone A., Bernabucci U. (2011). Cellular thermotolerance is associated with heat shock protein 70.1 genetic polymorphisms in holstein lactating cows. Cell Stress Chaperones 16, 441–448. doi: 10.1007/s12192-011-0257-7
Baumgard L. H., Rhoads R. P. (2013). Effects of heat stress on postabsorptive metabolism and energetics. Annu. Rev. Anim. Biosci. 1, 311–337. doi: 10.1146/annurev-animal-031412-103644
Becker C. A., Collier R. J., Stone A. E. (2020). Invited review: physiological and behavioral effects of heat stress in dairy cows. J. Dairy Sci. 8, 6751–6170. doi: 10.3168/jds.2019-17929
Bernabucci U., Ronchi B., Lacetera N., Nardone A. (2002). Markers of oxidative status in plasma and erythrocytes of transition dairy cows during hot season. J. Dairy Sci. 85, 2173–2179. doi: 10.3168/jds.S0022-0302(02)74296-3
Bhat S., Kumar P., Kashyap N., Deshmukh B., Dige M. S., Bhushan B., et al. (2016). Effect of heat shock protein 70 polymorphism on thermotolerance in tharparkar cattle. Veterinary World 9, 113–117. doi: 10.14202/vetworld.2016.113-117
Bhatti J. S., Bhatti G. K., Reddy P. H. (2017). Mitochondrial dysfunction and oxidative stress in metabolic disorders — a step towards mitochondria based therapeutic strategies. Biochim. Biophys. Acta (BBA) - Mol. Basis Dis. 1863, 1066–1077. doi: 10.1016/j.bbadis.2016.11.010
Bita C. E., Gerats T. (2013). Plant tolerance to high temperature in a changing environment: scientific fundamentals and production of heat stress-tolerant crops. Front. Plant Sci. 4. doi: 10.3389/fpls.2013.00273
Blackshaw J. K., Blackshaw A. W. (1994). Heat stress in cattle and the effect of shade on production and behaviour: A review. Aust. J. Exp. Agric. (Australia) 34, 285–295. doi: 10.1071/ea9940285
Bonfiglio S., Achilli A., Olivieri A., Negrini R., Colli L., Liotta L., et al. (2010). The enigmatic origin of bovine mtDNA haplogroup R: sporadic interbreeding or an independent event of bos primigenius domestication in Italy? PloS One 5, e15760. doi: 10.1371/journal.pone.0015760
Brown-Brandl T. M., Eigenberg R. A., Nienaber J. A. (2006). Heat stress risk factors of feedlot heifers. Livestock Sci. 105, 57–68. doi: 10.1016/j.livsci.2006.04.025
Brown-Brandl T. M., Eigenberg R. A., Nienaber J. A., Hahn G. L. (2005a). Dynamic response indicators of heat stress in shaded and non-shaded feedlot cattle. Biosyst. Eng. 90, 451–462. doi: 10.1016/j.biosystemseng.2004.12.006
Brown-Brandl T. M., Jones D. D., Woldt W. E. (2005b). Evaluating modelling techniques for cattle heat stress prediction. Biosyst. Eng. 91, 513–524. doi: 10.1016/j.biosystemseng.2005.04.003
Carabaño M. J. (2016). The challenge of genetic selection for heat tolerance: the dairy cattle example. Adv. Anim. Biosci. 7, 218–222. doi: 10.1017/s2040470016000169
Carabaño M. J., Ramón M., Menéndez-Buxadera A., Molina A., Díaz C. (2019). Selecting for heat tolerance. Anim. Front. 9, 62–68. doi: 10.1093/af/vfy033
Carvajal M. A., Alaniz A. J., Gutiérrez-Gómez C., Vergara P. M., Sejian V., Bozinovic F. (2021). Increasing importance of heat stress for cattle farming under future global climate scenarios. Sci. Total Environ. 801, 149661. doi: 10.1016/j.scitotenv.2021.149661
Chang R. L., Stanley J. A., Robinson M. C., Sher J. W., Li Z., Chan Y. A., et al. (2020). Protein structure, amino acid composition and sequence determine proteome vulnerability to oxidation-induced damage. EMBO J. 39. doi: 10.15252/embj.2020104523
Chen K.-L., Wang H.-L., Jiang L.-Z., Qian Y., Yang C.-X., Chang W.-W., et al. (2020). Heat stress induces apoptosis through disruption of dynamic mitochondrial networks in dairy cow mammary epithelial cells. In Vitro Cell. Dev. Biol. - Anim. 56, 322–331. doi: 10.1007/s11626-020-00446-5
Cheruiyot E. K., Haile-Mariam M., Cocks B. G., MacLeod I. M., Xiang R., Pryce J. E. (2021). New loci and neuronal pathways for resilience to heat stress in cattle. Sci. Rep. 11, 16619. doi: 10.1038/s41598-021-95816-8
Chinnery P. F., Hudson G. (2013). Mitochondrial genetics. Br. Med. Bull. 106, 135–159. doi: 10.1093/bmb/ldt017
Collier R. J., Baumgard L. H., Zimbelman R. B., Xiao Y. (2018). Heat stress: physiology of acclimation and adaptation. Anim. Front. 9, 12–19. doi: 10.1093/af/vfy031
Collier R. J., Collier J. L., Rhoads R. P., Baumgard L. H. (2008). Invited review: genes involved in the bovine heat stress response. J. Dairy Sci. 91, 445–454. doi: 10.3168/jds.2007-0540
Collier R. J., Dahl G. E., VanBaale M. J. (2006). Major advances associated with environmental effects on dairy cattle. J. Dairy Sci. 89, 1244–1253. doi: 10.3168/jds.S0022-0302(06)72193-2
Collier R. J., Gebremedhin K. G. (2015). Thermal biology of domestic animals. Annu. Rev. Anim. Biosci. 3, 513–532. doi: 10.1146/annurev-animal-022114-110659
Collier R. J., Renquist B. J., Xiao Y. A. (2017). 100-year review: stress physiology including heat stress. J. Dairy Sci. 100, 10367–10380. doi: 10.3168/jds.2017-13676
Conte G., Ciampolini R., Cassandro M., Lasagna E., Calamari L., Bernabucci U., et al. (2018). Feeding and nutrition management of heat-stressed dairy ruminants. Ital. J. Anim. Sci. 17, 604–620. doi: 10.1080/1828051x.2017.1404944
Cook N. B., Mentink R. L., Bennett T. B., Burgi K. (2007). The effect of heat stress and lameness on time budgets of lactating dairy cows. J. Dairy Sci. 90, 1674–1682. doi: 10.3168/jds.2006-634
Correa-Calderon A., Armstrong D., Ray D., DeNise S., Enns M., Howison C. (2004). Thermoregulatory responses of Holstein and Brown Swiss Heat-Stressed dairy cows to two different cooling systems. Int. J. Biometeorol. 48, 142–148. doi: 10.1007/s00484-003-0194-y
Cronjé P. B. (2005). Heat stress in livestock – the role of gut integrity in its aetiology and a potential role for betaine in its alleviation. Recent Adv. Anim. Nutr. Aust. 15, 107–122.
Deb R., Sajjanar B., Singh U., Kumar S., Brahmane M. P., Singh R., et al. (2013). Promoter variants at AP2 box region of Hsp70.1 affect thermal stress response and milk production traits in frieswal cross bred cattle. Gene 532, 230–235. doi: 10.1016/j.gene.2013.09.037
Del Corvo M., Lazzari B., Capra E., Zavarez L., Milanesi M., Utsunomiya Y. T., et al. (2021). Methylome patterns of cattle adaptation to heat stress. Front. Genet. 12. doi: 10.3389/fgene.2021.633132
Dikmen S., Khan F. A., Huson H. J., Sonstegard T. S., Moss J. I., Dahl G. E., et al. (2014). The SLICK hair locus derived from senepol cattle confers thermotolerance to intensively managed lactating holstein cows. J. Dairy Sci. 97, 5508–5520. doi: 10.3168/jds.2014-8087
Dorji J., Vander Jagt C. J., Chamberlain A. J., Cocks B. G., MacLeod I. M., Daetwyler H. D. (2022). Recovery of mitogenomes from whole genome sequences to infer maternal diversity in 1883 modern taurine and indicine cattle. Sci. Rep. 12, 5582. doi: 10.1038/s41598-022-09427-y
Dorji J., Vander Jagt C. J., Garner J. B., Marett L. C., Mason B. A., Reich C. M., et al. (2020). Expression of mitochondrial protein genes encoded by nuclear and mitochondrial genomes correlate with energy metabolism in dairy cattle. BMC Genomics 21. doi: 10.1186/s12864-020-07018-7
Du J., Di H. S., Guo L., Li Z. H., Wang G. L. (2008). Hyperthermia causes bovine mammary epithelial cell death by a mitochondrial-induced pathway. J. Thermal Biol. 33, 37–47. doi: 10.1016/j.jtherbio.2007.06.002
England K., O’Driscoll C., Cotter T. G. (2004). Carbonylation of glycolytic proteins is a key response to drug-induced oxidative stress and apoptosis. Cell Death Differentiation 11, 252–260. doi: 10.1038/sj.cdd.4401338
Ermak G., Davies K. J. A. (2002). Calcium and oxidative stress: from cell signaling to cell death. Mol. Immunol. 38, 713–721. doi: 10.1016/s0161-5890(01)00108-0
Fang H., Kang L., Abbas Z., Hu L., Chen Y., Tan X., et al. (2021). Identification of key genes and pathways associated with thermal stress in peripheral blood mononuclear cells of holstein dairy cattle. Front. Genet. 12. doi: 10.3389/fgene.2021.662080
Flohé L. (2013). The fairytale of the GSSG/GSH redox potential. Biochim. Biophys. Acta (BBA) - Gen. Subj. 1830, 3139–3142. doi: 10.1016/j.bbagen.2012.10.020
Fragkostefanakis S., Roth S., Schleiff E., Scharf K.-D. (2014). Prospects of engineering thermotolerance in crops through modulation of heat stress transcription factor and heat shock protein networks. Plant Cell Environ. 38, 1881–1895. doi: 10.1111/pce.12396
Fróna D., Szenderák J., Harangi-Rákos M. (2019). The challenge of feeding the world. Sustainability 11, 5816. doi: 10.3390/su11205816
Galán E., Llonch P., Villagrá A., Levit H., Pinto S., del Prado A. A. (2018). Systematic review of non-productivity-related animal-based indicators of heat stress resilience in dairy cattle. PloS One 13, e0206520. doi: 10.1371/journal.pone.0206520
Ganaie A. H., Shanker G., Bumla N. A., Ghasura R. S., Mir N. A. (2013). Biochemical and physiological changes during thermal stress in bovines. J. Veterinar Sci. Technol. 4, 126. doi: 10.4172/2157-7579.1000126
Ganesan S., Summers C. M., Pearce S. C., Gabler N. K., Valentine R. J., Baumgard L. H., et al. (2017). Short-term heat stress causes altered intracellular signaling in oxidative skeletal muscle. J. Anim. Sci. 95, 2438–2451. doi: 10.2527/jas.2016.1233
Gantner V., Bobic T., Gantner R., Gregic M., Kuterovac K., Novakovic J., et al. (2017). Differences in response to heat stress due to production level and breed of dairy cows. Int. J. Biometeorology 61, 1675–1685. doi: 10.1007/s00484-017-1348-7
Garner J. B., Chamberlain A. J., Vander Jagt C., Nguyen T. T. T., Mason B. A., Marett L. C., et al. (2020). Gene expression of the heat stress response in bovine peripheral white blood cells and milk somatic cells in vivo. Sci. Rep. 10. doi: 10.1038/s41598-020-75438-2
Gaughan J. B., Mader T. L. (2009). Effects of sodium chloride and fat supplementation on finishing steers exposed to hot and cold conditions. J. Anim. Sci. 87, 612–621. doi: 10.2527/jas.2008-1125
Gebremedhin K. G., Hillman P. E., Lee C. N., Collier R. J., Willard S. T., Arthington J. D., et al. (2008). Sweating rates of dairy cows and beef heifers in hot conditions. Trans. ASABE 51, 2167–2178. doi: 10.13031/2013.25397
Girod M., Enjalbert Q., Brunet C., Antoine R., Lemoine J., Lukac I., et al. (2014). Structural basis of protein oxidation resistance: A lysozyme study. PloS One 9, e101642. doi: 10.1371/journal.pone.0101642
Habashy W. S., Milfort M. C., Rekaya R., Aggrey S. E. (2019). Cellular antioxidant enzyme activity and biomarkers for oxidative stress are affected by heat stress. Int. J. Biometeorology 63, 1569–1584. doi: 10.1007/s00484-019-01769-z
Habeeb A. A., Gad A. E., Atta M. A. (2018). Temperature-humidity indices as indicators to heat stress of climatic conditions with relation to production and reproduction of farm animals. Int. J. Biotechnol. Recent Adv. 1, 35–50. doi: 10.18689/ijbr
Halli K., Vanvanhossou S. F., Bohlouli M., König S., Yin T. (2021). Identification of candidate genes on the basis of SNP by time-lagged heat stress interactions for milk production traits in German holstein cattle. PloS One 16, e0258216. doi: 10.1371/journal.pone.0258216
Hansen P. J. (2004). Physiological and cellular adaptations of zebu cattle to thermal stress. Anim. Reprod. Sci. 82, 349–360. doi: 10.1016/j.anireprosci.2004.04.011
Hansen P. (2013). Cellular and molecular basis of therapies to ameliorate effects of heat stress on embryonic development in cattle. Anim. Reprod. 10, 322–333.
Hassan F., Nawaz A., Rehman M. S., Ali M. A., Dilshad S. M. R., Yang C. (2019). Prospects of HSP70 as a genetic marker for thermo-tolerance and immuno-modulation in animals under climate change scenario. Anim. Nutr. 5, 340–350. doi: 10.1016/j.aninu.2019.06.005
Hayes B. J., Bowman P. J., Chamberlain A. J., Savin K., van Tassell C. P., Sonstegard T. S., et al. (2009). Validated genome wide association study to breed cattle adapted to an environment altered by climate change. PloS One 4, e6676. doi: 10.1371/journal.pone.0006676
Hendricks J., Mills K. E., Sirovica L. V., Sundermann L., Bolton S. E., von Keyserlingk M. A. G. (2022). Public perceptions of potential adaptations for mitigating heat stress on Australian dairy farms. J. Dairy Sci. 105, 5893–5908. doi: 10.3168/jds.2022-21813
Herbut P., Hoffmann G., Angrecka S., Godyń D., Vieira F. M. C., Adamczyk K., et al. (2021). The effects of heat stress on the behaviour of dairy cows – a review. Ann. Anim. Sci. 21, 385–402. doi: 10.2478/aoas-2020-0116
Higashikubo R., White R. A., Roti Roti J. L. (1993). Flow cytometric Brd Urd-pulse-chase study of heat induced cell-cycle progression delays. Cell Proliferation 26, 337–348. doi: 10.1111/j.1365-2184.1993.tb00329.x
Hodnik J. J., Jankovec M., Ježek J., Krušič Ž., Mitterhofer S., Starič J. (2021). Minimal erythema dose determination in holstein friesian cattle. Front. Veterinary Sci. 8. doi: 10.3389/fvets.2021.757452
Horowitz M. (2002). From molecular and cellular to integrative heat defense during exposure to chronic heat. Comp. Biochem. Physiol. A. Mol. Integr. Physiol. 131, 475–483. doi: 10.1016/S1095-6433(01)00500-1
Idris M., Uddin J., Sullivan M., McNeill D. M., Phillips C. J. C. (2021). Non-invasive physiological indicators of heat stress in cattle. Animals 11, 71. doi: 10.3390/ani11010071
Intergovernmental Panel on Climate Change. (2021). Intergovernmental panel on climate change working group I the physical science basis climate change 2021: working group I contribution to the sixth assessment report of the intergovernmental panel on climate change (United Nations: Intergovernmental Panel on Climate Change). Available at: https://www.ipcc.ch/report/ar6/wg1/.
Islam A., Lomax S., Doughty A., Islam M. R., Jay O., Thomson P., et al. (2021). Monitoring and mitigating heat stress in cattle. Front. Anim. Sci. 2. doi: 10.3389/fanim.2021.737213
Jackson I. L., Batinic-Haberle I., Sonveaux P., Dewhirst M. W., Vulaskovic Z. (2006). ROS production and angiogenic regulation by macrophages in response to heat therapy. Int. J. Hyperthermia 22, 263–273. doi: 10.1080/02656730600594027
Ji B., Banhazi T., Perano K., Ghahramani A., Bowtell L., Wang C., et al. (2020). Review of measuring, assessing and mitigating heat stress in dairy cattle. Biosyst. Eng. 199, 4–26. doi: 10.1016/j.biosystemseng.2020.07.009
Kamal R., Dutt T., Patel M., Dey A., Bharti P. K., Chandran P. C. (2018). Heat stress and effect of shade materials on hormonal and behavior response of dairy cattle: A review. Trop. Anim. Health Production 50, 701–706. doi: 10.1007/s11250-018-1542-6
Katschinski D., Boos K., Schindler S., Fandrey J. (2000). Pivotal role of reactive oxygen species as intracellular mediators of hyperthermia-induced apoptosis. J. Biol. Chem. 28, 21094–21098. doi: 10.1074/jbc.M001629200
Khan A., Dou J., Wang Y., Jiang X., Khan M. Z., Luo H., et al. (2020). Evaluation of heat stress effects on cellular and transcriptional adaptation of bovine granulosa cells. J. Anim. Sci. Biotechnol. 11. doi: 10.1186/s40104-019-0408-8
Kim W.-S., Ghassemi Nejad J., Roh S.-G., Lee H.-G. (2020). Heat-shock proteins gene expression in peripheral blood mononuclear cells as an indicator of heat stress in beef calves. Animals 10, 895. doi: 10.3390/ani10050895
Koopaee H. K., Koshkoiyeh A. E. (2014). SNPs genotyping technologies and their applications in farm animals breeding programs: review. Braz. Arch. Biol. Technol. 57, 87–95. doi: 10.1590/s1516-89132014000100013
Krebs H. A., Johnson W. A. (1937). Metabolism of ketonic acids in animal tissues. Biochem. J. 31, 645–660. doi: 10.1042/bj0310645
Kumar b.V.S., Ajeet K., Meena K. (2011). Effect of heat stress in tropical livestock and different strategies for its amelioration. J. Stress Physiol. Biochem. 7, 45–54.
Laible G., Cole S.-A., Brophy B., Wei J., Leath S., Jivanji S., et al. (2021). Holstein friesian dairy cattle edited for diluted coat color as a potential adaptation to climate change. BMC Genomics 22, 856. doi: 10.1186/s12864-021-08175-z
Lallawmkimi C. M. (2009). Impact of thermal stress and vitamin E supplementation on heat shock protein 72 and antioxidant enzymes in Murrah Buffaloes. [India: NDRI Deemed University, Karnal (Haryana)]. Ph. D. Thesis.
Lamb A. M., Gan H. M., Greening C., Joseph L., Lee Y., Morán-Ordóñez A., et al. (2018). Climate-driven mitochondrial selection: A test in Australian songbirds. Mol. Ecol. 27, 898–918. doi: 10.1111/mec.14488
Lee J., Lee S., Son J., Lim H., Kim E., Kim D., et al. (2020). Analysis of circulating-microRNA expression in lactating holstein cows under summer heat stress. PloS One 15, e0231125. doi: 10.1371/journal.pone.0231125
Lees A. M., Sejian V., Wallage A. L., Steel C. C., Mader T. L., Lees J. C., et al. (2019). The impact of heat load on cattle. Animals 9, 322. doi: 10.3390/ani9060322
Lewis Baida B. E., Swinbourne A. M., Barwick J., Leu S. T., van Wettere W. H. E. J. (2021). Technologies for the automated collection of heat stress data in sheep. Anim. Biotelemetry 9. doi: 10.1186/s40317-020-00225-9
Li Q.-L., Ju Z.-H., Huang J.-M., Li J.-B., Li R.-L., Hou M.-H., et al. (2011). Two novel SNPs inHSF1Gene are associated with thermal tolerance traits in Chinese holstein cattle. DNA Cell Biol. 30, 247–254. doi: 10.1089/dna.2010.1133
Li X. C., Peris D., Hittinger C. T., Sia E. A., Fay J. C. (2019). Mitochondria-encoded genes contribute to evolution of heat and cold tolerance in yeast. Sci. Adv. 5. doi: 10.1126/sciadv.aav1848
Li B., Zhang N., Wang Y.-G., George A. W., Reverter A., Li Y. (2018). Genomic prediction of breeding values using a subset of SNPs identified by three machine learning methods. Front. Genet. 9. doi: 10.3389/fgene.2018.00237
Liao X., Peng F., Forni S., McLaren D., Plastow G., Stothard P. (2013). Whole genome sequencing of gir cattle for identifying polymorphisms and loci under selection. Genome 56, 592–598. doi: 10.1139/gen-2013-0082
Little W., Collis K., Gleed P., Sansom B., Allen W., Quick A. (1980). Effect of reduced water intake by lactating dairy cows on behaviour, milk yield and blood composition. Veterinary Rec. 106, 547–551. doi: 10.1136/vr.106.26.547
Littlejohn M. D., Henty K. M., Tiplady K., Johnson T., Harland C., Lopdell T., et al. (2014). Functionally reciprocal mutations of the prolactin signalling pathway define hairy and slick cattle. Nat. Commun. 5, 5861. doi: 10.1038/ncomms6861
Liu J., Li L., Chen X., Lu Y., Wang D. (2019). Effects of heat stress on body temperature, milk production, and reproduction in dairy cows: A novel idea for monitoring and evaluation of heat stress — a review. Asian-Australasian J. Anim. Sci. 32, 1332–1339. doi: 10.5713/ajas.18.0743
Liu Y., Li D., Li H., Zhou X., Wang G. A. (2010). Novel SNP of the ATP1A1 gene is associated with heat tolerance traits in dairy cows. Mol. Biol. Rep. 38, 83–88. doi: 10.1007/s11033-010-0080-8
Luo H., Li X., Hu L., Xu W., Chu Q., Liu A., et al. (2021). Genomic analyses and biological validation of candidate genes for rectal temperature as an indicator of heat stress in holstein cattle. J. Dairy Sci. 104, 4441–4451. doi: 10.3168/jds.2020-18725
Marada A., Karri S., Singh S., Allu P. K., Boggula Y., Krishnamoorthy T., et al. (2016). Single point mutation in mitochondrial hsp70 cochaperone mge1 gains thermal stability and resistance. Biochemistry 55, 7065–7072. doi: 10.1021/acs.biochem.6b00232
Mertens D. R. (1997). Creating a system for meeting the fiber requirements of dairy cows. J. Dairy Sci. 80, 1463–1481. doi: 10.3168/jds.S0022-0302(97)76075-2
Mishra S. R. (2021). Behavioural, physiological, neuro-endocrine and molecular responses of cattle against heat stress: an updated review. Trop. Anim. Health Production 53. doi: 10.1007/s11250-021-02790-4
Molfino J., Clark C. E. F., Kerrisk K. L., García S. C. (2017). Evaluation of an activity and rumination monitor in dairy cattle grazing two types of forages. Anim. Production Sci. 57, 1557. doi: 10.1071/an16514
Morenikeji O. B., Ajayi O. O., Peters S. O., Mujibi F. D., De Donato M., Thomas B. N., et al. (2020). RNA-seq profiling of skin in temperate and tropical cattle. J. Anim. Sci. Technol. 62, 141–158. doi: 10.5187/jast.2020.62.2.141
Morera P., Basiricò L., Hosoda K., Bernabucci U. (2012). Chronic heat stress up-regulates leptin and adiponectin secretion and expression and improves leptin, adiponectin and insulin sensitivity in mice. J. Mol. Endocrinol. 48, 129–138. doi: 10.1530/jme-11-0054
Mufford J. T., Reudink M. W., Rakobowchuk M., Carlyle C. N., Church J. S. (2021). Using unmanned aerial vehicles to record behavioral and physiological indicators of heat stress in cattle on feedlot and pasture. Can. J. Anim. Sci. 102, 1–8. doi: 10.1139/cjas-2020-0125
Mujahid A., Pumford N. R., Bottje W., Nakagawa K., Miyazawa T., Akiba M., et al. (2007). Mitochondrial oxidative damage in chicken skeletal muscle induced by acute heat stress. J. Poultry Sci. 44, 439–445. doi: 10.2141/jpsa.44.439
Nardone A., Ronchi B., Lacetera N., Ranieri M. S., Bernabucci U. (2010). Effects of climate changes on animal production and sustainability of livestock systems. Livest Sci. 130, 57–69. doi: 10.1016/j.livsci.2010.02.011
Nguyen N. B. (2021). Strategies to improve dairy cow productivity and welfare in Vietnam (Australia: School of Veterinary Science, University of Queensland). PhD Thesis.
Nguyen T. T. T., Bowman P. J., Haile-Mariam M., Pryce J. E., Hayes B. J. (2016). Genomic selection for tolerance to heat stress in Australian dairy cattle. J. Dairy Sci. 99, 2849–2862. doi: 10.3168/jds.2015-9685
Olson T. A., Lucena C., Chase C. C., Hammond A. C. (2003). Evidence of a major gene influencing hair length and heat tolerance in bos taurus cattle1,2,3. J. Anim. Sci. 81, 80–90. doi: 10.2527/2003.81180x
Onasanya G. O., Msalya G. M., Thiruvenkadan A. K., Sreekumar C., Tirumurugaan G. K., Fafiolu A. O., et al. (2021). Heterozygous Single-Nucleotide Polymorphism Genotypes at Heat Shock Protein 70 Gene Potentially Influence Thermo-Tolerance among Four Zebu Breeds of Nigeria. Front. Genet. 12. doi: 10.3389/fgene.2021.642213
Oshino N., Chance B. (1977). Properties of glutathione release observed during reduction of organic hydroperoxide, demethylation of aminopyrine and oxidation of some substances in perfused rat liver, and their implications for the physiological function of catalase. Biochem. J. 162, 509–525. doi: 10.1042/bj1620509
Picard M., McEwen B. S., Epel E. S., Sandi C. (2018). An energetic view of stress: focus on mitochondria. Front. Neuroendocrinol. 49, 72–85. doi: 10.1016/j.yfrne.2018.01.001
Polsky L., von Keyserlingk M. A. G. (2017). Invited review: effects of heat stress on dairy cattle welfare. J. Dairy Sci. 100, 8645–8657. doi: 10.3168/jds.2017-12651
Porto-Neto L. R., Bickhart D. M., Landaeta-Hernandez A. J., Utsunomiya Y. T., Pagan M., Jimenez E., et al. (2018). Convergent evolution of slick coat in cattle through truncation mutations in the prolactin receptor. Front. Genet. 9. doi: 10.3389/fgene.2018.00057
Pryce J. E., Nguyen T. T. T., Cheruiyot E. K., Marett L., Garner J. B., Haile-Mariam M. (2022). Impact of hot weather on animal performance and genetic strategies to minimise the effect. Anim. Production Sci. 62, 726–735. doi: 10.1071/an21259
Ratnakaran A. P., Sejian V., Jose S., Vaswani S., Bagath M., Krishnan G., et al. (2017). Behavioral responses to livestock adaptation to heat stress challenges. Asian J. Anim. Sci. 11, 1–13. doi: 10.3923/ajas.2017.1.13
Renaudeau D., Collin A., Yahav S., de Basilio V., Gourdine J. L., Collier R. J. (2011). Adaptation to hot climate and strategies to alleviate heat stress in livestock production. animal 6, 707–728. doi: 10.1017/s1751731111002448
Rezayazdi K. (2001). Nutrient requirements of dairy cattle: seventh revised edition. 7th ed (Washington: National Academy of Sciences), ISBN: ISBN 978-0-309-06997-7.
Rhoads R. P., Baumgard L. H., Suagee J. K., Sanders S. R. (2013). Nutritional interventions to alleviate the negative consequences of heat stress. Adv. Nutr. 4, 267–276. doi: 10.3945/an.112.003376
Rhoads D. M., Umbach A. L., Subbaiah C. C., Siedow J. N. (2006). Mitochondrial reactive oxygen species. Contribution to oxidative stress and interorganellar signaling. Plant Physiol. 141, 357–366. doi: 10.1104/pp.106.079129
Rong Y., Zeng M., Guan X., Qu K., Liu J., Zhang J., et al. (2019). Association of HSF1 genetic variation with heat tolerance in Chinese cattle. Animals 9, 1027. doi: 10.3390/ani9121027
Saadeldin I. M., Swelum A. A.-A., Elsafadi M., Mahmood A., Osama A., Shikshaky H., et al. (2020). Thermotolerance and plasticity of camel somatic cells exposed to acute and chronic heat stress. J. Advanced Res. 22, 105–118. doi: 10.1016/j.jare.2019.11.009
Sackett D., Holmes P., Abbott K., Jephcott S., Barber M. (2006). Assessing the economic cost of endemic disease on the profitability of Australian beef cattle and sheep producers (Meat & Livestock Australia). Available at: https://www.mla.com.au/research-and-development/reports/2007/assessing-the-economic-cost-of-endemic-disease-on-the-profitability-of-australian-beef-cattle-and-sheep-producers/.
Sanchez W. K., McGuire M. A., Beede D. K. (1994). Macromineral nutrition by heat stress interactions in dairy cattle: review and original research. J. Dairy Sci. 77, 2051–2079. doi: 10.3168/jds.S0022-0302(94)77150-2
Schneider L., Volkmann N., Kemper N., Spindler B. (2020). Feeding behavior of fattening bulls fed six times per day using an automatic feeding system. Front. Veterinary Sci. 7. doi: 10.3389/fvets.2020.00043
Schutz M. M., Freeman A. E., Lindberg G. L., Koehler C. M., Beitz D. C. (1994). The effect of mitochondrial DNA on milk production and health of dairy cattle. Livestock Production Sci. 37, 283–295. doi: 10.1016/0301-6226(94)90123-6
Schütz K. E., Rogers A. R., Cox N. R., Tucker C. B. (2009). Dairy cows prefer shade that offers greater protection against solar radiation in summer: shade use, behaviour, and body temperature. Appl. Anim. Behav. Sci. 116, 28–34. doi: 10.1016/j.applanim.2008.07.005
Shan Q., Ma F., Wei J., Li H., Ma H., Sun P. (2019). Physiological functions of heat shock proteins. Curr. Protein Pept. Sci. 20. doi: 10.2174/1389203720666191111113726
Sieck R. L., Reith R. R., Fuller A. M., Grijalva P. C., Treffer L. K., Swanson R. M., et al. (2021). Beta-adrenergic agonists and heat stress impact skeletal muscle gene expression and mitochondrial function in beef cattle. Trans. Anim. Sci. 5, 164–169. doi: 10.1093/tas/txab157
Sigdel A., Abdollahi-Arpanahi R., Aguilar I., Peñagaricano F. (2019). Whole genome mapping reveals novel genes and pathways involved in milk production under heat stress in US holstein cows. Front. Genet. 10. doi: 10.3389/fgene.2019.00928
Slimen I., Najar T., Ghram A., Abdrrabba M. (2015). Heat stress effects on livestock: molecular, cellular and metabolic aspects, a review. J. Anim. Physiol. Anim. Nutr. 100, 401–412. doi: 10.1111/jpn.12379
Slimen I. B., Najar T., Ghram A., Dabbebi H., Ben Mrad M., Abdrabbah M. (2014). Reactive oxygen species, heat stress and oxidative-induced mitochondrial damage. A review. Int. J. Hyperthermia 30, 513–523. doi: 10.3109/02656736.2014.971446
Smid A. M. C., Weary D. M., von Keyserlingk M. A. G. (2020). Effect of outdoor open pack space allowance on the behavior of freestall-housed dairy cows. J. Dairy Sci. 103, 3422–3430. doi: 10.3168/jds.2019-17066
Soltan M. A. (2010). Effect of dietary chromium supplementation on productive and reproductive performance of early lactating dairy cows under heat stress. J. Anim. Physiol. Anim. Nutr. 94, 264–272. doi: 10.1111/j.1439-0396.2008.00913.x
Spinelli J. B., Haigis M. C. (2018). The multifaceted contributions of mitochondria to cellular metabolism. Nat. Cell Biol. 20, 745–754. doi: 10.1038/s41556-018-0124-1
Sreedhar A. S., Pardhasaradhi B. V. V., Khar A., Srinivas U. K. (2002). A cross talk between cellular signaling and cellular redox state during heat-induced apoptosis in a rat histiocytoma. Free Radic. Biol. Med. 32, 221–227. doi: 10.1016/s0891-5849(01)00796-1
Srikanth K., Kwon A., Lee E., Chung H. (2016). Characterization of genes and pathways that respond to heat stress in holstein calves through transcriptome analysis. Cell Stress Chaperones 22, 29–42. doi: 10.1007/s12192-016-0739-8
Srirattana K., McCosker K., Schatz T., St. John J. C. (2017). Cattle phenotypes can disguise their maternal ancestry. BMC Genet. 18, 59. doi: 10.1186/s12863-017-0523-5
Steele M. (2016). Does heat stress affect immune function in dairy cows? Veterinary Evidence 1. doi: 10.18849/ve.v1i3.39
Summer A., Lora I., Formaggioni P., Gottardo F. (2018). Impact of heat stress on milk and meat production. Anim. Front. 9, 39–46. doi: 10.1093/af/vfy026
Tager J. M., Groen A. K., Wanders R. J., Duszynski J., Westerhoff H. V., Vervoorn R. C. (1983). Control of mitochondrial respiration. Biochem. Soc. Trans. 11, 40–43. doi: 10.1042/bst0110040
Thornton P., Nelson G., Mayberry D., Herrero M. (2021). Increases in extreme heat stress in domesticated livestock species during the twenty-first century. Global Change Biol. 27, 5762–5772. doi: 10.1111/gcb.15825
Thulani S., Maliviwe M., Peter Ayodeji I. (2019). Heat tolerance level in dairy herds: A review on coping strategies to heat stress and ways of measuring heat tolerance. J. Anim. Behav. Biometeorology 7, 39–51. doi: 10.31893/2318-1265jabb.v7n2p39-51
United nations (2019). world population prospects 2019: highlights (New York: Department of Economic and Social Affairs Population Division).
Vakifahmetoglu-Norberg H., Ouchida A. T., Norberg E. (2017). The role of mitochondria in metabolism and cell death. Biochem. Biophys. Res. Commun. 482, 426–431. doi: 10.1016/j.bbrc.2016.11.088
van den Burg B., Eijsink V. G. (2002). Selection of mutations for increased protein stability. Curr. Opin. Biotechnol. 13, 333–337. doi: 10.1016/S0958-1669(02)00325-7
Vanderweele T. J., Ko Y.-A., Mukherjee B. (2013). Environmental confounding in gene-environment interaction studies. Am. J. Epidemiol. 178, 144–152. doi: 10.1093/aje/kws439
Ventura R. V., Brito L. F., Oliveira G. A., Daetwyler H. D., Schenkel F. S., Sargolzaei M., et al. (2020). A comprehensive comparison of high-density SNP panels and an alternative ultra-high-density panel for genomic analyses in nellore cattle. Anim. Production Sci. 60, 333. doi: 10.1071/an18305
Wang J., Li J., Wang F., Xiao J., Wang Y., Yang H., et al. (2020). Heat stress on calves and heifers: A review. J. Anim. Sci. Biotechnol. 11. doi: 10.1186/s40104-020-00485-8
Wang J., Yuan B., Xu Y., Huang B. (2018). Differential responses of amino acids and soluble proteins to heat stress associated with genetic variations in heat tolerance for hard fescue. J. Am. Soc. Hortic. Sci. 143, 45–55. doi: 10.21273/jashs04246-17
West J. W. (2003). Effects of heat-stress on production in dairy cattle. J. Dairy Sci. 86, 2131–2144. doi: 10.3168/jds.s0022-0302(03)73803-x
Wijffels G., Gaughan J., Allingham P. (2013). Heat stress nutrition: A comprehensive review and R&D program (Australia: Meat and Livestock Australia).
Yadav B., Pandey V., Yadav S. (2016). Effect of misting and wallowing cooling systems on milk yield, blood and physiological variables during heat stress in lactating Murrah buffalo. J. Anim. Sci. Technol. 58, 2. doi: 10.1186/s40781-015-0082-0
Yevtodiyenko A., Bazhin A., Khodakivskyi P., Godinat A., Budin G., Maric T., et al. (2021). Portable bioluminescent platform for in vivo monitoring of biological processes in non-transgenic animals. Nat. Commun. 12. doi: 10.1038/s41467-021-22892-9
Zhao Q., Wang J., Levichkin I. V., Stasinopoulos S., Ryan M. T., Hoogenraad N. J. A. (2002). Mitochondrial specific stress response in mammalian cells. EMBO J. 21, 4411–4419. doi: 10.1093/emboj/cdf445
Keywords: cattle, heat stress, heat tolerance, management strategies, genotypic selection
Citation: Scerri TM, Lomax S and Clark CEF (2023) Bovine heat stress management: current amelioration approaches and the case for a novel mitogenomic strategy. Front. Anim. Sci. 4:1169743. doi: 10.3389/fanim.2023.1169743
Received: 20 February 2023; Accepted: 09 August 2023;
Published: 25 August 2023.
Edited by:
Mohan Mondal, ICAR-National Dairy Research Institute, IndiaReviewed by:
Veronique Ouellet, Laval University, CanadaCopyright © 2023 Scerri, Lomax and Clark. This is an open-access article distributed under the terms of the Creative Commons Attribution License (CC BY). The use, distribution or reproduction in other forums is permitted, provided the original author(s) and the copyright owner(s) are credited and that the original publication in this journal is cited, in accordance with accepted academic practice. No use, distribution or reproduction is permitted which does not comply with these terms.
*Correspondence: Tiarna Macey Scerri, dHNjZTA2MzVAdW5pLnN5ZG5leS5lZHUuYXU=
Disclaimer: All claims expressed in this article are solely those of the authors and do not necessarily represent those of their affiliated organizations, or those of the publisher, the editors and the reviewers. Any product that may be evaluated in this article or claim that may be made by its manufacturer is not guaranteed or endorsed by the publisher.
Research integrity at Frontiers
Learn more about the work of our research integrity team to safeguard the quality of each article we publish.