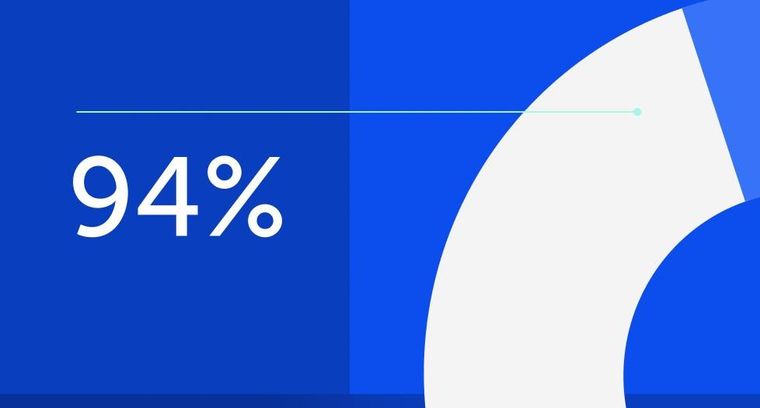
94% of researchers rate our articles as excellent or good
Learn more about the work of our research integrity team to safeguard the quality of each article we publish.
Find out more
ORIGINAL RESEARCH article
Front. Anim. Sci., 15 September 2022
Sec. Precision Livestock Farming
Volume 3 - 2022 | https://doi.org/10.3389/fanim.2022.997948
The usefulness of acoustic telemetry on the study of movements, interactions, and behaviors has been revealed by many field and laboratory studies. The process of attaching acoustic tags on fish can, however, impact their physiological, behavioral, and growth performance traits. The potential negative effects are still unknown for several species and behavioral attributes. Previous studies have attempted to shed light on the effects of tag implantation on fish, focusing mainly on fish growth and physiological parameters, and one or two behavioral properties mainly on the individual level. However, the effect of this procedure could also be expressed at the group level. This study investigated the short-term effects of dummy and active body-implanted acoustic tags on the group-level swimming performance of adult European seabass (Dicentrarchus labrax) using optical flow analysis. We studied four main swimming performance properties—group speed, alignment (polarization), cohesion, and exploratory behavior. To help in the interpretation of any detected differences, physiological stress-related parameters were also extracted. The results show that the tag implantation procedure has variable effects on the different swimming performance attributes of fish. Group cohesion, polarization, and the group’s exploratory tendency were significantly impacted initially, and the effect persisted but to a lesser extent two weeks after surgery. In contrast, group speed was not affected initially but showed a significant decrease in comparison with the control group two weeks post-surgery. In addition, the physiological parameters tested did not show any significant difference between the control and the treated group 14 days after the onset of the experiment. The findings suggest that the effect of tagging is non-trivial, leading to responses and response times that could affect behavioral studies carried out using acoustic telemetry.
Acoustic telemetry has been proven to be a useful tool for the better understanding of biological processes (Hussey et al., 2015; Abecasis et al., 2018; Schwinghamer et al., 2019; Villegas-Ríos et al., 2020). The wild adult chinook (Oncorhynchus tshawytscha) and coho salmon (Oncorhynchus kisutch) were among the first fish species used in such studies (Trefethen, 1956). Since then, acoustic transmitters have been incorporated in studies of a wide range of marine species (Brownscombe et al., 2019; Klinard and Matley, 2020) such as sharks (Espinoza et al., 2021), the salmonid smolt (Huusko et al., 2016), the Atlantic cod (Gadus morhua; Meager et al., 2009), the European seabass (Dicentrarchus labrax; Anras et al., 1997; Stamp et al., 2021), and the gilthead seabream (Sparus aurata; Arechavala-Lopez et al., 2012; Arechavala-Lopez et al., 2021). In addition, studies have benefited from the use of acoustic transmitters (Meager et al., 2009; Brownscombe et al., 2019; Muñoz et al., 2020); for example, studies on fish locomotion (Espinoza et al., 2021), the estimation of energetic costs (Wright et al., 2014; Zupa et al., 2015; Zupa et al., 2021; Alfonso et al., 2021a; Alfonso et al., 2022), residency patterns and habitat usage (Espinoza et al., 2020; Zhang et al., 2020; Lippi et al., 2022; Marques et al., 2022), intra-species/inter-species interactions (Barkley et al., 2020; Lees et al., 2020), feeding behavior (Føre et al., 2011), eco-physiology, and reproductive behavior (Klinard and Matley, 2020). Acoustic telemetry is, therefore, an important tool for behavioral and eco-physiological studies in aquatic environments.
The aquaculture industry has also adopted the use of acoustic telemetry systems, primarily in Atlantic salmon (Salmo salar L.) farming (Føre et al., 2011; Føre et al., 2017; Svendsen et al., 2021). Telemetry-based systems monitor the fish during farm operations to gather knowledge about the species’ responses during crowding and delousing events in commercial sea-cages (Føre et al., 2018; Carbonara et al., 2020) as well as during feeding (Føre et al., 2011). Researchers use acoustic tags to detect changes in the behavior of aquaculture species in relation to environmental conditions caused by the fall overturn, exposure to artificial underwater lights in fully stocked production cages (Ulvund et al., 2021), storm conditions, and feeding variations (Føre et al., 2011). Respective studies on Mediterranean aquaculture species such as the European seabass and gilthead seabream, although few, also exist. Telemetry sensors are explored as a tool for health and welfare monitoring of E. seabass farming (Alfonso et al., 2020a; Carbonara et al., 2021; Alfonso et al., 2022) in assessing optimal stocking density and diet regimes (Begout and Lagardere, 1995; Anras et al., 1997). Furthermore, acoustic transmitters have been used to study the swimming activity, space utilization, response of fish to variations in stocking density (Carbonara et al., 2019), and physiological response of gilthead seabream in aquaculture conditions (Alfonso et al., 2021b; Arechavala-Lopez et al., 2021).
The acoustic transmitters can be externally attached (Runde et al., 2022), inserted into the stomach via injection, or surgically implanted into an individual fish depending on the size, species, and objectives of the study (Hussey et al., 2015). Then, acoustic hydrophones or “receivers” deployed in the animal’s environment detect and record the acoustic signals transmitted by any tagged individuals within the detection range of the receivers. In fish acoustic telemetry studies, tags are most frequently implanted surgically into the celomic cavity (Jepsen et al., 2002; Cooke et al., 2011; Cooke et al., 2013).
Fitting a fish with an acoustic tag via intracelomic implantation requires capture, anesthesia, surgery, and release, all of which disturb the animal and can cause stress (Cooke et al., 2011; Walker et al., 2016). Fish under stress primarily activate a cascade of neuroendocrine responses, such as releasing catecholamines and glucocorticoids, and then display a range of physiological reactions (Barreto et al., 2022), e.g. an increased heart rate and the utilization of metabolic energy stores. They can also be influenced in a more systematic way, by affecting the whole-body performance, the fish growth and behavior, e.g. developing unusual swimming patterns (Barton, 2002; Skomal and Mandelman, 2012; Schreck and Tort, 2016; Urbinati et al., 2020). Acoustic telemetry tags may, therefore, influence the physiological, behavioral, and swimming performance attributes of the fish (Frank et al., 2009; McKenna et al., 2021), and these changes could be permanent or temporal, leading to erroneous data (Huusko et al., 2016; Klinard et al., 2018; Daniels et al., 2021; Barreto et al., 2022).
There are numerous studies addressing the impact of tag use and attachment on fish (Bridger and Booth, 2003). A common conception is that a transmitter should weigh less than 2% of the body mass of a fish or 1.25% when in water (Winter, 1983) as heavier tags could affect its swimming performance, growth, survival, and behavior (Winter, 1983; Paukert et al., 2001). Recent studies, however, suggest that this rule need not be strictly followed as it depends on the species and the question of interest (Paukert et al., 2001; Lacroix et al., 2004; Childs et al., 2011). In addition, numerous studies have revealed results that both support (Moore et al., 1990; Bégout Anras and Lagardère, 2004; Loher and Rensmeyer, 2011; Neves et al., 2018) and contradict (Adams et al., 1998; Brown et al., 2006; Svendsen et al., 2021) the idea that the surgery and presence of the tag do not cause changes in the physiology or behavior in different species. The studies vary in experimental duration, the effect on the fish, and the species used. The most studied species is the Atlantic salmon (Adams et al., 1998; Føre et al., 2021), but other species such as the Atlantic cod, European seabass, green sturgeon, European flounder and gilthead seabream have also been selected for investigation (Bégout Anras et al., 2003; Cooke et al., 2011; Montoya et al., 2012; Miller et al., 2014; Neves et al., 2018). There are short-term studies focusing on the first minutes, hours, and days after tag implantation (Lacroix et al., 2004; Montoya et al., 2012; Ammann et al., 2013; Neves et al., 2018; Svendsen et al., 2021), and long-term studies that focus on the effects years after the surgery (Koed and Thorstad, 2001; Childs et al., 2011; Smukall et al., 2019). Furthermore, most studies focus on the effect of the tags on the survival and physiological responses of the fish (Anras and Lagardère, 1998; Morris et al., 2000; Paukert et al., 2001; Bégout Anras and Lagardère, 2004; Childs et al., 2011; Loher and Rensmeyer, 2011; Ammann et al., 2013; Newton et al., 2016; Klinard et al., 2018), and fewer studies that focus on the behavioral and swimming alterations that tagging could cause (Swanberg and Geist, 1997; Adams et al., 1998; Koed and Thorstad, 2001; Zale et al., 2005; Montoya et al., 2012; Perry et al., 2013; Walker et al., 2016; Neves et al., 2018). These mainly test one swimming performance variable such as the speed, vertical distribution, or polarization of the group, or they use a general measure for activity.
Morphological and anatomical variations across fish species and life history stages reinforce the need to evaluate responses to surgical procedures and tag presence. As a wide spectrum of behaviors and swimming performance characteristics could be affected by stressors (Ginnaw et al., 2020), it is important to test tag implantation implications on multiple behavioral attributes, particularly when studying the individual or group movement responses of fish. Such studies are particularly necessary in aquaculture where the behavior of cultured fish under optimal and sub-optimal conditions needs to be determined and welfare indicators and their optimal ranges need to be quantified (Bridger and Booth, 2003).
In the current work, we used single cameras, computer vision techniques, and optical flow analysis to estimate group-level swimming features such as the speed, synchronization (a.k.a polarization), cohesion, and exploratory behavior of adult European seabass. In addition, we applied our methodology to evaluate the short-term effects (two weeks) of surgical implantation of dummy and active acoustic tags on the physiological stress indicators and on the group swimming performance of European seabass. The selection of the experimental duration was based on previous studies that showed recovery of the studied behavioral parameter within the first week of the treatment (Lacroix et al., 2004; Alfonso et al., 2021b). Based on recent studies, we hypothesize that the fish will show variable behavioral responses (Ginnaw et al., 2020).
The experimental protocol was approved by the Ethics Committee of the IMBBC and the relevant veterinary authorities (Ref Number 32257 09-02-2021) in accordance with legal regulations (EU Directive 2010/63).
The trial was conducted at the AquaLabs facility of the Institute of Marine Biology, Biotechnology, and Aquaculture (IMBBC), which is certified (EL91‐BIOexp‐04) for fish experimentation. A group of 45 fish of total length 29.92 ± 1.8 cm and weight 297.88 ± 43.5 g was obtained from the institute’s pilot-scale cage farm (Souda Bay, Crete, Figure 1) and transferred to our land-based facilities (to a 5 m3 flow-through rectangular tank of 3.0x3.0x0.6m dimensions) thirty days prior to the experiment to allow for adaptation (T=19 °C, pH=8.0, salinity=36 p s u, DO > 5 and ambient photoperiod). We built dummy acoustic tags, i.e. polyacetal (POM) cylinders of 23.3 mm length, 7 mm diameter, 1.4 g weight in water, and< 2% tag-to-body-weight ratio (Jepsen et al., 2005), inspired by the Thelmabiotel acoustic transmitters (https://www.thelmabiotel.com/, Figure 2B). For our study species (European seabass), we selected the Thelma Biotel 7.3 mm acoustic transmitters that were originally designed for use with salmon smolt and are the appropriate size for our experimental groups. We implanted them into the peritoneal cavity of twenty fish (WDummy=295.7±35.2 g ) on the 8th of March 2021, and used the remaining fish as the control group (Ncontrol= 25, WControll=286.6±40.3 g ). We subjected the fish in both groups to capture, anesthetization, weighing, and blood sampling.
Figure 2 Explanatory photographic material about the experimental procedure. (A) Blood sampling, (B) Dummy and active tags used for the experiment, (C) Intracelomic implantation of the tags.
The physiological stress indicators of the control and dummy groups were extracted at the beginning (before tag implantation) and the end (before tag removal) of the experiment. We drew blood samples from each group twice (10 fish per group) – on the 8th of March before the tagging procedure (to have a control value to the procedure) and on the 21st of March before the tag removal. The samples were collected from the caudal vessel via heparinized syringes and placed in heparin tubes (Figure 2A). Cortisol, glucose, and lactate levels were measured to estimate acute stress, and hematological indicators (hematocrit and hemoglobin) were determined to detect the potential emergence of chronic stress caused by the procedure. Following the determination of hematological parameters, blood was centrifuged (2,000 g) and plasma was aliquoted and stored at 20°C until analysis. Plasma cortisol was measured using a commercial enzyme immunoassay kit (Cortisol ELISA, DRG® International Inc, Germany). Glucose and lactate were measured by enzymatic colorimetric methods used routinely in the fish physiology lab of University of Crete (GOD/PAP, Biosis, Greece, and LO-POD, Spinreact, Sant Esteve De Bas (GI), Spain, for glucose and lactate, respectively). Hematocrit was measured in capillary tubes after centrifugation in a hematocrit microcentrifuge, and hemoglobin was determined using a commercial kit (Spinreact, Girona, Spain).
Prior to the surgery, we decreased the volume of the tank to 1 m3 and partially anesthetized the fish with 100 ppm clove oil to facilitate their capture. We then transferred each fish individually to a bucket with water containing 300 ppm clove oil and left it for a few minutes until fully anesthetized. We then measured the total length and weight of each fish and we collected a blood sample (explained above). For tagging, we subsequently placed the fish in a supine position in a V-shaped cavity with an elastic coating (to hold the fish and avoid damaging the protective mucus layer, Figure 2C) and inserted the tag through an incision made along the mid-abdominal line with a scalpel (after being disinfected with alcohol and washed with water) while lifting the fins with forceps to prevent any damage. We inserted the tag at an oblique angle to the fish so that it maintained a horizontal position inside the fish. The incision was closed by suturing between the two sides of the skin with absorbable monofilament sutures (the needle was passed through each side of the incision and closed with a surgeon’s knot) and then covered with betadine for antiseptic purposes. All fish survived the treatment.
Immediately after the handling, we returned the fish to a smaller tank (0.5 m3) until complete recovery, and then the treated group and the control group were placed into two different tanks (of 5 m3 volume each) reaching a stocking density of 1.2 Kg/m3 for the treated group and 1.5 Kg/m3 for the control group. We recorded behaviors in both tanks (from the top point of view) using fixed IP cameras (see the ‘camera setup and calibration’ and ‘data extraction and analysis’ sections) for a period of two weeks (08 March – 21 March 2021). We fed the fish once daily at around 12:00 and siphoned the tanks every two days (maintenance days). We commenced behavioral analysis on the second day after the surgery (i.e. after the experimental conditions, including the water transparency, in the tanks were set), excluding the maintenance days as this procedure could temporally impact fish behavior (Lacroix et al., 2004; Alfonso et al., 2021b).
In order to test the repeatability of our observations, we took advantage of a second trial in which a group of fish was implanted with active tags and monitored in a net pen cage. Hence, we monitored their behavior before transfer to cages in a set up similar to that of the dummy group (Figure 1). The tagging procedure was repeated using active acoustic tags in a group of 24 fish of total length 32.17 ± 6.4 cm and weight WActive= 398.2 ± 73.3 g , with a similar origin and procedure as the previous group. The stocking density during the recording period was 1.9 Kg/m3 . We implanted the transmitters (Thelma Biotel 7.3 mm diameter, 23.2 mm length, 1.8 g weight in water, and< 2% tag-to-body-weight ratio; Gruen and Huang, 2001) into the peritoneal cavity of the fish on the 26th of April 2021. All fish survived the treatment. After the surgery, we returned the fish to their tank and recorded their activity using the same camera set-up as above for a 2-week period (26 April – 10 May 2021). During this period feeding was realized once per day at around 12:00, and maintenance activities (siphoning the tanks) every two days. To avoid any potential additional stress, we did not draw blood samples from the fish.
For both experiments, the cameras were positioned at the same height above both tanks and pointed downwards (at a 90° angle with the surface) to the center of the tank. The cameras captured 6 fps and acquired frames of 1280 x 720 resolution (pixels) (HIKVISION DS-2CD1623G0-IZS). Before analyzing the collected images, the cameras were calibrated.
Single-camera calibration involves the estimation of intrinsic parameters (focal length, optical center or principal point, pixel size) to correct lens distortion and sea water refraction (Gruen and Huang, 2001; Hartley and Zisserman, 2004). In this study, geometrical calibration was applied using a pattern with known distances (chessboard). The calibration was conducted above water for the tanks after capturing a sufficient number of chessboard images. The cameras were calibrated using the OpenCV library in Python and the resulting parameters used to correct the extracted video frames.
We developed custom-made routines that can extract swimming performance attributes using OpenCV and Python and we applied them on consecutive ten-minute videos between 08:00 and 19:00 each day. The analysis was performed for three days – the 3rd, the 11th, and the 14th day – per trial (no maintenance of the tanks took place on those days). More specifically, four main variables were extracted, i.e. the group speed, the group polarization, the relative tank area the group covers, and the group’s exploratory tendency.
Group polarization and speed were extracted using optical flow analysis (Lucas and Kanade, 1981; Farnebäck, 2003). Initially, a K-Nearest Neighbors background subtractor was applied to segment each frame, i.e. to distinguish between the moving fish (foreground) and the static environment (background), and the segmented image was used as a mask for the next step. Next, optical flow analysis was performed using Farnebäck’s dense optical flow from OpenCV to track each pixel in the image for the consecutive time frames (Farnebäck, 2003). The velocity vector for each pixel at each time step was estimated, and Median and Gaussian filters were applied to smooth the speed and the direction values and remove errors. Next, the extracted mask image containing the position of the fish was applied to the vector image to exclude irrelevant background motion, and the average group speed and direction were extracted. In addition, group polarization, which describes the alignment of the group, was calculated from the following equation (Tunstrøm et al., 2013):
where ui is the unit direction of pixel i , and Op takes values between 0 (no alignment on average) and 1 (all fish are aligned).
The relative tank area (in percent) covered by the group in each timeframe was estimated and used as a measure for the cohesiveness of the group (MacGregor and Ioannou, 2021). To calculate it, the segmented contours corresponding to the fish were merged into a larger contour and its convex hull extracted.
Then, the convex hull area was calculated and divided by the total area of the tank (relative area). The relative area of the group was divided by the number of fish of each group and multiplied by the same number (N = 24), so that the relative group area is comparable between the groups (we assumed that fish overlaps and occlusions were not common, and if they occurred, they did not last for more than ~3−4 frames). Smaller values (closer to zero) indicate more cohesive groups, and larger values the opposite.
The exploratory tendency, i.e. the spatial preference of the group, was expressed in how often the group visited each tank site, and was calculated as follows: First, the video frame was analyzed to distinguish between the background pixels and the fish pixels (using the K-Nearest Neighbors background subtractor as above). If fish were present at a tank site represented by pixel i , the pixel took the value Ii = 1 ; otherwise the pixel took the value Ii = 0 . The exploration of the group at each site i for N time frames was then defined as:
In addition, the relative position of the group to the center of the tank (Ctank ) at each timeframe t was calculated as:
where, is the group center at timeframe t. As the fish are moving in a confined environment, the distance from the center approaches 0 if the group explores the whole tank while being less cohesive. The distance will be different from 0 if the fish explore the tank in a cohesive group. Heatmaps of the relative distance were then created to test if the fish groups showed a specific preference in the tank or visited all the tank sites.
Before testing any behavioral response to tag implantation, feeding activity (i.e. feed consumption) during the trial period was analyzed. Then, to test the effect of tagging on the physiological parameters of the control and the treated groups, one-way ANOVA was implemented using the Sigma Stat 3.1 statistical package (Systat Software, Inc., San Jose, CA, USA) followed by Tukey’s multiple comparison tests. Before each ANOVA test, data were analyzed for normality using the Kolmogorov–Smirnov test, and for homogeneity of variance using Levene’s test. When the previous assumptions were not followed, data were log-transformed before analysis (lactate). The assumption of homogeneity of variance was not met by the hemoglobin data, and they were analyzed with non-parametric ANOVA on ranks (a.k.a Kruskal-Wallis) test (Sigma Stat 3.1).
To detect differences in the cohesion between groups, we applied Kruskal-Wallis tests on our data in R (R version 4.2.0; R Core Team, 2018) as normality criterion was not met. Kruskal-Wallis tests were also used for the comparison between the control and treated groups for the polarization and the speed variables. After Kruskal-Wallis test gave significant results, we run Pairwise Wilcoxon Mann-Whitney Rank Sum Test to compute group pairwise differences. We also used R for our graphical presentation of the results.
The control and dummy group did not show significantly different weights (χ2 statistic: 0.6, p-value = 0.4). However, the weight of active group differed significantly from both, the control and the dummy group (χ2 statistic: 31, p-value< 0.001). In addition, in both treatments, i.e. the dummy and active, the treated groups did not differ in their consumption rate from the control group two weeks after surgery, and the apparent per capita normalized feed consumption rate (as feed weight per body weight) was approximately 0.8% per day .
Plasma glucose concentrations (mean ± SEM) did not present statistically significant differences between groups (3.9 ± 0.3 mmol l-1, 4.2 ± 0.4 mmol l-1, and 4.5 ± 0.3 mmol l-1 for the control of the 1st and 14th day and the dummy of the 14th day, respectively) (F2,36 = 1.1, P-value = 0.35, a = 0.05, Figure 3).
Figure 3 Plasma concentrations (mean ± SEM) of (A) cortisol, (B) glucose and (C) lactate of the control group in the 1st day (08 March 2021) and the control (N=25 fish) and dummy (N=20 fish) two weeks later (21 March 2021). Different letters indicate statistically significant differences between the groups (P< 0.05).
Plasma lactate concentrations (mean ± SEM), in contrast, present statistically significant differences, with the control group of the 1st day showing lower concentrations than the 14th day and the control and dummy groups (2.6 ± 0.2 mmol l-1, 5.8 ± 0.5 mmol l-1, and 4.6 ± 0.5 mmol l-1, respectively) (F2,36 = 26.0, P = 0.35, a< 0.001, Figure 3). There was no significant difference between the control and treated group on the 14th day of the trial however.
Plasma cortisol concentrations (mean ± SEM) were elevated in all groups (776.1 ± 30.8 ng ml-1, 712.6 ± 58.1 ng ml-1, and 799.3 ± 42.5 ng ml-1 for the control on the 1st and 14th day and the dummy on the 14th day, respectively) with no statistically significant differences (F2,35 = 0.96, P = 0.3, a = 0.05, Figure 3).
Hematocrit mean ± SEM values were 43.0 ± 1.3%, 49.0 ± 1.2%, and 44.1 ± 1.8% for the control on day 1 and day 14 and the dummy on the 14th day, respectively, with the control group on the 14th day presenting statistically significant higher values than the 1st day (F2,34 = 4.2, P = 0.02, a = 0.05, Figure 4).
Figure 4 Hematologic indicators (mean ± SEM). (A) Hematocrit and (B) hemoglobin concentrations of the control group on the 1st day (08 March 2021) and the control (N=25 fish) and dummy (N=20 fish) two weeks later (21 March 2021). Different letters indicate statistically significant differences between the groups (P< 0.05).
Hemoglobin concentrations (mean ± SEM) did not present statistically significant changes between the groups (6.9 ± 0.1 g dL-1, 7.5 ± 0.5 g dL-1, and 6.8 ± 0.4 g dL-1 for the control of the 1st and 14th day and the dummy of the 14th day, respectively; H2 = 1.0, P = 0.6, a = 0.05, Figure 4).
Both treated groups, i.e. dummy and the active, were significantly more cohesive than the control group on the third day post-surgery as they covered only 10 and 15% of the total tank area, respectively in contrast with the 20–30% coverage of the control group (χ2 statistic: 43.4, p-value< 0.001; Dummy p-value< 0.001; Active p-value< 0.001; Figure 5). The treated groups also statistically differed among themselves, with the dummy group being significantly more cohesive than the active group (p-value = 0.004). The cohesion decreased 11 days post-surgery for all the groups, but the differences between the control group and the treated group persisted (χ2 statistic: 42.2, p-value< 0.001; Dummy p-value< 0.001; Active p-value< 0.001) while the treated groups had the same cohesion (p-value = 0.6). The cohesion further decreased 14 days post-surgery for the treated groups. For the control group, however, the cohesion decreased between day 3 and day 11 while a slight, but significant, increase was observed between days 11 and 14. The differences between the control and the treated groups also persisted (χ2 statistic: 43.3, p-value< 0.001; Dummy p-value< 0.001; Active p-value< 0.001). In addition, both treated groups increased the area they covered fourteen days after the surgery (from ~10% to 20% for the dummy group and from ~15% to 28% for the active group) and remained similarly cohesive (p-value = 0.2).
Figure 5 Boxplots showing the group cohesion expressed as the relative area covered (in percent) by the control (N=25 fish), dummy (N=20 fish), and active group (N=24 fish), on the 3rd, the 11th, and the 14th day of the experiment. Black horizontal lines indicate the median values. Lower values indicate a higher cohesion. Statistically significant differences between treatments (groups) on each sampling (day) are indicated with different letters and between samplings for each treatment with different numbers.
The exploratory tendency of the tagged groups was initially very limited as the fish stayed in one of the tank’s corners, staying away from areas where there was the possibility of human presence (Figure 6). As fish were very cohesive at the beginning of the experiment, the distance between the group’s center and the center of the tank differed significantly, and the limited group exploratory tendency is represented by the asymmetry in the heatmap of Figure 6. As time progressed and the group cohesion decreased (see Figure 5), the group started covering the whole tank, and the group center did not differ from the center of the tank (the peak at the center of the two-dimensional distribution is at around (0, 0) distance from the tank center, Figure 6). In the first days after treatment, the dummy group showed a preference toward the left corner of the tank while the active group showed a preference for the right side. In contrast, the control group explored the whole tank from the first days of the experiment. Gradually, the exploratory tendency recovered for both the dummy and active groups, and in contrast to group cohesion, fish showed a recovery in their exploratory tendency 11 days after implantation. Two weeks after the surgery, fish explored all the tank sites equally.
Figure 6 Heatmaps showing the distance of the group center from the center of the tank for the control group (top row, light green, N=25), the dummy group (middle row, dark green, N=20), and the active group (bottom row, N=24) for the 3rd, the 11th, and the 14th day of the experiment. The intensity of the color indicates higher number of occurrences.
The tagged fish (both with dummy and active tags) showed a strong polarization initially, i.e. three days after surgery, reaching up to 0.9 correlation in their direction (Polcontrol= 0.5 ±0.2, Poldummy= 0.6 ±0.2, Polactive= 0.6 ±0.2, Figure 6). Eleven days after the surgery, polarization decreased, and the treated groups reached the same polarization level, which was also significantly different from the control group (χ2=72.4, p−value<0.001 ). The polarization decreased further fourteen days after surgery (Polcontrol= 0.3 ±0.2, Poldummy= 0.41 ±0.2, Polactive= 0.3 ±0.2 ), remaining significantly different between the control and the treated groups (χ2=17.4, p−value<0.001 ). Three days after surgery, the average speed did not differ significantly between groups Figure 7). Eleven days after the surgery, all groups showed a decrease in speed without significant differences between the groups (χ2=3.3, p−value<0.19 ). In contrast, group speed decreased and differed between the control and the treated groups fourteen days post-surgery, indicating a delayed response to the implantation procedure . Between the treated groups, the active group was slower.
Figure 7 (A) Two-dimensional density plots showing the covariance of the two swimming performance variables, i.e. the average speed and the group polarization, for the different tested groups, i.e. for the control group (a, d, g), the dummy group (b, e, h), and the active group (c, f, i), and for three different days post-surgery, i.e. day 3 (a, b, c), day 11 (d, e, f), and day 14 (g, h, i). (B) Violin plots of the group polarization (a) and the group speed (b) for the different treatments (the dummy, the active group, and the control group) and for the three different days. Statistically significant differences between treatments (groups) on each sampling (day) are indicated with different letters and between samplings for each treatment with different numbers.
In the current work, the short-term effects of acoustic tag implantation on the group-level swimming performance of European seabass were presented using dummy and active transmitters. The group-level swimming performance attributes studied here were affected differently by the implantation and showed distinct recovery responses, enhancing our initial hypothesis and the previous findings (Ginnaw et al., 2020) that the effect of stressors, in our case tag implantation, on different behavioral attributes is variable. Studies that will test the effect of tagging on multiple behavioral and swimming performance attributes and will define recovery times should be, therefore, carried out for a wide range of different species. These studies could be used as reference for future experiments studying the swimming patterns of tagged individuals and could help to infer correct conclusions on the behavior of the population. None of the physiological parameters differed significantly between the control and the dummy group fourteen days after surgery, indicating that any detected difference in the swimming performance of the group should not be attributed to acute stress levels.
The elevated cortisol concentrations can be explained by the sampling procedure, the decreased water volume in the tank during fish capturing (and the time needed to decrease the volume), netting, air exposure, and the transfer of each fish into a bucket for complete anesthesia. European seabass is known to be highly responsive to acute stress compared to other species (Fanouraki et al., 2011), and the maximum cortisol values are observed 30–60 min after the stressor. It is quite probable that due to the sampling procedure and its duration the cortisol concentrations were “saturated”, i.e., close to the maximum possible. The “saturation” in the cortisol values may mask potential differences in basal cortisol level between the control and the dummy group, so further studies are required to examine differences in the physiological parameters. Similar studies on the effect of tagging on the physiological responses of the European seabass and the gilthead seabream found no difference in the physiological levels between the first and the ninth day in the control and dummy groups (Montoya et al., 2012; Alfonso et al., 2020b). In addition, other studies on different species reported no differences in the physiological parameters tested between the control and the tagged fish (Moore et al., 1990; Loher and Rensmeyer, 2011).
Group cohesion was negatively impacted i.e. the treated groups remained more cohesive than the control group for the duration of the experiment and did not recover 14 days post-surgery. Preliminary studies for longer time periods showed that cohesion remains low even 20 days after the surgery (our unpublished data). Previous studies on the effect of different stressors on different behavioral characteristics demonstrated that group cohesion can be significantly affected, i.e. increased (Neo et al., 2018; Kleinhappel et al., 2019; Cerqueira et al., 2021) or decreased (Sadoul et al., 2017; Hayes et al., 2019; Alfonso et al., 2020a) depending on the applied stressor. The increased shoal cohesion measured in the current study can act, therefore, as a response mechanism to stress caused by the tagging procedure (Martins et al., 2012). Although other stressors related to the husbandry practice during the trial (oxygen concentration, for example) may have had an additional effect, the actual conditions of the rearing that were similar for all the groups (DO always above 60% of saturation, low stocking density of fish, etc.) did not allow the assumption that changes in cohesion were related to any other additional stressor apart from the actual tagging procedure. During the experiment, groups became less cohesive as time progressed, and the effect size was smaller between the treatments. Therefore, longer studies are required to examine if a further decrease will lead to the minimization of the effect or if the effect of the treatment on group cohesion will persist.
In contrast with cohesion, group exploratory tendency was initially affected by the tagging process, but it gradually recovered, i.e. two weeks after the surgery, the fish were visiting all tank sites. To our knowledge, there are no analogous telemetry studies on the effect of tag implantation on exploratory behavior. However, in acute stressor studies, changes in the exploratory behavior of fish showed that the exploratory tendency increased under the application of the stressors, in contrast with our finding (Bégout Anras and Lagardère, 2004; Archard et al., 2012). On the other hand, Cerqueira et al. (2021) showed that other stressors, such as confinement, decreased the exploratory tendency of the group and it is therefore reasonable to conclude that the effect on the exploratory tendency depends on the applied stressor.
Group speed was not affected by the tag implantation initially as there were no significant differences in the speed between the control and treated group but showed a significant decrease in comparison with the control group, fourteen days post-surgery, in the dummy and the active group. This delayed response may be indicative of a chronic stress response. Most studies that test for the effect of tag implantation on fish speed use different species (such as the Atlantic salmon and the gilthead seabream), different experimental durations, and an implicit measure of group speed such as activity and the critical swimming speed. Although these studies are not easily comparable with the current one, they are discussed for a better understanding of the differences observed. Thus, in a study of Montoya et al. (2012), the locomotor activity of gilthead seabream was used as an implicit measure of fish speed to test for the effect of tag implantation, and there were no statistically significant differences with respect to baseline activity levels observed during the week prior to surgery, a result that enhances our findings too. Other studies on the effects of tag implantation on the swimming performance focus on the critical speed of fish (Moore et al., 1990; Lacroix et al., 2004; Brown et al., 2006; Walker et al., 2016; Alfonso et al., 2022), an individual measure of metabolic performance that cannot be easily compared to the group swimming speed studied here. However, some of these studies found no significant differences between the control and the treated group (Moore et al., 1990; Brown et al., 2006; Alfonso et al., 2022), while others found a lower critical swimming speed of the tagged individuals that recovered 7 days after surgery (Lacroix et al., 2004). The variation in responses could be attributed to the species-specific response of the tagging procedure.
All groups in this study were initially synchronized in motion (i.e., they showed high polarization) and their polarization decreased gradually. The dummy group remained significantly more polarized two weeks after surgery, while the active group was less polarized than the control group. In both trials, the effect of tagging on polarization decreased over time but persisted even 20 days after the surgery (unpublished data). There are few studies on the effect of tag implantation on group polarization. Studies on the effect of other stressors on the shoal performance of model collective species (such as the three-spined stickleback fish, Gasterosteus aculeatus, and the Eurasian minnow, Phoxinus phoxinus) suggest that fish tend to move in higher synchrony after a stressor is applied regardless of the stressor and time scale. For example, Short et al. (2020) showed that the fish were significantly more aligned for 3 min after a sound stressor was turned on, and the difference between treatment and control was greatest during the first 20 s of the application of the stressor.
Consistent differences between the control and treated groups were found for all swimming performance attributes at the beginning of the experiment, except for group swimming speed. Group speed seemed to be unaffected by the surgery at the beginning of the experiment and remained at similar levels for the control and the treated groups during the whole experimental period for the dummy treatment. In contrast, for fish with active tags, speed was significantly different as time progressed, leading to significantly lower speeds than the control group. As demonstrated earlier, there is a statistically significant difference in the size between the group tagged with the active transmitters and the other two groups. It would be therefore reasonable to assume that the increased size of the fish in the active group could explain the decreased group speed in the active group. However, if this difference would be attributed to the size difference, we would expect it to appear from the beginning of the experiment. In contrast we showed that the speed did not differ initially. In addition to that, previous studies on body size-speed relationship (e.g., Bellwood and Fisher, 2001; Cano-Barbacil et al., 2020) suggest that we do not expect significant variations in the speed for a size difference of 2-3 cm. Another possible explanation for the inconsistency seen in the responses between the treated groups could be the expected group-level variation that emerges from the individual-level variability (MacGregor and Ioannou, 2021) as the results presented here refer to the response of one group of fish and are not averages of multiple groups. As group-level behavioral patterns can emerge from the characteristics of individuals within groups, as predicted by theory and experimental results (Romey, 1996; Couzin et al., 2002; Farine et al., 2017; Jolles et al., 2017), consistent behavioral differences between groups should emerge (Jolles et al., 2018) that could also partially explain the differences seen in the current study.
Acoustic telemetry has proven to be a very useful tool that enables scientists to study animal movement and interactions at a spatio-tempotal resolution that was almost impossible to achieve previously. The accumulated knowledge on fish movement and fish interactions can also provide a large database for aquaculture that could be used to classify movement patterns under different contexts, determine baseline behaviors, detect fish welfare indicators, and quantify their optimal ranges. These steps can then help improve management plans for fish in the wild and for aquaculture. The responsibility for better management and the improvement of fish welfare requires the assurance that tagging fish with acoustic transmitters has a minimal effect on the swimming performance attributes of interest. The current study showed that the effects can vary depending on the performance attribute studied, and that European seabass groups need more than 14 days after surgery to recover most of their performance attributes. Longer studies with multiple groups and different performance attributes are required to better estimate the recovery times.
The raw data supporting the conclusions of this article will be made available by the authors, without undue reservation.
The animal study was reviewed and approved by the Ethics Committee of the IMBBC and the relevant veterinary authorities (Ref Number 32257 09-02-2021).
NP and DG conceived and designed the study. NP, NM, DG and DV run the experiments. EF took the blood samples and analyzed the hormonal data. DG developed the methodology to track and analyze the fish movement behavior, analyzed the swimming behavior data, produced the figures, and wrote the first draft of the manuscript. All authors contributed to the final version of the manuscript.
This work was partially funded by the EU Horizon 2020 iFishIENCi project (818036).
The authors would like to thank Marlene deWilde for proofreading the manuscript and improving its appearance, and the reviewers for their constructive comments.
The authors declare that the research was conducted in the absence of any commercial or financial relationships that could be construed as a as a potential conflict of interest.
All claims expressed in this article are solely those of the authors and do not necessarily represent those of their affiliated organizations, or those of the publisher, the editors and the reviewers. Any product that may be evaluated in this article, or claim that may be made by its manufacturer, is not guaranteed or endorsed by the publisher.
Abecasis D., Steckenreuter A., Reubens J., Aarestrup K., Alós J., Badalamenti F., et al. (2018). A review of acoustic telemetry in Europe and the need for a regional aquatic telemetry network. Anim. Biotelemetry 6. doi: 10.1186/s40317-018-0156-0
Adams N. S., Rondorf D. W., Evans S. D., Kelly J. E., Perry R. W. (1998). Effects of surgically and gastrically implanted radio transmitters on swimming performance and predator avoidance of juvenile chinook salmon (Oncorhynchus tshawytscha). Can. J. Fisheries Aquat. Sci. 55, 781–787. doi: 10.1139/f97-285
Alfonso S., Sadoul B., Cousin X., Bégout M. L. (2020a). Spatial distribution and activity patterns as welfare indicators in response to water quality changes in European sea bass, dicentrarchus labrax. Appl. Anim. Behav. Sci. 226, 104974. doi: 10.1016/j.applanim.2020.104974
Alfonso S., Zupa W., Manfrin A., Fiocchi E., Dioguardi M., Dara M., et al. (2020b). Surgical implantation of electronic tags does not induce medium-term effect: Insights from growth and stress physiological profile in two marine fish species. Anim. Biotelemetry 8. doi: 10.1186/s40317-020-00208-w
Alfonso S., Zupa W., Spedicato M. T., Lembo G., Carbonara P. (2021a). Mapping the energetic costs of free-swimming gilthead Sea bream (Sparus aurata), a key species in European marine aquaculture. Biol. (Basel) 10, 1357. doi: 10.3390/biology10121357
Alfonso S., Zupa W., Spedicato M. T., Lembo G., Carbonara P. (2021b). “Use of telemetry sensors as a tool for health/welfare monitoring of European sea bass (Dicentrarchus labrax) in aquaculture,” in 2021 IEEE international workshop on metrology for the Sea: Learning to measure Sea health parameters, MetroSea 2021 - proceedings (Institute of Electrical and Electronics Engineers Inc), 262–267. doi: 10.1109/MetroSea52177.2021.9611579
Alfonso S., Zupa W., Spedicato M. T., Lembo G., Carbonara P. (2022). Using telemetry sensors mapping the energetic costs in European Sea bass (Dicentrarchus labrax), as a tool for welfare remote monitoring in aquaculture. Front. Anim. Sci. 3. doi: 10.3389/fanim.2022.885850
Ammann A. J., Michel C. J., MacFarlane R. B. (2013). The effects of surgically implanted acoustic transmitters on laboratory growth, survival and tag retention in hatchery yearling Chinook salmon. Environ. Biol. Fishes 96, 135–143. doi: 10.1007/s10641-011-9941-9
Anras M.-L. B., Lagardère J.-P. (1998). Weather related variability. consequences on the swimming activity of a marine fish. Comptes Rendus l’Academie Des. Sci. Ser. III Sci. la Vie 321, 641–648. doi: 10.1016/S0764-4469(98)80003-6
Anras M.-L. B., Lagardère J. P., Lafaye J.-Y. (1997). Diel activity rhythm of seabass tracked in a natural environment: group effects on swimming patterns and amplitudes 54 (1), 162–168. doi: 10.1139/f96-253
Archard G. A., Earley R. L., Hanninen A. F., Braithwaite V. A. (2012). Correlated behaviour and stress physiology in fish exposed to different levels of predation pressure. Funct. Ecol. 26, 637–645. doi: 10.1111/j.1365-2435.2012.01968.x
Arechavala-Lopez P., Lankheet M. J., Díaz-Gil C., Abbink W., Palstra A. P. (2021). Swimming activity of gilthead seabream (Sparus aurata) in swim-tunnels: Accelerations, oxygen consumption and body motion. Front. Anim. Sci. 2. doi: 10.3389/fanim.2021.679848
Arechavala-Lopez P., Uglem I., Fernandez-Jover D., Bayle-Sempere J. T., Sanchez-Jerez P. (2012). Post-escape dispersion of farmed seabream (Sparus aurata l.) and recaptures by local fisheries in the Western Mediterranean Sea. Fish Res. 121–122, 126–135. doi: 10.1016/j.fishres.2012.02.003
Barkley A. N., Broell F., Pettitt-Wade H., Watanabe Y. Y., Marcoux M., Hussey N. E. (2020). A framework to estimate the likelihood of species interactions and behavioural responses using animal-borne acoustic telemetry transceivers and accelerometers. J. Anim. Ecol. 89, 146–160. doi: 10.1111/1365-2656.13156
Barreto M. O., Rey Planellas S., Yang Y., Phillips C., Descovich K. (2022). Emerging indicators of fish welfare in aquaculture. Rev. Aquac 14, 343–361. doi: 10.1111/raq.12601
Barton B. A. (2002). Stress in fishes: A diversity of responses with particular reference to changes in circulating corticosteroids. Integr. Comp. Biol. 42, 517–525. doi: 10.1093/icb/42.3.517
Bégout Anras M. L., Covès D., Dutto G., Laffargue P., Lagardère F. (2003). Tagging juvenile seabass and sole with telemetry transmitters: Medium-term effects on growth. ICES J. Mar. Sci. 60, 1328–1334. doi: 10.1016/S1054-3139(03)00135-8
Bégout Anras M. L., Lagardère J. P. (2004). Measuring cultured fish swimming behaviour: First results on rainbow trout using acoustic telemetry in tanks. Aquaculture 240, 175–186. doi: 10.1016/j.aquaculture.2004.02.019
Begout M.-L., Lagardere J.-P. (1995). An acoustic telemetry study of seabream (Sparus aurata l.): First results on activity rhythm, effects of environmental variables and space utilization. Hydrobiologia 300–301, 417–423. doi: 10.1007/BF00024483
Bellwood D., Fisher R. (2001). Relative swimming speeds in reef fish larvae. Mar. Ecol. Prog. Ser. 211, 299–303. doi: 10.3354/meps211299
Bridger C. J., Booth R. K. (2003). The effects of biotelemetry transmitter presence and attachment procedures on fish physiology and behavior. Rev. Fisheries Sci. 11, 13–34. doi: 10.1080/16226510390856510
Brown R. S., Geist D. R., Deters K. A., Grassell A. (2006). Effects of surgically implanted acoustic transmitters >2% of body mass on the swimming performance, survival and growth of juvenile sockeye and Chinook salmon. J. Fish Biol. 69, 1626–1638. doi: 10.1111/j.1095-8649.2006.01227.x
Brownscombe J. W., Lédée E. J. I., Raby G. D., Struthers D. P., Gutowsky L. F. G., Nguyen V. M., et al. (2019). Conducting and interpreting fish telemetry studies: Considerations for researchers and resource managers. Rev. Fish Biol. Fish 29, 369–400. doi: 10.1007/s11160-019-09560-4
Cano-Barbacil C., Radinger J., Argudo M., Rubio-Gracia F., Vila-Gispert A., García-Berthou E. (2020). Key factors explaining critical swimming speed in freshwater fish: A review and statistical analysis for Iberian species. Sci. Rep. 10, 18947. doi: 10.1038/s41598-020-75974-x
Carbonara P., Alfonso S., Dioguardi M., Zupa W., Vazzana M., Dara M., et al. (2021). Calibrating accelerometer data, as a promising tool for health and welfare monitoring in aquaculture: Case study in European sea bass (Dicentrarchus labrax) in conventional or organic aquaculture. Aquac Rep. 21, 100817. doi: 10.1016/j.aqrep.2021.100817
Carbonara P., Alfonso S., Gai F., Gasco L., Palmegiano G., Spedicato M. T., et al. (2020). Moderate stocking density does not influence the behavioural and physiological responses of rainbow trout ( Oncorhynchus mykiss ) in organic aquaculture. Aquac Res. 51, 3007–3016. doi: 10.1111/are.14640
Carbonara P., Alfonso S., Zupa W., Manfrin A., Fiocchi E., Pretto T., et al. (2019). Behavioral and physiological responses to stocking density in sea bream (Sparus aurata): Do coping styles matter? Physiol. Behav. 212, 112698. doi: 10.1016/j.physbeh.2019.112698
Cerqueira M., Millot S., Silva T., Félix A. S., Castanheira M. F., Rey S., et al. (2021). Stressor controllability modulates the stress response in fish. BMC Neurosci. 22, 48. doi: 10.1186/s12868-021-00653-0
Childs A. R., Næsje T. F., Cowley P. D. (2011). Long-term effects of different-sized surgically implanted acoustic transmitters on the sciaenid arygyrosomus japonicus: Breaking the 2% tag-to-body mass rule. Mar. Freshw. Res. 62, 432–438. doi: 10.1071/MF10219
Cooke S. J., Midwood J. D., Thiem J. D., Klimley P., Lucas M. C., Thorstad E. B., et al. (2013). Tracking animals in freshwater with electronic tags: Past, present and future. Anim. Biotelemetry 1, 5. doi: 10.1186/2050-3385-1-5
Cooke S. J., Woodley C. M., Brad Eppard M., Brown R. S., Nielsen J. L. (2011). Advancing the surgical implantation of electronic tags in fish: A gap analysis and research agenda based on a review of trends in intracoelomic tagging effects studies. Rev. Fish Biol. Fish 21, 127–151. doi: 10.1007/s11160-010-9193-3
Couzin I. D., Krause J., James R., Ruxton G. D., Franks N. R. (2002). Collective memory and spatial sorting in animal groups. J. Theor. Biol. 218, 1–11. doi: 10.1006/jtbi.2002.3065
Daniels J., Brunsdon E. B., Chaput G., Dixon H. J., Labadie H., Carr J. W. (2021). Quantifying the effects of post-surgery recovery time on the migration dynamics and survival rates in the wild of acoustically tagged Atlantic salmon salmo salar smolts. Anim. Biotelemetry 9. doi: 10.1186/s40317-020-00228-6
Espinoza T., Burke C., Carpenter-Bundhoo L., Marshall S., Roberts D., Kennard M. (2020). Fine-scale acoustic telemetry in a riverine environment: Movement and habitat use of the endangered Mary river cod maccullochella mariensis. Endanger Species Res. 42, 125–131. doi: 10.3354/esr01046
Espinoza M., Lédée E. J. I., Smoothey A. F., Heupel M. R., Peddemors V. M., Tobin A. J., et al. (2021). Intra-specific variation in movement and habitat connectivity of a mobile predator revealed by acoustic telemetry and network analyses. Mar. Biol. 168. doi: 10.1007/s00227-021-03886-z
Føre M., Alfredsen J. A., Gronningsater A. (2011). Development of two telemetry-based systems for monitoring the feeding behaviour of Atlantic salmon (Salmo salar l.) in aquaculture sea-cages. Comput. Electron Agric. 76, 240–251. doi: 10.1016/j.compag.2011.02.003
Føre M., Frank K., Dempster T., Alfredsen J. A., Høy E. (2017). Biomonitoring using tagged sentinel fish and acoustic telemetry in commercial salmon aquaculture: A feasibility study. Aquac Eng. 78, 163–172. doi: 10.1016/j.aquaeng.2017.07.004
Føre M., Svendsen E., Alfredsen J. A., Uglem I., Bloecher N., Sveier H., et al. (2018). Using acoustic telemetry to monitor the effects of crowding and delousing procedures on farmed Atlantic salmon (Salmo salar). Aquaculture 495, 757–765. doi: 10.1016/j.aquaculture.2018.06.060
Føre M., Svendsen E., Økland F., Gräns A., Alfredsen J. A., Finstad B., et al. (2021). Heart rate and swimming activity as indicators of post-surgical recovery time of Atlantic salmon (Salmo salar). Animal Biotelemetry 9 (1), 1–13.
Fanouraki E., Mylonas C. C., Papandroulakis N., Pavlidis M. (2011). Species specificity in the magnitude and duration of the acute stress response in Mediterranean marine fish in culture. Gen. Comp. Endocrinol. 173, 313–322. doi: 10.1016/j.ygcen.2011.06.004
Farine D. R., Strandburg-Peshkin A., Couzin I. D., Berger-Wolf T. Y., Crofoot M. C. (2017). Individual variation in local interaction rules can explain emergent patterns of spatial organization in wild baboons. Proc. R. Soc. B: Biol. Sci. 284, 20162243. doi: 10.1098/rspb.2016.2243
Farnebäck G. (2003). “Two-frame motion estimation based on polynomial expansion,” in Image analysis. Eds. Bigun J., Gustavsson T. (Berlin, Heidelberg: Springer Berlin Heidelberg), 363–370.
Frank H. J., Mather M. E., Smith J. M., Muth R. M., Finn J. T., McCormick S. D. (2009). What is “fallback”?: Metrics needed to assess telemetry tag effects on anadromous fish behavior. Hydrobiologia 635, 237–249. doi: 10.1007/s10750-009-9917-3
Ginnaw G. M., Davidson I. K., Harding H. R., Simpson S. D., Roberts N. W., Radford A. N., et al. (2020). Effects of multiple stressors on fish shoal collective motion are independent and vary with shoaling metric. Anim. Behav. 168, 7–17. doi: 10.1016/j.anbehav.2020.07.024
Gruen A., Huang T. S. (2001). Calibration and orientation of cameras in computer vision (Springer Berlin Heidelberg: Springer Science & Business Media).
Hartley R., Zisserman A. (2004). Multiple view geometry in computer vision (Cambridge: Cambridge University Press). doi: 10.1017/CBO9780511811685
Hayes D. S., Branco P., Santos J. M., Ferreira T. (2019). Oxygen depletion affects kinematics and shoaling cohesion of cyprinid fish. Water (Basel) 11, 642. doi: 10.3390/w11040642
Hussey N. E., Kessel S. T., Aarestrup K., Cooke S. J., Cowley P. D., Fisk A. T., et al. (2015). Aquatic animal telemetry: A panoramic window into the underwater world. Sci. (1979) 348, 1255642. doi: 10.1126/science.1255642
Huusko R., Huusko A., Mäki-Petäys A., Orell P., Erkinaro J. (2016). Effects of tagging on migration behaviour, survival and growth of hatchery-reared Atlantic salmon smolts. Fish Manag Ecol. 23, 367–375. doi: 10.1111/fme.12180
Jepsen N., Koed A., Thorstad E. B., Baras E. (2002). “Surgical implantation of telemetry transmitters in fish: How much have we learned?,” in Aquatic telemetry (Dordrecht: Springer Netherlands), 239–248. doi: 10.1007/978-94-017-0771-8_28
Jepsen N., Schreck C. B., Clements S., Thorstad E. B. (2005). “A brief discussion on the 2% tag/bodymass rule of thumb,” in Aquatic telemetry: advances and applications. Eds. Spedicato M. T., Lembo G., Marmulla G. (Italy: Food and Agriculture Organization of the United Nations), 255–259.
Jolles J. W., Boogert N. J., Sridhar V. H., Couzin I. D., Manica A. (2017). Consistent individual differences drive collective behavior and group functioning of schooling fish. Curr. Biol. 27, 2862–2868.e7. doi: 10.1016/j.cub.2017.08.004
Jolles J. W., Laskowski K. L., Boogert N. J., Manica A. (2018). Repeatable group differences in the collective behaviour of stickleback shoals across ecological contexts. Proc. R. Soc. B: Biol. Sci. 285. doi: 10.1098/rspb.2017.2629
Kleinhappel T. K., Pike T. W., Burman O. H. P. (2019). Stress-induced changes in group behaviour. Sci. Rep. 9, 17200. doi: 10.1038/s41598-019-53661-w
Klinard N., Halfyard E. A., Fisk A. T., Stewart T. J., Johnson T. B. (2018). Effects of surgically implanted acoustic tags on body condition, growth, and survival in a small, laterally compressed forage fish. Trans. Am. Fish Soc. 147, 749–757. doi: 10.1002/tafs.10064
Klinard N. V., Matley J. K. (2020). Living until proven dead: Addressing mortality in acoustic telemetry research. Rev. Fish Biol. Fish 30, 485–499. doi: 10.1007/s11160-020-09613-z
Koed A., Thorstad E. B. (2001). Long-term effect of radio-tagging on the swimming performance of pikeperch. J. Fish Biol. 58, 1753–1756. doi: 10.1111/j.1095-8649.2001.tb02329.x
Lacroix G. L., Knox D., McCurdy P. (2004). Effects of implanted dummy acoustic transmitters on juvenile Atlantic salmon. Trans. Am. Fish Soc. 133, 211–220. doi: 10.1577/t03-071
Lees K., Mill A., Skerritt D., Robertson P., Fitzsimmons C. (2020). Spatial overlap, proximity, and interaction between lobsters revealed using acoustic telemetry. Mar. Ecol. Prog. Ser. 645, 109–124. doi: 10.3354/meps13376
Lippi D., Coxey M., Rooker J., Rezende S., Dance M., Gaspar A., et al. (2022). Use of acoustic telemetry to evaluate fish movement, habitat use, and protection effectiveness of a coral reef no-take zone (NTZ) in Brazil. Mar. Ecol. Prog. Ser. 688, 113–131. doi: 10.3354/meps14020
Loher T., Rensmeyer R. (2011). Physiological responses of pacific halibut, hippoglossus stenolepis, to intracoelomic implantation of electronic archival tags, with a review of tag implantation techniques employed in flatfishes. Rev. Fish Biol. Fish 21, 97–115. doi: 10.1007/s11160-010-9192-4
Lucas B. D., Kanade T. (1981). “An iterative image registration technique with an application to stereo vision,” in Proceedings of the 7th international joint conference on artificial intelligence - volume 2 IJCAI’81 (San Francisco, CA, USA: Morgan Kaufmann Publishers Inc), 674–679. Available at: http://dl.acm.org/citation.cfm?id=1623264.1623280.
MacGregor H. E. A., Ioannou C. C. (2021). Collective motion diminishes, but variation between groups emerges, through time in fish shoals. R Soc. Open Sci. 8, 210655. doi: 10.1098/rsos.210655
Marques J. P., Almeida P. R., Moreira P., Reis-Santos P., Prista N., Costa J. L., et al. (2022). “Acoustic telemetry unravels movements and habitat use patterns of juvenile meagre (A. regius) in the tagus estuary,” in SIBIC 2022 (Basel Switzerland: MDPI), 64. doi: 10.3390/blsf2022013064
Martins C. I. M., Galhardo L., Noble C., Damsgård B., Spedicato M. T., Zupa W., et al. (2012). Behavioural indicators of welfare in farmed fish. Fish Physiol. Biochem. 38, 17–41. doi: 10.1007/s10695-011-9518-8
McKenna J. E., Sethi S. A., Scholten G. M., Kraus J., Chalupnicki M. (2021). Acoustic tag retention and tagging mortality of juvenile cisco coregonus artedi. J. Great Lakes Res. 47, 937–942. doi: 10.1016/j.jglr.2021.03.020
Meager J. J., Skjæraasen J. E., Fernö A., Karlsen Ø., Løkkeborg S., Michalsen K., et al. (2009). Vertical dynamics and reproductive behaviour of farmed and wild Atlantic cod gadus morhua. Mar. Ecol. Prog. Ser. 389, 233–243. doi: 10.3354/meps08156
Miller E. A., Froehlich H. E., Cocherell D. E., Thomas M. J., Cech J. J., Klimley A. P., et al. (2014). Effects of acoustic tagging on juvenile green sturgeon incision healing, swimming performance, and growth. Environ. Biol. Fishes 97, 647–658. doi: 10.1007/s10641-013-0167-x
Montoya A., López-Olmeda J. F., Lopez-Capel A., Sánchez-Vázquez F. J., Pérez-Ruzafa A. (2012). Impact of a telemetry-transmitter implant on daily behavioral rhythms and physiological stress indicators in gilthead seabream (Sparus aurata). Mar. Environ. Res. 79, 48–54. doi: 10.1016/j.marenvres.2012.05.002
Moore A., Russell I. C., Potter E. C. E. (1990). The effects of intraperitoneally implanted dummy acoustic transmitters on the behaviour and physiology of juvenile Atlantic salmon, salmo salar l. J. Fish Biol. 37, 713–721. doi: 10.1111/j.1095-8649.1990.tb02535.x
Morris W. A., Follmann E. H., George J. C., O’hara T. (2000). “Surgical implantation of radio transmitters in Arctic broad whitefish in Alaska,” in Biotelemetry 15: proceedings of the 15th international symposium on biotelemetry (The Netherlands: International Society on Biotelemetry, Wageningen), 193–201.
Muñoz L., Aspillaga E., Palmer M., Saraiva J. L., Arechavala-Lopez P. (2020). Acoustic telemetry: A tool to monitor fish swimming behavior in Sea-cage aquaculture. Front. Mar. Sci. 7. doi: 10.3389/fmars.2020.00645
Neo Y. Y., Hubert J., Bolle L. J., Winter H. V., Slabbekoorn H. (2018). European Seabass respond more strongly to noise exposure at night and habituate over repeated trials of sound exposure. Environ. Pollut. 239, 367–374. doi: 10.1016/j.envpol.2018.04.018
Neves V., Silva D., Martinho F., Antunes C., Ramos S., Freitas V. (2018). Assessing the effects of internal and external acoustic tagging methods on European flounder platichthys flesus. Fish Res. 206, 202–208. doi: 10.1016/j.fishres.2018.05.015
Newton M., Barry J., Dodd J. A., Lucas M. C., Boylan P., Adams C. E. (2016). Does size matter? a test of size-specific mortality in Atlantic salmon salmo salar smolts tagged with acoustic transmitters. J. Fish Biol. 89, 1641–1650. doi: 10.1111/jfb.13066
Paukert C. P., Chvala P. J., Heikes B. L., Brown M. L. (2001). Effects of implanted transmitter size and surgery on survival, growth, and wound healing of bluegill. Trans. Am. Fish Soc. 130, 975–980. doi: 10.1577/1548-8659(2001)130<0975:eoitsa>2.0.co;2
Perry R. W., Plumb J. M., Fielding S. D., Adams N. S., Rondorf D. W. (2013). Comparing effects of transmitters within and among populations: Application to swimming performance of juvenile Chinook salmon. Trans. Am. Fish Soc. 142, 901–911. doi: 10.1080/00028487.2013.788556
R Core Team (2018) R: A language and environment for statistical computing. Available at: https://www.R-project.org/.
Romey W. L. (1996). Individual differences make a difference in the trajectories of simulated schools of fish. Ecol. Modell 92, 65–77. doi: 10.1016/0304-3800(95)00202-2
Runde B. J., Buckel J. A., Bacheler N. M., Tharp R. M., Rudershausen P. J., Harms C. A., et al. (2022). Evaluation of six methods for external attachment of electronic tags to fish: Assessment of tag retention, growth and fish welfare. J. Fish Biol. doi: 10.1111/jfb.14989
Sadoul B., Friggens N. C., Valotaire C., Labbé L., Colson V., Prunet P., et al. (2017). Physiological and behavioral flexibility to an acute CO2 challenge, within and between genotypes in rainbow trout. Comp. Biochem. Physiol. A Mol. Integr. Physiol. 209, 25–33. doi: 10.1016/j.cbpa.2017.04.002
Schreck C. B., Tort L. (2016). The concept of stress in fish 35, 1–34. doi: 10.1016/B978-0-12-802728-8.00001-1
Schwinghamer C. W., Tripp S., Phelps Q. E. (2019). Using ultrasonic telemetry to evaluate paddlefish spawning behavior in harry s. Truman reservoir, Missouri. N Am. J. Fish Manag 39, 231–239. doi: 10.1002/nafm.10263
Short M., White P. R., Leighton T. G., Kemp P. S. (2020). Influence of acoustics on the collective behaviour of a shoaling freshwater fish. Freshw. Biol. 65, 2186–2195. doi: 10.1111/fwb.13612
Skomal G. B., Mandelman J. W. (2012). The physiological response to anthropogenic stressors in marine elasmobranch fishes: A review with a focus on the secondary response. Comp. Biochem. Physiol. A Mol. Integr. Physiol. 162, 146–155. doi: 10.1016/j.cbpa.2011.10.002
Smukall M. J., Kessel S. T., Franks B. R., Feldheim K. A., Guttridge T. L., Gruber S. H. (2019). No apparent negative tagging effects after 13 years at liberty for lemon shark, negaprion brevirostris implanted with acoustic transmitter. J. Fish Biol. 94, 173–177. doi: 10.1111/jfb.13856
Stamp T., Clarke D., Plenty S., Robbins T., Stewart J. E., West E., et al. (2021). Identifying juvenile and sub-adult movements to inform recovery strategies for a high value fishery - European bass (Dicentrarchus labrax). ICES J. Mar. Sci. 78, 3121–3134. doi: 10.1093/icesjms/fsab180
Svendsen E., Føre M., Økland F., Gräns A., Hedger R. D., Alfredsen J. A., et al. (2021). Heart rate and swimming activity as stress indicators for Atlantic salmon (Salmo salar). Aquaculture 531, 735804. doi: 10.1016/j.aquaculture.2020.735804
Swanberg T. R., Geist D. R. (1997). Effects of intraperitoneal transmitters on the social interaction of rainbow trout. N Am. J. Fish Manag 17, 178–181. doi: 10.1577/1548-8675(1997)017<0178:eoitot>2.3.co;2
Trefethen P. S. (1956). Sonic equipment for tracking individual fish (Washington, D.C.: US Department of the Interior, Fish and Wildlife Service).
Tunstrøm K., Katz Y., Ioannou C. C., Huepe C., Lutz M. J., Couzin I. D. (2013). Collective states, multistability and transitional behavior in schooling fish. PloS Comput. Biol. 9, e1002915. doi: 10.1371/journal.pcbi.1002915
Ulvund J. B., Engebretsen S., Alfredsen J. A., Kristensen T., Urke H. A., Jansen P. A. (2021). Behavioural response of farmed Atlantic salmon (Salmo salar l.) to artificial underwater lights: Wavelet analysis of acoustic telemetry data. Aquac Eng. 95, 102196. doi: 10.1016/j.aquaeng.2021.102196
Urbinati E. C., Zanuzzo F. S., Biller J. D. (2020). “Stress and immune system in fish,” in Biology and physiology of freshwater Neotropical fish (Elsevier), 93–114. doi: 10.1016/B978-0-12-815872-2.00005-1
Villegas-Ríos D., Freitas C., Moland E., Thorbjørnsen S. H., Olsen E. M. (2020). Inferring individual fate from aquatic acoustic telemetry data. Methods Ecol. Evol. 11, 1186–1198. doi: 10.1111/2041-210X.13446
Walker R. W., Ashton N. K., Brown R. S., Liss S. A., Colotelo A. H., Beirão B. V., et al. (2016). Effects of a novel acoustic transmitter on swimming performance and predator avoidance of juvenile Chinook salmon: Determination of a size threshold. Fish Res. 176, 48–54. doi: 10.1016/j.fishres.2015.12.007
Winter J. D. (1983). Underwater biotelemetry. In: Nielsen L.A.Johnson D f., Eds., Fisheries Techniques, American Fisheries Society, Bethesda, MD, 371–395.
Wright S., Metcalfe J., Hetherington S., Wilson R. (2014). Estimating activity-specific energy expenditure in a teleost fish, using accelerometer loggers. Mar. Ecol. Prog. Ser. 496, 19–32. doi: 10.3354/meps10528
Zale A. V., Brooke C., Fraser W. C. (2005). Effects of surgically implanted transmitter weights on growth and swimming stamina of small adult westslope cutthroat trout. Trans. Am. Fish Soc. 134, 653–660. doi: 10.1577/T04-050.1
Zhang Y., Li Y., Zhang L., Wu Z., Zhu S., Li J., et al. (2020). Site fidelity, habitat use, and movement patterns of the common carp during its breeding season in the pearl river as determined by acoustic telemetry. Water (Basel) 12, 2233. doi: 10.3390/w12082233
Zupa W., Alfonso S., Gai F., Gasco L., Spedicato M. T., Lembo G., et al. (2021). Calibrating accelerometer tags with oxygen consumption rate of rainbow trout (Oncorhynchus mykiss) and their use in aquaculture facility: A case study. Animals 11, 1496. doi: 10.3390/ani11061496
Keywords: collective behavior, swimming performance, aquaculture, biotelemetry, acoustic transmitters, optical flow analysis, stressors, fish welfare
Citation: Georgopoulou DG, Fanouraki E, Voskakis D, Mitrizakis N and Papandroulakis N (2022) European seabass show variable responses in their group swimming features after tag implantation. Front. Anim. Sci. 3:997948. doi: 10.3389/fanim.2022.997948
Received: 19 July 2022; Accepted: 24 August 2022;
Published: 15 September 2022.
Edited by:
Suresh Neethirajan, Wageningen University and Research, NetherlandsReviewed by:
Sébastien Alfonso, COISPA Tecnologia & Ricerca, ItalyCopyright © 2022 Georgopoulou, Fanouraki, Voskakis, Mitrizakis and Papandroulakis. This is an open-access article distributed under the terms of the Creative Commons Attribution License (CC BY). The use, distribution or reproduction in other forums is permitted, provided the original author(s) and the copyright owner(s) are credited and that the original publication in this journal is cited, in accordance with accepted academic practice. No use, distribution or reproduction is permitted which does not comply with these terms.
*Correspondence: Dimitra G. Georgopoulou, ZC5nZW9yZ29wb3Vsb3VAaGNtci5ncg==
Disclaimer: All claims expressed in this article are solely those of the authors and do not necessarily represent those of their affiliated organizations, or those of the publisher, the editors and the reviewers. Any product that may be evaluated in this article or claim that may be made by its manufacturer is not guaranteed or endorsed by the publisher.
Research integrity at Frontiers
Learn more about the work of our research integrity team to safeguard the quality of each article we publish.