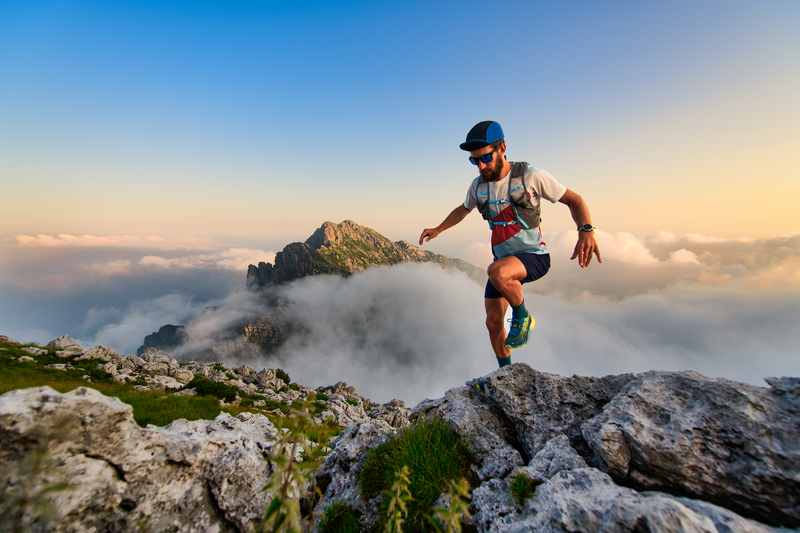
94% of researchers rate our articles as excellent or good
Learn more about the work of our research integrity team to safeguard the quality of each article we publish.
Find out more
REVIEW article
Front. Anim. Sci. , 03 October 2022
Sec. Animal Nutrition
Volume 3 - 2022 | https://doi.org/10.3389/fanim.2022.999338
This article is part of the Research Topic Seaweeds and Algae in Animal Nutrition View all 4 articles
Research is increasingly directed towards decreasing the greenhouse gases contribution, specifically methane, from the livestock agriculture sector. Macroalgae supplementation has emerged as a promising tool to mitigate enteric methane emission in ruminants. The mode of action responsible for the mitigation effect centers around the content of volatile halogenated compounds, primarily bromoform. The sub-tropical red seaweed, Asparagopsis taxiformis, is the most well researched bromoform containing species. While several studies, both in vitro and in vivo, have demonstrated the effectiveness of A. taxiformis at reducing enteric methane emission (> 80% reduction), questions surrounding sustainability, animal productivity, animal product quality, and commercial practicality remain. These questions by no means disqualify the practice of feeding macroalgae to cattle to reduce methane emission, but they must be answered before implementing macroalgae as a feed additive commercially. Also, limiting scientific inquiry to a few species reduces the potential of discovering other compounds and modes of action that could produce the desired mitigation effect without the inherit drawbacks of the current options. Work conducted in both ruminant nutrition and human health fields have identified numerous bioactive compounds within plants that exhibit anti-microbial functions that could modify the rumen microbiome for beneficial outcomes. These compounds are also found in macroalgae. Phlorotannins, saponins, sulfonated glycans, other halocarbons and bacteriocins found within macroalgae have demonstrated antimicrobial activity in vitro. However, it is unclear what effect these compounds may have when used in vivo. Once identified, extracting these compounds for supplementation in lieu of feeding the entire plant may be a more practical solution. Dietary inclusion levels of macroalgae in ruminant diets can be limited by variation in active ingredient concentration, palatability to cattle, and excessive dietary mineral content. There are multiple in vitro studies that have demonstrated a methane reduction potential of non-bromoform containing species, but inclusion levels are often well above the effective levels of A. taxiformis (< 0.5% of dietary dry matter). In some animal studies, A. taxiformis supplementation has led to decreased dry matter intake and productivity and elevated mineral accumulation, such as iodine, in animal products. Therefore, methane mitigation by macroalgae will likely have to occur at low dietary concentrations to be practical. This review aims to highlight potential benefits and challenges that feeding macroalgae as a tool for methane reduction may have on animal production, the environment, animal and consumer health.
Efforts to decrease the carbon footprint of human activities to mitigate climate change have increased globally across multiple economic sectors. In the animal agriculture sector, enteric methane emission from ruminant livestock has been identified as a key contributor to greenhouse gas (GHG) emissions. Methane (CH4) emissions from animal production as a whole need to be reduced by 24 to 47% by 2050 compared to 2010, in order to meet projected goals from the Paris Agreement (Masson-Delmotte et al., 2019; Arndt et al., 2022). It is estimated that one third of anthropogenic CH4 emissions is produced by ruminant livestock (Jackson et al., 2020). Methane emission from anerobic manure storage and enteric fermentation in ruminants is a byproduct of the metabolism of archaea, which reduces carbon dioxide (CO2) with hydrogen (H2) as a source of energy (Thauer, 2012). To further complicate matters, production and consumption of animal protein is projected to increase 68% by 2050 (Searchinger et al., 2018). Therefore, research has been even more directed towards finding methods which reduce enteric CH4 emission from dairy, beef and small ruminant operations. Animal and feed management, diet formulation, and rumen manipulation have been identified as feasible strategies to reduce absolute and/or product-based enteric CH4 emissions in a recent review by Arndt et al. (2022); this review highlighted rumen manipulation through feed additives, specifically macroalgae, as a potentially viable mitigation practice.
Macroalgae, commonly known as seaweed, represent a large domain of aquatic plants separated into three main taxa: Chlorophyta (green), Phaeophyceae (brown) and Rhodophyta (red). There are also three smaller taxa of seaweed which are not pertinent to this review and therefore will not be discussed. Green, brown, and red seaweeds are key marine habitats, and are harvested commercially for food and the abundance of bioactive compounds contained within them. One such compound, bromoform (CHBr3), has been proven to be highly effective at inhibiting methanogenesis (Machado et al., 2016a). It is thought that CHBr3, along with other halogenated volatile organic compounds (VOC), competitively bind to the enzymes and reductases that facilitate the final steps of reducing CO2 and H2 by archaea into CH4 (Wood et al., 1968). Bromoform is found within many seaweed species in low concentrations but has been discovered to accumulate in higher levels in the red seaweed Asparagopsis taxiformis (Carpenter and Liss, 2000; Machado et al., 2016a). Within the last ten years, researchers have investigated the effects of A. taxiformis on CH4 production in both in vitro and in vivo ruminant models and have concluded that it is highly effective as a CH4 mitigant for livestock. The aim of this paper is two-fold: (1) to describe the practicality and utilization of A. taxiformis as a mitigating feed additive for ruminants and (2) to explore the potential of other bioactive algal compounds to mitigate CH4 or improve animal performance.
As a member of the Bonnemaisoniaceae family, Asparagopsis taxiformis is a sub-tropical seaweed distributed in warm temperate waters that grows as a creeping rhizomatous structure on rocks and algal beds. It is considered ‘native’ in Indo-Pacific waters and parts of the Atlantic (Canary and Azores Islands), but has also been ‘introduced’ in waters off the coast of California and the eastern Mediterranean (Andreakis et al., 2004). This family of seaweeds, compared to other seaweeds, is known for producing and accumulating high concentrations of halogenated compounds (Paul et al., 2006). Bromoform and other brominated compounds are stored in gland cells residing within pericentral (parent) cells of Bonnemaisoniaceae, and are considered to be released into the environment upon mechanical exposure to combat predation and antagonistic bacteria (Paul et al., 2006). The unique structure of these red seaweeds allows accumulation of VOC to reach 2 to 6% of dry weight biomass (Mata et al., 2012). This high VOC content is what makes A. taxiformis, and to a lesser extent A. armata, appealing candidates for enteric CH4 emission reduction through rumen manipulation.
A growing body of work has established that low level inclusion (≤ 1%) of dietary dry matter (DM) of A. taxiformis can reduce, if not eliminate, enteric CH4 production (Table 1). Machado et al. (2014) demonstrated that inclusion of A. taxiformis at 20% organic matter (OM) with Flinders grass hay reduced CH4 production by 99% in vitro. Subsequent in vitro studies confirmed this antimethanogenic effect at doses below 2% substrate DM and identified CHBr3 as the primary compound responsible for this effect (Kinley et al., 2016; Machado et al., 2016b; Machado et al., 2016a; Machado et al., 2018). Chagas et al. (2019) further demonstrated that A. taxiformis was effective at reducing in vitro CH4 production at even lower inclusion rates (0.5 mg/g of DM).
Following on the in vitro work, in vivo supplementation of A. taxiformis (0.5 to 3% OM basis) in sheep reduced CH4 emissions 50 to 80% over 72 d (Li et al., 2018). Work conducted in steers decreased enteric CH4 yield (g/kg of DM) between 80 and 98% when diets were supplemented with A. taxiformis (0.2 to 0.5% OM basis), dependent on the dietary fiber content (Kinley et al., 2020; Roque et al., 2021). Interestingly, Roque et al. (2021) reported a decrease of dry matter intake (DMI) by 14% with an equivalent increase in feed conversion efficiency in steers fed A. taxiformis (0.5% OM). A similar VOC containing species, A. armata, reduced CH4 intensity (g/kg milk yield) by 60% and CH4 yield by 43% when fed at 1% OM to lactating dairy cows (Roque et al., 2019). However, DMI was also decreased by 38% in that study. Stefenoni et al. (2021) fed A. taxiformis to lactating Holsteins at 0.5% DM and observed a 55% reduction in CH4 yield. This study also reported decreased DMI and milk yield in cows fed A. taxiformis. Additionally, Stefenoni et al. (2021) reported a loss of antimethanogenic effect of the seaweed during the second half of the experiment. As this experiment was a 4 × 4 Latin square design, rumen adaptation was eliminated as a possible explanation for this inefficiency. The authors concluded the decreased effectiveness was a result of decreased CHBr3 concentration within the seaweed over time, from ~10 mg/kg to 3 mg/kg (day 0 and 120 of storage, respectively). Other previously mentioned studies did not report any decrease in CH4 mitigation effectiveness over time. Furthermore, a study conducted to establish a methodology for CHBr3 quantification found no decrease in CHBr3 concentration from A. taxiformis that had been freeze dried and stored at -20°C for 20 weeks (Romanazzi et al., 2021). Muizelaar et al. (2021) fed A. taxiformis at three levels (67, 133 and 333 g/d) and reported decreased DMI across all treatment levels. These authors also reported elevated CHBr3 excreted in milk during days 1 through 9, but CHBr3 was not consistently found in days 10 to 22 of feeding A. taxiformis. This was attributed to the inconsistency of seaweed intake. Upon completion of the study, two cows were sacrificed and their rumen walls were evaluated for abnormalities. The authors reported loss of rumen papillae and histological conformation of inflammation. These findings were comparable to rumen wall evaluation of sheep sacrificed in Li et al. (2018). However, both studies were unable to conclude that damage to the rumen epithelial were a direct result of A. taxiformis supplementation. Li et al. (2018) suggested that these changes could have been caused by mild ruminal acidosis associated with carbohydrate ingestion via the pelleted diet used to deliver the treatments. Muizelaar et al. (2021) did not report any clinical signs of acidosis. Additionally, both studies only examined rumen tissue from A. taxiformis animals, preventing comparison with control animals.
In addition to the contribution of enteric CH4 to climate change, it also accounts for gross feed energy intake losses between 2 and 12% (Johnson and Johnson, 1995). The extent of energy loss is tightly linked to carbohydrate fermentation and the resulting supply of available H2, which in turn influences the acetate:propionate ratio in ruminal contents (Wolin et al., 1997). In ruminal fermentation, acetate is regarded as a net H2 source, and propionate a H2 sink. Diets and conditions that produce a rumen environment with high H2 concentrations will drive fermentation away from pathways that produce H2 (acetate), resulting in higher propionate and lower CH4 production per unit of feed substrate (Janssen, 2010). The redirection and capture of H2 from CH4 to propionate is where opportunity exists to increase feed efficiency in livestock (Kinley et al., 2020).
When methanogenesis is inhibited using an additive like A. taxiformis, archaea are unable to consume the excess H2 resulting from fermentation, leading to an increase in propionate production and H2 accumulation within the rumen (Goel et al., 2009). In studies feeding A. taxiformis where eructated H2 was measured, H2 emission increased from 234 to 1700% compared to control treatments, depending on the inclusion level (Kinley et al., 2020; Roque et al., 2021; Stefenoni et al., 2021). In vivo studies which measured rumen VFA profile while feeding A. taxiformis observed decreases in acetate:propionate ratio between 12 and 45% (Li et al., 2018; Kinley et al., 2020; Stefenoni et al., 2021). With the exception of Li et al. (2018), where A. taxiformis was fed to sheep at 0.5 to 3.0% (OM basis), these studies did not report a decrease in total VFA concentration. Kinley et al. (2020) observed increased average daily weight gain of 26 and 22% for steers fed 0.1 and 0.2% (OM basis) A. taxiformis over 90 days, respectively. However, Stefenoni et al. (2021) reported a 6.5% decrease in milk yield, following a decrease in DMI, in lactating Holstein cows fed 0.5% (DM basis) A. taxiformis, with no difference in feed efficiency between treatments. Li et al. (2018) did not observe any change in liveweight gain in sheep fed 0.5 to 3.0% (OM basis) A. taxiformis.
It is difficult to reconcile the discrepancy in the above results, although species, diet composition and type of animal production (weight gain vs. milk yield) likely play some role, as animals will partition energy differently during lactation or growth. Moreover, 100% recovery and repartitioning of energy from available H2 into VFA production does not seem possible without the expansion of H2 sinks to the rumen. An in vitro study, in which methanogenesis was inhibited with 5 or 10 µM bromochloromethane reported 38 and 64% recovery of H2 in the form of short chain fatty acids, respectively (Goel et al., 2009). A study with lactating cows fed a different type of inhibitor, 3-nitroxypropanol (3-NOP), estimated 89 and 84% H2 recovery for control and 3-NOP treatments, respectively (Melgar et al., 2020). The authors were unable to determine the fate of the remaining H2 produced and highlighted the need for further research into rumen H2 dynamics. A recent study feeding dairy goats A. taxiformis combined with phloroglucinol, a H2 sink yielding acetate as a final product, observed a 74% decrease in eructated H2 compared with A. taxiformis alone (Romero et al., 2022a); total VFA and acetate concentrations in the rumen were also increased by A. taxiformis and phloroglucinol in that study. However, animal productivity metrics from this study have yet to be reported. Further research into combined inhibitor and H2 sink supplementation could potentially increase animal performance while simultaneously decreasing CH4 emissions and CH4 intensity (g/kg of milk or meat).
Results from the aforementioned studies indicate the large CH4 mitigation potential of feeding A. taxiformis but raise questions about animal safety and production effects of supplementation. Of primary concern for a livestock producer is the inconsistencies in DMI and performance. In studies where intake loss did not affect animal performance or was unobserved, a gradual increase in A. taxiformis inclusion was conducted over the animal’s adaptation period (Li et al., 2018; Kinley et al., 2020; Roque et al., 2021). This gradual adaptation was not applied in the studies that had substantial decreases in DMI and animal performance (Muizelaar et al., 2021; Stefenoni et al., 2021). If this product is to be utilized in commercial animal production, it is critical that a protocol of adaptation be established in order to mitigate performance loss in the form of meat or milk. The animal’s stage of production will also be of importance. For example, it has been established that DMI intake during the postpartum period in lactating dairy cattle is the key to maximize performance over the lactation and prevent inflammation and subsequent metabolic diseases (Kvidera et al., 2017). If a prepartum cow has not been acclimated to seaweed in their diet, a drop in intake during adaptation could trigger a decrease in animal health or performance across the rest of their lactation. The authors are unaware of any studies feeding A. taxiformis to prepartum animals or during the transition period.
Bromoform is categorized as a ‘potential human carcinogenic’ compound by the EPA, and although fed at low levels to livestock, it is currently unclear as to what impact feeding CHBr3 may have on animal health long term (U.S. Environmental Protection Agency National Center for Environmental Assessment (1987)). This concern is of higher priority in dairy cattle that persist within the herd for several years versus in a beef finishing system where animals are only supplement seaweed for ~90 d (Kinley et al., 2021). Personnel involved in A. taxiformis processing and feed manufacturing facilities could also potentially be at risk, if continuously exposed to A. taxiformis. This is a purely hypothetical concern, however, since potential exposure time and levels, manufacturing conditions, and A. taxiformis handling procedures have not been established or described. Future research should be directed to finding appropriate timing and implementation of seaweed supplementation to avoid these possible complications. Finally, controlled histological evaluations of rumen epithelial tissue should be conducted to assess the effects of VOC on these tissues over extended periods of exposure.
Seaweed cultivation, processing and storage conditions also need to be considered (Vijn et al., 2020). Previous work has utilized freeze dried product kept in some form of cold storage. The capability to store feed additives in a freezer or refrigerator is not common for dairy or livestock operations and will render the strategy impractical. Freeze dried A. taxiformis stored at 25°C had a 38% reduction in CHBr3 over the course of 12 weeks (Magnusson et al., 2020). In the same study, however, an oil immersion of A. taxiformis had no measurable decrease in CHBr3 concentration. This oil immersion technique could provide a solution to the issue of storage. Variables in seaweed production also need to be addressed. For example, phenotypic and temperature variation have an effect on CHBr3 content of Asparagopsis with content of bioactive compounds reaching tenfold differences (Mata et al., 2017). This suggests that wild harvested or commercial oceanic cultivation may produce vastly different products in terms of CHBr3 content. Land-based culture techniques could standardize plant variety as well as optimize growing conditions for consistent CHBr3 concentrations. For instance, providing a higher C/N ratio and increasing the availability of CO2 within the growing medium has been shown to increase CHBr3 content within the biomass of A. taxiformis (Mata et al., 2012).
Effects of CH4 inhibiting feed additives on methane production from anaerobic digesters is another relevant concern for livestock producers who utilize manure digesters on their operations. Digesters are being deployed by farmers in the United States as a way to offset carbon emissions and obtain carbon credits through government policy (Key and Sneeringer, 2011). Fortunately, CHBr3 appears to be quickly metabolized by the rumen microbes (Romero et al., 2022b) and is not excreted in animal manure (Kinley et al., 2020; Muizelaar et al., 2021). In situations where producers add feed refusals to their digester this may still present an issue. A recent in vitro study analyzed CH4 production from incubated manure from cows fed no inhibitor or 0.5% (OM basis) A. taxiformis, and the same manures with added A. taxiformis (Ramin et al., 2022). Enteric CH4 production was only numerically lower in A. taxiformis fed cows, and the addition of A. taxiformis to the manure decreased manure CH4 production by 50%. These results may indicate that the addition of A. taxiformis to manure digesters in the form of feed refusals may have a negative impact on their energy production. Nkemka et al. (2019) reported no effect on CH4 yield from anerobic leach bed digesters supplied manure from 3-NOP steers compared with control. Similarly, manure stored for 202 d from animals fed the CH4 inhibitor, 3-NOP, did not differ in CH4 emission when compared with animals fed no inhibitor (Owens et al., 2020). Both studies may indicate resilience to effects of dietary CH4 inhibitors in anerobic digester systems.
There are also potential concerns for the consumers of products derived from animals fed A. taxiformis. Whereas effects on consumer health are beyond the scope of this review, it is important to acknowledge the concerns surrounding the feeding of A. taxiformis to animals entering the food chain. As previously stated, Muizelaar et al. (2021) reported elevated levels of CHBr3 in milk. Levels ranged from not detectable to 9.1 µg/L in milk from cows on the Low treatment (~67g A. taxiformis/cow/d). This relatively small increase, that was transient throughout the study, may have been caused by inconsistent seaweed intake. Maximum reported CHBr3 levels in Med (133 g/h/d) and High (333 g/h/d) treatments reached 11 and 35 µg/L, respectively. However, these inclusion levels far exceed a minimum effective dose. Future work in this area should continue to quantify CHBr3 levels in milk over time. In studies involving steers no appreciable level of CHBr3 was found within the meat. A summary of toxicological risk to animals and humans concluded that at low inclusion levels A. taxiformis did not cause problems for ruminant animals or consumers through their products (Glasson et al., 2022). Stefenoni et al. (2021) conducted a milk sensory panel during which consumers were unable to discern between milk from cows fed A. taxiformis compared to control, but the ability to detect a difference approached a trend (P = 0.11; with 39% of participants correctly identified milk from A. taxiformis cows as different from milk from control cows). Additionally, milk from cows fed seaweed had 5- and 8-times higher concentrations of iodine and bromide, respectively. Bromide levels in this study from cows fed A. taxiformis at 0.5% (DM basis) averaged 40.4 mg/kg. For context, the World Health Organization recommends ≤ 6 mg/kg in water for adults and ≤ 2mg/kg for children (WHO, 2009). While acute toxicity to bromide is seldom seen in humans, chronic toxicity in animals has negative effects on endocrine and reproductive systems (VanBriesen, 2013). The increase of iodine in milk could have effects on consumer health; for example, in persons with abnormal thyroid function. Most seaweeds are known to have high mineral content due to the environment they are grown in and are used as a form of mineral supplementation in human and animal diets (Norton et al., 1981). Since minerals are sequestered from the growing environment, future land-based production facilities should be able to reduce mineral levels within seaweeds through water remediation. Monitoring of mineral levels in the diet and in animal products, manure and urine should be conducted to prevent any downstream effects on human health and the environment.
One final area of concern is the environmental impact of seaweed cultivation. It is unlikely that wild harvesting of seaweed will be adequate to meet the demand of the United States cattle market of ~3 million metric tons (MMT) dry product per annum (Vijn et al., 2020). Supplying the global herd of 1.4 billion cattle would not be feasible, not to mention impractical and cost prohibitive for pastoral smallholder farm systems (Vijn et al., 2020). Current supply of A. taxiformis is limited and expensive (Hristov et al., 2022). This is an untenable for any livestock production system, but the cost should decrease as more production and harvesting entities begin supplying the market. Therefore, large scale cultivation will need to be implemented along the coasts or in commercial facility. This will require energy in order to supply light, heat and salt for culture systems. In a land based production system it is estimated that A. taxiformis cultivation will emit 9.2 kg CO2e/kg of seaweed produced, with 48% of the CO2e being emitted from salt (Nilsson and Martin, 2022). It is important to note that this estimate was modeled using wind energy for drying down the seaweed using a refractance window drying technique. Recent studies using A. taxiformis have utilized lyophilization to dry the product which provides the highest concentration of CHBr3 in the final product (Vucko et al., 2017). The authors are unaware of any effects on CHBr3 content or activity when the seaweed is subjected to refractance window drying. Considering the decrease in mitigation effect observed in Stefenoni et al. (2021), processing of A. taxiformis should be optimized to retain CHBr3 concentration and activity level with the final product.
Bromoform itself poses potential hazards to the environment as an ozone depleting compound. All seaweeds combined are responsible for approximately 70% of the global bromoform flux. It stands to reason that increasing seaweed cultivation will increase the global CHBr3 emissions, especially when the species is known for higher CHBr3 content. However, current volume of all commercially harvested seaweeds (3.12 MMT) contribute only ~1% of the global CHBr3 flux, with the majority being emitted from wild seaweed (Glasson et al., 2022). Therefore, 100% implementation of A. taxiformis supplementation (at 1% of feed DM intake) in the U.S. herd of 93 million cattle would increase global CHBr3 emissions from cultivated seaweed to approximately 1.5%, as it will require half the biomass of current global seaweed biomass production (Vijn et al., 2020). Admittedly, estimates assuming full adoption across the livestock industry is unlikely but illustrates what the maximum contribution of CHBr3 to the atmosphere could be. As production of A. taxiformis ramps up it will be critical to continue monitoring atmospheric CHBr3 concentrations and quantify contributions from commercial seaweed systems.
It is important to note that seaweed cultivation can provide multiple benefits to the environment through nutrient extraction (Kim et al., 2019). In order to grow, seaweeds consume N, P and C, which can ameliorate harmful algal blooms, prevent eutrophication, and aid in buffering of seawater. If grown in open water, seaweed beds can also provide habitat for aquatic life. However, displacing native seaweed in lieu of growing a commercially viable seaweed may be antagonistic to local biodiversity. Land-based systems could employ this bioremediation to waste waters, reducing nutrient inputs to the system as well as ‘downstream’. Future efforts to integrate production systems to efficiently utilize nutrients may provide environmental benefits in addition to CH4 mitigation.
The associated benefits and risks of A. taxiformis need to be investigated and weighed against each other if this mitigation practice is to be adopted across the industry. This review seeks to highlight some of the initial questions that will accompany widescale implementation of the practice, but the decision to implement this technology may ultimately be made by the industry. A recent study by Passetti and Hegarty (2022) sought to assess industry interest and preparedness to use A. taxiformis as a methane inhibitor in livestock. A survey was sent to feed and feed supplement manufacturers and livestock managers in developed (87.5%) and developing (37.5%) countries to gauge their willingness to use additives and the priority to reduce GHG emissions. Methane inhibiting additives were a priority for 14% of feed manufacturers, with 14% of feed manufacturers being aware of using A. taxiformis as a CH4 inhibitor. Greenhouse gas management was not a priority for 81% of livestock managers. Conversely, feed efficiency and animal performance were the main motivation for 92% of livestock managers. This study was, by the admission of the authors, limited. However, the data underline the importance of continued research and development of CH4 inhibitors and H2 sinks in order to capitalize on the interest in animal performance and efficiency for the adoption of A. taxiformis and related technologies.
Exploration of seaweeds as CH4 mitigating feed additives has not been limited to Asparagopsis species. A review by Abbott et al. (2020) highlighted multiple in vitro studies with seaweeds using rumen inoculum that measured CH4 production and quantified CHBr3 content. While many studies reported decreases in CH4 production versus a control (no seaweed), supplementation levels often exceeded 2% of substrate DM or OM. Considering observed decreases in DMI with seaweed supplementation, biomass demand on seaweed production systems and the relatively high mineral content of seaweeds; inclusion levels within livestock diets should aim for the lowest effective dose (Norton et al., 1981; Erickson et al., 2012; Vijn et al., 2020; Muizelaar et al., 2021; Stefenoni et al., 2021). To date, Asparagopsis species appear to be the best candidate as a rumen manipulation additive due to effectiveness at low inclusion rates.
Seaweeds that inhibit methanogenesis may not be strictly limited to species with high CHBr3 content. Preliminary in vitro work on a non-CHBr3 seaweed species has been reported to reduce CH4 production at similar inclusion rates to in vitro work with A. taxiformis (Hristov et al., 2022). However, this undisclosed species and its mode of action requires further investigation and validation through in vivo studies. There are more than 200,000 secondary plant metabolites, many of which can be found in seaweeds (Patra and Saxena, 2010). Polyphenols, peptides, phlorotannins, alkaloids and sulfonated glycans are among the compounds present in seaweed species with known anti-microbial properties (Pomin, 2009; Abbott et al., 2020). However, seaweed compound specificity against methanogens and interactions with the rumen microbiome remain largely unexplored. Both in vitro and in vivo studies investigating the effects of secondary plant metabolites on methanogenesis are largely derived from terrestrial sources and have provided mixed results (Patra and Saxena, 2010; Patra et al., 2017; Abbott et al., 2020). In a review by Patra and Saxena (2010), saponins, tannins, flavonoids and organosulfur compounds all demonstrated potential to reduce CH4 production through direct inhibition or indirectly through suppression of protozoa growth.
Generally speaking, tannins have shown efficacy in decreasing CH4 emission in vivo but tend to impact animal performance by decreasing intake, protein digestibility and fiber digestibility (Arndt et al., 2022). Phlorotannins are structurally one of the simpler tannins and are specific to brown algae. The relatively smaller structural size may limit their effect on CH4 production, compared to larger compounds which are more effective at reducing CH4 (Hatew et al., 2016). Tannins from terrestrial plants are considered one of the few rumen manipulation strategies suitable for grazing systems since tanniferous plants can be grown within the pasture (Arndt et al., 2022). This advantage would be lost when using a marine source of tannin.
Results from studies supplementing terrestrial plant flavonoids, saponins and essential oils and their effects on methanogens and methanogenesis are variable. Some of this variation is due to the large diversity of compounds residing in each category. Seaweeds also contain variations of these secondary plant metabolites, although research from compounds specific to seaweeds are sparce. Still, terrestrial plant compound studies may help identify CH4 mitigating compounds in seaweeds with similar structure. An in vitro study reported six flavonoids that reduced CH4 in a dose dependent manner (Oskoueian et al., 2013). Reduction in CH4 yield ranged from 16 to 39% using myricetin, kaempferol, flavone, quercetin, naringin, rutin and catechin (ranked lowest to highest by CH4 reduction). Flavonoids (purity ≥ 98%) were dissolved in ethanol and included at 4.5% (w/w) of substrate DM, exceeding supplementation rates (%DM basis) of A. taxiformis required to produce a CH4 effect. Based on these concentrations, seaweed flavonoids will likely need to be extracted in order to have an effect on CH4 production. It is suggested that flavonoids directly inhibit methanogens and, in the case of catechin, act as a H2 sink (Oskoueian et al., 2013; Becker et al., 2014). Saponins, such as those found in green tea, seem to inhibit methanogenesis by directly inhibiting protozoa, decreasing protozoa-associated methanogen abundance through lower H2 production (Patra and Saxena, 2009). These alkaloids have been quantified within several red and green algae, but so far research has been limited to saponins from terrestrial plants (Abbott et al., 2020). Future research and classification of seaweed compounds for CH4 inhibition should focus on direct, qualitative identification of compounds that are structurally similar to terrestrial plant compounds that have proven CH4 mitigating effect.
Delivery method of anti-methanogenic feed additives should also be considered. In the case of A. taxiformis the entire plant is included in the diet. For a compound like CHBr3 this may well be the best option as the plant is able to retain the volatile compound within the tissue. Extracted CHBr3 could be more difficult and dangerous to handle for feed manufacturers and producers. However, other secondary plant metabolites could be extracted. This could produce a more concentrated product; decreasing the mass required in the diet, eliminating seasonal variation in plant concentration of the compound, and avoid dietary enrichment of trace minerals or heavy metals present in ocean water (Mata et al., 2017).
Feeding A. taxiformis to ruminant livestock may be the most effective rumen manipulation tool in terms of reducing CH4 emission. When paired with the possible decrease in gross feed energy loss and improved animal performance, A. taxiformis could provide a positive outcome for producers and the environment. However, improved animal performance will be contingent on avoiding the decreases in DMI observed in several studies. Potential negative effects on animal performance and health as well as human health should continue to be investigated. Many of these hurdles may have simple solutions. For example, having a defined protocol for diet adaptation to prevent DMI reductions, or reducing trace mineral content through controlled, land-based growing operations with guaranteed analysis on the final product. Supplementation of A. taxiformis with the goal of reducing CH4 only makes sense if there is a net reduction in carbon emissions and no damage to the ozone layer through increased CHBr3 emissions. Preliminary estimates seem to indicate that the benefits outweigh the risks. If improvements in production efficiency and bio-extraction of N, P from waterways are realized, A. taxiformis cultivation may be a beneficial enterprise for livestock producers and the environment. Environmental benefits will also be weighed alongside producers’ acceptance as the current cost of A. taxiformis, and potential negative effects on performance (i.e., DMI and milk yield) may limit market adoption. Animal productivity and feed efficiency seem to be the main drivers in the adoption of feed additives by livestock producers. Therefore, any concerns of decreased productivity need to be addressed. Possible negative effects from A. taxiformis supplementation on the health of animals, manufacturers, producers, and consumers should continue to be investigated. Due to low effective dose required to achieve CH4 mitigation and the reported lack of CHBr3 residue in the meat this may not be a cause for concern. However, under conditions of excess supplementation of A. taxiformis, CHBr3 is detected within milk, making it critical to monitor supplementation levels to lactating animals. Transfer of iodine and bromide into milk will still need to be brought to the attention of consumers in order for them to make informed decisions about their consumption of dairy products. The practicality of handling and storing seaweed products on farm will also need to be addressed. Reluctance to adopt this mitigation tool could be alleviated by increasing production of A. taxiformis, establishing dietary adaptation methods, and stabilizing the final product (e.g., oil immersion).
It is possible that other compounds found in macroalgae could have anti-methanogenic effects in vivo and should be explored. Secondary metabolites that are not classified as cancerous, stable at ambient temperatures and do not accumulate in milk or meat products would be preferential to both producers and consumers. It is also important that these metabolites do not sacrifice animal performance. Pairing inhibitors with H2 sinks may provide positive effects on animal performance and efficiency. Until one of these hypothetical compounds are proven to work in vivo, Asparagopsis taxiformis is the most effective naturally-derived rumen manipulation feed additive livestock producers have as a tool to mitigate enteric CH4 emissions.
All authors listed have made a substantial, direct, and intellectual contribution to the work and approved it for publication.
This work was supported by the USDA National Institute of Food and Agriculture Federal Appropriations under Project PEN 04539 and Accession number 1000803 (Washington, DC). Partial financial support for the study by the Walton Family Foundation and The Builders Initiative is also acknowledged.
The authors declare that the research was conducted in the absence of any commercial or financial relationships that could be construed as a potential conflict of interest.
All claims expressed in this article are solely those of the authors and do not necessarily represent those of their affiliated organizations, or those of the publisher, the editors and the reviewers. Any product that may be evaluated in this article, or claim that may be made by its manufacturer, is not guaranteed or endorsed by the publisher.
Abbott D.W., Aasen I. M., Beauchemin K. A., Grondahl F., Gruninger R., Hayes M., et al. (2020). Seaweed and seaweed bioactives for mitigation of enteric methane: Challenges and opportunities. Animals 10 (12), 1–28. doi: 10.3390/ani10122432
Andreakis N., Procaccini G., Kooistra W. H. C. F. (2004). Asparagopsis taxiformis and asparagopsis armata (Bonnemaisoniales, rhodophyta): Genetic and morphological identification of Mediterranean populations. Eur. J. Phycology 39 (3), 273–835. doi: 10.1080/0967026042000236436
Antaya N. T., Ghelichkhan M., Pereira A. B. D., Soder K. J., Brito A. F.. (2019). Production, milk iodine, and nutrient utilization in Jersey cows supplemented with the brown seaweed Ascophyllum nodosum (kelp meal) during the grazing season. J. Dairy Sci. 102 (9), 8040–8058. doi: 10.3168/jds.2019-16478
Arndt C., Hristov A. N., Price W. J., McClelland S. C., Pelaez A. M., Cueva S. F., et al. (2022). Full adoption of the most effective strategies to mitigate methane emissions by ruminants can help meet the 1.5 °C target by 2030 but not 2050. Proc. Natl. Acad. Sci. 119 (20), e2111294119. doi: 10.1073/pnas.2111294119
Becker P. M., van Wikselaar P. G., Franssen M. C. R., de Vos R. C. H., Hall R. D., Beekwilder J. (2014). Evidence for a hydrogen-sink mechanism of (+)Catechin-mediated emission reduction of the ruminant greenhouse gas methane. Metabolomics 10 (2), 179–895. doi: 10.1007/s11306-013-0554-5
Carpenter L. J., Liss P. S. (2000). On temperate sources of bromoform and other reactive organic bromine gases. J. Geophysical Res. Atmospheres 105 (D16), 20539–20547. doi: 10.1029/2000JD900242
Chagas J. C., Ramin M., Krizsan S. J. (2019). In vitro evaluation of different dietary methane mitigation strategies. Animals 9 (12), 11205. doi: 10.3390/ani9121120
Erickson P. S., Marston S. P., Gemmel M., Deming J., Cabral R. G., Murphy M. R., et al. (2012). Short communication: Kelp taste preferences by dairy calves. J. Dairy Sci. 95 (2), 856–858. doi: 10.3168/jds.2011-4826
Glasson C. R. K., Kinley R. D., de Nys R., King N., Adams S. L., Packer M. A., et al. (2022). Benefits and risks of including the bromoform containing seaweed asparagopsis in feed for the reduction of methane production from ruminants. Algal Res 102 (9):8040–8058. doi: 10.1016/j.algal.2022.102673
Goel G., Makkar H. P. S., Becker K. (2009). Inhibition of methanogens by bromochloromethane: Effects on microbial communities and rumen fermentation using batch and continuous fermentations. Br. J. Nutr. 101 (10), 1484–1925. doi: 10.1017/S0007114508076198
Hatew B., Stringano E., Mueller-Harvey I., Hendriks W. H., Hayot Carbonero C., Smith L. M. J., et al. (2016). Impact of variation in structure of condensed tannins from sainfoin (Onobrychis viciifolia) on in vitro ruminal methane production and fermentation characteristics. J. Anim. Physiol. Anim. Nutr. 100 (2), 348–360. doi: 10.1111/jpn.12336
Hristov A. N., Melgar A., Wasson D., Arndt C. (2022). Symposium review: Effective nutritional strategies to mitigate enteric methane in dairy cattle. J. Dairy Sci. doi: 10.3168/JDS.2021-21398
Jackson R. B., Saunois M., Bousquet P., Canadell J. G., Poulter B., Stavert A. R., et al. (2020). Increasing anthropogenic methane emissions arise equally from agricultural and fossil fuel sources. Environ. Res. Lett 15 (7):1002. doi: 10.1088/1748-9326/ab9ed2
Janssen P. H. (2010). Influence of hydrogen on rumen methane formation and fermentation balances through microbial growth kinetics and fermentation thermodynamics. Anim. Feed Sci. Technol 160 (1–2):1–22. doi: 10.1016/j.anifeedsci.2010.07.002
Johnson K. A., Johnson D. E. (1995). Methane emissions from cattle. J. Anim. Sci. 73 (8), 2483–2492. doi: 10.2527/1995.7382483x
Key N., Sneeringer S. (2011). arbon Prices and the Adoption of Methane Digesters on Dairy and Hog Farms, EB-16 U.S. Department of Agriculture, Economic Research Service. Available at: https://www.ers.usda.gov/webdocs/publications/42846/7864_eb16.pdf?v=0. (Accessed: 30 August 2022).
Kim J. K., Stekoll M., Yarish C. (2019). Opportunities, challenges and future directions of open-water seaweed aquaculture in the united states. Phycologia 58 (5), 446–615. doi: 10.1080/00318884.2019.1625611
Kinley R. D., De Nys R., Vucko M. J., MacHado L., Tomkins N. W. (2016). The red macroalgae asparagopsis taxiformis is a potent natural antimethanogenic that reduces methane production during in vitro fermentation with rumen fluid. Anim. Production Sci. 56 (3), 282–895. doi: 10.1071/AN15576
Kinley R. D., Martinez-Fernandez G., Matthews M. K., de Nys R., Magnusson M., Tomkins N. W. (2020). Mitigating the carbon footprint and improving productivity of ruminant livestock agriculture using a red seaweed. J. Cleaner Production 259, 120836. doi: 10.1016/j.jclepro.2020.120836
Kinley R. D., Tan S., Turnbull J., Askew S., Roque B. M. (2021). Changing the proportions of grass and grain in feed substrate impacts the efficacy of Asparagopsis taxiformis to inhibit methane production in vitro. Am. J. Plant Sci. 12 (12), 1835–1858. doi: 10.4236/ajps.2021.1212128
Kvidera S. K., Dickson M. J., Abuajamieh M., Snider D. B., Sanz Fernandez M. V., Johnson J. S., et al. (2017). Intentionally induced intestinal barrier dysfunction causes inflammation, affects metabolism, and reduces productivity in lactating Holstein cows. J. Dairy Sci. 100 (5), 4113–4127. doi: 10.3168/JDS.2016-12349
Li X., Norman H. C., Kinley R. D., Laurence M., Wilmot M., Bender H., et al. (2018). Asparagopsis taxiformis decreases enteric methane production from sheep. Anim. Production Sci. 58 (4), 681–885. doi: 10.1071/AN15883
Machado L., Magnusson M., Paul N. A., De Nys R., Tomkins N. (2014). Effects of marine and freshwater macroalgae on in vitro total gas and methane production. PloS One 9 (1), e852895. doi: 10.1371/journal.pone.0085289
Machado L., Magnusson M., Paul N. A., Kinley R., de Nys R., Tomkins N. (2016a). Identification of bioactives from the red seaweed asparagopsis taxiformis that promote antimethanogenic activity in vitro. J. Appl. Phycology 28 (5), 3117–3265. doi: 10.1007/s10811-016-0830-7
Machado L., Magnusson M., Paul N. A., Kinley R., de Nys R., Tomkins N. (2016b). Dose-response effects of asparagopsis taxiformis and oedogonium sp. on in vitro fermentation and methane production. J. Appl. Phycology 28 (2), 1443–1525. doi: 10.1007/s10811-015-0639-9
Machado L., Tomkins N., Magnusson M., Midgley D. J., de Nys R., Rosewarne C. P. (2018). In vitro response of rumen microbiota to the antimethanogenic red macroalga asparagopsis taxiformis. Microbial Ecol. 75 (3), 811–185. doi: 10.1007/s00248-017-1086-8
Magnusson M., Vucko M. J., Neoh T. L., de Nys R. (2020). Using oil immersion to deliver a naturally-derived, stable bromoform product from the red seaweed asparagopsis taxiformis. Algal Res. 51:1–7. doi: 10.1016/j.algal.2020.102065
Masson-Delmotte Valérie, Zhai P., Pörtner H.-O., Roberts D., Skea J., Shukla P. R., et al. (2019) Global warming of 1.5°C an IPCC special report on the impacts of global warming of 1.5°C above pre-industrial levels and related global greenhouse gas emission pathways, in the context of strengthening the global response to the threat of climate change. Available at: www.environmentalgraphiti.org.
Mata L., Gaspar H., Santos R. (2012). Carbon/Nutrient balance in relation to biomass production and halogenated compound content in the red alga asparagopsis taxiformis (Bonnemaisoniaceae). J. Phycology 48 (1), 248–535. doi: 10.1111/j.1529-8817.2011.01083.x
Mata L., Lawton R. J., Magnusson M., Andreakis N., de Nys R., Paul N. A. (2017). Within-species and temperature-related variation in the growth and natural products of the red alga asparagopsis taxiformis. J. Appl. Phycology 29 (3), 1437–1475. doi: 10.1007/s10811-016-1017-y
Melgar A., Harper M. T., Oh J., Giallongo F., Young M. E., Ott T. L., et al. (2020). Effects of 3-nitrooxypropanol on rumen fermentation, lactational performance, and resumption of ovarian cyclicity in dairy cows. J. Dairy Sci. 103 (1), 410–432. doi: 10.3168/jds.2019-17085
Muizelaar W., Groot M., van Duinkerken G., Peters R., Dijkstra J. (2021). Safety and transfer study: Transfer of bromoform present in asparagopsis taxiformis to milk and urine of lactating dairy cows. Foods 10 (3), 5845. doi: 10.3390/foods10030584
Nilsson J., Martin M. (2022). Exploratory environmental assessment of Large-scale cultivation of seaweed used to reduce enteric methane emissions. Sustain. Production Consumption 30, 413–423. doi: 10.1016/j.spc.2021.12.006
Nkemka V. N., Beauchemin K. A., Hao X. (2019). Treatment of feces from beef cattle fed the enteric methane inhibitor 3-nitrooxypropanol. Water Sci. Technol. 80 (3), 437–475. doi: 10.2166/wst.2019.302
Norton T. A., Chapman V. J., Chapman D. J. (1981). Seaweeds and their uses. J. Ecol. 69:1–334. doi: 10.2307/2259655
Oskoueian E., Abdullah N., Oskoueian A. (2013). Effects of flavonoids on rumen fermentation activity, methane production, and microbial population. BioMed. Res. Int 2013:1–8. doi: 10.1155/2013/349129
Owens J. L., Thomas B. W., Stoeckli J. L., Beauchemin K. A., McAllister T. A., Larney F. J., et al. (2020). Greenhouse gas and ammonia emissions from stored manure from beef cattle supplemented 3-nitrooxypropanol and monensin to reduce enteric methane emissions. Sci. Rep. 10 (1), 1–145. doi: 10.1038/s41598-020-75236-w
Passetti R. A.C., Hegarty R. S. (2022). “An assessment of industry preparedness for methane-lowering feed additives tract,” in 8th Annual Global Greenhouse Gas & Animal Agriculture Conference. 225.
Patra A., Park T., Kim M., Yu Z. (2017). Rumen methanogens and mitigation of methane emission by anti-methanogenic compounds and substances. J. Anim. Sci. Biotechnol 8 (1):1–18. doi: 10.1186/s40104-017-0145-9
Patra A. K., Saxena J. (2009). Dietary phytochemicals as rumen modifiers: A review of the effects on microbial populations. Antonie van Leeuwenhoek Int. J. Gen. Mol. Microbiol 96 (4):363–375. doi: 10.1007/s10482-009-9364-1
Patra A. K., Saxena J. (2010). A new perspective on the use of plant secondary metabolites to inhibit methanogenesis in the rumen. Phytochemistry 71 (11–12), 1198–1222. doi: 10.1016/j.phytochem.2010.05.010
Paul N. A., De Nys R., Steinberg P. D. (2006). Chemical defence against bacteria in the red alga asparagopsis armata: Linking structure with function. Mar. Ecol. Prog. Ser. 306, 87–101. doi: 10.3354/meps306087
Pomin V. H. (2009). Review: An overview about the structure-function relationship of marine sulfated homopolysaccharides with regular chemical structures. Biopolymers 91 (8), 601–609. doi: 10.1002/BIP.21200
Ramin M., Chagas J. C. C., Guyader T., Kriszan S. J. (2022). “Reducing methane production of stored feces by asparagopsis taxiformis from Swedish dairy cows,” in 8th Annual Global Greenhouse Gas & Animal Agriculture Conference. 231.
Romanazzi D., Sanchez-Garcia C., Svenson J., Mata L., Pes K., Hayman C. M., et al. (2021). Rapid analytical method for the quantification of bromoform in the red seaweeds asparagopsis armata and asparagopsis taxiformis using gas chromatography-mass spectrometry. ACS Agric. Sci. Technol. 1 (5), 436–425. doi: 10.1021/acsagscitech.1c00161
Romero P., Belanche A., Hueso R., Ramos-Morales E., Salwen J. K., Kebreab E., et al. (2022b). “In vivo rumen microbial degradation of bromoform and the impact on rumen fermentation,” in 8th Annual Global Greenhouse Gas & Animal Agriculture Conference. 291.
Romero P., Huang R., Jimenez E., Palma-Hidalgo J., Martin-Garcia A.I., Ungerfeld E. M., et al. (2022a). “In vivo study of combining asparagopsis taxiformis and phloroglucinol to reduce methane production and improve rumen fermentation efficiency in goats,” in In 8th Annual Global Greenhouse Gas & Animal Agriculture Conference. 239.
Roque B. M., Salwen J. K., Kinley R., Kebreab E. (2019). Inclusion of asparagopsis armata in lactating dairy cows’ diet reduces enteric methane emission by over 50 percent. J. Cleaner Production 234, 132–138. doi: 10.1016/j.jclepro.2019.06.193
Roque B. M., Venegas M., Kinley R. D., De Nys R., Duarte T. L., Yang X., et al. (2021). Red seaweed (Asparagopsis taxiformis) supplementation reduces enteric methane by over 80 percent in beef steers. PloS One 16:1–20. doi: 10.1371/journal.pone.0247820
Searchinger T., Waite R., Hanson C., Ranganathan J., Matthews E. (2019). World Resources Institute-Creating a sustainable food future. Available at: https://www.wri.org/research/creating-sustainable-food-future.
Stefenoni H. A., Räisänen S. E., Cueva S. F., Wasson D. E., Lage C. F. A., Melgar A., et al. (2021). Effects of the macroalga asparagopsis taxiformis and oregano leaves on methane emission, rumen fermentation, and lactational performance of dairy cows. J. Dairy Sci 104 (4):4157–4173. doi: 10.3168/jds.2020-19686
Thauer R. K. (2012). The Wolfe cycle comes full circle. Proc. Natl. Acad. Sci. United States America 109 (38), 15084–15085. doi: 10.1073/PNAS.1213193109
U.S. Environmental Protection Agency National Center for Environmental Assessment (1987). Integrated Risk Information Systems Chemical Assessment Summary- Bromoform; CASRN 75-25-2. Available at: https://cfpub.epa.gov/ncea/iris/iris_documents/documents/subst/0214_summary.pdf. (Accessed: 30 August 2022).
VanBriesen J. M. (2013). Potential drinking water effects of bromide discharges from coal-fired electric power plants. Formal Comments of the Environmental Integrity Project on the Effluent Limitations Guidelines and Standards for the Steam Electric Power Generating Point Source Category, Appendix B of Water of Docket EPA-HQ-OW-2009-0819-4687. Available at: https://www3.epa.gov/region1/npdes/merrimackstation/pdfs/Comments2RevisedDraftPermit/VanBriesenReport.pdf.
Vijn S., Compart D. P., Dutta N., Foukis A., Hess M., Hristov A. N., et al. (2020). Key considerations for the use of seaweed to reduce enteric methane emissions from cattle. Front. Veterinary Sci. 7. doi: 10.3389/fvets.2020.597430
Vucko M. J., Magnusson M., Kinley R. D., Villart Céline, de Nys R. (2017). The effects of processing on the in vitro antimethanogenic capacity and concentration of secondary metabolites of asparagopsis taxiformis. J. Appl. Phycology 29 (3), 1577–1865. doi: 10.1007/s10811-016-1004-3
WHO (2009). Bromide in drinking water: Background document for development of WHO guidelines for drinking-water quality (Geneva, Switzerland: World Health Organization). Available at: http://www.who.int/water_sanitation_health/dwq/chemicals/Fourth_Edition_Bromide_Final_January_2010.pdf.
Wolin M. J., Miller T. L., Stewart C. S. (1997). Microbe-microbe interactions. The Rumen Microbial Ecosystem Dordrecht: Springer, 467–491. doi: 10.1007/978-94-009-1453-7_11
Keywords: seaweed, enteric methane, cattle, sustainability, climate
Citation: Wasson DE, Yarish C and Hristov AN (2022) Enteric methane mitigation through Asparagopsis taxiformis supplementation and potential algal alternatives. Front. Anim. Sci. 3:999338. doi: 10.3389/fanim.2022.999338
Received: 20 July 2022; Accepted: 31 August 2022;
Published: 03 October 2022.
Edited by:
Alexandros Mavrommatis, Agricultural University of Athens, GreeceReviewed by:
Panagiotis Simitzis, Agricultural University of Athens, GreeceCopyright © 2022 Wasson, Yarish and Hristov. This is an open-access article distributed under the terms of the Creative Commons Attribution License (CC BY). The use, distribution or reproduction in other forums is permitted, provided the original author(s) and the copyright owner(s) are credited and that the original publication in this journal is cited, in accordance with accepted academic practice. No use, distribution or reproduction is permitted which does not comply with these terms.
*Correspondence: Derek E. Wasson, ZGV3NTE0OEBwc3UuZWR1
Disclaimer: All claims expressed in this article are solely those of the authors and do not necessarily represent those of their affiliated organizations, or those of the publisher, the editors and the reviewers. Any product that may be evaluated in this article or claim that may be made by its manufacturer is not guaranteed or endorsed by the publisher.
Research integrity at Frontiers
Learn more about the work of our research integrity team to safeguard the quality of each article we publish.