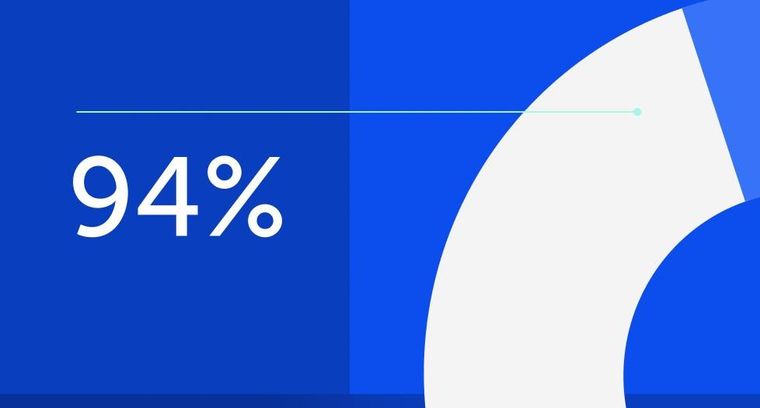
94% of researchers rate our articles as excellent or good
Learn more about the work of our research integrity team to safeguard the quality of each article we publish.
Find out more
ORIGINAL RESEARCH article
Front. Anim. Sci., 06 February 2023
Sec. Animal Nutrition
Volume 4 - 2023 | https://doi.org/10.3389/fanim.2023.1123532
This article is part of the Research TopicMitigating the Impact of Animal Production on the Environment: The Ecosystem IntegrationView all 8 articles
Despite considerable effort to develop and optimise additives to reduce methane emissions from cattle, little information on additive effectiveness exists for cattle under grazing scenarios. As the majority of Australian cattle production occurs on grazing land it is pertinent to report on the use of additives under simulated conditions. The current study evaluated the addition of nine additives to Rhodes grass hay under in vitro conditions, to estimate their impact on methane (CH4), gas production, and rumen fermentation parameters (volatile fatty acids, rumen pH and in vitro dry matter digestibility [IVDMD]). Citral extract at 0.1% of rumen media decreased all CH4 production parameters, but reduced gas production and digestibility, compared to a 100% hay control. Similarly, Sandalwood essential oil decreased CH4 production at 48 h, IVDMD and gas production, compared to the control. Biochar + nitrates at 5 and 8% DM, and Biochar + Asparagopsis at 5% DM decreased cumulative CH4 production (15.6%, 25.9%, 23.8%, respectively; P < 0.01), compared to the control. No changes in IVDMD and gas production were observed. As such, the biochar additives were considered the most promising additives from those evaluated with a substrate designed to replicate Australian grazing systems.
To understand the magnitude of reducing enteric methane (CH4) emissions from Australian grazing systems, it is necessary to understand the composition of the beef cattle industry in Australia. Beef cattle systems account for ~55% of the agricultural sector (Greenwood et al., 2018). Grazing systems are responsible for 1,152.61 gigagrams of CH4, corresponding to 79.5% of total emissions from cattle population, 59.8% of total domestic ruminants, and 53.2% of total Australian agricultural emissions in 2019 (AGEIS, 2019).
Consumers are becoming increasingly aware of the carbon cycle and the contribution of enteric CH4 emissions from cattle. When coupled with the primary goal of producing high-quality protein, systems which can mitigate emissions and sequester carbon are becoming increasingly desirable. For this reason, the Australian Red Meat Industry set the ambitious target to be carbon neutral by 2030. To achieve such a status within the given timeframe, adoption of commercially ready strategies must be a target. As such, this study aimed to screen commercially available, and novel feed compounds with the potential for immediate commercialisation, for their ability to reduce CH4 without affecting rumen fermentation parameters. Rapid in vitro screening allows the evaluation of multiple treatments and variables at the same time, by simulating ruminal digestion kinetics and restricting ambient influence, but considering variability in rumen fluid conditions (Cabezas-Garcia et al., 2021).
Anti-methanogenic additives selected for the current study, had previously shown direct modes of action against CH4 production, by promoting synthesis of bacteriocin-like compounds (Deng et al., 2018), limiting the synthesis of isoprenoids units, transmembrane proteins and adhesion-like proteins which are important on methanogens cell wall (Busquet et al., 2005), inhibiting subunits genes (Mtr and Eha) involved in methanogenesis (Zhou et al., 2018) and suppression of electron transfer in the reduction of methyl coenzyme M into CH4 (Kung et al., 2003). Similarly, these compounds have also shown indirect modes of action including damage of bacterial integrity as well as enzymatic activity (Wanapat et al., 2008; Oskoueian et al., 2013), creation of an alternative hydrogen sink (e.g. propionate) (Patra and Yu, 2012; Joch et al., 2016), or development of microbial biofilms (Thies and Rillig, 2012).
Unfortunately, despite promising reductions in CH4, some evidence suggests anti-methanogenic additives may have adverse effects on rumen fermentation parameters and nutrient digestion, as well as ruminal microbial adaptation. Therefore, the objective of this study was to evaluate the capacity of four commercial additives and three non-commercial compounds to reduce CH4 concentration and production at 24 and 48 hours under in vitro conditions, as well as their influence on rumen fermentation parameters at the end of the incubation.
Rumen fluid collections were conducted in accordance with the Australian Code of Practice for the Care and Use of Animals for Scientific Purposes with approval from The University of Queensland Animal Ethics Committee (SA2019/08/707).
The location of this study was at UQ Gatton research Dairy, the University of Queensland, Gatton campus. Three cannulated Holstein steers, fed exclusively with Rhode grass hay, were used for rumen fluid collection. These collections occurred two to three hours after morning feeding. Rumen fluid samples were collected from the dorsal, anterior ventral, medium ventral, posterior dorsal and posterior ventral regions of the rumen and transferred into a pre-warmed thermos. In the laboratory, rumen fluid was purged under constant CO2 and stored at 39°C until in vitro incubations.
For all in vitro incubations Rhode grass hay was used as the substrate to mimic Northern Queensland grazing conditions. The hay was dried at 60°C for 48 h and ground through a 1-mm sieve (Retsch ZM 200; Haan, NW, Germany) for chemical composition analysis and in vitro incubations.
A total of 9 additives were evaluated, compared to a Rhodes grass only control. Additives names have been changed per an agreement with manufacturers. The additives evaluated in the current study comprised 1) Garlic powder (garlic powder, lauric and stearic acids and citrus extract); 2) Biochar + nitrates; 3) Biochar + Asparagopsis (The pyrolysis of both biochar additives was 600°C for 30 min); 4) Commercial essential oil blend (EO blend); 5) Citral extract (95% of purity; SIGMA-Aldrich, St Louis, United States of America); 6) Pure Sandalwood essential oil (EO) extract (The Paperbark Company, West Leederville, Australia); 7) Bacillus probiotic additive (B. licheniformis and B. Subtilis), and Sugar cane extract (phenolic compound and flavonoids) evaluated as either 8) powder, or 9) liquid, considering each presentation as an independent additive. Inclusion levels were selected following manufacturers recommendations and doses used in previous studies demonstrating effective CH4 reduction. Additives in powder form were included on a dry matter basis, replacing the amount of substrate incubated. Whereas, liquid additives with included based on a percentage of total media incubated (rumen fluid and buffer; Table 1). Powder additives with a particle size >1 mm were ground to 1 mm, to ensure homogenisation with the Rhodes grass substrate.
Additives and control (substrate only, 100% hay) were incubated in triplicates on 3 separate runs, using in vitro batch culture fermentation method described in Meale et al. (2012). Additionally, 3 blanks bottles with no substrate or additive were included in each run, totalling 261 bottles across the entire experiment. Garlic powder and Citral extract were only incubated in two runs due to COVID-19 restrictions.
A total of 0.5 g of Rhode grass (substrate) was placed into ANKOM bags with solid additives. Liquid additives were transferred into bottles prior to the inclusion of rumen fluid, and immediately capped with rubber stoppers. On incubation day, amber serum bottles were warmed at 39°C in the incubator for 60 min and gassed with CO2 before the inclusion of 25 mL of 2:1 mixture of rumen fluid: buffer saturated with CO2. Modified McDougall’s buffer was used in the current study (Czerkawski and Breckenridge, 2007). Bottles were capped with rubber stoppers, and transferred into the incubator at 39°C with rotatory shaker at 120 oscillations/min.
Culture bottles were removed at 24 and 48 h to estimate gas production using water displacement apparatus (Fedorah and Hrudey, 1983). To estimate CH4, 17 mL of fermentation gas were collected per bottle at both incubation times, using 20 mL syringe and transferred into a 12 mL evacuated exetainer, guaranteeing positive pressure. At 48 h of incubation, culture bottles were opened and immediately placed on ice to cease microbial fermentation. pH was measured immediately (Activon Model 209, Gladesville, NSW, Australia). A sub-sample (1.5 mL) of each bottle culture was transferred into 2 mL Eppendorf tube with 300 μL of metaphosphoric acid (20% w/w) and stored at -20°C for further volatile fatty acids (VFA) analysis. ANKOM bags were extracted from bottles, rinsed and washed to remove culture media and dried at 60°C for 24 h.
Samples of Rhodes grass hay used feed steers were collected, mixed and dry for the follow chemical composition analysis. Dry matter (DM) content was estimated at 105°C [AOAC (2005), method 934.01]. Ash was determined at 550°C for 8 h [AOAC (2005), method 942.05]. Ash free neutral detergent fibre (aNDFom) content was calculated using the procedure mentioned in (Mertens, 2002). Acid detergent fibre (ADF) was estimated following AOAC (2005, method 973.18) Nitrogen content was determined using distillation and titration method (Sweeney, 1989). For N estimation was used LECO CN928 carbon/nitrogen combustion analyser AOAC (2005, method 990.03) (LECO Corporation; St Joseph, MI, United States of America). Crude protein (CP) was calculated by multiplying N content by 6.25. Substrate consisted of 90.37% DM, 9.31% CP, 8.9% ash, 66.19% NDF and 36.9% ADF.
A total of 3 mL sub-sample of fermentation gas was removed from the exetainers and analysed for CH4, using gas chromatography following the procedure from Chaves et al. (2006). For the current study, CH4 was expressed as mg CH4/g digested DM and total net gas production (mL)/g DM incubated. Total (TVFA) and individual VFA percentage of TVFA were calculated using gas chromatography, following specifications and procedure mentioned in (Forwood et al., 2019). Subsamples obtained from blank culture bottles were analysed to estimate net total VFA production.
ANKOM bags were weighed after washing and drying at 60°C for 48 h to calculate substrate digested over the incubation period (IVDMD). For additives in powder form, IVDMD was adjusted following the equation presented in Teoh et al. (2019), where digestible DM (g) was corrected by extracting the soluble DM fraction (Table 2) from the residues after 48 h of incubation.
Data obtained from in vitro fermentations were analysed by additive type (liquid and powder) as completely randomized design using PROC MIXED of SAS software (9.4 version, SAS institute Inc., Cary, NC), using treatment (additives) as fixed effects, run and the interaction of run and treatment were considered random effects. Incubation run was used as an error term to estimate treatment effects on CH4, rumen fermentation parameters and IVDMD. Treatment effects were determined comparing treatment dose replicates means with control (100% Rhodes grass hay), using least squares mean linear hypothesis test (LSMEANS/DIFF) with Dunnett adjustment, declaring significance at P ≤ 0.05 and tendency at 0.05 < P ≤ 0.10.
Most of the additives evaluated in the current study did not elicit changes in in vitro rumen fermentation parameters, CH4 or IVDMD at either timepoint, as well as CH4 cumulative concentration and production (Tables 3, 4).
Table 3 Effects of liquid additives (% media volume) on rumen fermentation parameters, IVDMD and methane over 24 and 48 h of incubation, through three in vitro batch culture fermentation runs.
Table 4 Effects of powder additives (% DM) on rumen fermentation parameters, IVDMD and methane over 24 and 48 h of incubation, through three in vitro batch culture fermentation runs.
When comparing the liquid additives, cumulative CH4 production (mL; P < 0.01) and CH4 per gram of DM incubated (P < 0.01) were lower than the control with Citral extract at 0.1% of culture media (40.45% and 41.0%). Inclusion of Sandalwood EO at 5% DM resulted in a 25.6% reduction (P<0.01) in CH4 at 48 h, but did not affect cumulative CH4 (Table 3; P > 0.05). Despite this, inclusion of Citral extract at the highest dose (0.1% culture media) decreased total gas fermentation parameters compared to the control, where cumulative gas production decreased (mL; P < 0.01) by 24.3% (Table 3). The highest dose Sandalwood EO (5% culture media) also lowered cumulative gas production (mL) by 16.0%, compared to the control. Media pH increased (P<0.01) with the inclusion of 0.05 and 0.1% Citral, and 3 and 5% Sandalwood EO, without an observable change in TVFA concentration or production or individual VFA concentrations (Table 3). Citral extract at 0.1% of culture media and Sandalwood EO at 3% and 5% of culture media, decreased IVDMD by 19.6%, 12.4% and 13.3%, respectively, compared to the control.
Of the powder additives, Biochar + nitrates and Biochar + Asparagopsis at their highest doses (8 and 5% DM, respectively) were able to elicit a reduction in cumulative CH4 at 48 h (25.9 and 23.8% expressed as mL CH4; and 22.8 and 19.8% when expressed as mL CH4/g DM, respectively), compared to the control (Table 4). Biochar + nitrates at 5% DM also showed a reduction in cumulative CH4 at 48h (mL), but this was not observed on a mL/g DM basis. No effect was observed on gas production, TVFAs or individual percentages of TVFAs. With the exception of acetate, which tended (P=0.07) to be higher than the control, with the inclusion of 8% DM Biochar + nitrates (Table 4). Media pH was higher than the control with the addition of the highest doses of the two biochar additives; however, the change was not considered biologically relevant as pH levels were maintained above 6.0, favouring microbial growth (Calsamiglia et al., 2002). Despite no other observed effects, garlic powder inclusion at 5% DM decreased IVDMD by 11.2%, compared to the control.
Multiple strategies have been examined with the intention to decrease CH4, yet the majority have shown only low and/or short-term anti-methanogenic effectivity, with some also exhibiting negative impacts on rumen fermentation, nutrient digestibility and feed efficiency (Buddle et al., 2011; Beauchemin et al., 2020; Black et al., 2021). There is also limited evidence of additive effectiveness when included in forage-based diets, or for use in grazing systems. Thus, the purpose of the current study was to provide foundational evidence on the most promising additives to limit CH4 emissions in the Australian context. The central hypothesis was that selected commercial additives and non-commercial anti-methanogenic compounds, with different modes of action, would reduce CH4 without impacting rumen fermentation parameters, under in vitro conditions with a forage-based substrate. The results obtained from this study did not support the hypothesis, as just two additives were able to decrease CH4 with minimal impact on rumen fermentation parameters, including gas production, total and individual VFA percentage, and IVDMD.
In the current study, recommended manufacturers doses or doses based on previous published literature were used. Most of the additives and compounds evaluated did not exhibit the ability to inhibit CH4, suggesting either an insufficient dose or perhaps these additives may not be as effective when included in a forage-based diet. For instance, Durmic et al. (2014) reported that the commercial essential oil blend reduced CH4 concentration with a dose of 0.01 mg, using a grain-based substrate. However, in the current trial, this additive with higher doses, did not alter CH4 parameters. Although these discrepancies in additive effectiveness might be considered negatively, these anti-methanogenic strategies may still have an important role to reduce CH4 in intensive cattle production systems. It is important to consider that some additives have shown promise to promote animal productivity, equating to a potential reduction in methane intensity. Both benefits can facilitate their utilisation by Australian producers. In this sense, we support the idea presented in Beauchemin et al. (2020) which proposed the evaluation of combining additives with alternatives mode of actions, on CH4 production and animal productivity.
Biochar additives (Biochar + nitrates and Biochar + Asparagopsis) presented the most promising outcomes, where cumulative CH4 production was decreased expressed as both millilitres of methane and per unit of DM. Interestingly, Biochar + Asparagopsis only reduced methane at 24h of incubation while the other Biochar additive sustained methane reduction at both sampling times and thus, reduced cumulative production. Importantly, theses additives did not affect gas production parameters, or IVDMD. The lack of effect on these parameters suggests that CH4 inhibition was not simply a result of reduced microbial fermentation or substrate digestion. This indicates that cattle supplementation with these Biochar additives, may have the potential to improve cattle growth and feed efficiency as more metabolic energy and hydrogen can be utilised for metabolite synthesis, instead of being utilised in methanogenesis (Patra and Yu, 2012).
Total VFA concentration or production was not affected by biochar additives. However, a tendency for Biochar + nitrate at 8% to increase the percentage of acetate (P = 0.07) was observed. An increase in acetate by this additive was not expected as evidence demonstrates a positive correlation with CH4 production due to the release of CO2 and H2 during acetogenesis, which are the main sources for hydrogenotrophic methanogens to produce CH4 (Janssen, 2010). It is speculated that the increase in acetate percentage with Biochar + nitrates resulted from the presence of potassium nitrates in the biochar, which may increase fibrolytic bacterial communities, but the presence of nitrates has also been shown to reduce firmicutes abundance (Zhao et al., 2015).
These outcomes indicate that both Biochar additives directly inhibited methanogenesis. However, the inclusion of post-processing compounds on these additives (KNO3 and Asparagopsis) may have contributed to these results and altered the mode of action against methanogenesis. In theory, biochar has a porous structure with a large internal surface area (Thies and Rillig, 2012). These features favour microbial biofilm development increasing substrate fermentation and metabolic energy utilisation (Teoh et al., 2019). In addition, evidence suggests that Biochar can adsorb fermentation gases including carbon dioxide to methanogenesis (Saleem et al., 2018). Nevertheless, considering the doses used for these additives, it is not feasible to consider this mode of action as a potential reason for the observed CH4 reduction. Conversely, when KNO3 is available in the rumen, ruminal bacterial can degraded into nitrites and later into ammonia. During these catabolic reactions, hydrogen is required, meaning that there is a direct competition with methanogens for metabolic hydrogen (Newbold et al., 2014). Due to high nitrate reactivity in the rumen, redox potential increases which can limit the growth of most rumen bacteria communities (Marais et al., 1988). For instance, rumen nitrate concentration above 2 mg/L inhibits Ruminococcus spp and Fibrobacter succinogenes growth, which are high hydrogen producers (Lee and Beauchemin, 2014; Yang et al., 2016). Additional, Asparagopsis has the capacity to reduce CH4 production up to 90% under in vitro conditions. This red macro algae has alternative compounds such as Bromochloromethane, bromoform and chloroform which are methane analogs (Black et al., 2021). These compounds can bind the prosthetic group of methyl-coenzyme M which participates in the last step of methanogenesis. Furthermore, Bromocloromethane compounds can promote propionate production which is considered an alternative hydrogen sink (Roque et al., 2021). However, the latest Asparagopsis property is discarded in the current trial as Biochar + Asparagopsis did not influence TVFA or the percentage of propionate in TVFA. Indeed, both biochar additives studied here, have the potential to become commercial additives, but it is necessary to determine the exact KNO3 and Asparagopsis concentrations present, especially as the latter at inclusions up to 0.20% of organic matter basis, may generate residues in milk and meat, and reduce quality of these products (Kinley et al., 2020). Further studies should confirm the potential impact of including Asparagopsis compounds in biochar, especially Bromochloroacetic acid and Dibromoacetic acid which are considered as ozone-depleting substance (Chipperfield et al., 2020).
Sandalwood EO at 5% DM decreased CH4 production at 48 h (mL) of incubation by 25.6% (P < 0.01). However, the highest two doses reduced IVDMD, and the highest dose reduce gas production, compared with the control, suggesting that this additive at the highest dose may limit substrate availability for ruminal microbiota, and consequently CH4 synthesis. This mode of action to reduce CH4 is not desirable for an anti-methanogenic additive as it may compromise rumen bacterial growth, ruminal fermentation and potentially cattle productivity (Benchaar and Greathead, 2011). An interesting feature observed with this additive was a lag effect, where CH4 inhibition occurred at the last period of the incubation (48h). Sandalwood EO comprised components including Santalo, Bisabolol, Baergamatol and Farneso, but there is limited knowledge on its potential mode of action against methanogenesis. In previous studies, Sandalwood EO at different doses (25 to 500 ul/L) decreased CH4 concentration up to 48% under in vitro conditions (Durmic et al., 2014; Jahani-Azizabadi et al., 2019), but in the current study, the highest methane reduction was 25.6%. In relation with fermentation parameters, there is a consistent tendency of Sandalwood oil to reduce TVFA production and IVDMD, but inconsistent results are observed on gas production, where in the current study was reduced 13.3% with highest dose, Durmic et al. (2014) by 28.15%, but Jahani-Azizabadi et al. (2019) did not observe changes in this parameter. Consideration of the cost of this additive and supply factors would need to be accounted for, as this additive is not currently marketed to the cattle industry and the scale of production necessary could be a limitation to its adoption.
Citral at 0.10% of culture media showed a reduction in CH4 parameters. Unfortunately, the inhibition of CH4 was also accompanied by reductions in IVDMD and gas production parameters. Previous studies have observed low CH4 concentration with the presence of Citral, but similar to the current study, this EO compound, also affected other fermentation parameters such as TVFA and propionic acid percentage (Joch et al., 2016). Citral is known to have strong anti-bacterial properties due the capacity to increase bacterial membrane permeability, intracellular pH and at the same time directly competes with bacteria to access proteins and nucleic acids. These changes leads to membrane damage, inhibition of DNA transcription and enzymatic activities, as well as ATP leakage (Dorman and Deans, 2000; Wanapat et al., 2008; Shi et al., 2016). In this sense, it is hypothesized that the doses used in the current incubations supressed bacterial growth and thus microbial fermentation. Opposing outcomes were observed by Khan (2021) in which citral inclusion at 25 uL/gDMi reduced CH4 production (47%) in a RUSITEC incubation for 16 days. However, in the same study, Citral increased total gas production, with a reduction of TVFA, acetic and propionic percentage on TVFA, and IVDMD. Importantly to mention that a low substrate digestion and total or individual VFA percentage, indicates that ruminal bacterial fermentation was affected, meaning that gases produced during fermentation should decrease as well. Indeed, Citral has anti-methanogenic properties, but unfortunately its inclusion with Rhodes grass was accompanied by a strong impact on microbial fermentation. In this sense, further studies should determine appropriate doses which may inhibit CH4 with minimum impact on microbial fermentation.
Overall, high doses of both Citral and Sandalwood EO cannot be considered as anti-methanogenic alternatives for immediate industry adoption, due to the impact on rumen fermentation parameters observed using substrates representative of the Australian grazing system under in vitro conditions. However, the potential utilisation of both compounds should focus on low concentrations. In addition, future studies should identify and isolate Sandalwood EO compounds which are responsible for the reduction in CH4 without affecting feed digestibility, TVFA and individual VFAs percentages. Moreover, the isolation of Sandalwood EO compounds, might contribute as well with the identification of natural or synthetic compounds with similar metabolic structure and function, which potentially decrease dietary inclusion and costs.
In conclusion, four additives showed promising outcomes to decrease CH4 concentration when included with a Rhodes grass hay substrate. However, in some cases this was accompanied by a reduction in bacterial fermentation and substrate digestibility. Biochar + Asparagopsis decreased CH4 after 48h of in vitro incubations, Biochar + nitrates reduced cumulative methane production. In both cases, substrate digestion and rumen bacterial fermentation were not affected, but individual VFA percentages were altered. However, until more knowledge about concentration of KNO3 and Asparagopsis in both Biochar additives is available, these cannot be proposed as potential anti-methanogenic alternatives. Citral and Sandalwood EO decreased CH4, and inhibited rumen microbial fermentation at high doses. Thus, we recommend that only low doses should be considered in further studies, or in the case of Sandalwood EO, the identification and extraction of its compounds which may inhibit CH4, without altering microbial fermentation or nutrient digestibility, should be evaluated. Ultimately, any additive which shows promise under in vitro conditions should then be evaluated in vivo to validate the effectiveness of the additive prior to any recommendation for industry adoption.
The raw data supporting the conclusions of this article will be made available by the authors, without undue reservation.
The animal study was reviewed and approved by University of Queensland Production animal Committee.
MP, SM and AC contributed to conception and design of the study. All authors contributed to the methodology and data collection. AC performed the statistical analysis. MP wrote the first draft of the manuscript. SM and AC wrote sections of the manuscript. All authors contributed to manuscript revision, read, and approved the submitted version.
The authors declare that this study is fully funded by Meat & Livestock Australia (MLA) through the Sustainability program.
The authors acknowledge the technical support of the analytical services team at UQ Gatton.
The authors declare that the research was conducted in the absence of any commercial or financial relationships that could be construed as a potential conflict of interest.
All claims expressed in this article are solely those of the authors and do not necessarily represent those of their affiliated organizations, or those of the publisher, the editors and the reviewers. Any product that may be evaluated in this article, or claim that may be made by its manufacturer, is not guaranteed or endorsed by the publisher.
AGEIS (2019). National greenhouse gas inventory - Paris agreement inventory (Canberra ACT, Australia: Australian goverment, Department of Industry, Science, Energy and Resources).
Beauchemin K. A., Ungerfeld E. M., Eckard R. J., Wang M. (2020). Review: Fifty years of research on rumen methanogenesis: lessons learned and future challenges for mitigation. Animal 14, s2–s16. doi: 10.1017/S1751731119003100
Benchaar C., Greathead H. (2011). Essential oils and opportunities to mitigate enteric methane emissions from ruminants. Anim. Feed Sci. Technol. 166-167, 338–355. doi: 10.1016/j.anifeedsci.2011.04.024
Black J. L., Davison T. M., Box I. (2021). Methane emissions from ruminants in Australia: Mitigation potential and applicability of mitigation strategies. Animals 11 (4):951. doi: 10.3390/ani11040951
Buddle B. M., Denis M., Attwood G. T., Altermann E., Janssen P. H., Ronimus R. S., et al. (2011). Strategies to reduce methane emissions from farmed ruminants grazing on pasture. Vet. J. 188 (1), 11–17. doi: 10.1016/j.tvjl.2010.02.019
Busquet M., Calsamiglia S., Ferret A., Carro M. D., Kamel C. (2005). Effect of garlic oil and four of its compounds on rumen microbial fermentation. J. Dairy Sci. 88 (12), 4393–4404. doi: 10.3168/jds.S0022-0302(05)73126-X
Cabezas-Garcia E. H., Danielsson R., Ramin M., Huhtanen P. (2021). In vitro incubations do not reflect In vivo differences based on ranking of low and high methane emitters in dairy cows. Animals 11 (11), 3112. doi: 10.3390/ani11113112
Calsamiglia S., Ferret A., Devant M. (2002). Effects of pH and pH fluctuations on microbial fermentation and nutrient flow from a dual-flow continuous culture system. J. Dairy Sci. 85 (3), 574–579. doi: 10.3168/jds.S0022-0302(02)74111-8
Chaves A. V., Thompson L. C., Iwaasa A. D., Scott S. L., Olson M. E., Benchaar C., et al. (2006). Effect of pasture type (alfalfa vs. grass) on methane and carbon dioxide production by yearling beef heifers. Can. J. Anim. Sci. 86 (3), 409–418. doi: 10.4141/A05-081
Chipperfield M. P., Hossaini R., Montzka S. A., Reimann S., Sherry D., Tegtmeier S. (2020). Renewed and emerging concerns over the production and emission of ozone-depleting substances. nature reviews. Earth Environ. 1 (5), 251–263. doi: 10.1038/s43017-020-0048-8
Czerkawski J. W., Breckenridge G. (2007). Design and development of a long-term rumen simulation technique (Rusitec). Br. J. Nutr. 38 (3), 371–384. doi: 10.1079/BJN19770102
Deng K. D., Xiao Y., Ma T., Tu Y., Diao Q., Chen Y. H., et al. (2018). Ruminal fermentation, nutrient metabolism, and methane emissions of sheep in response to dietary supplementation with bacillus licheniformis. Anim. Feed Sci. Technol. 241:38–44. doi: 10.1016/j.anifeedsci.2018.04.014
Dorman H. J. D., Deans S. G. (2000). Antimicrobial agents from plants: Antibacterial activity of plant volatile oils. J. Appl. Microbiol. 88 (2), 308–316. doi: 10.1046/j.1365-2672.2000.00969.x
Durmic Z., Moate P. J., Eckard R., Revell D. K., Williams R., Vercoe P. E. (2014). In vitro screening of selected feed additives, plant essential oils and plant extracts for rumen methane mitigation: Novel anti-methanogenic feed additives. J. Sci. Food Agric. 94 (6), 1191–1196. doi: 10.1002/jsfa.6396
Fedorah P. M., Hrudey S. E. (1983). A simple apparatus for measuring gas production by methanogenic cultures in serum bottles. Environ. Technol. Lett. 4 (10), 425–432. doi: 10.1080/09593338309384228
Forwood D. L., Hooker K., Caro E., Huo Y., Holman D. B., Meale S. J., et al. (2019). “Crop sorghum ensiled with unsalable vegetables increases silage microbial diversity,” (Frontiers in Microbiology) 10:2599. doi: 10.3389/fmicb.2019.02599
Greenwood P. L., Gardner G. E., Ferguson D. M. (2018). Current situation and future prospects for the Australian beef industry - a review. Asian-Australasian J. Anim. Sci. 31 (7), 992–1006. doi: 10.5713/ajas.18.0090
Jahani-Azizabadi H., Durmic Z., Vadhanabhuti J., Vercoe P. E. (2019). Effect of some Australian native shrubs essential oils on in vitro rumen microbial fermentation of a high-concentrate diet. J. Anim. Plant Sci. 29 (1), 8–15.
Janssen P. H. (2010). Influence of hydrogen on rumen methane formation and fermentation balances through microbial growth kinetics and fermentation thermodynamics. Anim. Feed Sci. Technol. 160 (1), 1–22. doi: 10.1016/j.anifeedsci.2010.07.002
Joch M., Cermak L., Hakl J., Hucko B., Duskova D., Marounek M. (2016). In vitro screening of essential oil active compounds for manipulation of rumen fermentation and methane mitigation. Asian-Australasian J. Anim. Sci. 29 (7), 952–959. doi: 10.5713/ajas.15.0474
Khan M. (2021). Citral and linalool reduce methane production through actions on the rumen microbiota, specifically inhibiting methanogenic archaea (Doctor of philosophy, The University of Western Australia) doi: 10.26182/z10f-6j19.
Kinley R. D., Martinez-Fernandez G., Matthews M. K., de Nys R., Magnusson M., Tomkins N. W. (2020). Mitigating the carbon footprint and improving productivity of ruminant livestock agriculture using a red seaweed. J. Cleaner Production 259, 120836. doi: 10.1016/j.jclepro.2020.120836
Kung L. Jr., Smith K. A., Smagala A. M., Endres K. M., Bessett C. A., Ranjit N. K., et al. (2003). Effects of 9,10 anthraquinone on ruminal fermentation, total-tract digestion, and blood metabolite concentrations in sheep1. J. Anim. Sci. 81 (1), 323–328. doi: 10.2527/2003.811323x
Lee C., Beauchemin K. A. (2014). A review of feeding supplementary nitrate to ruminant animals: nitrate toxicity, methane emissions, and production performance. Can. J. Anim. Sci. 94 (4), 557–570. doi: 10.4141/cjas-2014-069
Marais J. P., Therion J. J., Mackie R. I., Kistner A., Dennison C. (1988). Effect of nitrate and its reduction products on the growth and activity of the rumen microbial population. Br. J. Nutr. 59 (2), 301–313. doi: 10.1079/BJN19880037
Meale S. J., Chaves A. V., Baah J., McAllister T. A. (2012). Methane production of different forages in In vitro ruminal fermentation. Asian-Australasian J. Anim. Sci. 25 (1), 86–91. doi: 10.5713/ajas.2011.11249
Mertens D. (2002). Gravimetric determination of amylase-treated neutral detergent fiber in feeds with refluxing in beakers or crucibles: Collaborative study. J. AOAC Int. 85, 1217–1240.
Newbold J. R., van Zijderveld S. M., Hulshof R. B. A., Fokkink W. B., Leng R. A., Terencio P., et al. (2014). The effect of incremental levels of dietary nitrate on methane emissions in Holstein steers and performance in nelore bulls1. J. Anim. Sci. 92 (11), 5032–5040. doi: 10.2527/jas.2014-7677
Oskoueian E., Abdullah N., Oskoueian A. (2013). Effects of flavonoids on rumen fermentation activity, methane production, and microbial population. BioMed. Res. Int. 2013, 349129. doi: 10.1155/2013/349129
Patra A. K., Yu Z. (2012). Effects of essential oils on methane production and fermentation by, and abundance and diversity of, rumen microbial populations. Appl. Environ. Microbiol. 78 (12), 4271–4280. doi: 10.1128/AEM.00309-12
Roque B. M., Venegas M., Kinley R. D., de Nys R., Duarte T. L., Yang X., et al. (2021). Red seaweed (Asparagopsis taxiformis) supplementation reduces enteric methane by over 80 percent in beef steers. PloS One 16 (3), e0247820. doi: 10.1371/journal.pone.0247820
Saleem A. M., Ribeiro G. O. Jr., Yang W. Z., Ran T., Beauchemin K. A., McGeough E. J., et al. (2018). Effect of engineered biocarbon on rumen fermentation, microbial protein synthesis, and methane production in an artificial rumen (RUSITEC) fed a high forage diet. J. Anim. Sci. 96 (8), 3121–3130. doi: 10.1093/jas/sky204
Shi C., Song K., Zhang X., Sun Y., Sui Y., Chen Y., et al. (2016). Antimicrobial activity and possible mechanism of action of citral against cronobacter sakazakii. PloS One 11 (7), e0159006. doi: 10.1371/journal.pone.0159006
Sweeney R. A. (1989). Generic combustion method for determination of crude protein in feeds: collaborative study. J. Assoc. Off Anal. Chem. 72 (5), 770–774. doi: 10.1093/jaoac/72.5.770
Teoh R., Caro E., Holman D. B., Joseph S., Meale S. J., Chaves A. V. (2019). Effects of hardwood biochar on methane production, fermentation characteristics, and the rumen microbiota using rumen simulation. Front. Microbiol. 10. doi: 10.3389/fmicb.2019.01534
Thies J. E., Rillig M. C. (2009). Characteristics of Biochar: Biological properties. Biochar for environmental management: Science and technology, ed Lehmann J., Joseph S. First ed (London, United Kingdom).
Wanapat M., Cherdthong A., Pakdee P., Wanapat S. (2008). Manipulation of rumen ecology by dietary lemongrass (Cymbopogon citratus stapf.) powder supplementation. J. Anim. Sci. 86 (12), 3497–3503. doi: 10.2527/jas.2008-0885
Yang C., Rooke J. A., Cabeza I., Wallace R. J. (2016). Nitrate and inhibition of ruminal methanogenesis: Microbial ecology, obstacles, and opportunities for lowering methane emissions from ruminant livestock. Front. Microbiol. 7. doi: 10.3389/fmicb.2016.00132
Zhao L., Meng Q., Ren L., Liu W., Zhang X., Huo Y., et al. (2015). Effects of nitrate addition on rumen fermentation, bacterial biodiversity and abundance. Asian-Australasian J. Anim. Sci. 28 (10), 1433–1441. doi: 10.5713/ajas.15.0091
Keywords: in vitro batch culture fermentation, methane, anti-methanogenic additives, rumen fermentation, forage-based diet
Citation: Parra MC, Forwood DL, Chaves AV and Meale SJ (2023) In vitro screening of anti-methanogenic additives for use in Australian grazing systems. Front. Anim. Sci. 4:1123532. doi: 10.3389/fanim.2023.1123532
Received: 14 December 2022; Accepted: 26 January 2023;
Published: 06 February 2023.
Edited by:
Melissa Duplessis, Agriculture and Agri-Food Canada (AAFC), CanadaReviewed by:
Mariana Ornaghi, State University of Maringá, BrazilCopyright © 2023 Parra, Forwood, Chaves and Meale. This is an open-access article distributed under the terms of the Creative Commons Attribution License (CC BY). The use, distribution or reproduction in other forums is permitted, provided the original author(s) and the copyright owner(s) are credited and that the original publication in this journal is cited, in accordance with accepted academic practice. No use, distribution or reproduction is permitted which does not comply with these terms.
*Correspondence: Sarah J. Meale, cy5tZWFsZUB1cS5lZHUuYXU=
†These authors have contributed equally to this work
Disclaimer: All claims expressed in this article are solely those of the authors and do not necessarily represent those of their affiliated organizations, or those of the publisher, the editors and the reviewers. Any product that may be evaluated in this article or claim that may be made by its manufacturer is not guaranteed or endorsed by the publisher.
Research integrity at Frontiers
Learn more about the work of our research integrity team to safeguard the quality of each article we publish.