- 1Department of Animal Science, Texas A&M University and Texas A&M AgriLife Research, College Station, TX, United States
- 2Texas A&M AgriLife Research and Extension Center, Overton, TX, United States
In cattle, prenatal transportation stress has been associated with differential methylation of genes related to metabolism, but the effects of prenatal transportation stress on skeletal muscle mitochondria and oxidative stress have not been investigated. We tested the hypothesis that prenatally stressed calves would exhibit increased skeletal muscle mitochondrial function resulting in greater oxidative stress than calves from non-stressed dams. Serum and longissimus thoracis muscle samples were collected from yearling Brahman calves whose mothers were stressed by transportation at five time points during gestation [i.e., prenatally stressed (PNS); eight bulls and six heifers] and control calves (CON; four bulls and six heifers). Serum was evaluated for concentration of the stress hormone, cortisol and for a marker of muscle perturbation, creatine kinase activity. Muscle samples were analyzed for concentration of a by-product of lipid peroxidation, malondialdehyde, and activity of the antioxidants, superoxide dismutase and glutathione peroxidase. Additionally, muscle mitochondrial volume density and function were estimated by citrate synthase and cytochrome c oxidase activities, respectively. Data were analyzed using mixed linear models with sex, treatment, and the sex × treatment interaction as fixed effects. No investigated variable differed between CON and PNS calves (p ≥ 0.3). These data suggest that prenatal transportation stress does not have an impact on skeletal muscle mitochondrial metabolism or markers of stress or muscle damage in Brahman yearling calves at rest. However, previously reported negative impacts of prenatal stress on inflammatory responses suggest that PNS calves may be differentially equipped to handle an acute stressor. Future research should investigate the energetic and inflammatory implications of acute stressors in animals subjected to prenatal stress.
1 Introduction
Prenatal and early-life stressors have been associated with alterations in postnatal behavior, health, and metabolism in several species (De Kloet et al., 2005; Collier et al., 2011; Danese and McEwen, 2012; Dahl et al., 2016). In livestock, stressors such as under- or overfeeding, heat, and transportation during pregnancy may negatively affect the offspring. Outcomes of prenatal stress include suppressed immune function (Otten et al., 2015), elevated cytokine responses to a stressor (Littlejohn et al., 2019), impaired growth (Posont and Yates, 2019), and altered mitochondrial metabolism (Pillai et al., 2016).
Transportation of cattle, in particular, is often a necessity in the beef industry due to the locations of various production operations, the availability of grazing resources, and the worldwide demand for beef. Transportation is a stress stimulus, which can have detrimental effects on the health and wellbeing of animals (Minka and Ayo, 2009; Chen et al., 2015). Transportation stress while in utero increased pituitary gland weight in fetal calves (Lay et al., 1997b) and delayed clearance of exogenous cortisol in suckling calves (Lay et al., 1997a). Additionally, calves subjected to transportation-induced prenatal stress had increased serum cortisol concentrations and were more temperamental (as assessed by pen score and exit velocity at weaning) compared with calves from non-stressed dams (Littlejohn et al., 2016). In a separate study of non-transported cattle, we demonstrated that temperamental heifers had increased serum cortisol concentrations and lesser skeletal muscle efficiency of mitochondrial coupling of oxidative phosphorylation and electron transfer, as well as altered antioxidant activities, compared with calm heifers (Owen et al., 2020). Collectively, these results suggest that animals that were stressed by transport in utero may have impaired energetics and an elevated oxidative load. However, the effects of prenatal transportation stress on skeletal muscle mitochondria and oxidative stress have not been evaluated.
Our prior study of the effects of prenatal transportation stress on the methylation of deoxyribonucleic acid (DNA) in peripheral blood leukocytes of suckling bulls and heifers revealed differential methylation of genes associated with behavior, immunity, stress response, and metabolism (Littlejohn et al., 2018; Baker et al., 2020; Littlejohn et al., 2020). Of special interest was the finding that metabolically important genes were affected by prenatal transportation stress (Littlejohn et al., 2018). Subsequent evaluation of the dataset determined that cytochrome c oxidase assembly factor (COX14) and creatine kinase, mitochondrial 1B (CKMT1B) were hypomethylated and cytochrome c oxidase assembly factor 5 (COA5), cytochrome c oxidase subunit 5A (COX5A), and nuclear respiratory factor 1 (NRF1) were hypermethylated (Table 1; Littlejohn et al., unpublished observation). This surrogate analysis of DNA methylation in leukocytes predicts that hypomethylated genes would lead to increased gene expression and hypermethylated genes would lead to reduced gene expression in skeletal muscle, and, furthermore, suggests that bovine skeletal muscle mitochondria may be impacted by prenatal transportation stress.
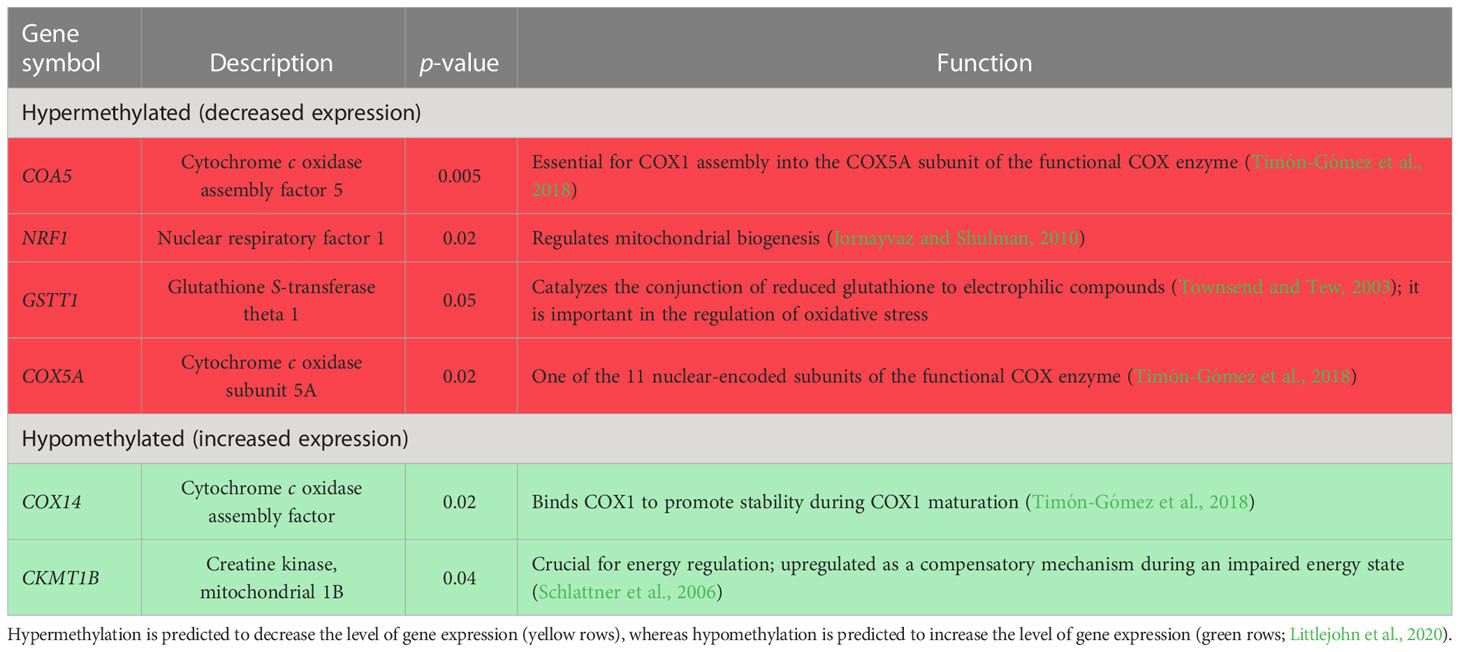
Table 1 Genes either hyper- or hypomethylated in leukocytes of previously studied suckling prenatally stressed (PNS) bulls compared with non-stressed control bulls.
Thus, this study aimed to identify the impact of prenatal transportation stress on skeletal muscle mitochondria, antioxidant activity, lipid peroxidation, muscle damage, and serum cortisol in yearling Brahman bulls and heifers. We hypothesized that prenatally stressed yearling cattle would have greater skeletal muscle mitochondrial volume density and function than non-stressed yearling cattle. Additionally, as elevated mitochondrial volume density may result in increased reactive oxygen species (ROS) production, we hypothesized that mitochondrial adaptations would elevate markers of oxidative stress and muscle damage.
2 Materials and methods
All procedures were conducted in compliance with the Guide for the Care and Use of Agricultural Animals in Research and Teaching (FASS, 2010), and its earlier versions, and approved by the Texas A&M AgriLife Research Agricultural Animal Care and Use Committee (AUP 2017-006A and 2018-017A).
2.1 Cattle
Purebred registered yearling Brahman bulls and heifers whose dams were either (a) exposed to transportation during gestation as a prenatal stressor; or (b) not transported during gestation were used in this study. Details of this stress model were previously described by Lay et al. (1996); Price et al. (2015), and Littlejohn et al. (2016). Briefly, for this study, pregnant Brahman cows (n = 37) were transported in a trailer for 2-h periods at 60 ± 5 d, 80 ± 5 d, 100 ± 5 d, 120 ± 5 d, and 140 ± 5 d of gestation. These cows were artificially inseminated to a single sire following naturally occurring estrus and confirmed as having conceived during the week they reached 45 days after insemination in 2018. The earliest time point to initiate transportation stress (i.e., d 60) was selected to be after placentation was completed in the cow in order to minimize the risk of inducing early embryonic or fetal loss (Schlafer et al., 2000). The latest time point to apply transportation stress (d 140; i.e., the middle trimester of gestation) was selected to be before a stress-induced elevation in the secretion of fetal adrenal cortisol might induce premature parturition in the cow (Senger, 2003; Hopper, 2015). Additionally, these days aligned with the ontogeny of skeletal muscle cells in fetal calves, from the peak of primary myogenesis to the peak of secondary myogenesis (Bonnet et al., 2010; Du et al., 2010). These stages of gestation are windows of susceptibility when prenatal stressors, such as undernutrition or drought, may limit the number of muscle fibers available for hypertrophy in postnatal life (Du et al., 2010). Non-transported pregnant cows (n = 35) were designated as a control group. These cows were artificially inseminated to the same single sire as the transported cows. Transported and control cows were maintained together under the same nutritional conditions in the same coastal bermudagrass and ryegrass pastures at the Texas A&M AgriLife Research and Extension Center in Overton, TX, USA.
In the spring of 2019, the 37 pregnant transported cows gave birth to a total of 16 bull and 14 heifer calves (prenatally stressed group; PNS), and the pregnant cows that were maintained as controls gave birth to 12 bull and 14 heifer calves (control group; CON). Control and PNS bull and heifer calves were maintained with their dams as cow–calf units together under the same nutritional conditions in the same coastal bermudagrass and ryegrass pastures at the Texas A&M AgriLife Research and Extension Center in Overton, TX, USA, from birth through to weaning in the fall of 2019. At weaning, calves received vaccinations to prevent clostridial and respiratory diseases. Weaned calves were maintained together in the same coastal bermudagrass and ryegrass pastures from weaning through to yearling age (early 2020). At that time, a subset of the PNS and CON Brahman calves were available for assignment to the study reported herein. Specifically, the CON group consisted of four bulls and six heifers; the PNS group consisted of eight bulls and six heifers.
2.2 Sample collection
On the day of collection, all animals (11.3 ± 0.1 months of age) were moved into processing pens in groups by sex nearest a covered squeeze chute. Blood and muscle samples were collected from all animals on the same day within a 5-h time period from 10:00 to 15:00. Blood was collected by jugular venipuncture into evacuated tubes without anticoagulant (Vacutainer™; Becton, Dickinson and Co., Franklin Lanes, NJ, USA). Blood samples were allowed to clot for 6 h at 4°C, then centrifuged at 1,700 × g for 30 min to harvest serum. Serum aliquots were stored at −80°C until the analyses of cortisol concentrations and creatine kinase (CK) activity.
Muscle tissue samples were collected from the longissimus thoracis (LT) of each animal, as previously described (Owen et al., 2020). Following cleaning of the site with povidone scrub and 70% ethanol, a 14-gauge needle was used to create the initial puncture through the skin, then tissue was collected using a 14-gauge tissue collection tool (SuperCore™ Semi-Automatic Biopsy Instrument; Argon Medical Devices, Frisco, TX, USA) inserted to a standardized depth of 3 cm. Samples were placed into cryovials and immediately flash frozen in liquid nitrogen, then stored at −80°C until the analyses of citrate synthase (CS), cytochrome c oxidase (CCO), glutathione peroxidase (GPx), and superoxide dismutase (SOD) activities, and the concentration of malondialdehyde (MDA) via colorimetry on a microplate reader (Synergy H1; BioTek Instruments, Winooski, VT, USA).
2.3 DNA methylation
The DNA methylation data presented in Table 1 were derived from the analysis of leukocytes isolated from bull calves in a prior study (Littlejohn et al., 2018), which were a separate set of bull calves from the calves discussed herein. Briefly, isolated and purified DNA underwent reduced representation bisulfite sequencing to identify differential methylation of DNA in PNS compared with CON bull calves (Methyl-MiniSeq™; Zymo Research, Irvine, CA, USA), which is described in greater detail by Littlejohn et al. (2018).
2.4 Serum creatine kinase activity
Undiluted serum samples were analyzed for CK activity using a commercially available kit (CK Liqui-UV; EKF Diagnostics, Boerne, TX, USA), modified to a 96-well plate format. Per the kit instructions, plates were read using a microplate reader (Synergy H1), and CK activity was indirectly determined via the increase in NADPH absorbance per min at 340 nm. Samples were analyzed in duplicate with an intra-assay and inter-assay coefficient of variation (CV) of 4.5% and 8.7%, respectively.
2.5 Serum cortisol concentration
Serum cortisol concentrations were determined by a single-antibody radioimmunoassay (RIA), which utilized polypropylene tubes coated with cortisol antiserum (MP Biomedicals, Orangeburg, NY, USA), as previously described (Littlejohn et al., 2016). The cortisol antiserum cross-reactivity with steroids was 0.03% for aldosterone; 0.94% for corticosterone; 0.26% for deoxycorticosterone; 0.02% for progesterone; and 0.01% for estradiol. The radiolabeled tracer was 125I-cortisol. Radioactivity was detected by using an automatic gamma counter (COBRA II Auto-Gamma; PerkinElmer, Inc., Shelton, CT, USA) for 2 min, and counts per minute were converted to ng/mL using a microcomputer assay analysis program (Assay Zap; Biosoft, Cambridge, UK). All serum samples were analyzed in duplicate 25-μL aliquots within a single assay, with an intra-assay CV of 4.5%.
2.6 Skeletal muscle mitochondrial enzyme activities
Mitochondrial volume density and function of skeletal muscle samples were determined by analysis of CS and CCO activities, respectively (Larsen et al., 2012; Meinild Lundby et al., 2018). Frozen muscle samples were cryopulverized (Spectrum™ Bessman Tissue Pulverizer; Spectrum Laboratories, Inc., Rancho Dominguez, CA, USA), and, subsequently, sonicated (F60 Sonic Dismembrator; Fisher Scientific, Waltham, MA, USA) in a sucrose homogenization buffer (20 mM Tris, 40 mM KCl, 2 mM EGTA, and 250 mM sucrose, pH 7.4) with 0.05% detergent (n-dodecyl-β-D-maltoside; Sigma D4641), as previously described (Owen et al., 2020). Supernatant was collected and stored at −80°C until analysis. For both CS and CCO activities, a 40-fold dilution of the muscle homogenate was analyzed in duplicate. Activities were measured as previously described (Spinazzi et al., 2012; Owen et al., 2020). Citrate synthase activity was determined using the linear rate of reaction of free coenzyme A (CoASH) with DTNB [5,5′-dithiobis-(2-nitrobenzoic acid)] at 412 nm, and CCO activity was determined using the linear rate of oxidation of fully reduced cytochrome c at 550 nm, both at 37°C. Each assay was run on a single plate, with intra-assay CVs of 3.7% for CS and of 5.5% for CCO. Activities were normalized to total protein, determined using the Coomassie Bradford Protein Assay Kit (Thermo Scientific, Rockford, IL, USA). Cytochrome c oxidase activity was further normalized to mitochondrial content using CS activity, giving a measure of intrinsic mitochondrial function (Larsen et al., 2012; Meinild Lundby et al., 2018).
2.7 Skeletal muscle antioxidant enzyme activities
Superoxide dismutase and GPx activities were determined using commercially available kits (SOD: NWK-SOD02, Northwest Life Science Specialties, LLC, Vancouver, WA, USA; GPx: Cayman 703,102, Cayman Chemical, Ann Arbor, MI, USA) at 560 nm and 350 nm, respectively. Cryopulverized muscle tissue was homogenized in an extraction buffer (0.1 M KH2PO4, 1 mM EDTA, pH 7.2), sonicated on ice, and centrifuged at 14,000 × g for 2 min at 0°C, as previously described (Owen et al., 2020). Supernatant was collected and stored at −80°C until analysis. For SOD, a 40-fold dilution of the homogenate was analyzed in duplicate on a single plate with an intra-assay CV of 1.1%. SOD activity was measured by monitoring the rate of auto-oxidation of the hematoxylin reagent. One unit of SOD activity was defined as the amount of enzyme that inhibited the rate of cytochrome c reduction by half at 25°C. For GPx, a 20-fold dilution of the homogenate was analyzed in triplicate, with an inter-assay CV of 2.9% and an intra-assay CV of 9.8%. GPx activity was indirectly determined by the linear rate of oxidation of NADPH to NADP+ via a coupled reaction with glutathione reductase. Both activities were normalized to protein content, which was determined using the Coomassie Bradford Protein Assay Kit.
2.8 Skeletal muscle malondialdehyde concentration
Malondialdehyde concentration was quantified using a commercially available kit (NWK-MDA01; Northwest Life Science Specialties, LLC). Powdered muscle tissue was diluted, that is, 1 mg of tissue to 10 µL of the provided extraction buffer, sonicated on ice, and centrifuged at 11,000 × g for 10 min at 4°C. Supernatant was collected and stored at −80°C until analysis. A 10-fold dilution of the homogenate was analyzed in triplicate on a single plate, with an intra-assay CV of 3.6%. MDA activity was determined by the reaction of thiobarbituric acid (TBA) with MDA to form MDA-TBA2, which absorbs strongly at 532 nm. A spectral scan from 400 nm to 700 nm was performed. Samples were within the standard range of the assay (0.1 µM to 10 µM).The concentration of MDA was then normalized to total protein content, as determined using the Coomassie Bradford Protein Assay Kit.
2.9 Statistical analysis
Data were analyzed using mixed linear models in SAS (Version 9.4; SAS Institute Inc., Cary, NC, USA). Fixed effects included sex (bull or heifer), treatment (PNS or CON), and the interaction of the two variables. Prior to statistical analysis, outliers were removed if two or more standard deviations from the mean for each response variable. Outliers for intrinsic (relative to CS) CCO activity included one CON heifer, one PNS heifer, and one PNS bull. There was one PNS heifer outlier for CK activity and one CON bull outlier for GPx activity. There were no outliers for any of the other measurements. The results are presented as estimates of least squares means ± SEM. Significance was declared at a p-value ≤ 0.05, and trends were declared when 0.05 < p < 0.10.
3 Results
Serum CK and cortisol were similar among all cattle regardless of sex, treatment, or the interaction of sex and treatment (Table 2).
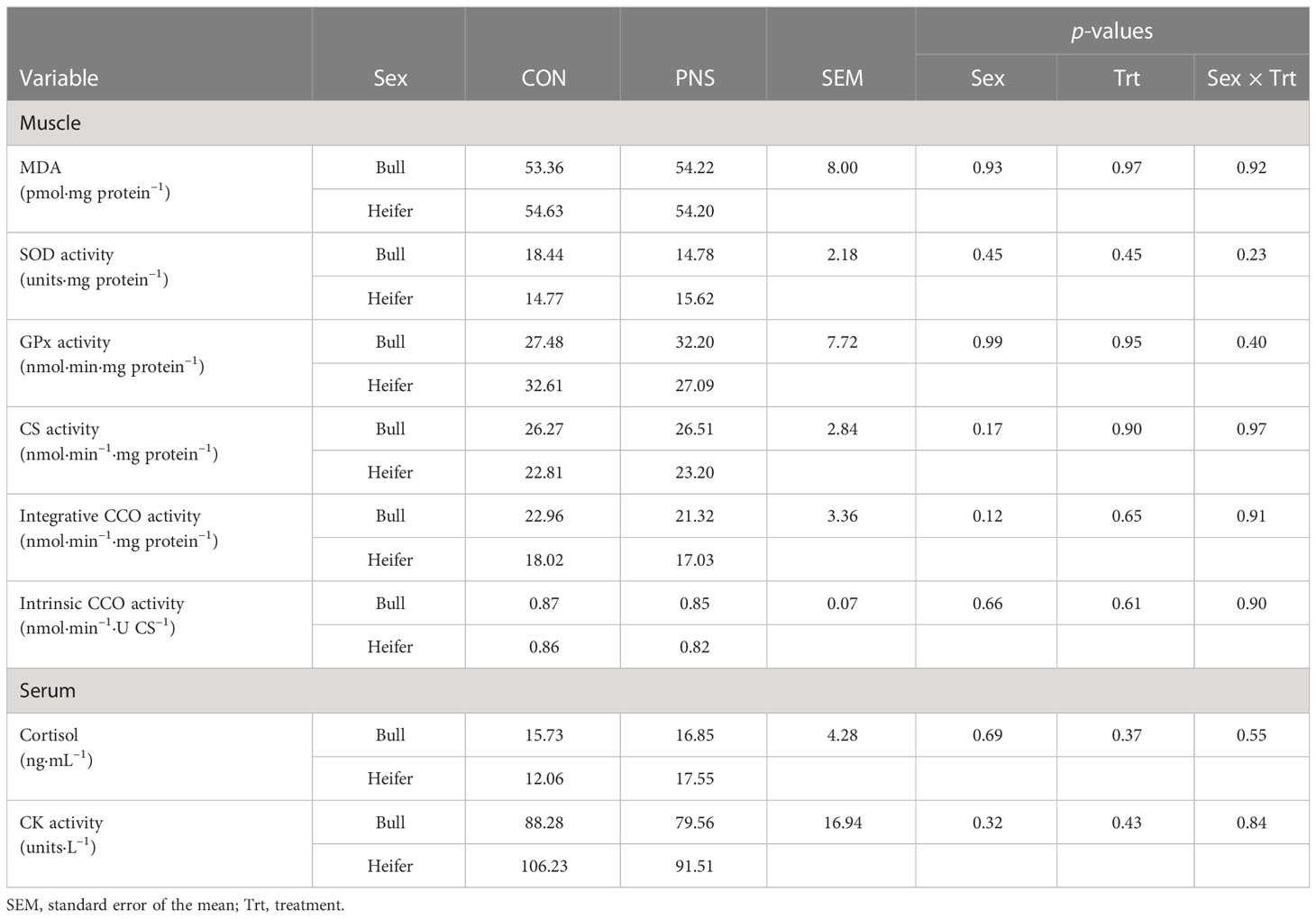
Table 2 Longissimus thoracis malondialdehyde (MDA) concentrations and superoxide dismutase (SOD), glutathione peroxidase (GPx), citrate synthase (CS), and cytochrome c oxidase (CCO) activities, and serum cortisol concentration and creatine kinase (CK) activity of prenatally stressed (PNS) Brahman bull (n = 8) and heifer (n = 6) yearling calves and control (CON) bull (n = 4) and heifer (n = 6) calves.
Skeletal muscle CS, CCO, SOD, and GPx activities and MDA concentrations did not differ by sex, treatment, or the sex × treatment interaction (Table 2).
4 Discussion
Two converging lines of evidence from our group catalyzed the present inquiry regarding the potential effects of prenatal transportation stress on bovine skeletal muscle mitochondria. We previously reported that temperament affected muscle mitochondrial energetics (Owen et al., 2020), and we identified methylomic and phenotypic differences related to temperament and metabolic hormones in PNS calves (Littlejohn et al., 2018; Littlejohn et al., 2020). Studies in mice and rats have identified that multiple forms of psychosocial stress, such as noise exposure, cage overcrowding, and acute or chronic restraint, induce mitochondrial adaptation within different tissues (Picard and McEwen, 2018b). Notably, the transportation of livestock involves all of the aforementioned forms of stress. Mitochondria are key regulators of stress; systemic stress mediators trigger mitochondrial structural and functional changes that may eventually damage mitochondrial DNA and impair energy production. This leads to the production of mitochondrial signals (mitokines), which can influence the cumulative cellular and systemic stress load, or allostatic load, in multiple ways (for a full review, see Picard and McEwen, 2018a). Thus, the mitochondria of animals exposed to transportation stress in utero may undergo adaptations to modulate the stress response and, consequently, have altered energetic requirements. However, the effects of prenatal transportation stress on skeletal muscle mitochondria and oxidative stress had not been evaluated. Contrary to our hypothesis, in the current study, PNS calves showed no differences in mitochondrial volume density or function compared with CON calves as yearlings. Additionally, calf muscle antioxidant activities (i.e., SOD and GPx) and lipid peroxidation (i.e., MDA concentration), myofiber perturbation (i.e., serum CK activity), and serum cortisol concentration were unaffected by transportation stress in utero. The results suggest that prenatal transportation stress did not negatively impact skeletal muscle in yearling Brahman calves that were maintained in unstressed physiological and environmental conditions.
As previously mentioned, differential methylation of genes associated with metabolism, immunity, and the stress response was observed in white blood cells from PNS calves (Littlejohn et al., 2018; Baker et al., 2020; Littlejohn et al., 2020), suggesting that PNS animals may have altered energetic and immune responses. Specifically, COA5, COX5A, and NRF1 were hypermethylated in the leukocytes of PNS calves, indicating that these genes would have decreased levels of expression, whereas CKMT1B was hypomethylated, predicting increased levels of expression in skeletal muscle (Table 1; Littlejohn et al., unpublished observation). Decreased levels of expression of COA5 and COX5A, and assumed translation to their respective proteins, may impact the assembly of the CCO enzyme, which one would expect to affect its function as complex IV, the final complex of electron transfer within the mitochondria. Additionally, NRF1 regulates mitochondrial biogenesis through the activation of mitochondrial transcription factor A, which drives the transcription of mitochondrial enzymes (Jornayvaz and Shulman, 2010). Together, the proposed decrease in expression of COA5, COX5A, and NRF1 could impair mitochondrial function in leukocytes, which may or may not be an accurate surrogate for muscle cells. This contrasts with the current results, which indicate no differences in mitochondrial volume density or organelle function in the skeletal muscle of PNS calves. Expression of CKMT1B was also predicted to increase in leukocytes, which suggests elevated creatine kinase activity. Creatine kinase functions to generate adenosine triphosphate (ATP) through transferring a phosphate group from phosphocreatine to adenosine diphosphate (ADP). Creatine kinase relies on a small pool of phosphocreatine to generate ATP; therefore, CK is not an efficient form of energy production for prolonged periods of time. However, CK also functions in the alternative direction to produce phosphocreatine and ADP from ATP. The function of CK varies based on the tissue-specific isoforms. Specifically, mitochondrial creatine kinase activity has been shown to be upregulated in times of impaired energy states (Schlattner et al., 2006). In the current study, serum CK was determined as an indirect assessment of muscle damage. Intense skeletal muscle contraction may cause damage to the muscle fiber, which is believed to result in the leakage of CK into circulation (Noakes, 1987; Clarkson et al., 1992).
Importantly, PNS calves are reported to have altered immune function, which may indicate a need for compensatory changes in mitochondrial function and energy production to maintain homeostasis. Specifically, previous reports indicate that PNS calves have elevated resting (pre-lipopolysaccharide challenge) pro-inflammatory cytokine concentrations [tumor necrosis factor alpha (TNF-α) and interleukin 6 (IL-6)], as well as a greater increase in pro-inflammatory interferon gamma (IFN-γ) concentrations following a systemic lipopolysaccharide challenge compared with non-stressed calves (Littlejohn et al., 2019). Systemic cytokines, muscle myokines, and muscle methylome were not assessed in the current study, but should be considered in future investigations (Gifford et al., 2012; Deters and Hansen, 2022).
Immune cells and mitochondria produce ROS for various purposes, such as the destruction of pathogens and cellular signaling. However, elevated ROS production could lead to oxidative stress without efficient antioxidant regulation. Oxidative stress is the imbalance between free radicals and antioxidants in favor of the former, potentially leading to cellular damage (Finaud et al., 2006). Two antioxidant enzymes vital to the prevention of oxidative stress are SOD and GPx. Superoxide dismutase is responsible for converting superoxide (O2−) to hydrogen peroxide (H2O2). Hydrogen peroxide can then be detoxified to water by either catalase or GPx. In the current study, there were no differences in SOD or GPx activities in the skeletal muscle of PNS calves compared with CON calves. There was also no difference in muscle MDA, a marker of lipid peroxidation, or serum CK activity, a marker of myofiber perturbation.
Most likely, the lack of differences noted in this study may be attributed to the fact that the calves were not subjected to any provocative environmental or immunological stressors. During the pre-transport loading process and while in transit, tissue damage can occur, which incites an inflammatory response (Van Engen and Coetzee, 2018) quantified by increased acute-phase proteins (Arthington et al., 2003). Mitochondria sense and respond to a vast array of environmental, metabolic, and neuroendocrine stressors and biological stress mediators (for a full review see, Picard and McEwen, 2018a). Particularly, acute exposure of myotubes to IL-6 (a pro-inflammatory cytokine) increased oxygen consumption rates and the production of mitochondrial ROS (Abid et al., 2020). Therefore, exposure and response to stress may exacerbate the immune and metabolic differences in PNS animals (Deters and Hansen, 2020).
In conclusion, our data suggest that calves subjected to prenatal transportation stress do not experience any impairment or improvement of mitochondrial function or volume density when in an unstressed state. Additionally, our results did not suggest oxidative stress or muscle damage in PNS calves at rest, but these markers may be different in PNS calves following exposure to an environmental or managerial stressor. Thus, further research is required to develop a thorough understanding of the impacts of prenatal stress on progeny.
Data availability statement
The original contributions presented in the study are included in the article/supplementary material. Further inquiries can be directed to the corresponding author.
Ethics statement
The animal study was reviewed and approved by the Texas A&M AgriLife Research Agricultural Animal Care and Use Committee (AUP 2017-006A and 2018-017A).
Author contributions
SW-S, TW, and RR conceived the study. SW-S, TW, RR, CL, and DR designed the study. RR and CL managed the cattle. SW-S, TW, and CG collected and analyzed the samples. SW-S, DR, and CG analyzed the data. LW, CG, SW-S, RR, and TW wrote the manuscript. All authors contributed to the article and approved the submitted version.
Funding
This work was supported in part by USDA-NIFA grants (2018-67015-28131; 2019-67015-2957; 2021-67015-33,392), Multi-State Hatch Projects NC1184, W4112, and W4173, and the Texas A&M University One Health Initiative.
Acknowledgments
The authors thank Catherine Wellman, Audrey Earnhardt, Brittni Littlejohn, Deborah Price, Don Neuendorff, and Dustin Law for their technical assistance.
Conflict of interest
The authors declare that the research was conducted in the absence of any commercial or financial relationships that could be construed as a potential conflict of interest.
Publisher’s note
All claims expressed in this article are solely those of the authors and do not necessarily represent those of their affiliated organizations, or those of the publisher, the editors and the reviewers. Any product that may be evaluated in this article, or claim that may be made by its manufacturer, is not guaranteed or endorsed by the publisher.
References
Abid H., Ryan Z. C., Delmotte P., Sieck G. C., Lanza I. R. (2020). Extramyocellular interleukin-6 influences skeletal muscle mitochondrial physiology through canonical JAK/STAT signaling pathways. FASEB J. 34 (11), 14458–14472. doi: 10.1096/fj.202000965rr
Arthington J. D., Eicher S. D., Kunkle W. E., Martin F. G. (2003). Effect of transportation and commingling on the acute-phase protein response, growth, and feed intake of newly weaned beef calves. J. Anim. Sci. 81 (5), 1120–1125. doi: 10.2527/2003.8151120x
Baker E. C., Cilkiz K. Z., Riggs P. K., Littlejohn B. P., Long C. R., Welsh T. H., et al. (2020). Effect of prenatal transportation stress on DNA methylation in Brahman heifers. Livestock Sci. 240:104116. doi: 10.1016/j.livsci.2020.104116
Bonnet M., Cassar-Malek I., Chilliard Y., Picard B. (2010). Ontogenesis of muscle and adipose tissues and their interactions in ruminants and other species. Animal 4 (7), 1093–1109. doi: 10.1017/s1751731110000601
Chen Y., Arsenault R., Napper S., Griebel P. (2015). Models and methods to investigate acute stress responses in cattle. Animals 5 (4), 1268–1295. doi: 10.3390/ani5040411
Clarkson P. M., Nosaka K., Braun B. (1992). Muscle function after exercise-induced muscle damage and rapid adaptation. Med. Sci. Sports Exerc. 24 (5), 512–520. doi: 10.1249/00005768-199205000-00004
Collier C. T., Williams P. N., Carroll J. A., Welsh T. H. Jr., Laurenz J. C. (2011). Effect of maternal restraint stress during gestation on temporal lipopolysaccharide-induced neuroendocrine and immune responses of progeny. Domest. Anim. Endocrinol. 40 (1), 40–50. doi: 10.1016/j.domaniend.2010.08.005
Dahl G. E., Tao S., Monteiro A. P. A. (2016). Effects of late-gestation heat stress on immunity and performance of calves. J. Dairy Sci. 99 (4), 3193–3198. doi: 10.3168/jds.2015-9990
Danese A., McEwen B. S. (2012). Adverse childhood experiences, allostasis, allostatic load, and age-related disease. Physiol. Behav. 106 (1), 29–39. doi: 10.1016/j.physbeh.2011.08.019
De Kloet E. R., Joëls M., Holsboer F. (2005). Stress and the brain: from adaptation to disease. Nat. Rev. Neurosci. 6 (6), 463–475. doi: 10.1038/nrn1683
Deters E. L., Hansen S. L. (2020). Invited Review: Linking road transportation with oxidative stress in cattle and other species. Appl. Anim. Sci. 36 (2), 183–200. doi: 10.15232/aas.2019-01956
Deters E. L., Hansen S. L. (2022). Long-distance transit alters liver and skeletal muscle physiology of beef cattle. Animal 16 (6), 100555. doi: 10.1016/j.animal.2022.100555
Du M., Tong J., Zhao J., Underwood K. R., Zhu M., Ford S. P., et al. (2010). Fetal programming of skeletal muscle development in ruminant animals. J. Anim. Sci. 88 (13 Suppl), E51–E60. doi: 10.2527/jas.2009-2311
FASS (2010). Guide for Care and Use of Agricultural Animals in Research and Teaching. 3rd ed (Champaign, IL: Federation of Animal Science Societies).
Finaud J., Lac G., Filaire E. (2006). Oxidative stress. Sports Med. 36 (4), 327–358. doi: 10.2165/00007256-200636040-00004
Gifford C. A., Holland B. P., Mills R. L., Maxwell C. L., Farney J. K., Terrill S. J., et al. (2012). Growth and Development Symposium: Impacts of inflammation on cattle growth and carcass merit. J. Anim. Sci. 90 (5), 1438–1451. doi: 10.2527/jas.2011-4846
Hopper R. M. (2015). Inducing parturition or abortion in cattle (Ames, IA: Bovine Reproduction. John Wiley & Sons).
Jornayvaz F. R., Shulman G. I. (2010). Regulation of mitochondrial biogenesis. Essays Biochem. 47, 69–84. doi: 10.1042/bse0470069
Larsen S., Nielsen J., Hansen C. N., Nielsen L. B., Wibrand F., Stride N., et al. (2012). Biomarkers of mitochondrial content in skeletal muscle of healthy young human subjects. J. Physiol. 590 (14), 3349–3360. doi: 10.1113/jphysiol.2012.230185
Lay J. D.C., Friend T. H., Randel R. D., Jenkins O. C., Neuendorff D. A., Kapp G. M., et al. (1996). Adrenocorticotropic hormone dose response and some physiological effects of transportation on pregnant Brahman cattle. J. Anim. Sci. 74 (8), 1806–1811. doi: 10.2527/1996.7481806x
Lay J. D.C., Randel R. D., Friend T. H., Carroll J. A., Welsh T. H. Jr., Jenkins D. C., et al. (1997b). Effects of prenatal stress on the fetal calf. Domest. Anim. Endocrinol. 14 (2), 73–80. doi: 10.1016/s0739-7240(96)00115-4
Lay J. D.C., Randel R. D., Friend T. H., Jenkins O. C., Neuendorff D. A., Bushong D. M., et al. (1997a). Effects of prenatal stress on suckling calves. J. Anim. Sci. 75 (12), 3143. doi: 10.2527/1997.75123143x
Littlejohn B. P., Burdick Sanchez N. C., Carroll J. A., Price D. M., Vann R. C., Welsh T. H. Jr., et al. (2019). Influence of prenatal transportation stress on innate immune response to an endotoxin challenge in weaned Brahman bull calves. Stress 22 (2), 236–247. doi: 10.1080/10253890.2018.1523895
Littlejohn B. P., Price D. M., Banta J. P., Lewis A. W., Neuendorff D. A., Carroll J. A., et al. (2016). Prenatal transportation stress alters temperament and serum cortisol concentrations in suckling Brahman calves. J. Anim. Sci. 94 (2), 602–609. doi: 10.2527/jas.2015-9635
Littlejohn B. P., Price D. M., Neuendorff D. A., Carroll J. A., Vann R. C., Riggs P. K., et al. (2020). Influence of prenatal transportation stress-induced differential DNA methylation on the physiological control of behavior and stress response in suckling Brahman bull calves. J. Anim. Sci. 98 (1):skz368. doi: 10.1093/jas/skz368
Littlejohn B. P., Price D. M., Neuendorff D. A., Carroll J. A., Vann R. C., Riggs P. K., et al. (2018). Prenatal transportation stress alters genome-wide DNA methylation in suckling Brahman bull calves. J. Anim. Sci. 96 (12), 5075–5099. doi: 10.1093/jas/sky350
Meinild Lundby A. K., Jacobs R., Gehrig S., De Leur J., Hauser M., Bonne T. C., et al. (2018). Exercise training increases skeletal muscle mitochondrial volume density by enlargement of existing mitochondria and not de novo biogenesis. Acta Physiol. 222 (1), e12905. doi: 10.1111/apha.12905
Minka N., Ayo J. (2009). Physiological responses of food animals to road transportation stress. Afr. J. Biotechnol. 8 (25):6601–6613.
Noakes T. D. (1987). Effect of exercise on serum enzyme activities in humans. Sports Med. 4 (4), 245–267. doi: 10.2165/00007256-198704040-00003
Otten W., Kanitz E., Tuchscherer M. (2015). The impact of pre-natal stress on offspring development in pigs. J. Agric. Sci. 153 (5), 907–919. doi: 10.1017/s0021859614001361
Owen R. N., Latham C. M., Long C. R., Randel R. D., Welsh T. H. Jr., White-Springer S. H. (2020). Temperament influences mitochondrial capacity in skeletal muscle from 8 through 18 mo of age in Brahman heifers. J. Anim. Sci. 98 (10), skaa291. doi: 10.1093/jas/skaa291
Picard M., McEwen B. S. (2018a). Psychological stress and mitochondria: a conceptual framework. Psychosomat. Med. 80 (2), 126–140. doi: 10.1097/psy.0000000000000544
Picard M., McEwen B. S. (2018b). Psychological stress and mitochondria: a systematic review. Psychosomat. Med. 80 (2), 141–153. doi: 10.1097/psy.0000000000000545
Pillai S. M., Sereda N. H., Hoffman M. L., Valley E. V., Crenshaw T. D., Park Y.-K., et al. (2016). Effects of poor maternal nutrition during gestation on bone development and mesenchymal stem cell activity in offspring. PloS One 11 (12), e0168382. doi: 10.1371/journal.pone.0168382
Posont R. J., Yates D. T. (2019). Postnatal nutrient repartitioning due to adaptive developmental programming. Vet. Clin. North Am.: Food Anim. Pract. 35 (2), 277–288. doi: 10.1016/j.cvfa.2019.02.001
Price D. M., Lewis A. W., Neuendorff D. A., Carroll J. A., Burdick Sanchez N. C., Vann R. C., et al. (2015). Physiological and metabolic responses of gestating Brahman cows to repeated transportation. J. Anim. Sci. 93 (2), 737–745. doi: 10.2527/jas.2013-7508
Schlafer D. H., Fisher P. J., Davies C. J. (2000). The bovine placenta before and after birth: placental development and function in health and disease. Anim. Reprod. Sci. 60-61, 145–160. doi: 10.1016/S0378-4320(00)00132-9
Schlattner U., Tokarska-Schlattner M., Wallimann T. (2006). Mitochondrial creatine kinase in human health and disease. Biochim. Biophysi. Acta 1762 (2), 164–180. doi: 10.1016/j.bbadis.2005.09.004
Senger P. L. (2003). Pathways to Pregnancy and Parturition. 2nd ed (Pullman, WA: Current Conceptions, Inc.).
Spinazzi M., Casarin A., Pertegato V., Salviati L., Angelini C. (2012). Assessment of mitochondrial respiratory chain enzymatic activities on tissues and cultured cells. Nat. Protoc. 7 (6), 1235. doi: 10.1038/nprot.2012.058
Timón-Gómez A., Nývltová E., Abriata L. A., Vila A. J., Hosler J., Barrientos A. (2018). Mitochondrial cytochrome c oxidase biogenesis: Recent developments. Semin. Cell. Dev. Biol. 76, 163–178. doi: 10.1016/j.semcdb.2017.08.055
Townsend D. M., Tew K. D. (2003). The role of glutathione-S-transferase in anti-cancer drug resistance. Oncogene 22 (47), 7369–7375. doi: 10.1038/sj.onc.1206940
Keywords: Brahman, cattle, energetics, mitochondria, muscle, prenatal stress
Citation: Wesolowski LT, Guy CP, Long CR, Randel RD, Riley DG, Welsh TH Jr. and White-Springer SH (2023) Prenatal transportation stress does not impact resting skeletal muscle mitochondrial function or antioxidant activity in Brahman calves. Front. Anim. Sci. 4:1163531. doi: 10.3389/fanim.2023.1163531
Received: 10 February 2023; Accepted: 05 July 2023;
Published: 09 August 2023.
Edited by:
Mohan Mondal, ICAR-National Dairy Research Institute, IndiaReviewed by:
Allison M. Meyer, University of Missouri, United StatesJon Schoonmaker, Purdue University, United States
Copyright © 2023 Wesolowski, Guy, Long, Randel, Riley, Welsh and White-Springer. This is an open-access article distributed under the terms of the Creative Commons Attribution License (CC BY). The use, distribution or reproduction in other forums is permitted, provided the original author(s) and the copyright owner(s) are credited and that the original publication in this journal is cited, in accordance with accepted academic practice. No use, distribution or reproduction is permitted which does not comply with these terms.
*Correspondence: Sarah H. White-Springer, cy53aGl0ZUBhZy50YW11LmVkdQ==