- 1Department of Animal Science, University of Tennessee, Knoxville, TN, United States
- 2Department of Animal Science, Texas A&M University, College Station, TX, United States
- 3Department of Entomology and Plant Pathology, University of Tennessee, Knoxville, TN, United States
Uterine bacterial community abundances shift throughout the estrous cycle, potentially altering the immunological environment of the uterus and impacting subsequent fertility. The objective of the current study was to evaluate the immunological impact of lipopolysaccharide (LPS), as a model for potentially pathogenic bacteria, throughout the uterine endometrium between the luteal and follicular phase of the estrous cycle. Bovine uterine tracts were harvested in mid-luteal (n = 7) or follicular (n = 7) phase. Explants were collected from the contralateral and ipsilateral horn relative to the dominant follicle or corpus luteum, then subjected to one of three treatments: uncultured control, cultured control, or cultured with LPS (1 µg/mL). Explants underwent RNA extraction and targeted RNA sequencing for expression analyses of 40 immune response related genes. Sequencing reads were mapped to Bos taurus genome in CLC Genomics Workbench. Resulting total read counts were normalized by housekeeping gene GAPDH and analyzed for overall expression profile by Orthogonal Projections to Latent Structures Discriminant Analysis (OPLS-DA) and Variable Importance in Projection (VIP) analyses in Metaboanalyst. Individual gene expression differences were determined by GLIMMIX procedure in SAS with fixed effects of treatment, estrous phase, uterine horn, and their interaction, with random effect of individual uterus. Expression of 29 genes were affected among treatment groups, with seven genes increased in LPS treatment compared to other groups (P < 0.05). Multiple genes were affected by estrous phase and uterine horn, independent of treatment (P < 0.05). The OPLS-DA analyses indicated overall gene expression differences due to clustering by estrous cycle and treatment (P < 0.001), with no effect of uterine horn (P > 0.10). Similar clustering was observed between luteal and follicular phase explants of controls, but distinct separate clustering between phases with LPS treatment (P = 0.001). According to VIP analyses, mucins were identified as contributing the most to differences observed between phase and treatment. In conclusion, estrous cycle phase resulted in differing overall endometrial gene expression profiles of immune response to LPS treatment. Therefore, altered immunological environment of the uterus in response to bacteria at different estrous cycle stages may lead to differences in reproductive success.
Introduction
Reproductive failures and pregnancy losses cost the beef industry millions of dollars annually (Bellows et al., 2002). In postpartum cows, bacterial infections in the uterus are a common cause of low rebreeding success (Bonnett et al., 1993; LeBlanc et al., 2002; Sheldon et al., 2009; Ribeiro et al., 2013). Through parturition and uterine involution, there is high risk for bacterial contamination and infection resulting in uterine diseases. Bacterial communities in healthy postpartum cattle have shown to influence rebreeding success (Ault et al., 2019a; Ault et al., 2019b). Cows that did not become pregnant had a greater abundance of uterine disease-associated bacterial genera in the uterus, although no presence of infection was observed (Ault et al., 2019a). Interestingly, many of these potentially pathogenic bacteria in postpartum cows with uterine disease have also been found in the healthy virgin heifer and pregnant uterus (Moore et al., 2017). Specific genera of potentially pathogenic bacteria associated with uterine diseases include Acinetobacter, Fusobacteria, Proteus, Prevotella, and Peptostreptococcus, and many are classified as Gram-negative bacteria. Gram-negative bacteria are defined by their cell wall composition with a thin peptidoglycan layer and outer membrane containing lipopolysaccharide (LPS), which is considered an endotoxin that can elicit a significant immune response in the host (Dong et al., 2017). Through toll like receptor signaling, LPS stimulates a pro-inflammatory reaction in various tissues, mainly through the production of cytokines and chemokines (Nagaraja et al., 1978; Takeuchi et al., 1999; Huemann and Roger, 2002; Elson et al., 2006; Lu et al., 2008). Cytokines and chemokines are commonly known for regulating inflammation and immune cell recruitment (Dimberg, 2010), but are also involved in reproductive processes such as corpus luteum (CL) function, embryo development, placental attachment, and preventing rejection of the fetus (Zolti et al., 1991; Simón et al., 1998; Penny et al., 1999; Ealy et al., 2021). The presence of cytokines and chemokines have shown to fluctuate across the estrous cycle and pregnancy, and throughout puberty attainment (Krakowski and Zdzisinska, 2007; Oliveira et al., 2013; Ault-Seay et al., 2021; Poole et al., 2021). Due to the importance of inflammatory balance in the uterus (Raghupathy, 2001), minor shifts in bacterial communities may disrupt this balance and negatively influence reproductive success.
Similar to cytokines and chemokines, fluctuations in bacterial communities of the reproductive tract occur between the luteal and follicular phases of the estrous cycle (Laguardia-Nascimento et al., 2015; Ault et al., 2019a; Ault et al., 2019b; Quereda et al., 2020). The concentration of both local and systemic hormones, such as progesterone and estrogen, is a major distinguishing factor between phases of the estrous cycle, but their direct effect on bacterial communities is not well understood. However, the effects of reproduction-associated steroid hormones on the uterine endometrium may indirectly impact the uterine bacterial community composition. Changes in both bacterial diversity and taxonomic composition have been observed over natural estrous cycles, estrus synchronization, and differences in pregnancy status (Laguardia-Nascimento et al., 2015; Ault et al., 2019a; Ault et al., 2019b; Quereda et al., 2020). Major differences observed in bacterial communities between pregnant and non-pregnant cows occurred prior to insemination during estrus synchronization when CL regression would begin to occur and the transition from a progesterone dominated to estrogen dominated environment (Ault et al., 2019a; Ault et al., 2019b). Evaluating the impact of bacteria on the uterus during different phases of the estrous cycle will contribute to the understanding of microbial impact on reproductive performance. Additionally, gene expression analyses indicated distinct separation of global gene expression profiles between the contralateral and ipsilateral uterine horns, relative to the dominant follicle or CL, potentially due to the presence of a hormone concentration gradient (Pope et al., 1982; Boos et al., 1986; Takahashi et al., 2016; Sánchez et al., 2018). Therefore, the local hormonal concentration may impact endometrial activity and immune response to bacteria differently depending on the uterine horn. Assessing the response of the contralateral and ipsilateral endometrium in each phase of the estrous cycle will determine the response to bacteria through the hormone concentration differences and varying responses across the uterus. By first evaluating the impact of potentially pathogenic bacterial component LPS on the uterus and uterine environment through the estrous cycle, future studies may determine the direct influence of the reproductive tract microbiome on the uterus leading to differences in breeding success.
The objective of the current study was to evaluate the impact of LPS, as model for potentially pathogenic bacteria, on the endometrium throughout the uterus between the follicular and luteal phases of the estrous cycle. We hypothesized the immunological response in the endometrium to LPS will differ between both the luteal phase and follicular phase, and the ipsilateral and contralateral uterine horns.
Materials and methods
Collection and selection of reproductive tracts
Reproductive tracts were collected in late spring from the local abattoir immediately following slaughter. Only reproductive tracts with both ovaries attached and no abnormalities present on the ovaries were considered for sample collection. The reproductive tract and surrounding tissues were required to have moderate adiposity corresponding to a moderate BCS; tracts with no signs of adipose or excessive adiposity were not utilized. Tracts were then selected in the mid- to late luteal phase (progesterone dominated tract; n = 7), or in the follicular phase (estrogen dominated tract; n = 7). To determine the phases, gross ovarian measurements were evaluated to determine reproductive tract estrous cycle phase using standards previously described (Ireland et al., 1980; Miyamoto et al., 2000; Arosh et al., 2002). Example images of the contralateral and ipsilateral ovaries, relative to the dominant follicle or CL, on luteal and follicular phase tracts utilized for the current study are shown in Figures 1A–F. For the follicular phase (Figures 1A, B), a large, dominant, pre-ovulatory follicle (≥ 13 mm diameter) must be present on one ovary, and its closest uterine horn was considered the ipsilateral horn (Figure 1A). The second ovary had a small, regressing CL and/or small, non-dominant follicle(s), in which the closest uterine horn would be the contralateral horn (Figure 1B). For the luteal phase (Figures 1C–F), tracts were characterized by a large current CL on one ovary, and a small follicle(s) on either ovary; the uterine horn closest to the ovary with the CL was considered the ipsilateral horn. Luteal phase tracts were utilized if the CL was tan to orange in color externally and internally, ~1.5 to 2 cm in diameter, with some vasculature over apex and on periphery (Figures 1C, E). The follicles on either ovary were <10 mm in diameter, or not visible (Figures 1D, F). Reproductive tracts meeting these criteria for inclusion were transported on ice to the laboratory for processing within 1 hour (h).
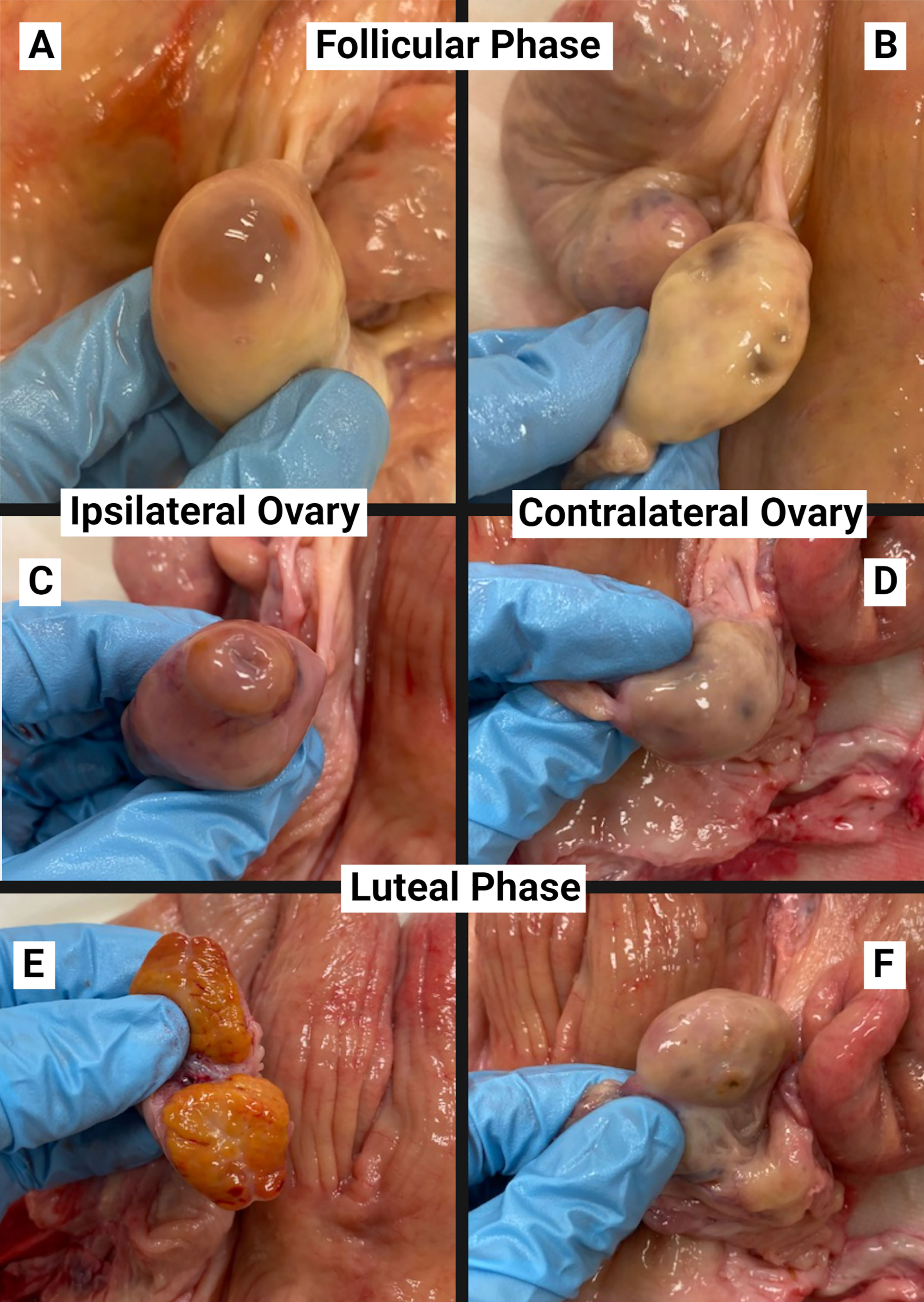
Figure 1 Examples of ovaries for staging of the estrous cycle on follicular phase (A, B) and luteal phase (C–F) reproductive tracts utilized for explant collection from the ipsilateral (A, C, E) and contralateral (B, D, F) uterine horn.
Endometrial explant collection, culture, and treatment
Pinching the cervix closed, reproductive tracts were rinsed with ethanol and water. The uterus was then cut away from all other tissues and the contralateral and ipsilateral horns of the uterus were separated. Each horn was opened longitudinally for collection of endometrial explants. If any signs of placental membranes, infection, excessive mucus, or abnormalities were observed once either uterine horn was opened, the whole tract was discarded and excluded from the study.
The contralateral and ipsilateral horns were split into even halves, resulting in four sections per uterus. Endometrial explants were collected using an 8mm biopsy punch. Explants were taken from the intercaruncular area approximately 1-1/12” from the uterine body and approximately 1-1/12” past the uterine bifurcation towards the tip of the uterine horn of the contralateral and ipsilateral horns. Any visible myometrium obtained during the biopsy punch was trimmed from the explant. Three explants were taken from each section for three treatments [uncultured control (UC), cultured control (CC), and LPS] resulting in 12 endometrial explants per tract. Figure 2 depicts sections of each uterine horn and collected explants. Explants serving as uncultured controls were immediately snap frozen in liquid nitrogen and stored at -80°C. Explants for both culture treatments were placed in 20 mL of Hank’s Balanced Salt Solution (HBSS; Gibco, Waltham, Massachusetts, USA) until all explants were collected. Explants were transferred from the first HBSS medium to a second conical of 20 mL of HBSS medium, and transferred to 20 mL HBSS containing 1% antibiotic and antimycotic media (ABAM; Gibco, Waltham, Massachusetts, USA) until plating for culture within 20 minutes.
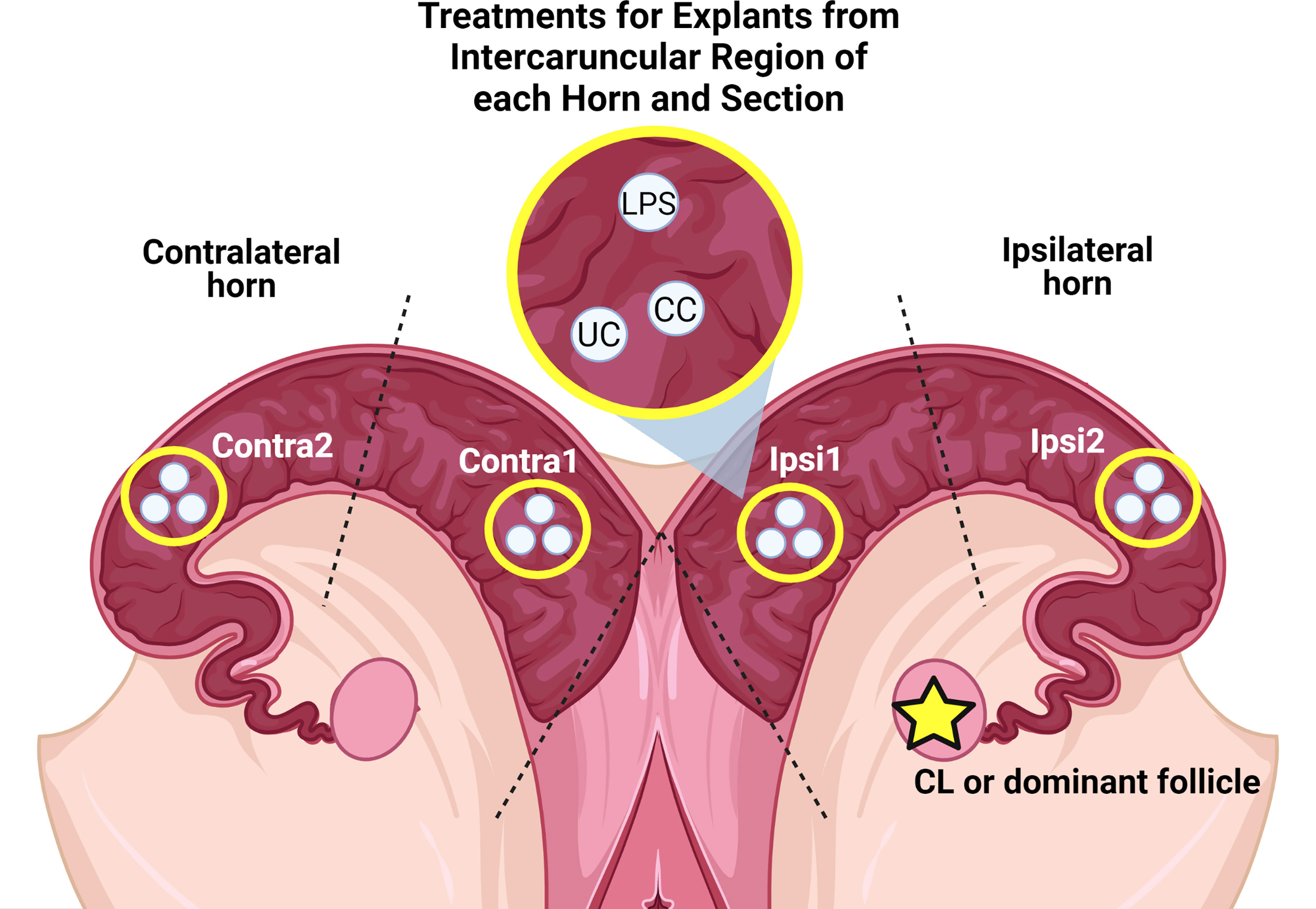
Figure 2 Sections of uterine horns with collected explants from the intercaruncular regions and their respective treatments. LPS: Cultured with complete media + LPS. CC: Control cultured in complete media without LPS. UC: Uncultured control.
Explants were removed from HBSS with 1% ABAM and plated into individual wells with 1 mL of Roswell Park Memorial Institute 1640 medium (RPMI; Gibco, Waltham, Massachusetts, USA) with 1% ABAM that was equilibrated to culture conditions in incubator. Plates with explants were incubated for 2 h at 38.8°C with 5% CO2 and air for removal of cellular debris and red blood cells. After incubation, explants were transferred to a new plate into individual wells containing 1 mL of fresh equilibrated medium (RPMI with 1% ABAM) for cultured controls or fresh equilibrated medium containing 1 µg/mL LPS (Ultrapure LPS, E. coli 0111:B4; In vivoGen, San Diego, CA, USA; Dohmen et al., 2000; Herath et al., 2006). Following incubation at 38.8°C with 5% CO2 and air for 8 h, explants were removed and snap frozen in liquid nitrogen, then stored at -80°C.
RNA extraction, cDNA synthesis, and targeted RNA sequencing
Total RNA was extracted from ~50 mg of endometrial explant tissue using TRIzol (Invitrogen, Carlsbad, CA, USA) and chloroform (Thermo Scientific, USA) in combination with the Qiagen RNeasy kit (Qiagen, Hilden, Germany). Explants were homogenized in TRIzol for four minutes with a 5 mm steal bead (Qiagen, Hilden, Germany) on the tissue homogenizer (Fisher). Following homogenization, 100 µL chloroform was added to the homogenized sample and centrifuged at 12,000 × g for 15 minutes at 4°C. The aqueous layer was then transferred to a new tube containing 1.5x volume of 100% ethanol. The resulting solution was added to a RNeasy column and proceeded with the Qiagen RNeasy kit according to manufacturer instructions. Quantity and quality of extracted RNA was evaluated via NanoDrop 1000 spectrophotometer (Thermo Scientific, Rockford, IL, USA) and Agilent 2100 bioanalyzer (Agilent, Santa Clara, CA, USA). Extracted RNA with a RIN value < 6 and concentration < 35 ng/uL were not utilized for cDNA synthesis and further analyses. Synthesis of cDNA from extracted RNA was performed using the High Capacity cDNA Reverse Transcription kit (Applied Biosystems, Waltham, MA, USA) according to manufacturer protocol with ~500 ng/mL of RNA.
Genes of interest related to immune response and associated with reproductive processes were selected for targeted RNA sequencing and identified using the NCBI Gene database from the Bos taurus genome. A total of 40 genes were selected including interleukin family genes (IL-1α, IL-1β, IL-4, IL-6, IL-10, IL-17A, IL-36RA, LIF), various cytokines (TGFβ, TNFα, VEGFA), CXCL chemokines (CXCL1, CXCL2, CXCL8, CXCL10), CCL chemokines (CCL2, CCL3, CCL4), mucins (MUC1, MUC4, MUC13, MUC15), steroid receptors (PGR, ESR1, ESR2), interferon associated genes (IFNγ, MX1, MX2, ISG15, OAS2, IRF1, IRF3, IRF8), colony stimulating factors (CSF2, CSF3), prostaglandin pathway genes (PTGES, PTGES2, PTGESR2), and toll-like receptor pathway genes (TLR4, NFκB1). For all genes of interest, primers were designed in NCBI Primer BLAST to span across an intron and result in amplicons of 90-110 base pairs. Quantification of housekeeping gene GAPDH was included for sample normalization. Table 1 lists genes of interests with their respective accession numbers from the NCBI database and resulting primers. Amplification of cDNA was performed in duplicate via Hi-Plex approach optimized by Floodlight Genomics (Knoxville, TN, USA), followed by multiplex sequencing using a 2 × 150 kit on an Illumina HiSeq X (San Diego, CA, USA). Resulting reads were mapped to the Bos taurus genome using CLC Genomics Workbench version 9.5.2 (Qiagen, Bethesda, MD, USA). Reads were standardized according to total read count per sample to obtain the proportion of reads for each gene. Values for each sample duplicates were averaged among each gene within each sample for further analyses (Mihelic et al., 2020).
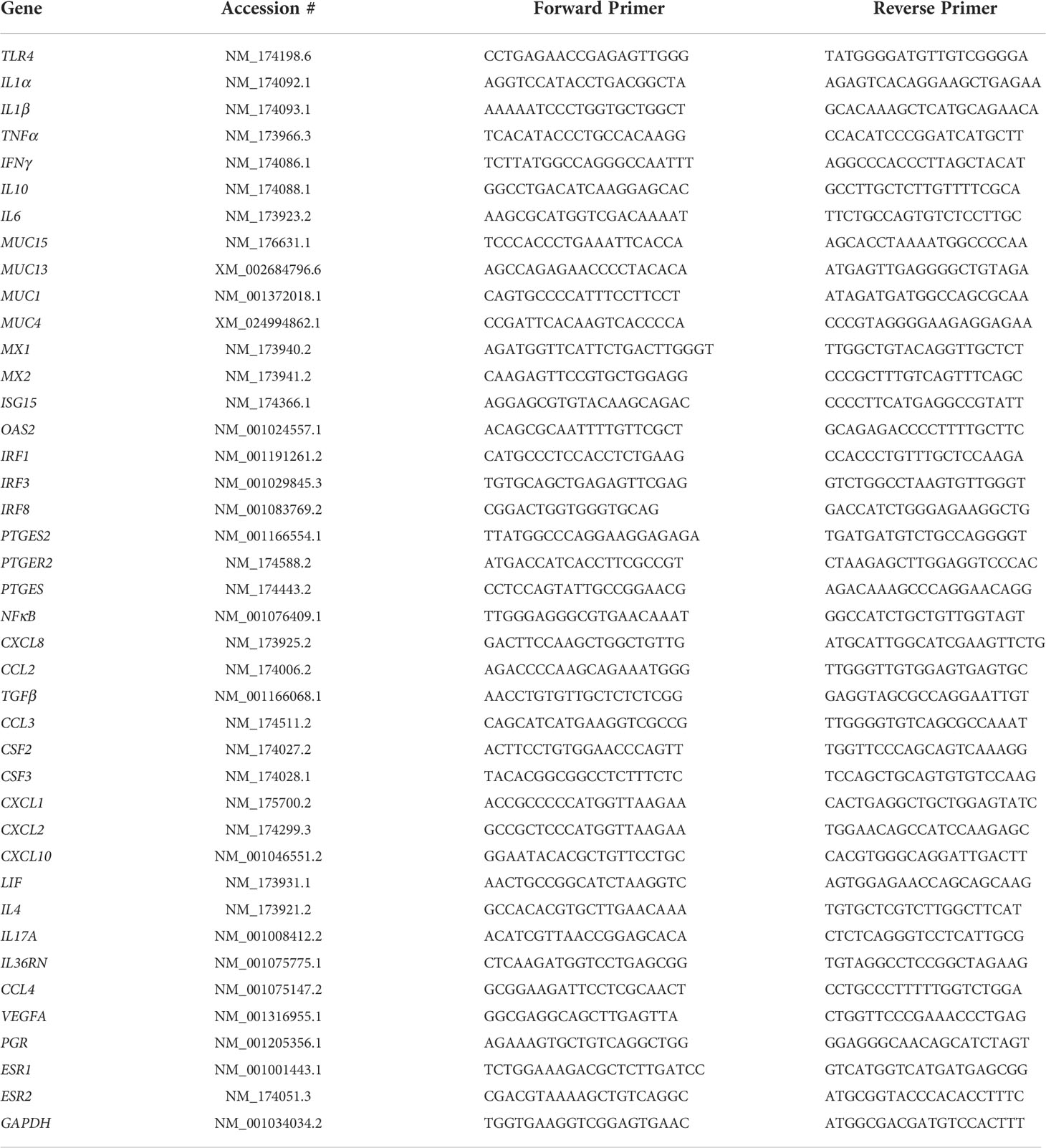
Table 1 Genes of interest and NCBI gene accession number with resulting forward and reverse primers for targeted RNA sequencing.
Statistical analyses
Resulting gene expression values were imported to Metaboanalyst 5.0 and normalized according to the expression of housekeeping gene GAPDH, which indicated stable expression among treatment, estrous cycle phase, and uterine horn. Orthogonal Projections to Latent Structures Discriminant Analysis (OPLS-DA) were performed in Metaboanalyst 5.0 to evaluate the overall gene expression differences between groups. Variable importance in projection (VIP) plots were generated to determine genes contributing to clustering differences between groups in the OPLS-DA. Resulting normalized expression values from Metaboanalyst 5.0 were also utilized for a GLIMMIX procedure in SAS 9.4 with effects of treatment (uncultured and cultured controls, and LPS), estrous cycle phase (follicular and luteal), uterine horn (contralateral and ipsilateral), and their interaction, with random effect of uterus. Section of the uterine horn from which the explant was collected was evaluated but did not affect any individual gene expression or overall gene expression via OPLS-DA and was removed from all analyses. Normal distribution of gene expression values were evaluated by the UNIVARIATE procedure and genes with a Shapiro-Wilk score < 0.85 were log transformed. Gene expression values are reported as the mean normalized total count ± standard error of the mean (SEM). Significance for all analyses was determined by a P ≤ 0.05, and tendencies with P ≤ 0.10.
Results
Estrous cycle phase
Overall expression of targeted genes of interest was evaluated by OPLS-DA. Distinct clustering of samples was observed between luteal and follicular phase samples with slight overlap (P < 0.001; Figure 3A). Clustering within each phase indicates similar overall expression of targeted genes within each phase. The overlap observed between phases indicates some similarity of gene expression not impacted by phase of the estrous cycle. The five most influential genes contributing to observed clustering within each phase, determined from VIP scores, were MUC13, MUC1, IFNγ, ISG15, and ESR1 (Figure 3B). Of these genes, MUC13, MUC1, ISG15, and ESR1 had greater expression in explants of luteal than follicular phase, while IFNγ had greater expression in the follicular than luteal phase (Figure 3B).
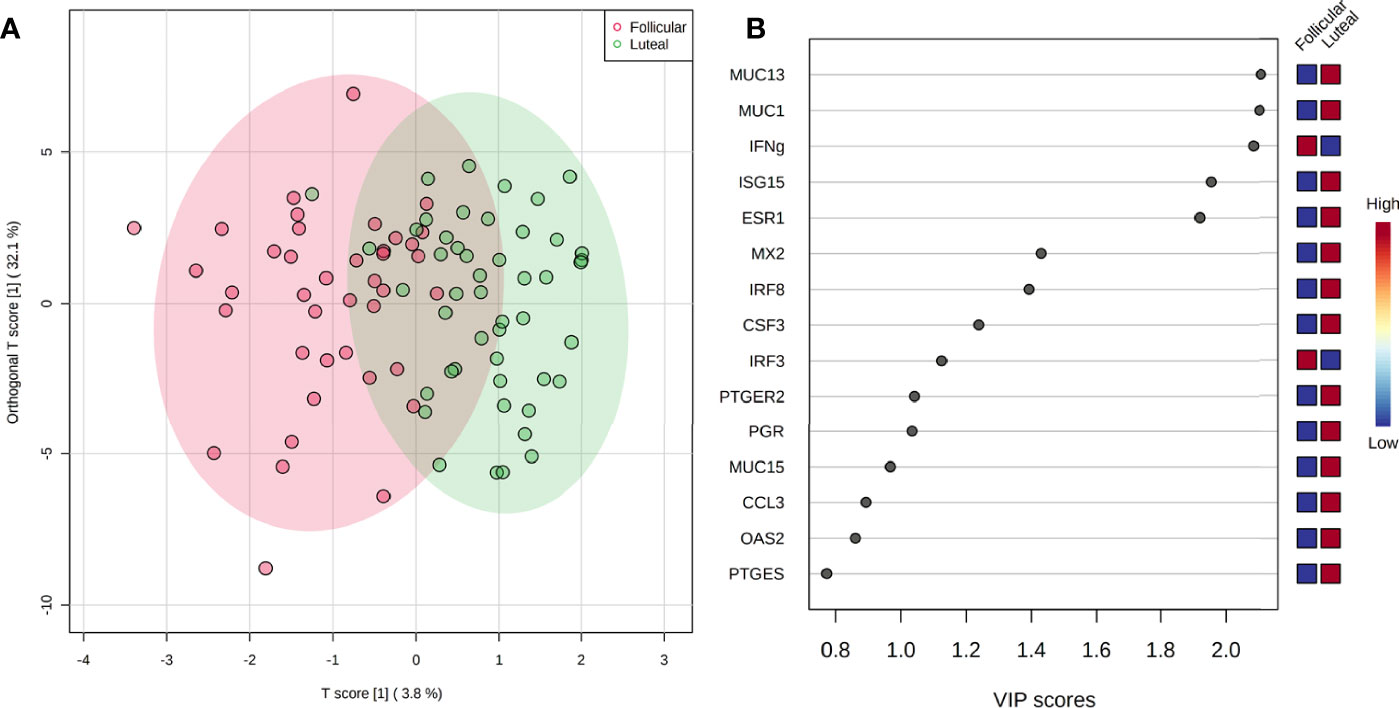
Figure 3 Overall gene expression clustering of explants between the estrous cycle phases (P < 0.001) by OPLS-DA (A) with corresponding VIP plot (B) indicating genes contributing to observed clustering.
Differences in the expression of individual genes by estrous phase was determined by ANOVA. Estrous cycle phase affected expression of genes ESR1, ISG15, IL-1α, PTGER2, PGR, MUC1, MUC13, and NFκB1 (P < 0.05), and tended to affect CXCL8 and IL-1β (P = 0.09; Table 2). All genes impacted by estrous cycle phase had greater expression in explants from the luteal phase tissue compared with follicular phase tissues (Table 2).
Explant culture and LPS treatment
Treatment of explants indicated clustering, with complete separation of uncultured controls from cultured controls and LPS, which also clustered, but with slight overlap (P < 0.001; Figure 4A). Scores from VIP determined CXCL8, IL-6, IL-1β, CXCL2, and IL-1α to contribute the most to differences between treatment groups (Figure 4B). Cytokines CXCL8, IL-6, IL-1β, and CXCL2 had greater expression in cultured control explants, with the least expression in uncultured control explants. Expression of IL-1α was greatest in LPS treated and the least in uncultured controls (Figure 4B). Due to observed difference between uncultured and cultured explants, cultured explants serve as a better control to determine the specific effect of LPS. Therefore, an OPLS-DA was conducted with uncultured controls removed. In comparison of cultured controls and LPS treated explants, clear separation of overall gene expression between the groups was observed (P < 0.001; Figure 4C). The VIP plot indicated CSF2, CCL3, LIF, IL-10, and OAS2 contributed the most to the separation of cultured controls and LPS treated explants, with greater expression in LPS explants except LIF which was greater in cultured controls (Figure 4D).
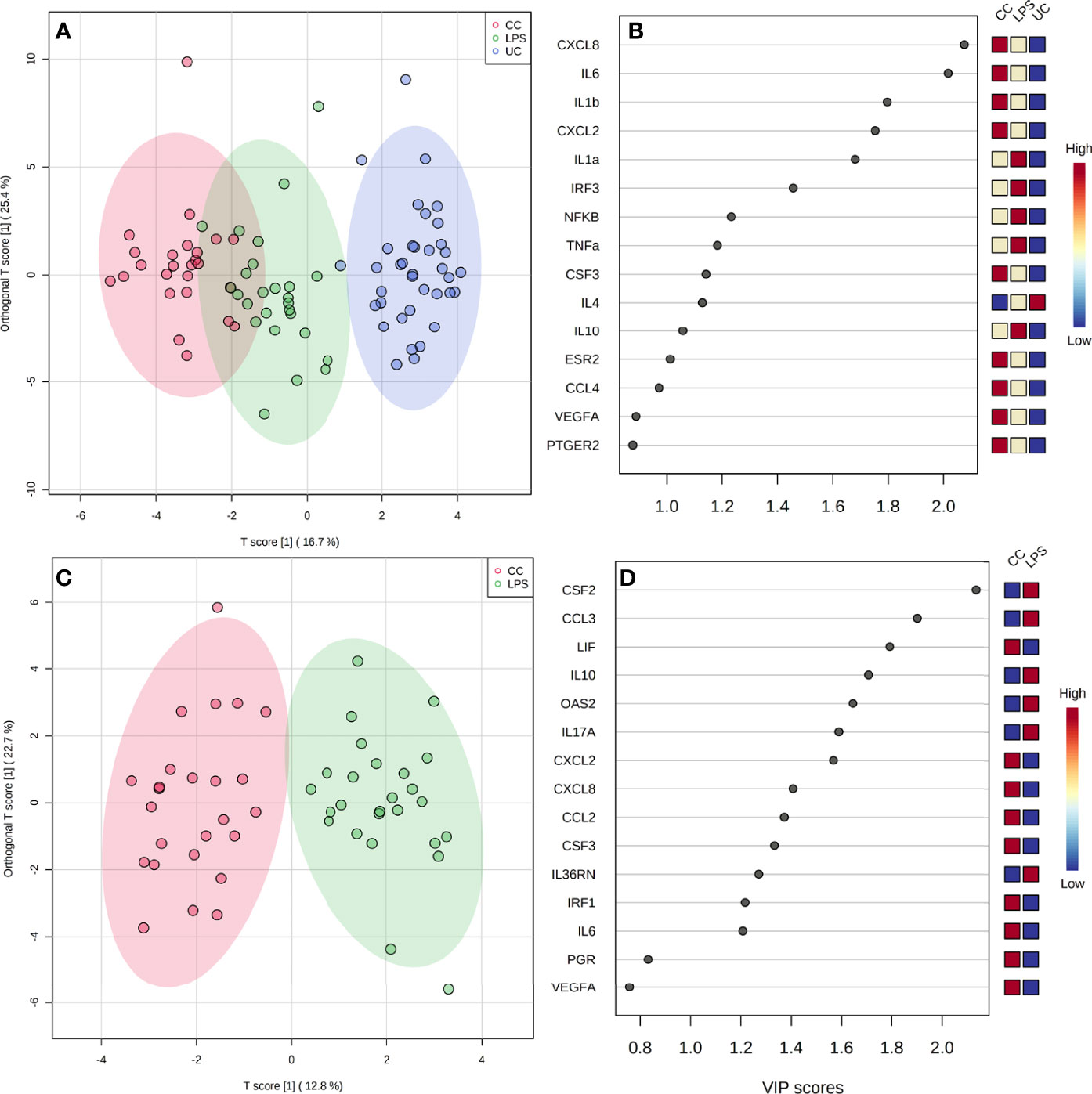
Figure 4 Overall gene expression clustering of explants between all treatments [(A); P < 0.001], and between LPS and cultured control treatments (C), by OPLS-DA, with corresponding VIP plots (B, D) indicating genes contributing to observed clustering. CC, Cultured Controls; LPS, Lipopolysaccharide; UC, Uncultured Controls.
The expression of 29 genes were affected by treatment (P < 0.05, Table 3). Of these genes, 15 were affected between uncultured versus cultured explants. Expression of CCL4, TNFα, ESR1, IRF3, IL-1β, VEGFA, IL-6, TLR4, CXCL2, ISG15, PTGER2, ESR2, and NFκB1 was the least in uncultured explants, with greater expression in cultured tissue and did not differ between cultured controls and LPS explants (P < 0.05, Table 3). In contrast, IFNγ (P = 0.003) and IL-4 (P < 0.0001) had the greatest expression in uncultured explants, with lesser expression in cultured tissue but similar expression between cultured controls and LPS explants. Explants from cultured control treatment group had greater expression of CXCL8, TGFB, CSF3, PGR, and MX1 relative to the LPS and uncultured control groups (P < 0.05, Table 3). Six genes increased expression in response to LPS including IL-10, IL-1α, IL-17A, CSF2, CCL3, and IL-36RN, with greatest expression in LPS explants, intermediary expression in cultured control explants, and lowest expression in uncultured explants (P < 0.05, Table 3). Conversely, LPS explants had lower expression of IRF1 (P = 0.005) and CCL2 (P < 0.0001) compared to uncultured and cultured control explants which were similar in expression (Table 3).
Uterine horn
Separation of overall gene expression was not observed by uterine horn as profiles completely overlapped in the OPLS-DA (P = 0.41). However, expression of individual genes differed by uterine horn including ESR1, IL-10, OAS2, MUC1, MUC13, IFNγ, and MUC15 (P ≤ 0.05), with tendencies for CCL4, IRF8, OAS2, NFκB1, and VEGFA (P ≤ 0.10; Table 4). The ipsilateral horn had the greatest expression of all affected genes (Table 4).
Interactions of estrous cycle phase, treatment, and uterine horn
The relationship between phase of the estrous cycle and treatment is depicted in Figures 5A–C. Separation with slight overlap of follicular and luteal phase explants in uncultured (P = 0.001, Figure 5A) and cultured controls (P = 0.01, Figure 5B) was observed. However, distinct separation with no overlap of follicular and luteal phase explants in response to LPS treatment was observed (P = 0.001, Figure 5C), indicating differing responses between estrous cycle phases to LPS than other treatments. The VIP for the genes contributing to differences in observed clustering between phases specifically for LPS treatment is depicted in Figure 5D.
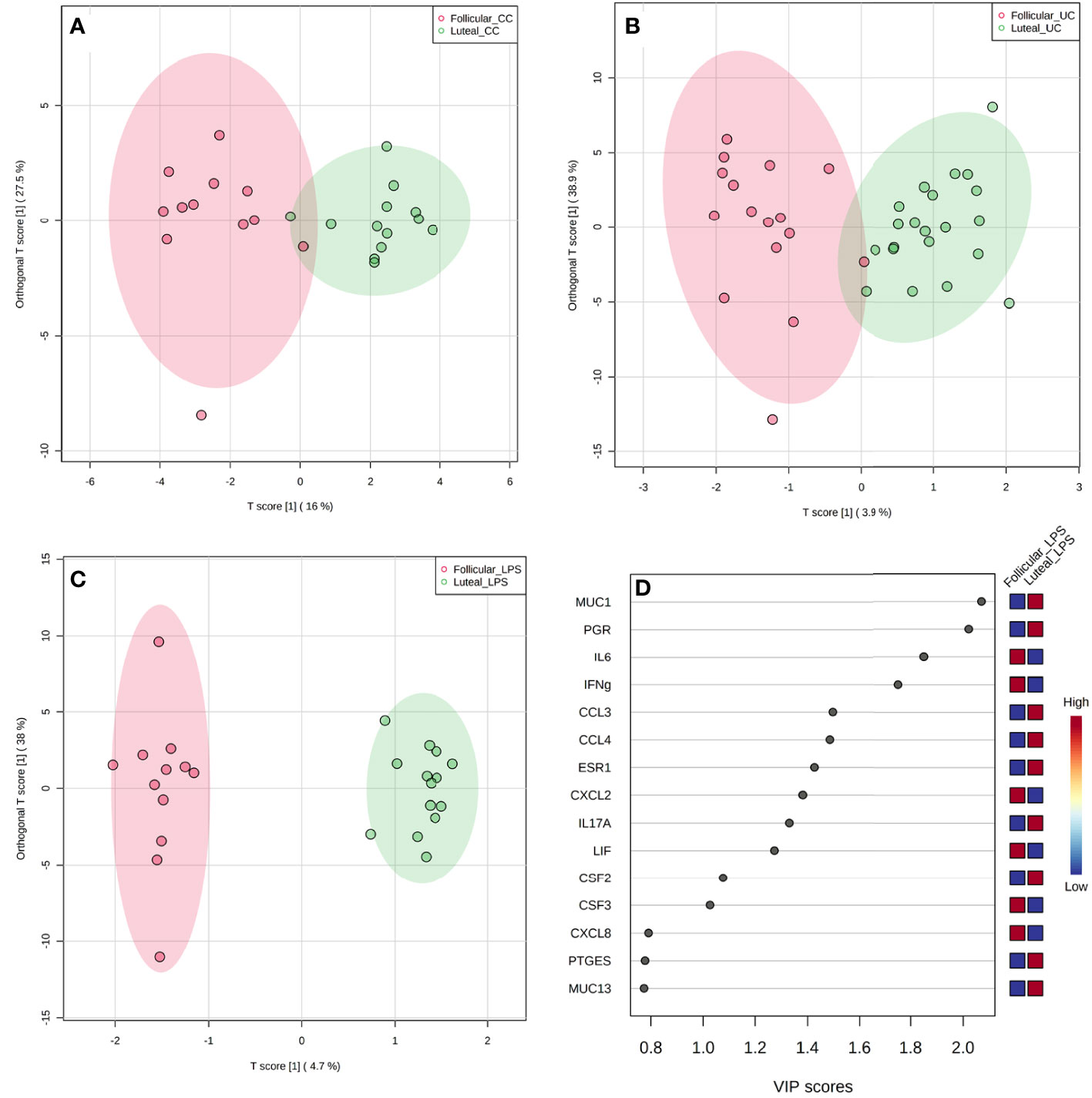
Figure 5 Overall gene expression clustering by OPLS-DA between follicular and luteal phase explants for uncultured control [(A); UC; P = 0.001], cultured control [(B); CC; P = 0.01], and LPS [(C); P = 0.001] treatments with VIP plot (D) corresponding to LPS treatment clustering between phases.
For individual gene expression differences, the interaction of treatment × phase was observed for IL-1α (P < 0.01) and PGR (P = 0.05), with tendencies for ISG15 (P = 0.10) and NFκB1 (P = 0.07). For IL-1α, the least expression was observed for uncultured explants of luteal and follicular phase, with the greatest expression in LPS explants of luteal and follicular phase. Similarly, luteal and follicular uncultured explants had the least expression of PGR. However, follicular LPS and cultured control explant PGR expression did not differ from uncultured controls, though expression was higher in luteal cultured control explants than all other groups.
No interactions between treatment × horn were observed, but the interaction of phase × horn was detected for ESR1 and MUC13 (P = 0.05). Expression of ESR1 and MUC13 followed the same pattern, with greatest expression detected in the ipsilateral horn of luteal phase explants, with similar expression in the luteal contralateral horn and follicular ipsilateral horn. However, less expression of ESR1 and MUC13 was observed in the follicular contralateral horn (P = 0.05). No genes were affected by the triple interaction of treatment, phase, and horn.
Discussion
Potentially pathogenic bacteria are present in healthy microbial communities, including the uterine microbiome, but can increase in abundance and lead to a diseased state if provided the necessary shift in their environment (Karstrup et al., 2017). Various stimuli such as diet and the external environment can influence microbiome composition and its respective environment, leading to greater abundances of potentially pathogenic bacteria (Li et al., 2012; Monteiro and Faciola, 2020). Recently, shifts in the abundance of common potential pathogens in a healthy uterus through the estrous cycle were associated with influencing breeding success (Ault et al., 2019a). Interactions of bacteria with the uterine environment leading to changes in reproductive outcomes must be explored to better understand their role in reproductive efficiency. Therefore, the current study utilized LPS as a model for potentially pathogenic bacteria to evaluate its effects on the local immune response of the uterine endometrium between phases of the estrous cycle and across the uterine horns. Differences in the expression of LPS binding pattern recognition receptor TLR4 and transcription factor NFκB1 were not detected in LPS treated explants. Previous studies have reported the ability of LPS to increase expression of TLR4, with other studies reporting minimal to no difference between control and LPS challenged tissues, potentially due to differences in LPS concentration and duration of exposure (Cronin et al., 2012; Swangchan-Uthai et al., 2012; Fu et al., 2013; Ding et al., 2020). The TLR4 receptors are present on endometrial tissue, including epithelial and stromal layers, and resident immune cells, such as macrophages, without LPS challenge in preparation for bacterial response (Herath et al., 2006; Dadarwal et al., 2017). Although tissues were selected based on negative signs of pregnancy and infection, level of TLR4 expression may be influenced by the state of the uterine microbiome, previous infection, or reproductive status prior to tissue collection.
No differences in the individual cytokine gene expression were detected in commonly LPS-stimulated cytokines TNFα, IL-6, and IL-1β of the current study. The LPS concentration used has been indicated to be present in the bovine postpartum uterus and validated to elicit a response (Dohmen et al., 2000; Herath et al., 2006), with responses to LPS occurring as early as 3 h, up to 24 h (Borges et al., 2012; Swangchan-Uthai et al., 2012; Fu et al., 2013). However, the early response to LPS may result in various patterns of cytokine concentrations. Concentration of TNFα peaked by 1 h in bovine endometrial culture with LPS, while IL-1β did not reach peak concentration until 3 to 6 h post LPS, with both decreasing up to 24 h (Swangchan-Uthai et al., 2012; Fu et al., 2013). Other pro-inflammatory cytokines IL-1α and IL-17A, and chemokine CCL3, however, had greater expression in LPS treated explants of the current study. Similarly, bovine endometrial epithelial cells treated with LPS indicated IL-1α to be the most upregulated pro-inflammatory cytokine gene, and one of the most upregulated genes among all immune response genes (Oguejiofor et al., 2015). A greater response of IL-1α than IL-1β and IL-6 relative to controls was observed in LPS treated endometrial cells, suggesting IL-1α aids in upregulating the inflammatory response to LPS (Healy et al., 2014). The IL-17 signalling pathway was also identified as a major inflammatory pathway in LPS stimulation (Ding et al., 2020). The chemokine CCL3 is produced by immune cells, such as macrophages and dendritic cells, to upregulate an inflammatory response by recruiting additional immune cells for LPS clearance (Jing et al., 2003). Therefore, an in vivo model may result in greater overall cytokine and chemokine concentrations due to increased immune cell infiltration from other locations to the endometrium. In addition to these increases in pro-inflammatory cytokine expression, regulatory cytokine IL-10 also had greater expression in explants treated with LPS. Regulatory cytokine expression, such as IL-10, can increase in response to elevated pro-inflammatory cytokine concentrations to mediate the level of inflammation (Dadarwal et al., 2017). An increased ratio of IL-1α and IL-1β to IL-10 concentration in postpartum cows with uterine disease was associated with failure to rebreed (Herath et al., 2009). Increases in regulatory and anti-inflammatory cytokines can result from a transition in macrophage activation or by production of the affected tissue cells. Stromal cells of the endometrium have indicated greater production of IL-10 when exposed to LPS compared to controls and epithelial cells treated with LPS (Koh et al., 2018). Therefore, treatment of LPS on endometrial explants in the current study resulted in increased pro-inflammatory cytokine expression, but concurrent upregulation of IL-10 expression may indicate an attempt to balance the inflammatory state of the endometrium.
Steroid hormones estradiol and progesterone dominate specific phases of the estrous cycle to prepare the uterus for reproductive events such as pregnancy development and maintenance. As expected, differences in the gene expression of PGR and ESR1 were observed between phases, with PGR expression highest during the luteal phase of the current study. The abundance of progesterone receptors on the endometrium epithelial cells peaks during mid-luteal phase with increasing progesterone secretion (Robinson et al., 2001; Okumu et al., 2010). Following mid-luteal phase, nuclear progesterone receptors begin to decrease in the surface endometrial epithelial cells, a prerequisite of uterine receptivity and the endometrial luteolytic mechanism, but remain present in stromal cells (Kimmins and MacLaren, 2001; Okumu et al., 2010). Additionally, PTGER2 expression was greater in luteal phase explants of the current study. Secretion of PGE2 and its receptor PTGER2 expression has previously shown to be greater during mid-diestrus when progesterone secretion is greatest, then decreases around the time of luteolysis (Arosh et al., 2003; Zhang et al., 2017). Therefore, greater expression of PGR and PTGER2 in the current study, in addition to careful selection of reproductive tracts after staging the estrous cycle, would indicate successful selection of uteri during the mid-luteal phase when maternal blood progesterone concentrations are high. Due to the importance of controlling the maternal immune system response to prevent rejection of the fetus, progesterone dominance is typically classified as an anti-inflammatory period (Raghupathy, 2001; Schjenken et al., 2012). However, IL-1α, IL-1β, and CXCL8, which are classically categorized as pro-inflammatory, were detected in greater abundance in luteal phase explants in the current study. High progesterone supplementation on bovine endometrial epithelial cells has indicated increases in pro-inflammatory cytokine expression (Cui et al., 2020). But when separated by estrous cycle stage, concentrations of IL-1α, IL-1β, and CXCL8 were greater in early to mid-diestrus, but decreased by late-diestrus in non-pregnant animals, suggesting preparation for pregnancy beginning around the time of maternal recognition (Oliveira et al., 2013). Therefore, fluctuations of individual inflammatory cytokines occur throughout the estrous cycle, reaching an anti-inflammatory state by the time of pregnancy establishment (Oliveira et al., 2013). However, these differences across the cycle may indicate a greater susceptibility when exposed to stressors such as pathogenic bacteria and their LPS membrane component.
The inflammatory response to a stressor is not based on the expression of a single immune response mediator, but the cohesive actions of multiple cytokines, chemokines, and other immune response related proteins from a variety of immune cells and tissues. Therefore, the overall profile of immune-related gene expression may be a better representation of the response to LPS and differences between estrous cycle phases. Interestingly, profiles grouped by estrous cycle phases but with similar gene expression of both control treatment groups, but distinct profiles were observed in response to LPS treatment between the follicular and luteal phase. The mucin-family genes, MUC1 and MUC13, were affected by estrous cycle phase with greater expression in the luteal than follicular phase, and were determined by VIP as the top contributors to differences in overall gene expression between phases and phase response to LPS. Mucins are anti-adhesive proteins that serve as a defense mechanism for the innate immune system, especially in the reproductive tract (Brayman et al., 2004). Greater expression of mucins has been associated with uterine disease and infertility, potentially preventing trophoblast attachment (Horne et al., 2005; Kasimanickam et al., 2014). Decreases in endometrium epithelial PGR expression during the late luteal phase, especially before maternal recognition of pregnancy in pregnant animals, is associated with reductions in mucin expression to prepare the uterus for conceptus attachment and implantation (Carson et al., 2000; Spencer et al., 2006). As mucins are a surface protein in the uterine lumen, the greater expression of PGR, MUC1, and MUC13 in luteal phase explants of the current study indicate mid-luteal phase uteri prior to the loss of PGR on endometrial epithelial cells. Although individual mucin expression was not affected by LPS treatment, OLPS-DA analysis indicated MUC1 to contribute the most to the difference between luteal and follicular phase response to LPS. Additionally, pro-inflammatory IL-6 and IFNy were important contributors to phase response to LPS, being higher in follicular phase explants, although they did not differ in individual expression by treatment. Due to the protective role of mucins to bind bacteria and prevent invasion into uterine tissue (Brayman et al., 2004; Sando et al., 2009), mucin expression differences between estrous phases may be a result of the total cytokine and other immune factor responses, therefore affecting uterine response to bacteria.
Greater progesterone concentrations have been previously observed in the cranial portion of the ipsilateral horn closest to the CL, compared to the contralateral horn, suggesting a hormonal gradient throughout the uterus potentially impacting the uterine environment in response to progesterone actions (Pope et al., 1982; Weems et al., 1988; Takahashi et al., 2016). Global gene expression analysis has indicated greater variation between the contralateral and ipsilateral horns during the early estrous cycle that decreased towards the period of uterine receptivity to pregnancy (Sánchez et al., 2018). All mucins evaluated in the current study had greater expression in the ipsilateral than contralateral horn. The strong influence of steroid hormones on the expression of mucins potentially contributed to their observed differences by uterine horn, although divisions of each horn did not impact expression in the current study. In addition to mucins, multiple other genes were affected by explants taken from the contralateral and ipsilateral horn. Expression of major angiogenic cytokine VEGFA was increased in the ipsilateral horn compared to the contralateral horn. Blood flow to the ipsilateral horn, especially in preparation for pregnancy is crucial to supply the early embryo with nutrients for survival (Ford et al., 1979). Changes in VEGFA suggest vascular development cytokines begin to stimulate these changes in the estrous cycle of non-pregnant animals. Additionally, both regulatory cytokine IL-10 and pro-inflammatory IFNy had greater expression in the ipsilateral horn. Previous studies indicated altered response of the ipsilateral or contralateral uterine tissue to culture with a conceptus (Bagés-Arnal et al., 2020). Due to the importance of a controlled immune response for pregnancy development (Raghupathy, 2001), differences in cytokine expression between uterine horns may indicate regulation of immune status is necessary in the ipsilateral horn for pregnancy establishment and maintenance. Therefore, the majority of genes that differed between the contralateral and ipsilateral uterine horns are important for pregnancy establishment and maintenance, however further investigation is needed to determine how they may impact breeding success.
In conclusion, estrous cycle phase resulted in differing overall endometrial gene expression profiles of immune response to LPS treatment. Multiple immune response genes differed between the follicular and luteal phases. Mucins are greatly impacted by the dominant steroid hormone present of each estrous cycle phase and their protective role during high abundance of the luteal phase likely impacts the resulting immune response to a stimulus such as LPS. However, overall gene expression profile did not differ between uterine horns and did not influence response to LPS treatment. Further research is needed to understand how these differences in estrous cycle response to LPS correlate to changes in the uterine bacterial communities and their direct effects on pregnancy establishment.
Data availability statement
The datasets presented in this study can be found in online repositories. The names of the repository/repositories and accession number(s) can be found below: https://www.ncbi.nlm.nih.gov/, PRJNA824812.
Ethics statement
Ethical review and approval was not required for the animal study because no live animals were utilized for the research.
Author contributions
TA-S was responsible for primary manuscript preparation, experimental design, sampling, RNA extraction, and data analyses. RP assisted with laboratory analyses and manuscript preparation. KL was responsible for targeted RNA sequencing. BV and ES assisted with targeted RNA sequencing preparation and data analyses. SM, KP, FS, DM, KM, and PM were responsible for experimental design development and manuscript preparation. All authors contributed to the article and approved the submitted version.
Funding
The authors would like to thank the state of Tennessee through UT AgResearch, the Department of Animal Science, and the USDA National Institute of Food and Agriculture, Hatch Project No. 1019048 for providing funding and support for this research.
Acknowledgments
Thank you to Southern Provisions LLC for allowing us to collect the reproductive tracts utilized for the project. Thank you to the graduate and undergraduate students that assisted in collecting and processing of reproductive tracts.
Conflict of interest
The authors declare that the research was conducted in the absence of any commercial or financial relationships that could be construed as a potential conflict of interest.
Publisher’s note
All claims expressed in this article are solely those of the authors and do not necessarily represent those of their affiliated organizations, or those of the publisher, the editors and the reviewers. Any product that may be evaluated in this article, or claim that may be made by its manufacturer, is not guaranteed or endorsed by the publisher.
References
Arosh J. A., Banu S. K., Chapdelaine P., Emond V., Kim J. J., MacLaren L. A., et al. (2003). Molecular cloning and characterization of bovine prostaglandin E2 receptors EP2 and EP4: Expression and regulation in endometrium and myometrium during the estrous cycle and early pregnancy. Endocrinology 144 (7), 3076–3091. doi: 10.1210/en.2002-0088
Arosh J. A., Parent J., Chapdelaine P., Sirois J., Fortier M. A. (2002). Expression of cyclooxygenases 1 and 2 and prostaglandin e synthase in bovine endometrial tissue during the estrous cycle. Biol. Reprod. 67 (1), 161–169. doi: 10.1095/biolreprod67.1.161
Ault T. B., Clemmons B. A., Reese S. T., Dantas F. G., Franco G. A., Smith T. P. L., et al. (2019a). Bacterial taxonomic composition of the postpartum cow uterus and vagina prior to artificial insemination. J. Anim. Sci. 97 (10), 4305–4313. doi: 10.1093/jas/skz212
Ault T. B., Clemmons B. A., Reese S. T., Dantas F. G., Franco G. A., Smith T. P. L., et al. (2019b). Uterine and vaginal bacterial community diversity prior to artificial insemination between pregnant and nonpregnant postpartum cows. J. Anim. Sci. 97 (10), 4298–4304. doi: 10.1093/jas/skz210
Ault-Seay T. B., Harrison T. D., Brandt K. J., Payton R. R., Schneider L. G., Myer P. R., et al. (2021). Short Communication: The effects of protein level on cytokines and chemokines in the uterine environment of beef heifers during development. J. Anim. Sci. doi: 10.1093/jas/skab105
Bagés-Arnal S., Sánchez J. M., Fernandez-Fuertes B., McDonald M., Behura S. K., Spencer T. E., et al. (2020). Location relative to the corpus luteum affects bovine endometrial response to a conceptus. J. Reprod. 159 (5), 643–657. doi: 10.1530/rep-19-0464
Bellows D. S., Ott S. L., Bellows R. A. (2002). Review: Cost of reproductive diseases and conditions in cattle. Prof. Anim. Sci. 18 (1), 26–32. doi: 10.15232/S1080-7446(15)31480-7
Bonnett B. N., Wayne Martin S., Meek A. H. (1993). Associations of clinical findings, bacteriological and histological results of endometrial biopsy with reproductive performance of postpartum dairy cows. Prev. Vet. Med. 15 (2), 205–220. doi: 10.1016/0167-5877(93)90114-9
Boos A., Ehlers J., Roming L. G., Schwarz R. (1986). Progesterone concentration in uterine flushings from the ipsi- and contralateral uterine horn in relation to the corpus luteum periodicum and in blood serum in cattle. DTW Dtsch. Tierarztl Wochenschr. 93, 243–245.
Borges Á.M., Healey G. D., Sheldon I. M. (2012). Explants of intact endometrium to model bovine innate immunity and inflammation ex vivo. Am. J. Reprod. Immunol. 67 (6), 526–539. doi: 10.1111/j.1600-0897.2012.01106.x
Brayman M., Thathiah A., Carson D. D. (2004). MUC1: A multifunctional cell surface component of reproductive tissue epithelia. Reprod. Biol. Endocrinol. 2 (1), 4. doi: 10.1186/1477-7827-2-4
Carson D. D., Bagchi I., Dey S. K., Enders A. C., Fazleabas A. T., Lessey B. A., et al. (2000). Embryo Implantation. Dev. Bio. 223(2):217–237. doi: 10.1006/dbio.2000.9767
Cronin J. G., Turner M. L., Goetze L., Bryant C. E., Sheldon I. M. (2012). Toll-like receptor 4 and MYD88-dependent signaling mechanisms of the innate immune system are essential for the response to lipopolysaccharide by epithelial and stromal cells of the bovine endometrium. Biol. Reprod. 86 (2), 1–9. doi: 10.1095/biolreprod.111.092718
Cui L., Wang H., Lin J., Wang Y., Dong J., Li J., et al. (2020). Progesterone inhibits inflammatory response in E.coli- or LPS-Stimulated bovine endometrial epithelial cells by NF-κB and MAPK pathways. Dev. Comp. Immunol. 105:103568. doi: 10.1016/j.dci.2019.103568
Dadarwal D., Palmer C., Griebel P. (2017). Mucosal immunity of the postpartum bovine genital tract. Theriogenology 104, 62–71. doi: 10.1016/j.theriogenology.2017.08.010
Dimberg A. (2010). The chemokine system in experimental and clinical hematology. Curr. Top. Microbiol. Immunol. 341, 3–12. doi: 10.1007/82_2010_18
Ding X., Lv H., Deng L., Hu W., Peng Z., Yan C., et al. (2020). Analysis of Transcriptomic Changes in Bovine Endometrial Stromal Cells Treated With Lipopolysaccharide. Front. Vet. Sci. 7, 575865–575865. doi: 10.3389/fvets.2020.575865
Dohmen M. J. W., Joop K., Sturk A., Bols P. E. J., Lohuis J. A. C. M. (2000). Relationship between intra-uterine bacterial contamination, endotoxin levels and the development of endometritis in postpartum cows with dystocia or retained placenta. Theriogenology 54 (7), 1019–1032. doi: 10.1016/S0093-691X(00)00410-6
Dong H., Tang X., Zhang Z., Dong C. (2017). Structural insight into lipopolysaccharide transport from the gram-negative bacterial inner membrane to the outer membrane. Biochim. Biophys. Acta (BBA) Mol. Cell Biol. Lipids 1862 (11), 1461–1467. doi: 10.1016/j.bbalip.2017.08.003
Ealy A. D., Speckhart S. L., Wooldridge L. K. (2021). Cytokines that serve as embryokines in cattle. Animals 11 (8), 2313. doi: 10.3390/ani11082313
Elson G., Dunn-Siegrist I. N., Daubeuf B., Pugin J. R. (2006). Contribution of toll-like receptors to the innate immune response to gram-negative and gram-positive bacteria. Blood 109 (4), 1574–1583. doi: 10.1182/blood-2006-06-032961
Ford S. P., Chenault J. R., Echternkamp S. E. (1979). Uterine blood flow of cows during the oestrous cycle and early pregnancy: Effect of the conceptus on the uterine blood supply. J. Reprod. Fertil. 56(1), 53–62. doi: 10.1530/jrf.0.0560053
Fu Y., Liu B., Feng X., Liu Z., Liang D., Li F., et al. (2013). Lipopolysaccharide increases toll-like receptor 4 and downstream toll-like receptor signaling molecules expression in bovine endometrial epithelial cells. Vet. Immunol. Immunopathol. 151 (1), 20–27. doi: 10.1016/j.vetimm.2012.09.039
Healy L. L., Cronin J. G., Sheldon I. M. (2014). Endometrial cells sense and react to tissue damage during infection of the bovine endometrium via interleukin 1. Sci. Rep. 4 (1), 7060. doi: 10.1038/srep07060
Herath S., Fischer D. P., Werling D., Williams E. J., Lilly S. T., Dobson H., et al. (2006). Expression and function of toll-like receptor 4 in the endometrial cells of the uterus. Endocrinology 147 (1), 562–570. doi: 10.1210/en.2005-1113
Herath S., Lilly S. T., Santos N. R., Gilbert R. O., Goetze L., Bryant C. E., et al. (2009). Expression of genes associated with immunity in the endometrium of cattle with disparate postpartum uterine disease and fertility. Reprod. Biol. Endocrinol. 7 (1), 55. doi: 10.1186/1477-7827-7-55
Heumann D., Roger T. (2002). Initial responses to endotoxins and Gram-negative bacteria. Clinica Chimica Acta 323(1), 59–72. doi: 10.1016/S0009-8981(02)00180-8
Horne A. W., Lalani E. N., Margara R. A., Ryder T. A., Mobberley M. A., White J. O., et al. (2005). The expression pattern of MUC1 glycoforms and other biomarkers of endometrial receptivity in fertile and infertile women. Physiol. Endocrinol. 72(2), 216–229. doi: 10.1002/mrd.20307
Ireland J. J., Murphee R. L., Coulson P. B. (1980). Accuracy of predicting stages of bovine estrous cycle by gross appearance of the corpus luteum. J. Dairy Sci. 63 (1), 155–160. doi: 10.3168/jds.S0022-0302(80)82901-8
Jing H., Vassiliou J., Ganea D. (2003). Prostaglandin E2 inhibits production of the inflammatory chemokines CCL3 and CCL4 in dendritic cells. J. Leukocyte Biol. 74 (5), 868–879. doi: 10.1189/jlb.0303116
Kasimanickam R., Kasimanickam V., Kastelic J. P. (2014). Mucin 1 and cytokines mRNA in endometrium of dairy cows with postpartum uterine disease or repeat breeding. Theriogenol. 81(7), 952–958.e952. doi: 10.1016/j.theriogenology.2014.01.018
Kimmins S., MacLaren L. A. (2001). Oestrous cycle and pregnancy effects on the distribution of oestrogen and progesterone receptors in bovine endometrium. Placenta 22 (8), 742–748. doi: 10.1053/plac.2001.0708
Koh Y. Q., Mitchell M. D., Almughlliq F. B., Vaswani K., Peiris H. N. (2018). Regulation of inflammatory mediator expression in bovine endometrial cells: effects of lipopolysaccharide, interleukin 1 beta, and tumor necrosis factor alpha. Physiol. Rep. 6 (9), e13676. doi: 10.14814/phy2.13676
Krakowski L., Zdzisinska B. (2007). Selected cytokines and acute phase proteins in heifers during the ovarian cycle course and in different pregnancy periods. J. Bulletin-Veterinary Institute Pulawy 51 (1), 31.
Laguardia-Nascimento M., Branco K. M., Gasparini M. R., Giannattasio-Ferraz S., Leite L. R., Araujo F. M., et al. (2015). Vaginal microbiome characterization of nellore cattle using metagenomic analysis. PloS One 10 (11), e0143294. doi: 10.1371/journal.pone.0143294
LeBlanc S. J., Duffield T. F., Leslie K. E., Bateman K. G., Keefe G. P., Walton J. S., et al. (2002). Defining and diagnosing postpartum clinical endometritis and its impact on reproductive performance in dairy cows. J. Dairy Sci. 85 (9), 2223–2236. doi: 10.3168/jds.S0022-0302(02)74302-6
Li S., Khafipour E., Krause D. O., Kroeker A., Rodriguez-Lecompte J. C., Gozho G. N., et al. (2012). Effects of subacute ruminal acidosis challenges on fermentation and endotoxins in the rumen and hindgut of dairy cows. J. Dairy Sci. 95 (1), 294–303. doi: 10.3168/jds.2011-4447
Lu Y. C., Yeh W. C., Ohashi P. S. (2008). LPS/TLR4 signal transduction pathway. Cytokine 42 (2), 145–151. doi: 10.1016/j.cyto.2008.01.006
Mihelic R., Winter H., Powers J. B., Das S., Lamour K., Campagna S. R., et al. (2020). Genes controlling polyunsaturated fatty acid synthesis are developmentally regulated in broiler chicks. Br. Poult. Sci. 61 (5), 508–517. doi: 10.1080/00071668.2020.1759788
Miyamoto Y., Skarzynski D. J., Okuda K. (2000). Is tumor necrosis factor α a trigger for the initiation of endometrial prostaglandin F2α release at luteolysis in cattle? Biol. Reprod. 62 (5), 1109–1115. doi: 10.1095/biolreprod62.5.1109
Monteiro H. F., Faciola A. P. (2020). Ruminal acidosis, bacterial changes, and lipopolysaccharides. J. Anim. Sci. 98 (8), 1–9. doi: 10.1093/jas/skaa248
Moore S. G., Ericsson A. C., Poock S. E., Melendez P., Lucy M. C. (2017). Hot topic: 16S rRNA gene sequencing reveals the microbiome of the virgin and pregnant bovine uterus. J. Dairy Sci. 100 (6), 4953–4960. doi: 10.3168/jds.2017-12592
Nagaraja T. G., Bartley E. E., Fina L. R., Anthony H. D. (1978). Relationship of rumen gram-negative bacteria and free endotoxin to lactic acidosis in cattle. J. Anim. Sci. 47 (6), 1329–1337. doi: 10.2527/jas1978.4761329x
Oguejiofor C. F., Cheng Z., Abudureyimu A., Fouladi-Nashta A. A., Wathes D. C. (2015). Global transcriptomic profiling of bovine endometrial immune response in vitro. i. Effect of lipopolysaccharide on innate immunity. Biol. Reprod. 93 (4), 1–13. doi: 10.1095/biolreprod.115.128868
Okumu L. A., Forde N., Fahey A. G., Fitzpatrick E., Roche J. F., Crowe M. A., et al. (2010). The effect of elevated progesterone and pregnancy status on mRNA expression and localisation of progesterone and oestrogen receptors in the bovine uterus. J. Reprod. 140 (1), 143–153. doi: 10.1530/rep-10-0113
Oliveira L. J., Mansourri-Attia N., Fahey A. G., Browne J., Forde N., Roche J. F., et al. (2013). Characterization of the Th profile of the bovine endometrium during the oestrous cycle and early pregnancy. PloS One 8 (10), 1–13. doi: 10.1371/journal.pone.0075571
Penny L. A., Armstrong D., Bramley T. A., Webb R., Collins R. A., Watson E. D. (1999). Immune cells and cytokine production in the bovine corpus luteum throughout the oestrous cycle and after induced luteolysis. J. Reprod. 115 (1), 87–96. doi: 10.1530/jrf.0.1150087
Poole R. K., Ault-Seay T. B., Payton R. R., Myer P. R., Lear A. S., Pohler K. G. (2021). Evaluation of reproductive tract cytokines in post-partum beef cows relating to reproductive microbiota and fertility outcomes. Front. Anim. Sci. 2 (20). doi: 10.3389/fanim.2021.704714
Pope W. F., Maurer R. R., Stormshak F. (1982). Distribution of progesterone in the uterus, broad ligament, and uterine arteries of beef cows. Anat. Rec. 203 (2), 245–249. doi: 10.1002/ar.1092030206
Quereda J. J., Barba M., Mocé M. L., Gomis J., Jiménez-Trigos E., García-Muñoz Á., et al. (2020). Vaginal microbiota changes during estrous cycle in dairy heifers. Front. Vet. Res. 7 (371). doi: 10.3389/fvets.2020.00371
Raghupathy R. (2001). Pregnancy: success and failure within the Th1/Th2/Th3 paradigm. Semin. Immunol. 13 (4), 219–227. doi: 10.1006/smim.2001.0316
Ribeiro E. S., Lima F. S., Greco L. F., Bisinotto R. S., Monteiro A. P. A., Favoreto M., et al. (2013). Prevalence of periparturient diseases and effects on fertility of seasonally calving grazing dairy cows supplemented with concentrates. J. Dairy Sci. 96 (9), 5682–5697. doi: 10.3168/jds.2012-6335
Robinson R., Mann G., Lamming G., Wathes D. (2001). Expression of oxytocin, oestrogen and progesterone receptors in uterine biopsy samples throughout the oestrous cycle and early pregnancy in cows. J. Reprod. 122 (6), 965–979. doi: 10.1530/rep.0.1220965
Sánchez J. M., Passaro C., Forde N., Browne J. A., Behura S. K., Fernández-Fuertes B., et al. (2018). Do differences in the endometrial transcriptome between uterine horns ipsilateral and contralateral to the corpus luteum influence conceptus growth to day 14 in cattle? Biol. Reprod. 100 (1), 86–100. doi: 10.1093/biolre/ioy185
Sando L., Pearson R., Gray C., Parker P., Hawken R., Thomson P. C., et al. (2009). Bovine Muc1 is a highly polymorphic gene encoding an extensively glycosylated mucin that binds bacteria. J. Dairy Sci. 92 (10), 5276–5291. doi: 10.3168/jds.2009-2216
Schjenken J. E., Tolosa J. M., Paul J. W., Clifton V. L., Smith R. (2012). Mechanisms of maternal immune tolerance during pregnancy. Recent Adv. Res. Hum. Placenta 11, 211–242.
Sheldon I., Price S., Cronin J., Gilbert R., Gadsby J. (2009). Mechanisms of infertility associated with clinical and subclinical endometritis in high producing dairy cattle. Reprod. Domest. Anim. 44 (s3), 1–9. doi: 10.1111/j.1439-0531.2009.01465.x
Simón C., Moreno C., Remohí J., Pellicer A. (1998). Cytokines and embryo implantation. paper presented at the workshop on paracrine mechanisms in female reproduction, Seville, Spain, June 1997.1. J. Reprod. Immunol. 39 (1), 117–131. doi: 10.1016/S0165-0378(98)00017-5
Spencer T. E., Johnson G. A., Bazer F. W., Burghardt R. C., Palmarini M. (2006). Pregnancy recognition and conceptus implantation in domestic ruminants: roles of progesterone, interferons and endogenous retroviruses. J. Reprod. Fertil. Dev. 19(1), 65–78. doi: 10.1071/RD06102
Swangchan-Uthai T., Lavender C. R. M., Cheng Z., Fouladi-Nashta A. A., Wathes D. C. (2012). Time course of defense mechanisms in bovine endometrium in response to lipopolysaccharide. Biol. Reprod. 87 (6), 1–13. doi: 10.1095/biolreprod.112.102376
Takahashi H., Haneda S., Kayano M., Matsui M. (2016). Differences in progesterone concentrations and mRNA expressions of progesterone receptors in bovine endometrial tissue between the uterine horns ipsilateral and contralateral to the corpus luteum. J. Vet. Med. Sci. 78 (4), 613–618. doi: 10.1292/jvms.15-0366
Takeuchi O., Hoshino K., Kawai T., Sanjo H., Takada H., Ogawa T., Takeda K., and Akira S. (1999). Differential roles of TLR2 and TLR4 in recognition of gram-negative and gram-positive bacterial cell wall components. Immunity 11 (4), 443–451. doi: 10.1016/S1074-7613(00)80119-3
Weems C., Lee C., Weems Y., Vincent D. L. (1988). Distribution of progesterone to the uterus and associated vasculature of cattle. Endocrinol. Japan 35 (4), 625–630. doi: 10.1507/endocrj1954.35.625
Zhang S., Liu B., Mao W., Li Q., Fu C., Zhang N., et al. (2017). The effect of prostaglandin E2 receptor (PTGER2) activation on growth factor expression and cell proliferation in bovine endometrial explants. Prostaglandins Leukot. Essent. Fatty Acids 122, 16–23. doi: 10.1016/j.plefa.2017.06.012
Keywords: bovine, follicular, lipopolysaccharide, luteal, uterus, bacteria, gram-negative, cytokine
Citation: Ault-Seay TB, Payton RR, Moorey SE, Pohler KG, Schrick FN, Shepherd EA, Voy BH, Lamour KH, Mathew DJ, Myer PR and McLean KJ (2022) Endometrial gene expression in response to lipopolysaccharide between estrous cycle phases and uterine horns in cattle. Front. Anim. Sci. 3:939876. doi: 10.3389/fanim.2022.939876
Received: 09 May 2022; Accepted: 29 June 2022;
Published: 27 July 2022.
Edited by:
Mohan Mondal, Eastern Regional Station, Kalyani, IndiaReviewed by:
Cristiane Gonçalves Titto, University of São Paulo, BrazilM Karunakaran, National Dairy Research Institute (ICAR), India
Nishant Kumar, Indian Council of Agricultural Research (ICAR), India
Copyright © 2022 Ault-Seay, Payton, Moorey, Pohler, Schrick, Shepherd, Voy, Lamour, Mathew, Myer and McLean. This is an open-access article distributed under the terms of the Creative Commons Attribution License (CC BY). The use, distribution or reproduction in other forums is permitted, provided the original author(s) and the copyright owner(s) are credited and that the original publication in this journal is cited, in accordance with accepted academic practice. No use, distribution or reproduction is permitted which does not comply with these terms.
*Correspondence: Kyle J. McLean, a21jbGVhMTBAdXRrLmVkdQ==; Phillip R. Myer, cG15ZXJAdXRrLmVkdQ==