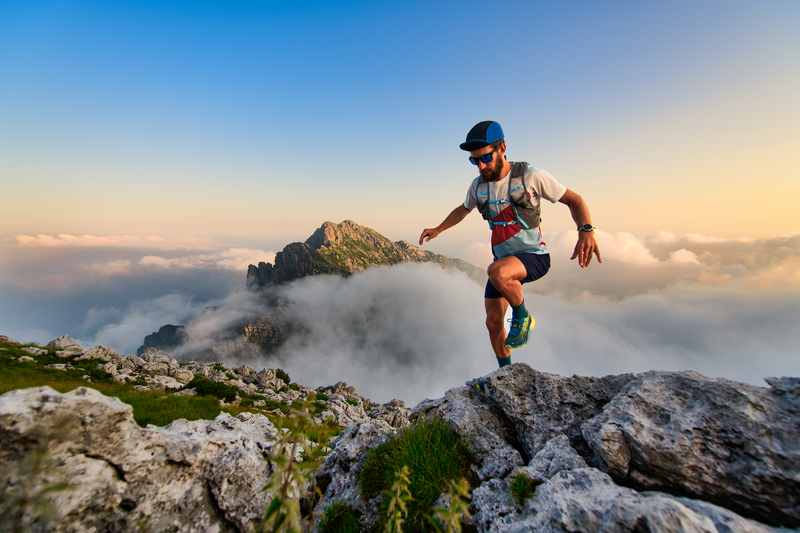
95% of researchers rate our articles as excellent or good
Learn more about the work of our research integrity team to safeguard the quality of each article we publish.
Find out more
REVIEW article
Front. Anim. Sci. , 13 April 2022
Sec. Animal Physiology and Management
Volume 3 - 2022 | https://doi.org/10.3389/fanim.2022.851574
Among the air-breathing vertebrates, regarding respiratory efficiency, the avian respiratory system rests at the evolutionary zenith. Structurally, it is separated into a lung that serves as a gas exchanger and air sacs that mechanically ventilate the lung continuously and unidirectionally in a caudocranial direction. Largely avascular, the air sacs are delicate, transparent, compliant and capacious air-filled spaces that are not meaningfully involved in gas exchange. The avian lungs are deeply and firmly attached to the vertebrae and the ribs on the dorsolateral aspects, rendering them practically rigid and inflexible. The attachment of the lung to the body wall allowed extreme subdivision of the exchange tissue into minuscule and stable terminal respiratory units, the air capillaries. The process generated a large respiratory surface area in small lungs with low volume density of gas exchange tissue. For the respiratory structures, invariably, thin blood-gas barrier, large respiratory surface area and large pulmonary capillary blood volume are the foremost adaptive structural features that confer large total pulmonary morphometric diffusing capacities of O2. At parabronchial level, the construction and the arrangement of the airway- and the vascular components of the avian lung determine the delivery, the presentation and the exposure of inspired air to capillary blood across the blood-gas barrier. In the avian lung, crosscurrent-, countercurrent- and multicapillary serial arterialization systems that stem from the organization of the structural parts of the lung promote gas exchange. The exceptional respiratory efficiency of the avian respiratory system stems from synergy of morphological properties and physiological processes, means by which O2 uptake is optimized and high metabolic states and capacities supported. Given that among the extant animal taxa insects, birds and bats (which accomplished volancy chronologically in that order) possess structurally much different respiratory systems, the avian respiratory system was by no means a prerequisite for evolution of powered flight but was but one of the adaptive solutions to realization of an exceptionally efficient mode of locomotion.
Birds are a highly conspicuous and profoundly fascinating animal taxon (Gould and Gould, 2007; Robbins, 2017; BirdLife nternational, 2018). Important as a source of food (Rude, 2016), they have had a close historical association with human beings (Hill, 2019; Kost, 2019; Kost and Hussain, 2019) and have greatly socio-culturally influenced past civilizations from as far back as ~3 million years ago (Spottiswoode et al., 2016). Bird watching (ornithology) is an “old” human pursuit that continues to gain popularity in the modern times (Cordell et al., 1999; Cordell and Herbert, 2002). Displaying one of the greatest evolutionary adaptive radiations known in animal life (Cooney et al., 2017), birds comprise more than 10,000 species (Brusatte et al., 2015) and possibly as many as 20,470 ones (Barrowclough et al., 2016). With a global distribution, birds have and continue to greatly impact on the ecosystems they occupy (Tabur and Ayvaz, 2010; Garfinkel et al., 2020). In the remotest parts of Earth, new species of birds continue to be discovered (Barnett and Buzzetti, 2014). While some species of birds are weak flyers and others have lost flight altogether, all of them originated from volant progenitors (Welty and Baptista, 1988; Benton, 2014). Birds are particularly sensitive to adverse changes in environmental conditions (Morrison, 1986; Hollamby et al., 2006; Morelli et al., 2014; Alexandrino et al., 2016). Because they can easily physically vacate injurious habitats, birds are reliable bioindicator- or sentinel animals of environmental health (Amat and Green, 2010; Egwumah et al., 2017; Mekonen, 2017). The sensitivity of birds to environmental perturbations (Llacuna et al., 1993; Mutua et al., 2016) have been attributed to the structural complexity and the exceptional efficiency of the avian respiratory system (King, 1966; Duncker, 1971; Scheid, 1979; Fedde, 1980; McLelland, 1989; Maina, 2005). Air pollution causes respiratory distress and sickness in birds, alters their lifestyle, increases stress levels, leads to immunosuppression and drastically reduces reproductive success (Sanderfoot and Holloway, 2017). In ecotopes, specific diversity and numerical abundance are instructive signatures of environmental integrity (Stolen et al., 2005; Wade et al., 2014; Sanderfoot and Holloway, 2017). Suggesting worsening global environmental conditions, as many as 1,313 (~13%) of the extant bird species are listed on the International Union for Conservation of Nature (IUCN) Red List of threatened species (IUCN, 2020). In North America, during the last half-century, a net loss of abundance of birds as high as 29% (of the 1970 abundance), a value that translates into an overall loss of ~3 billion birds, was reported by Rosenberg et al. (2019) and since 1980, the European population of turtle doves (Streptopelia turtur) has declined by ~80% (De Vries et al., 2021). Global seasonal changes of temperature has greatly affected the geographical distribution and the migratory patterns of birds (Visser et al., 2009; Clairbaux et al., 2019; Horton et al., 2020). The speeds at which they travel, the migratory distances they cover and the routes they follow have considerably changed (Jonzén et al., 2006; Schaefer et al., 2008; Covino et al., 2020). Interestingly, higher environmental temperatures have compelled birds to expend more energy on physiological processes such as thermoregulation, making their bodies smaller and wingspans larger (Weeks et al., 2019). Many species of birds have and continue to be instructively used in biological studies (Konishi et al., 1989; Zink, 2012). The insights gained have meaningfully contributed to conservation endeavors (Higuchi, 2012). The anthropogenic activities that most adversely affect birds have been identified and means by which they can be mitigated formulated (Reichert et al., 2012).
Among the air-breathing vertebrates, the avian respiratory system, the lung-air sac system, is structurally and functionally unique. It is separated into a lung that serves as a gas exchanger and air sacs that serve as mechanical ventilators (King, 1966; Duncker, 1971; McLelland, 1989; Powell and Hopkins, 2004; Maina, 2005; Powell, 2015, 2022; Lawson et al., 2020). The biological aspects of the avian respiratory system have been studied since about five centuries ago (Coitier, 1573): subsequent to that time, it has been unremittingly investigated (McLelland, 1989). Some of the findings have been controversial while some aspects remain unknown (Maina, 2017a). A state that does not appear to have changed in the present times, Donald Farner (Farner, 1970) ranked the avian respiratory system among the most controversial organ systems of his time. Literature reviews that inform on the advances made while highlighting aspects that require further investigation are critical to advancing scientific knowledge. This succinct treatise outlines the structure and function of the avian respiratory system. It underscores and critically synthesizes the current understanding of the biology of the avian respiratory system while endeavoring to place it in its proper place in the broad range of the evolved vertebrate gas exchangers (Maina, 1998, 2011).
The avian lungs are small, compact, wedge-shaped structures that are firmly attached to the vertebrae and ribs on the dorsolateral aspects in the coelomic cavity (King, 1966; Duncker, 1971; King and McLelland, 1984; McLelland, 1989; Maina, 2005, 2015a,b, 2017b) [Figures (1–4)]: as much as one-third of the volume of the avian lung is held tightly between the boney structures (King and Molony, 1971). The airway (bronchial) system comprises a complex three-tiered assemblage that consists of the intrapulmonary primary bronchus, secondary bronchi and parabronchi (tertiary bronchi) (Duncker, 1971; McLelland, 1989; Maina, 2005) [Figures (5,6)]. As the intrapulmonary primary bronchus transits the lung, from the various aspects of its luminal circumference (surface), it originates the medioventral secondary bronchi, the mediodorsal secondary bronchi, the lateroventral secondary bronchi and the laterodorsal secondary bronchi. The secondary bronchi are named according to the part of the lung they deliver air to King (1966); Duncker (1971); King and McLelland (1984); McLelland (1989), and Maina (2005). The parabronchi comprise a parabronchial lumen that is surrounded by an exchange tissue mantle [Figure (7)]. Atria project outwards from the parabronchial lumen [Figure (7)] and give rise to infundibula [Figures (8,9)] that in turn originate air capillaries [Figure (9)]. The air- and the blood capillaries are the terminal respiratory units of the avian lung [Figures (10–15)]. They constitute as much ~80% of the volume of the exchange tissue (Maina, 1982a, 1989, 2005; Maina et al., 1989) [Figures (10–13)]. The air capillaries range in diameter from ~3 μm in the lungs of small species of birds (Maina, 1982a, 1989, 2005), 10 μm in penguins (Spheniscidae), swans (Anatidae) and the coot (Fulica atra) (Duncker, 1972) to ~20 μm in the ostrich one (Struthio camelus) (Maina and Nathaniel, 2001). For mammals, the smallest alveoli (diameters of ~50 μm) exist in the bat- and the shrew lungs (Tenney and Remmers, 1963). While for the mammalian lung, the alveolar blood capillaries are exposed to air on the two sides of the interalveolar septum (Maina, 1987, 1990, 2015c). In the avian lung, the blood capillaries are exposed to air about all around their boundaries (Maina, 2015a,b, 2017a,b) [Figures (14,15)]: the design increases the respiratory surface area. Like for the mammalian lung (Weibel, 1973), the blood-gas barrier of the avian lung comprises an epithelial cell that is covered by a surfactant lining, a basement membrane and an endothelial cell (Maina and King, 1982; Maina and West, 2005) [Figures (15), (16)]. The air capillaries are rotund structures that interconnect via narrow passageways [Figures (10,11)] while the blood capillaries comprise conspicuous spatially connected sections that are about as long as they are wide (Woodward and Maina, 2005, 2008; Maina and Woodward, 2009) [Figures (12,13)].
Figure 1. Except for (2), that comes from an ostrich (Struthio camelus), all the figures are from or are prepared based on the lung of the domestic fowl (Gallus gallus variant domesticus). (1,2) The wedge-shaped lungs (Ln) showing costovertebral sulci (*). Tr, trachea; EPPB, extrapulmonary primary bronchus. (3) Lung (Ln) firmly attached to the ribs at the costovertebral sulci (*) and to the horizontal septum (arrows). *, costal sulci; VC, vertebral column. (4) Deep costovertebral impressions (*). Circles, parabronchi. (5,6) Medial view of a latex cast preparation of the lung (5) and a drawing of the lung (as if transparent) (6) showing the complexity of the airway system. EPPB, extrapulmonary primary bronchus; MVSB, medioventral secondary bronchi; LVSB, lateral ventral secondary bronchi; MDSB, mediodorsal secondary bronchi; Pb, parabronchi; dots, ostia. (7) Parabronchial lumen (PL) and exchange tissue mantle (ET) of a parabronchus. Arrows, atria. (8) An atrium giving rise to infundibula (If). Insert: If, infundibulum. (9) Edge of a parabronchial lumen showing atrial muscle (AM) and atria (At). If, infundibula; *, air capillaries. (10,11) A three-dimensional reconstruction seral section reconstruction (10) and a latex rubber cast preparation (11) of air capillaries (AC). Circles, narrow interconnecting passageways. (12,13) Critical point dried preparation (12) and latex rubber cast preparation (13) showing blood capillaries (BC) that intertwine closely with air capillaries (AC). Er, erythrocytes. (14) Networking of the air capillaries (AC) and the blood capillaries (BC). Arrows, blood-gas barrier; circles, epithelial-epithelial cell connections; boxed areas, sites where blood capillaries connect directly. Er, erythrocytes; *, epithelial cell. (15) Exposure of a blood capillary (BC) across the blood-gas barrier (arrows). Er, erythrocytes; BC, blood capillaries; AC, air capillaries; circles, epithelial-epithelial connections of the blood capillaries; EC, endothelial cell. Insert: An air capillary (*) that is wholly surrounded by blood capillaries that contain erythrocytes (*).
Figure 2. The figures are from or based on the lung of the domestic fowl (Gallus gallus variant domesticus). (16) Blood-gas barrier that comprises an epithelial cell (arrows) that is covered by surfactant (thin black line), basement membrane (*) and endothelial cell (En). Er, erythrocyte; *, plasma layer. (17,18) Medial- (17) and lateral (18) views of three-dimensional serial section computer reconstruction of the lung showing the complexity of the airway system. MVSB, medioventral secondary bronchi; MDSB, mediodorsal secondary bronchi; PB, primary bronchus, PP, paleopulmonic parabronchi; NP, neopulmonic parabronchi. (19) Schematic diagram showing airflow through the lung. Inspired air flows through the intrapulmonary primary bronchus (IPPB) and a smaller volume passes through the neopulmonic parabronchi (white arrows). The expired air is directed into the paleopulmonic parabronchi (yellow arrows) via the mediodorsal secondary bronchi (MDSB) where the flow is continuous and unidirectional in a caudocranial direction (long dashed red arrows). Tr, trachea; MVSB, medioventral secondary bronchi; circles, ostia. Air sacs: (i), cervical-; (ii), clavicular-; (iii), cranial-thoracic-; (iv), caudal thoracic- and (v), abdominal-. Modified from McLelland (1989). Insert: Dorsal view of a latex rubber cast preparation of the lung showing paleopulmonic parabronchi (PP) interconnecting the medioventral secondary bronchi on the medial side (*) and medioventral secondary bronchi on the lateral side of the lung (*).
Most avian lungs are markedly morphologically heterogeneous. In such cases, they comprise two structurally- and functionally different parts, namely the paleopulmo (“old lung”) and the neopulmo (“new lung”) (Duncker, 1971): respectively, the former is largely found on the dorsolateral aspect of the lung while the latter is largely located on the ventromedial one respectively [Figures (17,18)]. The paleopulmo largely consists of the parabronchi that connect the mediodorsal secondary bronchi to the medioventral secondary bronchi [Figures (19)–insert) while the neopulmo is formed by the parabronchi that mainly join the mediodorsal secondary bronchi, the lateroventral secondary bronchi and the laterodorsal secondary bronchi (King and McLelland, 1984; McLelland, 1989). While the paleopulmo exists in lungs of all species of birds, the neopulmo is poorly developed or totally lacking in those of the less-derived species such as the emu, the penguins and the kiwi (Apteryx) (Duncker, 1971; King and McLelland, 1984; McLelland, 1989). While the basis of separating the avian lung into paleopulmonic and neopulmonic parts continues to be challenged and debated and its evolutionary significance, if any, questioned (King and McLelland, 1984; López, 1995; Maina, 2003a,b, 2005, 2017a; Moura and Correia-Pinto, 2017), the consideration helped resolve the question why the CO2 concentration is higher in the inspired air reaching the caudal air sacs compared with the atmospheric one, a condition that puzzled avian physiologists for a long time (Scheid, 1979; Fedde, 1980, 1998; Scheid and Piiper, 1989; Jaensch, 2015). Before the identification of the neopulmonic part of the avian lung, it was believed that the inspired air reached the caudal air sacs directly through the primary bronchus [Figures (5,6)]. It was hence problematical to explain the difference between the CO2 concentrations in the two volumes of air, i.e., the inspired air and air in the caudal air sacs. It is now acknowledged that in lungs of species of birds where a neopulmo exists, some of the inspired air passes through it (neopulmo) where it collects CO2 in the exchange tissue (Piiper, 1978) [Figure (19)]. In penguin lungs that lack a neopulmo, interestingly, gas tension measurements showed higher partial pressure of CO2 in the caudal air sacs (Powell and Hempleman, 1985): it was suggested that the feature may be explained by a possibility that airflow in all the paleopulmonic parabronchi might not occur in a caudocranial direction.
While differences in topographical location, size and arrangement exist between the paleopulmonic- and the neopulmonic parts of the avian lung, no morphometric variations occur between the structural components of the exchange tissue (Maina, 1982b; Maina et al., 1983). Jones (1982) suggested that the neopulmonic parabronchi that are tidally ventilated, may serve as reservoirs of CO2 and hence minimize elimination of CO2 from the exchange tissue, averting respiratory alkalosis. The ostrich can pant continuously for 8 h without suffering respiratory alkalosis (Schmidt-Nielsen et al., 1969). In spontaneously breathing unanesthetized ducks, Holle et al. (1977) observed that the neopulmo was relatively better perfused with blood compared with the entire lung and together with the paleopulmonic parabonchi, blood flow decreased in the direction of the ventilatory gas flow, lowering the partial pressure of O2 and increasing that of CO2 in the lung gas. Without providing experimental evidence, Duncker (1972) speculated that where the neopulmo is well-developed, it may serve as the most important site of gas exchange or it may even be the exclusive site of gas exchange during rest and that the paleopulmo may only significantly participate in gas exchange during exercise. With the development of the neopulmonic parabronchi, consequent reduction of airflow through the intrapulmonary primary bronchus might explain the significant decrease in the distal luminal diameter of the airway Duncker (1972).
The air sacs are capacious, delicate and transparent air chambers that connect to the lung at sites termed ostia that are located on the cranial-, ventral- and distal edges of the lung [Figures (5,6), (19)]. The air sacs constitute the largest part of the total volume of the avian respiratory system (Jürgens and Gros, 2002; Jaensch, 2015). Variable in size and location, they comprise the cervical-, the interclavicular-, the craniothoracic-, the caudothoracic- and the abdominal air sacs (King and McLelland, 1984; McLelland, 1989; Maina, 2005). Functionally, the first three air sacs constitute the cranial group while the last two form the caudal one [Figure (19)]. In the domestic fowl, Cook et al. (1986) observed that the ostium of the abdominal air sacs had a sphincteric ring with well-innervated smooth muscle tissue. In clinical veterinary medicine, the air sacs are used for radiographic differentiation and identification of the internal organs during endoscopy. Also, they provide an alternative route for administration of gaseous anesthesia (Bennett, 2009; Jaensch, 2015). Because the air sacs extend out of the coelomic cavity, with some of them coming to lie subcutaneously (Bezuidenhout et al., 1999), they are susceptible to damage from trauma. Infections of air sacs (air sacculitis) (Clippinger, 1997) can rapidly spread to the lungs. Accidental rupture of air sacs commonly occurs after incidents such as when a bird strikes a transparent window pane in flight. The pathology presents as abnormal swelling of a part of the body (Petevinos, 2006; Kamani et al., 2009; Speer, 2016; Hollinger, 2017). It can be relieved by aspiration of air under sterile conditions or by surgical intervention (Clippinger, 1997; Jung, 2001; Browning et al., 2019).
Interestingly, in birds, some air sacs such as the cervicocephalic one that originates from infraorbital sinuses and some that are largely located under the skin have no direct connection with lungs or lung-associated air sacs (Akester et al., 1973): they are better developed in strongly volant birds (Jaensch, 2015). The subcutaneous air sacs of the pelicans that are among the largest flying birds (Harrison, 1991) serve as shock absorbers when the bird it strikes the surface of the water during a dive and thereafter aid in remaining afloat (Redrobe, 2015). With variations between species, air sacs pneumatize proximate avian bones (King and Kelly, 1956; King, 1966; Hogg, 1984; McLelland, 1989; O'Connor, 2004; Schachner et al., 2021). The historic suggestion that air sacs reduced the overall weight of birds and hence permits them to fly is fallacious. No correlation has been found between flight efficiency and endurance with extent of air sacs development: some of the flightless species of birds have well-developed air sacs. The internal surface of the air sacs is lined by a simple squamous epithelium that overlies a connective tissue substratum (Carlson and Beggs, 1973; Walsh and McLelland, 1974; Bezuidenhout, 2005; Jaensch, 2015). Near the ostia, isolated sites that are covered by ciliated cuboidal- and columnar epithelia exist (Fletcher, 1980). In diving species, the walls of the air sacs are thicker and a columnar epithelium preponderates (Jaensch, 2015). Largely avascular (Fletcher, 1980), the air sacs do not significantly participate in gas exchange (Magnussen et al., 1976; Powell and Hempleman, 1985). The simple experiment performed by Soum (1896) is often cited (e.g., Schmidt-Nielsen, 1983, p. 48–49) in support of this observation: after the ostial connection to the large abdominal air sacs was closed-off (plugged) and carbon monoxide (CO) introduced into the air sac, it was noted that, despite birds being highly sensitive to CO poisoning (Schmidt-Nielsen, 1983; Reiblein, 2017), the animals did not suffer any discomfort from the lethal, tasteless, odorless and combustible gas. In synchronized bellows-like manner, the cranial- and the caudal groups of air sacs [Figures (19), (20–23)] ventilate the lung (specifically the paleopulmonic parabronchi) continuously in a caudocranial direction (Scheid, 1979; Fedde, 1980).
Figure 3. (20–23) Schematic diagrams showing air flow through the lung-air sac system of birds. (20) A given volume of inspired air flows directly through the intrapulmonary primary bronchus and indirectly across the neopulmonic parabronchi (dashed arrows) to the caudal air sacs during the first inspiratory cycle. *, location where inspiratory aerodynamic valving occurs. Insert: Lateral view of a latex rubber cast of the lung-air sac system of the domestic fowl showing air sacs (*) and the parts of the respiratory system that comprise the lung, the cranial air sacs and the caudal air sacs (dashed cyan lines). (21) The given volume of air flows from the caudal air sacs into the paleopulmonic parabronchi of the lung during the first expiratory cycle. *, location where expiratory aerodynamic valving occurs. Insert: Dorsal view of a latex rubber cast of the lung-air sac system of the domestic fowl showing air sacs (*) and the parts of the respiratory system that comprise the lung, the cranial air sacs and the caudal air sacs (dashed cyan lines). (22) The given volume of air moves from the lung into the cranial air sacs during the second inspiratory cycle. Insert: View of the abdominal air sac of a developing chick lung. (23) The given volume of inspired air moves from the cranial air sacs to the outside during the second expiratory cycle. Ln, lungs; circles, ostia; parallel arrows (21,22), ventilation of the paleopulmonic parabronchi that is continuous and unidirectional in a caudocranial direction. (24) Lateral view of a fresh (unfixed) lung of the domestic fowl (Gallus gallus variant domesticus) that has been removed from the body attachments–its shape and size have been well-retained. *, costovertebral sulci. (25,26) Histological preparations of the lung of the ostrich (Struthio camelus) showing crosscurrent- and countercurrent gas exchange systems: the former comprises the air flow through the parabronchial lumen (open) arrow and venous blood from the periphery of the parabronchus (red arrow) while the later comprises outward diffusion of O2 in the air capillaries (lime colored arrow) and the flow of venous blood from the periphery (red arrow). IPBV, interparabronchial artery; *, intraparabronchial artery; ET, exchange tissue; PL, parabronchial lumen; At, atria; *, atrial muscles.
Because like the mammalian lung it developments by invagination from the primitive pharynx (Merkus et al., 1996; Burri, 1999; Maina, 2003a,b, 2006; Kimura and Deutsch, 2007; Schittny, 2017), on the whole, the lung-air sac system of birds is tidally ventilated. However. compared with the simple and direct in-and-out, i.e., tidal, process that occurs in the mammalian lung, the flow of air in the avian respiratory system is more complicated. It takes two full respiratory cycles, i.e., two inspiratory- and two expiratory cycles, for a given volume of inspired air to move through the lung-air sac [Figures (20–23)]. During the first inspiratory cycle, the air moves from outside to the caudal air sacs [Figure (20)]; in the first expiratory cycle, the air moves from the caudal air sacs into the lung [Figure (21)]; during the second inspiratory cycle, the air is shoved from the lung into the cranial air sacs [Figure (22)]; and in the second expiratory cycle, it moves from the cranial air sacs to the outside [Figure (23)]. The paleopulmonic parabronchi of the avian lung are continuously and unidirectionally ventilated in a caudocranial direction by synchronized activities of the air sacs (Scheid, 1979; Fedde, 1980) [Figures (21–23)].
Birds lack an anatomical structure which is structurally- and functionally analogous to the muscular diaphragm of mammals (King and McLelland, 1984; McLelland, 1989). The avian respiratory system is located in the coelom, a continuous space that is surrounded by a skeletomuscular body wall (Brackenbury, 1972; King and McLelland, 1984; McLelland, 1989). In birds, the liver and not lungs (as is the case for mammals) surrounds the heart. The ventral aspect of the avian lung attaches to the horizontal septum [Figure (3)], a connective tissue band of which tension is controlled by the costoseptal muscles (King and McLelland, 1984; McLelland, 1989). However, the septum does not alter the size and the shape of the lung (Fedde et al., 1964a,b). The uncinate processes that are associated with the ribs exist in most species of birds except for groups such as screamers (Anhimidae) (McLelland, 1989; Fowler and Cubas, 2001): the structures are involved in the ventilation of the lung (Welty, 1979; Codd et al., 2005; Tickle et al., 2007, 2009; Tickle and Codd, 2009; Codd, 2010; Tickle, 2010). By strengthening the rib cage, the uncinate processes enable it to tolerate the forces generated by the movements of the wings (Kardong, 1988). The sizes of the uncinate processes vary between species of birds and correlate with their lifestyles (Tickle et al., 2007; Tickle and Codd, 2009; Codd, 2010): the structures are small in nonvolant birds, long in diving species and intermediate in size in non-specialist ones.
The secure attachment of the avian lung to the ribs and the vertebrae [Figures (1–4)] has rendered it practically rigid and inflexible. During a respiratory cycle, the volume of the avian lung changes by a mere 1.4% (Jones et al., 1985). From a supposed in-built self-stabilizing system (a tensegrity system) (Maina, 2007a), the avian lung well-maintains its size and shape even after it is removed from its attachment to the body wall [Figure (24)]: a well-developed collagen-, smooth muscle- and elastic fiber scaffold (Maina et al., 2010) may generate a tensegrity system. Other structural features/properties that may also constrain volume changes include: (a) complete fusion of the parietal- and visceral pleurae with obliteration of the pleural cavity (King and McLelland, 1984); (b) location of the lung mainly at the craniodorsal part of the coelomic cavity (Inserts: Figures (20,21)], the part of the body where the skeletal system is most stable (Duncker, 1971); and (c) activities of the costoseptal muscles that counteract compression of the lung during expiration (Fedde et al., 1964a; Mackelprang and Goller, 2013).
The firm “fixation” of the avian lung to the body wall [Figures (1–4), (20,21)-inserts) greatly determined its function. Extreme subdivision of the exchange tissue into minuscular terminal respiratory units (air capillaries) could only occur in a strong and rigid lung. The intense internal compartmentalization (subdivision) of the exchange tissue of the avian lung generated large respiratory surface area in a small lung (Maina, 1989, 1994, 1998, 2000a, 2002, 2011; Maina et al., 1989). From their extremely small sizes (Duncker, 1971, 1972, 1974; Maina, 1982a; Maina and Nathaniel, 2001; Woodward and Maina, 2005, 2008; Maina and Woodward, 2009) and hence high mean surface curvature, all other factors constant, the surface tension forces that prevail on the surface of the air capillaries should be relatively greater compared with those of other vertebrate lungs. Theoretically, if the avian lung was compliant (like the mammalian one), from the envisaged high surface tension forces, very large physical forces would be needed to dilate (open) the air capillaries to take in inspired air and under steady state, the respiratory units would be highly unstable. Paradoxically, albeit their minuscular sizes, the air capillaries and the blood capillaries are very strong (Macklem et al., 1979; Powell et al., 1985; West et al., 2006, 2007a,b,c, 2010; Maina, 2007a,b; Watson et al., 2008; Jimoh and Maina, 2013). Existence of type-IV collagen in the blood-gas barrier and the epithelial-epithelial connections (Jimoh and Maina, 2013) as well as the morphologies of the air capillaries and the blood capillaries foremost account for the strengths of the respiratory units (Maina, 1982a, 1988; West et al., 2006). The complex assemblage of the air- and the blood capillaries [Figures (12–14)] was termed “honeycomb-like arrangement” by West et al. (2006).
In the lung, the delivery, the presentation and the exposure of deoxygenated blood to the inspired air considerably determine gas exchange efficiency. For the avian lung, at the parabronchial level, three main functional designs exist. These are: (a) a crosscurrent gas exchange system that is formed between the inwards (centripetal) flow of deoxygenated blood relative to the flow of air in the parabronchial lumen: the directions are orthogonal (perpendicular) relative to each other [Figures (25,26), (27–31)]; (b) a countercurrent gas exchange system that comprises the inwards flow of deoxygenated blood into the blood capillaries and the outward (centrifugal) diffusion of O2 in the air capillaries: the directions are parallel and opposite [Figures (25,26), (30,31)]; and (c) a multicapillary serial arterialization system that entails delivery of the deoxygenated blood to the blood capillaries and that of the sequential flow of parabronchial air into the air capillaries in the exchange tissue of the parabronchi [Figures (27–31)]. From the multicapillary serial arterialization system, the amount of O2 in the oxygenated (arterial) blood that returns to the heart from the lung (via the pulmonary vein) derives from an additive effect in which infinitely small quantities of O2, that are exchanged between the air capillaries and the blood capillaries, are pooled [Figures (27–31)]. The multicapillary serial arterialization system largely increases the gas exchange efficiency by extending the time and distance that deoxygenated blood is exposed to inspired air. In the lung of the mature domestic fowl, the total length of the parabronchi is ~300 m (King and Molony, 1971).
Figure 4. (27) Schematic diagram showing the crosscurrent- and the multicapillary serial arterialization systems in the avian lung. The former comprises the orthogonal relationship between the directions of airflow in the parabronchial lumen (Pb) and that of venous blood from the periphery into the blood capillaries (BC) (pink arrows) while the later comprises the sequential gas exchange between air capillaries and blood capillaries along the length of a parabronchus. SB, secondary bronchus; A, intraparabronchial artery; V, intraparabronchial vein. (28) Frontal view stereogram of a parabronchus showing the crosscurrent arrangement between airflow along the parabronchial lumen (white arrow; PL) and that of venous blood (red arrow) from the periphery. BV, interparabronchial blood vessels; ET, exchange tissue. (29) Side view stereogram of a parabronchus illustrating blood supply to and drainage from the parabronchial exchange tissue (ET) indicating the multicapillary serial arterialization system where venous (deoxygenated) blood (large pink arrow) is oxygenated in the exchange tissue (thin pink arrows) between the air- and the blood capillaries in sequence along the length of a parabronchus. Large and thin red arrows, oxygenated blood; yellow arrow, airflow along the parabronchial lumen; A, intraparabronchial artery; V, intraparabronchial vein; ET, exchange tissue. (30) Three-dimensional serial section computer reconstruction of part of a parabronchus showing crosscurrent-, countercurrent and the multicapillary serial arterialization systems of the avian lung. The first system comprises the relationships between the flows of air in the parabronchial lumen (small pink arrows) and that of venous blood from the periphery (large dark brown arrows); the second system comprises the outward diffusion of O2 in the air capillaries (lime colored arrows) relative to that of venous blood (large dark brown arrows); and the last system is formed by the sequential oxygenation of blood between the air- and the blood capillaries in the exchange tissue (ET) of a parabronchus. IPA, interparabronchial artery; *, intraparabronchial artery; dots, blood capillaries; At, atria; If, infundibula; *, air capillaries. (31) Diagram showing the multicapillary serial arterialization system, where gas exchange occurs in succession between the air capillaries (* and small black dashed arrows) and the blood capillaries (* and small dashed pink arrows) in the exchange tissue (ET) of a parabronchus. DB and brown arrows, deoxygenated (venous) blood; OB and yellow arrows, oxygenated blood; MDSB, mediodorsal secondary bronchus; MVSB, medioventral secondary bronchus; large open arrows, airflow from a MDSB to a MVSB across a parabronchus. Inserts: A–longitudinal view of a parabronchus showing atria (*) evaginating into the exchange tissue (ET); B–Latex cast preparation of parabronchial arterial blood vessels. IPA, interparabronchial artery; arrows, intraparabronchial arteries; PL, parabronchial lumen. The cylindrical lines show the parabronchial outline (solid line) and the dashed line that of the parabronchial lumen.
Without empirical evidence and based solely on known flight capabilities and efficiencies of birds, an energetically costly form of locomotion that requires uptake and expenditure of large amounts of O2 (Bairlein et al., 2015; Butler, 2016), it was claimed that a highly efficacious countercurrent gas exchange system existed and explained the exceptional proficiency of the avian lung (Schmidt-Nielsen et al., 1969; Bretz and Schmidt-Nielsen, 1971). However, by an elegant experiment in which the direction of airflow through the parabronchial system of a duck lung was reversed, Scheid and Piiper (1972) showed that the PCO2 and the PO2 in air as well as in the arterial- and the mixed venous blood did not change. It showed that a countercurrent gas exchange system DID NOT explain the gas exchange efficiency of the avian lung. If it did, the experimental manipulation would have created a cocurrent system, a design that creates a totally different respiratory gas exchange profile. A crosscurrent gas exchange system and NOT a countercurrent one explains the gas exchange mechanism in the avian lung. A countercurrent arrangement between the air capillaries and the blood capillaries has been well morphologically corroborated (West et al., 1977; Maina, 1988, 2005, 2008; Woodward and Maina, 2005; Maina and Woodward, 2009) [Figures (26), (30)]. While the contribution, if any, of the countercurrent gas exchange system to the respiratory efficiency of the avian lung is presently indeterminate (Piiper and Scheid, 1973; Scheid, 1979), some of the reasons why the system may not contribute significantly to gas exchange are: (a) convective flow of blood occurs in the blood capillaries while diffusion of O2 take place in the air capillaries and (b) because the blood- and the air capillaries entwine very closely [Figures (12–14)], the respiratory units contact over very short distances that constantly change in direction. As concerns the construction of a countercurrent system, where the exchanging fluid media are directly exposed to each other over a critical distance, both from functional- and structural considerations, a true countercurrent gas exchange system may not exist in the avian lung.
Scheid et al. (1972) showed that the pattern of airflow that occurs in lungs of living birds is maintained in pump-ventilated paralyzed as well as in fixed, i.e., dead, lungs. The finding established that structure-specific features determined the unidirectional ventilatory airflow that occurs across the paleopulmonic parabronchi of the avian lung. For the mere reasons that the theory was intuitively appealing and that similar structures had been described in fluid conducting tubular anatomical structures (e.g., in veins), it was for a longtime argued that valves or sphincters controlled airflow in the avian lung (McLelland, 1989; Maina, 2017a). More recently, Jones et al. (1981) pointed out that the complexity of airflow in the avian lung shows that particular sites of the airway system should function as valves. Albeit intense search, no structural and/or functional valves or sphincters of the classical anatomical- and physiological nature (Sam et al., 2021) have been identified anywhere in the avian respiratory system. They do not even exist at sites where from what is known of the flow pattern of air in the lung they would be expected to occur such as: (a) at or near the orifices of the medioventral secondary bronchi, where inspired air is shunted across the openings of the airways into the intrapulmonary primary bronchus [Figure (20)] in a process termed inspiratory aerodynamic valving (Banzett et al., 1987, 1991; Butler et al., 1988; Wang et al., 1988, 1992; Maina and Africa, 2000; Maina and Nathaniel, 2001; Maina et al., 2009; Nguyen et al., 2021) and (b) at the area where air leaving the caudal air sacs is directed into the lung via the mediodorsal secondary bronchi instead of exiting via the intrapulmonary primary bronchus (the way it came in) in a process termed expiratory aerodynamic valving (Kuethe, 1988; Brown et al., 1995) [Figure (21)]: inspiratory aerodynamic valving entails convective acceleration of inspired air past the orifices of the medioventral secondary bronchi while expiratory aerodynamic valving, that has been less well-investigated, may depend on dynamic compression of the intrapulmonary primary bronchus that might result from airflow resistance and velocity but not from gas density (Banzett et al., 1987, 1991; Butler et al., 1988; Kuethe, 1988; Wang et al., 1988, 1992; Brown et al., 1995; Maina et al., 2009; Nguyen et al., 2021). Using a novel mathematical model that mimics the experimental observations of the airflow in the avian respiratory system, Harvey and Ben-Tal (2016) showed that the unidirectional ventilatory airflow that occurs in the paleopulmonic parabronchi of the avian lung may be generated by either inspiratory- or effective expiratory valving and that the two processes are critical to the generation of the high efficiencies of airflow in the lung. The efficiencies of inspiratory aerodynamic valving and expiratory aerodynamic valving may depend on other factors such as compliance and pressure differences between the air sacs (Brackenbury, 1971, 1972). CO2 dependent flow resistance, especially at the orifices of the medioventral secondary bronchi, may also contribute to generation of inspiratory aerodynamic valving (Molony et al., 1976).
In the lung of the domestic fowl, Maina and Africa (2000) reported an intensely vascularized swelling that was located at the distal part of the lumen of the extrapulmonary primary bronchus, close to the origin of the first medioventral secondary bronchus [Figures (34–36)]. At a corresponding site, using radio opaque gas, experimentally, in the lung of the goose (Anser anser), Wang et al. (1992) showed a constriction at the distal part of the extrapulmonary primary bronchus: the opening widened during ventilatory hyperpnia and constricted under elevated CO2 in the inspired air during eupneic breathing. The constriction was termed ‘segmentum accelerans’ by Wang et al. (1992). Maina and Africa (2000) suggested that the structure might function like the penile erectile (cavernous) tissue, where influx of blood causes tumescence and efflux collapse: for the avian lung, the processes should respectively result in narrowing and widening of the lumen of the distal part of the primary bronchus. Banzett et al. (1991) and Wang et al. (1992) speculated that during hyperpnea, the segmentum accelerans nearly flattens out to the level of the wall of the extrapulmonary primary bronchus while during eupneic breathing, the lumen constricts. All other factors constant, according to Bernoulli's Principle (Batchelor, 2000; Anderson, 2016), narrowing of the lumen should accelerate the flow of the inspired air, creating convective forward momentum that propels air past the orifices of the medioventral secondary bronchi [Figure (36)]. Interestingly, a segmentum accelerans does not exist in the ostrich lung (Maina and Nathaniel, 2001). If the structure is lacking in some species of birds, it seems like the segmentum accerelans is not critical to generation of inspiratory aerodynamic valving in the avian lung. Lawson et al. (2020) observed that the asymmetry of the primary bronchi distal to the medioventral secondary bronchi suggests that inspiratory aerodynamic valving occurs within a broad range of bronchial dimensions. Features such as sizes, shapes and orientations of the airways [Figures (37,38)] and the presence of upstream structures such as the larynx, the trachea, the syrinx and the cranial air sacs and downstream ones such as the caudal air sacs may to various extents influence inspiratory aerodynamic valving. By a computational fluid dynamics study of the ostrich lung, Maina et al. (2009) showed that the sizes, the shapes and the relative orientations of the passageways determine the pattern of airflow in the avian lung. For the duck lung, while Jones et al. (1981) observed that the orifices of the medioventral secondary bronchi did not change in size or shape during a respiratory cycle, Molony et al. (1976) reported that airflow resistance across the openings of the medioventral secondary bronchi depended on the PCO2 in the inspired air, with resistance being high under low PCO2 and vice versa. Barnas et al. (1978) reported that the smooth muscles of the avian lung respond to fluctuations in intrapulmonary CO2 concentrations. Variations in intrapulmonary gas CO2 alter airflow resistance through the avian lung (Ray and Fedde, 1969 and under hypercapnia, the airways contract forcefully (Leitner and Roumy, 1974; Barnas et al., 1978). Powell et al. (1981) determined that in the avian lung, concentration of CO2 in the inspired air is an important factor in determining airflow valving. Mostly at the parabronchial level, the avian lung is considerably endowed with the contractile smooth muscle and elastic tissue (Maina et al., 2010): it suggests that dimensional changes possibly occur in those parts (King and Cowie, 1969; King and Molony, 1971).
Figure 5. (32–38) are from or based on the lung of the domestic fowl (Gallus gallus variant domesticus) while (39–41) are based on computational fluid dynamics (CFD) work on the ostrich (Struthio camelus) lung. (32–35) Location and structure of a swelling (segmenum accelerans) (arrow). EPPB, extrapulmonary primary bronchus; IPPB, intrapulmonary primary bronchus; *, blood vessels; MVSB, medioventral secondary bronchi. (36) Diagram showing convective acceleration of inspired air by a segmentum accelerans (SA), in a process termed inspiratory aerodynamic valving. EPPB, extrapulmonary primary bronchus; IPPB, intrapulmonary primary bronchus; dashed circle, location of medioventral secondary bronchi. The segmentum accerelans convectively accelerates the inspired air, shunting it past the orifices of the medioventral secondary bronchi. Insert: Computer generated side view of airways of an ostrich lung showing an extrapulmonary primary bronchus (EPPB), an intrapulmonary primary bronchus (IPPB) and medioventral secondary bronchi (MVSB-i–iii). (37,38) Orientations of medioventral secondary bronchi (MVSB) in relation to the intrapulmonary primary bronchi (IPPB): the feature may influence inspiratory aerodynamic valving. (39) Computer generated preparations showing orientation of the medioventral secondary bronchi (MVSB) relative to the intrapulmonary primary bronchus (IPPB) and the extrapulmonary primary bronchus (EPPB). (40) CFD airflow simulations across the extrapulmonary primary bronchus (EPPB), the medioventral secondary bronchi (MVSB) and the intrapulmonary primary bronchus (IPPB): most of the air flows into the second and the third MVSB and the rest of it downstream into the IPPB. (41) CFC simulation of air flow after adding a constriction (segmentum accerelans) to the model (arrow): most of the air flows into the third MVSB and some downstream into the intrapulmonary primary bronchus (IPPB). EPPB, extrapulmonary primary bronchus; MVSB, medioventral secondary bronchi.
The total volume of the avian respiratory system, i.e., the volume of the lung and that of the air sacs together with their extensions into bones comprises ~20% of the volume of a bird's body (Duncker, 1971): in the mute swan (Cygnus olor), the value is, however, as high as 34% (Bech and Johansen, 1980). For animals of equivalent body mass, the total volume of the avian respiratory system is 3 to 5 times larger than that of the mammalian lung (Tenney and Remmers, 1963) and two times larger than the reptilian one (Tenney and Tenney, 1970). Strong positive correlation exists between the total volume of the avian respiratory system and body mass (Lasiewski and Calder, 1971). For birds, the volume of the lung is 27% smaller than that of a nonvolant mammal of equivalent body mass (Maina, 1989, 1993, 2005; Maina et al., 1989) and the value correlates strongly with body mass (r = 0.9970) [Figure (42)]. From measurements of lung weight, Jürgens et al. (1981) determined that bats have heavier lungs compared with small nonflying mammals. Maina et al. (1982a), Maina and King (1984), and Maina et al. (1989, 1991) established that bats have much larger lung volumes compared with the nonflying mammals. The mass specific lung volume, i.e., the volume of the lung per unit body mass, of a bat is 2–5 times larger than that of a shrew (Gehr et al., 1980) and about double that of a hummingbird (Dubach, 1981). In amphibian- and reptilian species, compared with body mass, the lung volume correlates more strongly with the metabolic rate (Tenney and Tenney, 1970). In birds, the mass-specific lung volume of 42.8- and 42.9 cm3.kg−1, respectively of the Andean goose (Chloephaga melanoptera) (Maina et al., 2017) and the violet-eared humming bird (Colibri coruscans) (Dubach, 1981), are the highest values.
Figure 6. Plots of pulmonary morphometric parameters of birds against body mass (42–44) and comparisons of the harmonic mean thicknesses of the blood-gas barrier between birds, bats and nonflying mammals (45). Lung volume (42), respiratory surface area (43) and pulmonary capillary blood volume (44) correlate strongly with body mass. In lungs of birds, bats and nonflying mammals, the thicknesses of the blood-gas barrier correlate weakly with body mass (45): birds have the thinnest blood-gas barrier followed by bats and nonflying mammals. The data on which the plots were prepared come from or are cited in Gehr et al. (1981), Maina and King (1984), Maina (1989, 2005), and Maina et al. (1989, 1991).
In birds, the respiratory surface area correlates strongly with body mass (r = 0.9952) [Figure (43)]. The Andean goose (Maina et al., 2017), the violet-eared hummingbird (Dubach, 1981) and the African rock martin (Hirundo fuligula) (Maina, 1984, 1989, 2005; Maina et al., 1989), respectively with mass-specific respiratory surface area values of 96.5, 87.1, and 86.5 cm2.g−1, are the highest: they correlate with factors such as lifestyle and metabolic capacities. The Andean goose is a large high altitude dwelling bird that flies to high altitudes (Fjeldså and Krabbe, 1990); the hummingbirds are generally very small species of birds that considerably adopt hovering as the mode of locomotion as they forage (Sholtis et al., 2015; Skandalis et al., 2017): hovering is energetically a highly costly mode of flight that is supported by diverse morphological- and physiological specializations (Maina, 2000b) that allow the body weight to be wholly supported by power generated by flapping light muscles (Suarez, 1992; Wells, 1993; Winter, 1998; Clark and Dudley, 2010); and the African rock martin is a small highly energetic passerine bird (Irwin, 1977) that flies swiftly over long distances (Turner and Rose, 1989). The lowest mass-specific respiratory surface areas have been reported in the nonvolant (flightless) or weakly volant species of birds that include the emu (Dromaius novaehollandiae) (5.4 cm2.g−1) (Maina and King, 1989), the domestic fowl (8.7 cm2.g−1) (Abdalla et al., 1982), the Humbolt penguin (Spheniscus humboldt) (18.1cm2.g−1) (Maina and King, 1987) and the domestic turkey (Melleagris gallopavo) (7.0 cm2.g−1) (Abdalla and Maina, 1981). The emu is a large bird that evolved in a continent (Australia) that historically had low level of predation, especially from placental mammals (Sales, 2007). For the nonvolant mammals, the highest mass-specific respiratory surface area of 43 cm2g−1 has been reported in lungs of shrews, e.g., Crocidura flavescens and Sorex sp. (Gehr et al., 1980): the shrews are the smallest and most highly metabolically active nonflying mammals (Fons and Sicart, 1976). Interestingly, while birds have smaller lungs compared with mammals (Maina and King, 1984; Maina, 1989; Maina et al., 1989, 1991) and the volume density of the exchange in the lung is on average <50% (Maina et al., 1982b, 1989; Maina, 1989, 2005) compared with that of more than 85% for the mammalian lung (Gehr et al., 1981; Maina and King, 1984; Maina, 1987, 1990; Maina et al., 1991), the respiratory surface area of the avian lung is ~15% greater than that of the lung of a mammal of equivalent body mass (Maina, 1989, 2005; Maina et al., 1989): the larger respiratory surface area in the smaller avian lung largely stems from the extreme subdivision of the exchange tissue into extremely small air capillaries [Figures (14), (25,26)].
For the avian lung, the pulmonary capillary blood volume correlates strongly with body mass (r = 0.9899) [Figure (44)] (Maina, 1989; Maina et al., 1989). Between 58 and 80% of the volume of blood in the avian lung is found in the blood capillaries (Maina, 1984, 1989, 2005; Duncker and Güntert, 1985a,b; Maina et al., 1989). Compared with that of the exchange tissue of the mammalian lung (Weibel, 1963), for a bird of equivalent body mass the pulmonary capillary blood volume is 2.5 to 3 times greater (Maina, 1989; Maina et al., 1989). For a 45 kg body mass ostrich, with a total volume off the lung of 1.6 l, 250 cm3 of pulmonary capillary blood volume is exposed to air over a respiratory surface area of 183 m2 across a tissue barrier 0.560 μm thin (Maina and Nathaniel, 2001): the respiratory surface area is about that of a singles tennis. Particularly in popular literature, the allegoric statement that the respiratory surface area of the human lung is equivalent to that of a tennis court (Koeppen et al., 2010) is greatly over-exaggerated (Rao and Johncy, 2022): the surface area which is ~135 m2 (average of the alveolar surface area and that of the pulmonary capillary endothelium) (Gehr et al., 1978) is not even close to that of a singles tennis court!: the definite measurements of a tennis court are 195.71 m2 for a singles court and 260.86 m2 for a doubles one (https://olympics.com/en/featured-news/tennis-court-markings-dimensions-size-types-variety-surfacehard-grass-clay—accessed on 23-02-2022). In the avian lung, the pulmonary capillary blood volume is exposed to air just about all round the wall of the blood capillary [Figure (15)].
Compared with the arithmetic mean thickness (τt), the harmonic mean thickness of the blood-gas barrier (τht) is the appropriate parameter for characterizing the diffusing capacity of the lung for O2 (Weibel and Knight, 1964; Weibel, 1973). The ratio of τt to τht expresses the sporadic attenuation, i.e., the corrugation, of the blood-gas barrier (Weibel and Knight, 1964; Meban, 1980; Maina and King, 1982). In birds, with τhts of 0.099 and 0.090 μm, respectively, the violet-eared hummingbird (Dubach, 1981) and the African rock martin (Maina, 1984) have the thinnest blood-gas barriers while the thickest ones exist in lungs of the ostrich (0.560 μm) (Maina and Nathaniel, 2001) and the Humboldt penguin (0.530 μm) (Maina and King, 1987). Welsch and Aschauer (1986) ascribed the thick blood-gas barrier of the penguin lung to the abundance of connective tissue (especially collagen fibers) in the basement lamina of the blood-gas barrier, a feature that might afford tolerance of hydrostatic pressures during deep dives. Compared with the mammalian lung, for animals of equivalent body mass, the blood-gas barriers of the avian lung are 56 to 67% thinner (Maina, 1989, 2005; Maina et al., 1989). For birds, bats and the nonflying mammals, the scaling constants (‘b’) between the thicknesses of the blood-gas barrier (τht) and body mass are respectively 0.0687, 0.0246 and 0.0618 (Maina, 1989, 2005; Maina et al., 1989) [Figure (45)]. Compared with the ‘b’ values for the volume of the lung (1.5867), the respiratory surface area (0.8674) and the pulmonary capillary blood volume (0.9594) for birds [Figures (42–44)], the relatively low allometric constant for the blood-gas barrier suggests that regarding body size (mass), the thicknesses of the tissue barrier has been exceptionally optimized.
Compared with the mammalian, the avian lung is ~10 times more efficient (O'Malley, 2008). It extracts relatively more O2 from the inspired air (Lasiewski and Calder, 1971; Scheid and Piiper, 1972; Scheid, 1979; Farmer, 2015): the oxygenated blood that exits the lungs has greater O2 tension compared with that in the exhaled air (Scheid, 1979; Schmidt-Nielsen, 1983). Synergy of various structural- and functional features and processes explains the exceptional respiratory efficiency of the avian respiratory system (Maina, 2008). The foremost adaptive attributes are the unidirectional ventilatory airflow across the paleopulmonic parabronchi where the exchange tissue is continuously supplied with air with the greatest PO2. Crosscurrent-, multicapillary serial arterialization- and possibly countercurrent gas exchange systems and pulmonary morphometric specializations that include vast respiratory surface area, large pulmonary capillary blood volume and thin blood-gas barrier fundamentally explain the exceptional gas exchange efficiency of the avian respiratory system.
The author confirms being the sole contributor of this work and has approved it for publication.
The author appreciates financial support from the National Research Foundation (NRF) of South Africa in this and other research works.
The author declares that the research was conducted in the absence of any commercial or financial relationships that could be construed as a potential conflict of interest.
All claims expressed in this article are solely those of the authors and do not necessarily represent those of their affiliated organizations, or those of the publisher, the editors and the reviewers. Any product that may be evaluated in this article, or claim that may be made by its manufacturer, is not guaranteed or endorsed by the publisher.
I gratefully appreciate the contributions made by students and collaborators over the years.
Abdalla, M. A., and Maina, J. N. (1981). Quantitative analysis of the exchange tissue of the avian lung. (Galliformes). J Anat. 134, 677–680.
Abdalla, M. A., Maina, J. N., King, A. S., King, D. Z., and Henry, J. (1982). Morphometrics of the avian lung. 1. The domestic fowl, Gallus domesticus. Respir. Physiol. 47, 267–278. doi: 10.1016/0034-5687(82)90057-3
Akester, A. R., Pomeroy, D. E., and Purton, M. D. (1973). Subcutaneous air pouches in marabu stock. (Leptoptios curmeniferus). J Zool. (Lond). 170, 493–499. doi: 10.1111/j.1469-7998.1973.tb05063.x
Alexandrino, E., Buechley, E., Piratelli, A., Ferraz, K. M. P. M.B., Moral, R. D. A., Sekercioglu, Ç. H., et al. (2016). Bird sensitivity to disturbance as an indicator of forest patch conditions: an issue in environmental assessments. Ecol. Indicators. 66, 369–381. doi: 10.1016/j.ecolind.2016.02.006
Amat, J. A., and Green, A. J. (2010). “Waterbirds as bioindicators of environmental conditions,” in Conservation monitoring in freshwater habitats: a practical guide and case studies, Schneider, M. (ed.). Berlin: Springer Science. p. 45–52. doi: 10.1007/978-1-4020-9278-7_5
Anderson, J. D. (2016). “Some reflections on the history of fluid dynamics,” in Handbook of fluid dynamics, 2nd edtn, Johnson, R. W. (ed.). Boca Raton FL, CRC Press. p. 13–28. doi: 10.1201/b19031-4
Bairlein, F., Fritz, J., Scope, A., Schwendenwein, I., Stanclova, G., van Dijk, G., et al. (2015). Energy expenditure and metabolic changes of free-flying migrating northern bald ibis. PLoS ONE. 10, e0134433. doi: 10.1371/journal.pone.0134433
Banzett, R. B., Nations, C. S., Barnas, J. L., Lehr, J. L., and Jones, J. H. (1987). Inspiratory aerodynamic valving in goose lungs depends on gas density and velocity. Respir. Physiol. 70, 287–300. doi: 10.1016/S0034-5687(87)80051-8
Banzett, R. B., Nations, C. S., Wang, N., Fredberg, J. J., and Butler, P. J. (1991). Pressure profiles show features essential to aerodynamic valving in geese. Respir. Physiol. 84, 295–309. doi: 10.1016/0034-5687(91)90125-3
Barnas, G., Mather, F. B., and Fedde, M. R. (1978). Response of avian intrapulmonary smooth muscle to changes in carbon dioxide concentration. Poult. Sci. 57, 1400–1407. doi: 10.3382/ps.0571400
Barnett, J. M., and Buzzetti, D. R. C. (2014). A new species of Cichlocolaptes Reichenbach. (1853). (Furnariidae), the ‘gritador-do-nordeste’, an undescribed trace of the fading bird life of northeastern Brazil. Rev. Brasileira. Ornitologia. 22, 75–94. doi: 10.1007/BF03544237
Barrowclough, G. F., Cracraft, J., Klicka, J., and Zink, R. M. (2016). How many kinds of birds are there and why does it matter? PLoS ONE. 11, e0166307. doi: 10.1371/journal.pone.0166307
Batchelor, G. K. (2000). An Introduction to Fluid Dynamics. Cambridge: Cambridge University Press. doi: 10.1017/CBO9780511800955
Bech, C., and Johansen, K. (1980). Ventilation and gas exchange in the mute swan, Cygnus olor. Respir. Physiol. 39, 285–295. doi: 10.1016/0034-5687(80)90060-2
Bennett, A. (2009). Avian respiratory and thoracic surgery. Availale online at: https://www.dvm360.com/view/avian-respiratory-and-thoracic-surgery-proceedings-0 (accessed August 03, 2021).
Bezuidenhout, A. J. (2005). Light and electron microscopic study of the thoracic respiratory air sacs of the fowl. Anat. Histol. Embryol. 34, 185–191. doi: 10.1111/j.1439-0264.2005.00592.x
Bezuidenhout, A. J., Groenewald, H. B., and Soley, J. T. (1999). An anatomical study of the respiratory air sacs in ostriches. Onderst. J. Vet. Res. 66, 317–325.
BirdLife nternational. (2018). State of the world's birds: Taking the pulse of the planet. Cambridge, UK: BirdLife International.
Brackenbury, J. H. (1971). Airflow dynamics in the avian lung as determined by direct and indirect methods. Respir. Physiol. 13, 319–329. doi: 10.1016/0034-5687(71)90036-3
Brackenbury, J. H. (1972). Lung-air-sac anatomy and respiratory pressure in the bird. J. Exp. Bio.l 57, 543–550. doi: 10.1242/jeb.57.2.543
Bretz, W. L., and Schmidt-Nielsen, K. (1971). Bird respiration: flow patterns in the duck lung. J. Exp. Biol. 54, 103–118. doi: 10.1242/jeb.54.1.103
Brown, R. E., Kovacs, C. E., Butler, J. P., Wang, N., Lehr, J., and Banzett, R. B. (1995). The avian lung: is there an aerodynamic expiratory valve? J. Exp. Biol. 198, 2349–2357. doi: 10.1242/jeb.198.11.2349
Browning, G. R., Eshar, D., Tucker-Mohl, K., and Berke, K. (2019). Diagnosis and surgical repair of a chronic ruptured cervical air sac in a double yellow-headed amazon parrot. (Amazona Ochrocephala Oratrix). J. Exotic. Pet. Med. 29, 45–50. doi: 10.1053/j.jepm.2018.07.008
Brusatte, S. L., O'Connor, J. K., and Jarvis, E. D. (2015). The origin and diversification of birds. Curr. Biol. 25, 888-898. doi: 10.1016/j.cub.2015.08.003
Burri, P. H. (1999). “Lung development and pulmonary angiogenesis,” in Lung disease. Gaultier, C., Bourbon, J., Post, M. (eds.). New York: Oxford University Press. p. 122–151. doi: 10.1007/978-1-4614-7537-8_5
Butler, J. P., Banzett, R. B., and Fredberg, J. J. (1988). Inspiratory valving in avian bronchi: Aerodynamic considerations. Respir. Physiol. 73, 241–256. doi: 10.1016/0034-5687(88)90010-2
Butler, P. J. (2016). The physiological basis of bird flight. Phil Trans R Soc. (Lond). B 371, 2015038420150384. doi: 10.1098/rstb.2015.0384
Carlson, H. C., and Beggs, E. C. (1973). Ultrastructure of the abdominal air sac of the fowl. Res. Vet. Sci. 14, 148–150. doi: 10.1016/S0034-5288(18)33904-3
Clairbaux, M., Fort, J., Matherson, P., Porter, W., and Strøm Grèmillet, D. (2019). Climate change could overturn bird migration: transarctic flights and high-latitude residency in a sea ice free Arctic. Sci. Rep. 9, 17767. doi: 10.1038/s41598-019-54228-5
Clark, J. C., and Dudley, R. (2010). Hovering and forward flight energetics in Anna's and Allen's hummingbirds. Physiol. Biochem. Zool. 83, 654–662. doi: 10.1086/653477
Clippinger, T. L. (1997). Diseases of the lower respiratory tract of companion birds. Semin Avian Exotic Pet Med 6, 201–208. doi: 10.1016/S1055-937X(97)80006-0
Codd, J. R. (2010). Uncinate processes in birds: morphology, physiology and function. Comp. Biochem. Physiol. A Mol. Integr. Physiol. 156, 303–308. doi: 10.1016/j.cbpa.2009.12.005
Codd, J. R., Boggs, D. F., Perry, S. F., and Carrier, D. R. (2005). Activity of three muscles associated with the uncinate processes of the giant Canada goose, Branta canadensis maximus. J. Exp. Biol. 208, 849–857. doi: 10.1242/jeb.01489
Coitier, V. (1573). Anatomia avium. In: Externum et internarum praecipalium humani corporis partium tabulae arque anatomicae exercitationes. Germany, Nuremberg. p. 130–133.
Cook, R. D., Vaillant, C. R., and King, A. S. (1986). The abdominal air sac ostium of the domestic fowl: A sphincter regulated by neuro-epithelial cells? J. Anat. 149, 101–111.
Cooney, C. R., Bright, J. A., Capp, E. J. R., Chira, A. M., Hughes, E. C., Moody, C. J. A., et al. (2017). Mega-evolutionary dynamics of the adaptive radiation of birds. Nature. 542, 344–347. doi: 10.1038/nature21074
Cordell, H. K., and Herbert, N. G. (2002). The popularity of birding is still growing. Birding. 54–61. Available online at: https://www.semanticscholar.org/paper/The-Popularity-of-Birding-is-Still-Growing-Cordell-Herbert/9599dcccb15a422ca023b46187ff4e1a124f7236
Cordell, H. K., Herbert, N. G., and Pandolfi, F. (1999). The growing popularity of birding in the United States. Birding. 31, 168–176.
Covino, K. M., Horton, K. G., and Morris, S. R. (2020). Seasonally specific changes in migration phenology across 50 years in the black-throated blue warbler. Auk. 137, 1–11. Available online at: https://digitalcommons.lmu.edu/cgi/viewcontent.cgi?article=1091&context=bio_fac
De Vries, EHJ, Foppen, RPB, van der Jeugd, H, and Jongejans, E. (2021). Searching for the causes of decline in the Dutch population of European turtle doves. (Streptopelia turtur). IBIS. 164, 552–573. doi: 10.1111/ibi.13031
Dubach, M. (1981). Quantitative analysis of the respiratory system of the house sparrow, budgerigar, and violet-eared hummingbird. Respir. Physiol. 46, 43–60. doi: 10.1016/0034-5687(81)90067-0
Duncker, H. R. (1971). The lung-air sac system of birds. a contribution to the functional anatomy of the respiratory apparatus. Ergeb Anat Entwicklung. 45, 1–171.
Duncker, H. R. (1972). Structure of the avian lung. Respir. Physiol. 14, 4–63. doi: 10.1016/0034-5687(72)90016-3
Duncker, H. R. (1974). Structure of the avian respiratory tract. Respir. Physiol. 22, 1–34. doi: 10.1016/0034-5687(74)90044-9
Duncker, H. R., and Güntert, M. (1985a). “The quantitative design of the avian respiratory system: From hummingbird to the mute swan,” in BIONA Report No. 3, Nachtigall, W. (ed.). Stuttgart: Gustav- Fischer Verlag. p. 361–378.
Duncker, H. R., and Güntert, M. (1985b). “Morphometric analysis of the avian respiratory system,” in Vertebrate morphology, Duncker, H. R., Fleischer, G. (eds.). Stuttgart: Gustav-Fischer. p. 383–387.
Egwumah, F. A., Egwumah, P. O., and Edet, D. (2017). Paramount roles of wild birds as bioindicators of contamination. Int. J. Avian. Wildlife Biol 2, 194–199. doi: 10.15406/ijawb.2017.02.00041
Farmer, C. G. (2015). The evolution of unidirectional pulmonary airflow. Physiology. 30, 260–272. doi: 10.1152/physiol.00056.2014
Fedde, M. R. (1980). The structure and gas flow pattern in the avian lung. Poult. Sci. 59, 2642–2653. doi: 10.3382/ps.0592642
Fedde, M. R. (1998). Relationship of structure and function of the avian respiratory system to disease susceptibility. Poult. Sci. 77, 1130–1138. doi: 10.1093/ps/77.8.1130
Fedde, M. R., Burger, R. E., and Kitchell, R. L. (1964a). Anatomic and electromyographic studies of the costopulmonary muscles in the cock. Poult. Sci. 43, 1177–1184. doi: 10.3382/ps.0431177
Fedde, M. R., Burger, R. E., and Kitchell, R. L. (1964b). Electromyographic studies of the effects of bodily position and anaethesia on the activity of the respiratory muscles of the domestic fowl. Poult. Sci. 43, 839–846. doi: 10.3382/ps.0430839
Fjeldså, J, and Krabbe, N. (1990). Birds of the High Andes: A Manual to the Birds of the Temperate Zone of the Andes and Patagonia, South America. Copenhagen, Denmark: Zoological Museum of the University of Copenhagen.
Fletcher, O. J. (1980). Pathology of the avian respiratory system. Poult. Sci. 59, 2666–2679. doi: 10.3382/ps.0592666
Fons, R., and Sicart, R. (1976). Contribution à la connaissance du métabolisme énergétique chez deux Crocidurinae: Suncus etruscus. (Savi 1822) et Crocidura russula. (Herman 1780), Insectivora, Soricidae, Mammalia. Mammalia. 40, 229–311. doi: 10.1515/mamm.1976.40.2.299
Fowler, M. E., and Cubas, Z. S. (2001). Biology, medicine, and surgery of South American wild animals. Hoboken, NJ: Wiley-Blackwell. doi: 10.1002/9780470376980
Garfinkel, M. B., Minor, E. S., and Whelan, C. J. (2020). Birds suppress pests in corn but release them in soybean crops within a mixed prairie/agriculture system. Condor: Ornithol Appl. 122, 1–12. doi: 10.1093/condor/duaa009
Gehr, P., Bachofen, M., and Weibel, E. R. (1978). The normal human lung: ultrastructure and morphometric estimation of diffusion capacity. Respir. Physiol. 31, 121–140. doi: 10.1016/0034-5687(78)90104-4
Gehr, P., Mwangi, D. K., Amman, A., Maloiy, G. M. O., Taylor, C. R., and Weibel, E. R. (1981). Design of the mammalian respiratory system. V. Scaling morphometric diffusing capacity to body mass: Wild and domestic animals. Respir. Physiol. 44, 41–86. doi: 10.1016/0034-5687(81)90077-3
Gehr, P., Sehovic, S., Burri, P. H., Classen, H., and Weibel, E. R. (1980). The lung of shrews: morphometric estimation of diffusion capacity. Respir. Physiol. 44, 61–86.
Gould, J. L., and Gould, C. G. (2007). Animal architects: Building and the evolution of intelligence. New York, NY: Basic Books.
Harvey, E. P., and Ben-Tal, A. (2016). Unidirectional airflow through avian lungs: new insights from a piecewise linear mathematical model. PLoS Comput. Biol. 12, e1004637. doi: 10.1371/journal.pcbi.1004637
Higuchi, H. (2012). Bird migration and the conservation of the global environment. J. Ornithol. 153, 3–14. doi: 10.1007/s10336-011-0768-0
Hill, E. (2019). Humans, birds and burial practices at Ipiutak, Alaska: Perspectivism in the Western Arctic. Environ. Archaeol. 24, 434–448. doi: 10.1080/14614103.2018.1460031
Hogg, D. A. (1984). The distribution of pneumatization in the skeleton of the adult domestic fowl. J. Anat. 138, 617–629.
Hollamby, S., Afema-Azikuru, J., Waigo, S., Cameron, K., Rae Gandolf, A., and Norris A Sikarskie, J. G. (2006). Suggested guidelines for use of avian species as biomonitors. Environ. Monit. Assess. 118, 13–20. doi: 10.1007/s10661-006-0770-9
Holle, J. P., Meyer, M., and Scheid, P. (1977). Oxygen affinity of duck blood determined by in vivo and in vitro techniques. Respir. Physiol. 29, 355–361. doi: 10.1016/0034-5687(77)90009-3
Hollinger, H. (2017). Air sac rupture in birds. Available online at: https://wagwalking.com/bird/condition/air-sac-rupture#symptoms (accessed on August 03, 2021).
Horton, K. G., La Sorte, F. A., Sheldon, D., Lin, T. Y., Winner, K., Bernstein, G., et al. (2020). Phenology of nocturnal avian migration has shifted at the continental scale. Nature Clim. Change. 10, 63–68. doi: 10.1038/s41558-019-0648-9
Irwin, M. P. S. (1977). Variation, geographical arcs and gene-flow within the populations of the rock martin, Hirundo fuligula. (Ptyonoprogne) fuligula in eastern, southern and south-western Africa. Honeyguide. 91, 10–19.
IUCN. (2020). Red list of threatened species. Version 2020-2. Available online at: https://iucnredlist.org
Jaensch, S. (2015). Inspirational evolution: the avian lower respiratory tract. Available online at: https://www.aavac.com.au/files/2015-01.pdf
Jimoh, S. A., and Maina, J. N. (2013). Immuno-localization of type-IV collagen in the blood-gas barrier and the epithelial - epithelial cell connections of the avian lung. Biol Lett. 9, 20120951. doi: 10.1098/rsbl.2012.0951
Jones, J. H. (1982). Pulmonary blood flow distribution in panting ostriches. J. Appl. Physiol. 53, 1411–1417. doi: 10.1152/jappl.1982.53.6.1411
Jones, J. H., Effmann, E. L., and Schmidt-Nielsen, K. (1981). Control of air flow in bird lungs: Radiographic studies. Respir. Physiol. 45, 121–131. doi: 10.1016/0034-5687(81)90054-2
Jones, J. H., Effmann, E. L., and Schmidt-Nielsen, K. (1985). Lung volume changes during respiration in ducks. Respir. Physiol. 59, 15–25. doi: 10.1016/0034-5687(85)90014-3
Jonzén, N., Lindén A Ergon, T., Knudsen, E., Vik, J. O., Rubolini, D., Piacentinic, D., et al. (2006). Rapid advance of spring arrival dates in long distance migratory birds. Science. 312, 1959–1961. doi: 10.1126/science.1126119
Jung, B. (2001). Surgical repair of air sac rupture in a parrot. Tierärztliche Umschau. 56, 35–37. Available online at: https://www.gardenwildlifehealth.org/wp-content/uploads/sites/12/2019/12/Avian-Air-sac-rupture-factsheet_GWH.pdf
Jürgens, J. D., Bartels, H., and Bartels, R. (1981). Blood oxygen transport and organ weight of small bats and small nonflying mammals. Respir. Physiol. 45, 243-260. doi: 10.1016/0034-5687(81)90009-8
Jürgens, K. D., and Gros, G. (2002). Phylogeny of gas exchange systems. Anasthesiol Intensivmed Notfallmed Schmerzther. 37, 185–198. doi: 10.1055/s-2002-25080
Kamani, J., Tijjani, A., Yidawi, J. P., Gana, A. L., Egwu, O. K., and Gusi, A. M. (2009). Subcutaneous emphysema. (windpuff) in a 13 weeks old pullet: case report. Int. J. Poult. Sci. 8, 1121–1122. doi: 10.3923/ijps.2009.1121.1122
Kardong, K. V. (1988). Vertebrates: Comparative Anatomy, Function, Evolution. New York: McGraw-Hill.
Kimura, J., and Deutsch, G. H. (2007). Key mechanisms of early lung development. Pediatr. Dev Pathol 10, 335–347. doi: 10.2350/07-06-0290.1
King, A. (1966). Structural and functional aspects of the avian lungs and air sacs. Intern. Rev. Gen. Exp. Zool. 2, 171–267. doi: 10.1016/B978-1-4831-9978-8.50010-4
King, A. S., and Cowie, A. F. (1969). The functional anatomy of the bronchial muscle of the bird. J. Anat. 105, 323–336.
King, A. S., and Kelly, D. F. (1956). The aerated bones of Gallus domesticus: The fifth thoracic vertebra and sternalribs. Brit. Veter. J. 112, 279–283. doi: 10.1016/S0007-1935(17)46554-7
King, A. S., and McLelland, J. (1984). Birds: Their structure and function. London: Baillière Tindall.
King, A. S., and Molony, V. (1971). “The anatomy of respiration,” in: Physiology and biochemistry of the domestic fowl, Vol. 1, Bell, D. F., Freeman, B. M. (eds.). London: Academic Press. p. 347–384.
Koeppen, B., Stanton, B., and Berne, R. (2010). Berne and Levy principles of physiology. St. Louis. MO: Elsevier.
Konishi, M., Emlen, S. T., Ricklefs, R. E., and Wingfield, J. C. (1989). Contributions of bird studies to biology. Science. 246, 465–472. doi: 10.1126/science.2683069
Kost, C. (2019). Chasing the Halcyon Light - Human-kingfisher relations in Eastern HanDynasty China. (CE 25-220) and their material, sociocultural and ecological articulations. Environ. Archaeol. 4, 411–433. doi: 10.1080/14614103.2018.1563980
Kost, C., and Hussain, S. T. (2019). Archeo-ornithology: towards archaeology of human-bird interfaces. Environ. Archeol. 24, 337–358. doi: 10.1080/14614103.2019.1590984
Kuethe, D. O. (1988). Fluid mechanical valving of airflow in bird lungs. J. Exp. Biol. 136, 1–12. doi: 10.1242/jeb.136.1.1
Lasiewski, R. C., and Calder, W. A. (1971). A preliminary allometric analysis of respiratory variables in resting birds. Respir. Physiol. 11, 152–166. doi: 10.1016/0034-5687(71)90020-X
Lawson, A. B., Hedrick, B. P., Echols, S., and Schachner, E. R. (2020). Anatomy, variation, and asymmetry of the bronchial tree in the African grey parrot. (Psittacus erithacus). J. Morphol. 282, 701–719. doi: 10.1002/jmor.21340
Leitner, M. M., and Roumy, M. (1974). Vagal afferent activities related to the respiratory cycle in the duck: Sensitivity to mechanical, chemical and electrical stimuli. Respir. Physiol. 22, 41–56. doi: 10.1016/0034-5687(74)90046-2
Llacuna, S., Gorriz, A., Durfort, M., and Nadal, J. (1993). Effects of air pollution on passerine birds and small mammals. Arch. Environ. Contam. Toxicol. 24, 59–66. doi: 10.1007/BF01061089
López, J. (1995). “Anatomy and histology of the lung and air sacs of birds,” in Histology, ultrastructure, and immunohistochemistry of the respiratory organs in non- mammalian vertebrates, Pastor, L. M. (ed.). Murcia, Spain: Publicaciones de la Universitatd de University of Murcia. p. 179–233.
Mackelprang, R., and Goller, F. (2013). Ventilation patterns of the songbird lung/air sac system during different behaviors. J. Exp. Biol. 216, 3611–3619. doi: 10.1242/jeb.087197
Macklem, P., Bouverot, P., and Scheid, P. (1979). Measurement of the distensibility of the parabronchi in duck lungs. Respir. Physiol. 33, 23–35. doi: 10.1016/0034-5687(79)90004-5
Magnussen, H., Willmer, H., and Scheid, P. (1976). Gas exchange in the air sacs: contribution to respiratory gas exchange in ducks. Respir. Physiol. 26, 129–146. doi: 10.1016/0034-5687(76)90057-8
Maina, J. N. (1982a). A scanning electron microscopic study of the air- and blood capillaries of the lung of the domestic fowl. (Gallus domesticus). Experientia. 35, 614–616. doi: 10.1007/BF02327080
Maina, J. N. (1982b). Stereological analysis of the paleopulmo and neopulmo respiratory regions of the avian lung. (Streptopelia decaocto). IRCS Med Sci. 10, 328.
Maina, J. N. (1984). Morphometrics of the avian lung. 3. The structural design of the passerine lung. Respir. Physiol. 55, 291–309. doi: 10.1016/0034-5687(84)90052-5
Maina, J. N. (1987). The morphology and morphometry of the adult normal baboon lung. (Papio anubis). J. Anat 150, 229–245.
Maina, J. N. (1988). Scanning electron microscopic study of the spatial organization of the air- and blood conducting components of the avian lung. (Gallus gallus domesticus.) Anat. Rec. 222, 145–153. doi: 10.1002/ar.1092220206
Maina, J. N. (1989). “The morphometry of the avian lung,” in Form and function in birds, Vol. 4, King, A. S., McLelland, J. (eds.). London: Academic Press. p. 307–368.
Maina, J. N. (1990). A morphological and morphometric study of the prosimian lung: the lesser bushbaby, Galago senegalensis. J. Anat. 172, 129–148.
Maina, J. N. (1993). Morphometries of the avian lung: the structural-functional correlations in the design of the lungs of birds. Comp. Biochem. Physiol. A: Physiol. 105, 397–410. doi: 10.1016/0300-9629(93)90409-W
Maina, J. N. (1994). “Comparative respiratory morphology and morphometry: the functional design of the respiratory systems,” in Advances in comparative and environmental physiology, Gilles, R. (ed.). Berlin: Springer-Verlag. p. 111–232. doi: 10.1007/978-3-642-78598-6_4
Maina, J. N. (1998). The Gas Exchangers: Structure, Function, and Evolution of the Respiratory Processes. Berlin: Springer-Verlag.
Maina, J. N. (2000a). Comparative respiratory morphology: Themes and principles in the design and construction of the gas exchangers. Anat. Rec. 261, 25–44. doi: 10.1002/(SICI)1097-0185(20000215)261:1<25::AID-AR6>3.0.CO;2-7
Maina, J. N. (2000b). What it takes to fly: the structural and functional respiratory refinements in birds and bats. J. Exp. Biol. 203, 3045–3064. doi: 10.1242/jeb.203.20.3045
Maina, J. N. (2002). Structure, function and evolution of the gas exchangers: Comparative perspectives. J. Anat. 201, 281–304. doi: 10.1046/j.1469-7580.2002.00099.x
Maina, J. N. (2003a). A systematic study of the development of the airway. (bronchial) system of the avian lung from days 3 to 26 of embryogenesis: a transmission electron microscopic study on the domestic fowl, Gallus gallus variant domesticus. Tissue Cell. 35, 375–391. doi: 10.1016/S0040-8166(03)00058-2
Maina, J. N. (2003b). Developmental dynamics of the bronchial. (airway)- and air sac systems of the avian respiratory system from days 3 to 26 of life: a scanning electron microscopic study of the domestic fowl, Gallus gallus variant domesticus. Ana.t Embryol. 207, 119–134. doi: 10.1007/s00429-003-0333-6
Maina, J. N. (2005). The Design of the Lung-Air Sac System of Birds: Development, Structure, and Function. Heidelberg: Springer.
Maina, J. N. (2006). Development, structure, and function of a novel respiratory organ, the lung-air sac system of birds: To go where no other vertebrate has gone. Biol. Rev. 81, 545–579. doi: 10.1017/S1464793106007111
Maina, J. N. (2007a). Spectacularly robust! Tensegrity principle explains the mechanical strength of the avian lung. Respir. Physiol. Neurobiol 155, 1–10. doi: 10.1016/j.resp.2006.05.005
Maina, J. N. (2007b). Minutialization at its extreme best! The underpinnings of the remarkable strengths of the air- and the blood capillaries of the avian lung: a conundrum. Respir. Physiol. Neurobiol. 159, 141–145. doi: 10.1016/j.resp.2007.08.005
Maina, J. N. (2008). Functional morphology of the avian respiratory system, the lung-air sac system: efficiency built on complexity. Ostrich. 79, 117–132. doi: 10.2989/OSTRICH.2008.79.2.1.575
Maina, J. N. (2011). Bioengineering Aspects in the Design of Gas Exchangers: Comparative Evolutionary, Morphological, Functional, and Molecular Perspectives. Berlin: Springer-Verlag. doi: 10.1007/978-3-642-20395-4_5
Maina, J. N. (2015a). The design of the avian respiratory system. J. Ornithol. 156, 41–63. doi: 10.1007/s10336-015-1263-9
Maina, J. N. (2015b). Structural and biomechanical properties of the exchange tissue of the avian lung. Anat. Rec. 298, 1673–1688. doi: 10.1002/ar.23162
Maina, J. N. (2015c). “Morphological and morphometric properties of the blood-gas barrier: Comparative perspectives,”. in The vertebrate blood-gas barrier in health and disease: structure, development and remodeling, Makanya, A. N. (ed.). Heidelberg: Springer-Verlag. p. 15–38. doi: 10.1007/978-3-319-18392-3_2
Maina, J. N. (2017a). Pivotal debates and controversies on the structure and function of the avian respiratory system: setting the record straight. Biol. Rev. 92, 1475–1504. doi: 10.1111/brv.12292
Maina, J. N, . (ed.). (2017b). “Functional design of the mature avian respiratory system,” in The biology of the avian respiratory system: Evolution, development, structure and function. Switzerland: Springer, Cham. p. 191–218. doi: 10.1007/978-3-319-44153-5_8
Maina, J. N., Abdalla, M. A., and King, A. S. (1982a). Light microscopic morphometry of the lungs of 19 avian species. Acta. Anat. 112, 264–270. doi: 10.1159/000145519
Maina, J. N., and Africa, M. (2000). Inspiratory aerodynamic valving in the avian lung: functional morphological study of the extrapulmonary primary bronchus. J. Exp. Biol. 203, 2865–2876. doi: 10.1242/jeb.203.18.2865
Maina, J. N., Howard, C. V., and Scales, L. (1983). Length densities and maximum diameter distribution of the air capillaries of the paleopulmo and neopulmo region of the avian lung. Acta Stereol. 2, 101–107.
Maina, J. N., Jimoh, S. A., and Hosie, M. (2010). Implicit mechanistic role of the collagen, smooth muscle, and elastic tissue components in strengthening the air- and blood capillaries of the avian lung. J. Anat. 217, 597–608. doi: 10.1111/j.1469-7580.2010.01279.x
Maina, J. N., and King, A. S. (1982). The thickness of the avian blood-gas barrier: qualitative and quantitative observations. J. Anat. 134, 553–562.
Maina, J. N., and King, A. S. (1984). The structural functional correlation in the design of the bat lung. A morphometric study. J. Exp. Bio.l 111, 43–63. doi: 10.1242/jeb.111.1.43
Maina, J. N., and King, A. S. (1987). A morphometric study of the lung of a humboldt penguin. (Spheniscus humboldti). Zentralb Vet Med C Anat Histo Embryol. 16, 293–297.
Maina, J. N., and King, A. S. (1989). The lung of the emu, Dromaius novaehollandiae: A microscopic and morphometric study. J Anat. 163, 67–74.
Maina, J. N., King, A. S., and King, D. Z. (1982b). A morphometric analysis of the lung of a species of bat. Respir Physiol. 50, 1–11. doi: 10.1016/0034-5687(82)90002-0
Maina, J. N., King, A. S., and Settle, G. (1989). An allometric study of the pulmonary morphometric parameters in birds, with mammalian comparison. Philos Trans R Soc. (Lond) B. 326, 1–57. doi: 10.1098/rstb.1989.0104
Maina, J. N., McCracken, K. G., Chua, B., York, J. M., and Milsom, W. K. (2017). Morphological and morphometric specializations of the lung of the Andean goose, Chloephaga melanoptera: A lifelong high- altitude resident. PLoS ONE. 12:e0174395. doi: 10.1371/journal.pone.0174395
Maina, J. N., and Nathaniel, C. (2001). A qualitative and quantitative study of the lung of an ostrich, Struthio camelus. J Exp Bio.l 204, 2313–2330. doi: 10.1242/jeb.204.13.2313
Maina, J. N., Singh, P., and Moss, E. A. (2009). Inspiratory aerodynamic valving occurs in the ostrich, Struthio camelus lung: A computational fluid dynamics study under resting unsteady state inhalation. Respir. Physiol. Neurobiol. 169, 262–270. doi: 10.1016/j.resp.2009.09.011
Maina, J. N., Thomas, S. P., and Dallas, D. M. (1991). A morphometric study of bats of different size: correlations between structure and function of the chiropteran lung. Phil Trans R Soc. (Lond) B. 333, 31–50. doi: 10.1098/rstb.1991.0059
Maina, J. N., and West, J. B. (2005). Thin and strong! The bioengineering dilemma in the structural and functional design of the blood-gas barrier: Comparative and evolutionary perspectives. Physiol Rev. 85, 811–844. doi: 10.1152/physrev.00022.2004
Maina, J. N., and Woodward, J. D. (2009). Three-dimensional serial section computer reconstruction of the arrangement of the structural components of the parabronchus of the ostrich, Struthiocamelus. Anat Rec. 292, 1685–1698. doi: 10.1002/ar.21002
McLelland, J. (1989). “Anatomy of the lungs and air sacs,” in Form and function in birds, Vol IV, King A. S. McLelland J.(eds.). London: Academic Press. p. 221–279.
Mekonen, S. (2017). Birds as biodiversity and environmental indicator. J. Nat. Sci. Res. 7, 28–34. Available online at: https://www.iiste.org/Journals/index.php/JNSR/article/view/39931
Merkus, P. J., ten Have-Opbroek, A. A., and Quanjer, P. H. (1996). Human lung growth: a review. Pediatr Pulmonol. 21, 383–397. doi: 10.1002/(SICI)1099-0496(199606)21:6<383::AID-PPUL6>3.0.CO;2-M
Molony, V., Graf, W., and Scheid, P. (1976). Effects of CO2 on pulmonary airflow resistance in the duck. Respir. Physiol. 26, 333–349. doi: 10.1016/0034-5687(76)90004-9
Morelli, F., Jerzak, L., and Tryjanowski, P. (2014). Birds as useful indicators of high nature value. (HNV) farmland in Central Italy. Ecol. Indicators. 38, 236–242. doi: 10.1016/j.ecolind.2013.11.016
Morrison, M. L. (1986). “Bird populations as indicators of environmental change,” in Current ornithology, Vol. 3, Johnston, R. F. (ed.). New York: Plenum Press. p. 429–451. doi: 10.1007/978-1-4615-6784-4_10
Moura, R. S., and Correia-Pinto, J. (2017). “Molecular aspects of avian lung development,” in The biology of the avian respiratory system: evolution, development, structure and function, Maina, J. N. (ed.). Switzerland: Springer, Cham. p. 129–146. doi: 10.1007/978-3-319-44153-5_5
Mutua, M. P., Muya, S., and Gicheru, M. M. (2016). Protective roles of free avian respiratory macrophages in captive birds. Biol Res. 49, 29. doi: 10.1186/s40659-016-0090-7
Nguyen, Q. M., Oza, A. U., Abouezzi, J., Sun, G., Childress, S., Frederick, C., et al. (2021). Flow rectification in loopy network models of bird lungs. Phys Rev Lett. 126, 114501. doi: 10.1103/PhysRevLett.126.114501
O'Connor, P. M. (2004). Pulmonary pneumaticity in the postcranial skeleton of extant Aves: a case study examining Anseriformes. J Morph. 261, 141–161. doi: 10.1002/jmor.10190
O'Malley, B. (2008). Clinical anatomy and physiology of avian species - from bird brains and pigeon toes. Proceedings of the World small animal veterinary association. (2008). Available online at: https://www.vin.com/apputil/content/defaultadv1.aspx?id=3866642andpid=11268and (accessed February 23, 2022).
Petevinos, H. (2006). A method for resolving subcutaneous emphysema in a griffon vulture chick. (Gyps fulvus). J Exotic Pet Med. 15, 132–137. doi: 10.1053/j.jepm.2006.02.009
Piiper, J. (ed.)., (1978). “Origin of carbon dioxide in caudal air sacs of birds,” in Respiratory function in birds, adult and embryonic. Berlin: Springer-Verlag. p. 221–248. doi: 10.1007/978-3-642-66894-4_20
Piiper, J., and Scheid, P. (1973). “Gas exchange in the avian lung: Model and experimental evidence,” in, Comparative physiology, Bolis, L., Schmidt-Nielsen, K., Maddrell, S. H. P. (eds.). Elsevier, Amasterdam. p 161–185.
Powell, F. L. (2015). “Respiration,” in Sturkie's avian physiology, 6th edtn, Scanes, C. G. (ed.). San Diego, CA: Academic Press. p. 301–336. doi: 10.1016/B978-0-12-407160-5.00013-0
Powell, F. L. (2022). “Respiration,” in Sturkie's Avian Physiology, 7th edtn, Scanes, C. G., Dridi, S. (eds.). San Diego, CA: Academic Press. p. 445–484. doi: 10.1016/B978-0-12-819770-7.00033-5
Powell, F. L., Geiser, J., Gratz, R. K., and Scheid, P. (1981). Air flow in the avian respiratory tract: Variation of O2 and CO2 concentrations in the bronchi of the duck. Respir. Physiol. 44, 195–213. doi: 10.1016/0034-5687(81)90038-4
Powell, F. L., Hastings, R. H., and Mazzone, R. W. (1985). Pulmonary vascular resistance during unilateral pulmonary artery occlusion in ducks. Am. J. Physiol. 249, R34–R43. doi: 10.1152/ajpregu.1985.249.1.R39
Powell, F. L., and Hempleman, S. C. (1985). Sources of carbon dioxide in penguin air sacs. Am. J. Physiol. 248, R748–752. doi: 10.1152/ajpregu.1985.248.6.R748
Powell, F. L., and Hopkins, S. R. (2004). Comparative physiology of lung complexity: Implications for gas exchange. News Physiol. Sci. 19, 55–60. doi: 10.1152/nips.01469.2003
Rao, A. A., and Johncy, S. (2022). Tennis courts in the human body: a review of the misleading metaphor in medical literature. Cureus. 14, e21474. doi: 10.7759/cureus.21474
Ray, P. J., and Fedde, M. R. (1969). Responses to alterations in respiratory PO2 and PCO2 in the chicken. Respir. Physiol. 6, 135–143. doi: 10.1016/0034-5687(69)90051-6
Redrobe, S. (2015). “Pelecaniformes. (pelicans, tropicbirds, cormorants, frogatebirds, anhingas, gannets), Chapter 12,” in Fowler's zoo and wild animal medicine,Vol. 8, Saunders, W. B. (ed.). St Louis. p. 96–99. doi: 10.1016/B978-1-4557-7397-8.00012-8
Reiblein, E. (2017). Canary in a coal mine. Marine Log, Vol. 122, no. 9, Sept. (2017). p. 7. Gale OneFile: Business. Available online at: https://link.gale.com/apps/doc/A512184846/GPS?u=wedemoandsid=GPSandxid=086f9b8b (accessed February 20, 2022).
Reichert, B. E., Cattau, C. E., Fletcher, R. J., Kendall, W. L., and Kitchens, W. M. (2012). Extreme weather and experience influence reproduction in an endangered bird. Ecology. 93, 2580–2589. doi: 10.1890/12-0233.1
Robbins, J. (2017). The Wonder of Birds: What They Tell us About Ourselves, the World, and a Better Future. New York, NY: Spiegel and Grau, Penguin Random House,
Rosenberg, R. V., Dokter, A. M., Blancher, P. J., Sauer, J. R., Smith, A. C., Smith, P. A., et al. (2019). Decline of the North American avifauna. Science. 366, 120–124. doi: 10.1126/science.aaw1313
Rude, E. (2016). Tastes Like Chicken: A History of America's Favourite Bird. New York: Pegasus Books Ltd.
Sales, J. (2007). The emu. (Dromaius novaehollandiae): A review of its biology and commercial products. Avian and Poult. Biol. Rev. 18, 1–20. doi: 10.3184/147020607X245048
Sam, P., Jiang, J., and LaGrange, C. A. (2021). Anatomy, abdomen and pelvis, sphincter urethrae. In: StatPearls Publishing, Treasure Island. (FL). Available online at: www.ncbi.nlm.nih.gov/books/NBK482438/ (accessed February 23, 2022).
Sanderfoot, O. V., and Holloway, T. (2017). Air pollution impacts on avian species via inhalation exposure and associated outcomes. Environ Res Lett. 12, e083002. doi: 10.1088/1748-9326/aa8051
Schachner, E. R., Hedrick, B. P., Richbourg, H. A., Hutchinson, J. R., and Farmer, C. G. (2021). Anatomy, ontogeny and evolution of the archosaurian respiratory system: a case study on Alligator mississippiensis and Struthio camelus. J Anat. 238, 845–873. doi: 10.1111/joa.13358
Schaefer, H. C., Jetz, W., and Böhning-Gaese, K. (2008). Impact of climate change on migratory birds: community reassembly versus adaptation. Global Ecol. Biogeogr. 17, 38–49. Available online at: https://www.semanticscholar.org/paper/Impact-of-climate-change-on-migratory-birds%3A-versus-Schaefer-Jetz/6dfd194fa7f213c39b2c99d2ebc9bf0ae4f3d0ee
Scheid, P. (1979). Mechanisms of gas exchange in bird lungs. Rev Physiol Biochem Pharmacol. 86, 137–186. doi: 10.1007/BFb0031533
Scheid, P., and Piiper, J. (1972). Cross-currrent gas exchange in the avian lungs: Effects of reversed parabronchial air flow in ducks. Respir. Physiol. 16, 304–312. doi: 10.1016/0034-5687(72)90060-6
Scheid, P., and Piiper, J. (1989). “Respiratory mechanics and air flow in birds,” in Form and function in birds, Vol. 4, King, A. S, McLelland, J. (eds.). London: Academic Press. p. 364–391.
Scheid, P., Slama, H., and Piiper, J. (1972). Mechanisms of unidirectional flow in parabronchi of avian lungs: measurements in duck lung preparations. Respir. Physiol. 14, 83–95 doi: 10.1016/0034-5687(72)90019-9
Schittny, J. (2017). Development of the lung. Cell Tissue Res. 367, 427–444. doi: 10.1007/s00441-016-2545-0
Schmidt-Nielsen, K. (1983). Animal Physiology: Adaptation and Environment, 3rd edtn. Cambridge: Cambridge University Press.
Schmidt-Nielsen, K., Kanwisher, J., Lasiewski, R. C., Cohn, J. E., and Bretz, W. L. (1969). Temperature regulation and respiration in the ostrich. Condor. 71, 341–352. doi: 10.2307/1365733
Sholtis, K. M., Shelton, R. M., and Hedrick, T. L. (2015). Field flight dynamics of hummingbirds during territory encroachment and defense. PLoS ONE. 10, e0125659. doi: 10.1371/journal.pone.0125659
Skandalis, D. A., Segre, P. S., Bahlman, J. W., Groom, D. J. E., Welch, K. C. Jr, Witt, C. C., McGuire, J. A., et al. (2017). The biomechanical origin of extreme wing allometry in hummingbirds. Nat Commun. 8, 1047. doi: 10.1038/s41467-017-01223-x
Soum, J. M. (1896). Recherches physioloqiques sur l'appareil respiratoire des oiseaux. Ann Univ Lyon. 28, 1–126.
Speer, B. L. (2016). Current therapy in avian medicine and surgery, 1st edtn. St. Louis, Missouri: Elsevier.
Spottiswoode, C. N., Begg, K. S., and Begg, C. M. (2016). Reciprocal signaling in honeyguide-human mutualism. Science. 353, 387–389. doi: 10.1126/science.aaf4885
Stolen, E. D., Breininger, D. R., and Frederick, P. C. (2005). “Using waterbirds as indicators in estuarine systems Successes and perils,”. in Estuarine indicators, Bortone, S. A. (ed.). Boca Raton: CRC Press. p.409–422.
Suarez, R. K. (1992). Hummingbird flight: sustaining the highest mass-specific metabolic rates among vertebrates. Experientia. 48, 565–570. doi: 10.1007/BF01920240
Tabur, M. A., and Ayvaz, Y. (2010). Ecological importance of birds. Second International Symposium on Sustainable Development. Sarajevo, Bosnia-Herzegovina. p. 560–565. Available online at: https://omeka.ibu.edu.ba/items/show/3136/1/issd2010_science_book
Tenney, S. M., and Remmers, J. E. (1963). Comparative quantitative morphology of the mammalian lung: Diffusing area. Nature. (Lond). 197, 54–56. doi: 10.1038/197054a0
Tenney, S. M., and Tenney, J. B. (1970). Quantitative morphology of cold-blooded lungs: Amphibia and Reptilia. Respir. Physiol. 9, 197–215. doi: 10.1016/0034-5687(70)90071-X
Tickle, P., Nudds, R., and Codd, J. (2009). Uncinate process length in birds scales with resting metabolic rate. PLoS ONE. 4, e5667. journal.pone.0005667 doi: 10.1371/journal.pone.0005667
Tickle, P. G., and Codd, J. R. (2009). Ontogenetic development of the uncinate processes in the domestic turkey. (Meleagris gallopavo). Poultry Sci. 88, 179–184. doi: 10.3382/ps.2008-00349
Tickle, P. G., Ennos, A. R., Lennox, L. E., Perry, S. F., and Codd, J. R. (2007). Functional significance of the uncinate processes in birds. J. Exp. Biol. 210, 3955–3961. doi: 10.1242/jeb.008953
Turner, A. K., and Rose, C. (1989). A handbook to the swallows and martins of the world. London: Christopher Helm.
Visser, M. E., Perdeck, A. C., van Balen, J. H., and Both, C. (2009). Climate change leads to decreasing bird migration distances. Glob. Chang. Biol. 15, 1859–1865. doi: 10.1111/j.1365-2486.2009.01865.x
Wade, A. S. I., Barov, B., Burfield, I. J., Gregory, R. D., Norris, K., Vorisek, P., et al. (2014). A niche- based framework to assess current monitoring of European forest birds and guide indicator species' selection. PLoS ONE. 9, e97217. doi: 10.1371/journal.pone.0097217
Walsh, C., and McLelland, J. (1974). The ultrastructure of the avian extrapulmonary respiratory epithelium. Acta. Anat. 89, 412–422. doi: 10.1159/000144302
Wang, N., Banzett, R. B., Butler, J. P., and Fredberg, J. J. (1988). Bird lung models show that convective inertia effects inspiratory aerodynamic valving. Respir. Physiol. 73, 111–124. doi: 10.1016/0034-5687(88)90131-4
Wang, N., Banzett, R. B., Nations, C. S., and Jenkins, E. A. (1992). An aerodynamic valve in the avian primary bronchus. J Exp. Biol. 262, 441–445. doi: 10.1002/jez.1402620411
Watson, R. R., Fu, Z., and West, J. B. (2008). Minimal distensibility of pulmonary capillaries in avian lungs compared with mammalian lungs. Respir. Physiol. Neurobiol. 160, 208–214. doi: 10.1016/j.resp.2007.09.013
Weeks, B. C., Willard, D. E., Zimova, M., Ellis, A. A., Witynski, M. L., Hennen, M., et al. (2019). Shared morphological consequences of global warming in North American migratory birds. Ecol Lets. 23, 316–325. doi: 10.1111/ele.13434
Weibel, E. R. (1963). Morphometry of the human lung. Berlin: Springer Verlag. doi: 10.1007/978-3-642-87553-3
Weibel, E. R. (1973). Morphological basis of the alveolar-capillary gas exchange. Physiol. Rev. 53, 419–495. doi: 10.1152/physrev.1973.53.2.419
Weibel, E. R., and Knight, B. W. (1964). A morphometric study on the thickness of the pulmonary air-blood barrier. J. Cell Biol. 21:367–384. doi: 10.1083/jcb.21.3.367
Wells, D. J. (1993). Muscle performance in hovering hummingbirds. J. Exp Biol. 178, 39–57. doi: 10.1242/jeb.178.1.39
Welsch, U., and Aschauer, B. (1986). Ultrastructural observations on the lung of the emperor penguin. (Apternodytes forsteri). Cell Tissue Res. 243, 137–144. doi: 10.1007/BF00221862
West, J. B., Fu, Z., Deerinck, T. J., Mackey, M. R., Obayashi, J. T., and Ellisman, M. H. (2010). Structure-function studies of blood and air capillaries in chicken lung using 3D electron microscopy. Respir. Physiol. Neurobiol. 170, 202–209. doi: 10.1016/j.resp.2009.12.010
West, J. B., Watson, R. R., and Fu, Z. (2006). The honeycomb-like structure of the bird lung allows a uniquely thin blood-gas barrier. Respir. Physiol. Neurobiol. 152, 115–118. doi: 10.1016/j.resp.2005.12.009
West, J. B., Watson, R. R., and Fu, Z. (2007a). Major differences in the pulmonary circulation between birds and mammals. Respir. Physiol. Neurobiol. 157, 382–390. doi: 10.1016/j.resp.2006.12.005
West, J. B., Watson, R. R., and Fu, Z. (2007b). The human lung: Did evolution get it wrong? Eur Respir J. 29, 11-17. doi: 10.1183/09031936.00133306
West, J. B., Watson, R. R., and Fu, Z. (2007c). Support of pulmonary capillaries in avian lung. Respir. Physiol. Neurobiol. 159, 146. j.resp.2007.08.006. doi: 10.1016/j.resp.2007.08.006
West, N. H., Bamford, O. S., and Jones, D. R. (1977). A scanning electron microscope study of the microvasculature of the avian lung. Cell Tissue Res. 176, 553–564. doi: 10.1007/BF00231407
Winter, Y. (1998). Energetic cost of hovering flight in a nectar-feeding bat measured with fast-response respirometry. J. Comp. Physiol. B. 168, 434–444. doi: 10.1007/s003600050163
Woodward, J. D., and Maina, J. N. (2005). A 3D digital reconstruction of the components of the gas exchange tissue of the lung of the muscovy duck, Cairina moschata. J. Ana. 206, 477–492. doi: 10.1111/j.1469-7580.2005.00413.x
Woodward, J. D., and Maina, J. N. (2008). Study of the structure of the air- and blood capillaries of the gas exchange tissue of the avian lung by serial section three-dimensional reconstruction. J. Microsc. 230, 84–93. doi: 10.1111/j.1365-2818.2008.01958.x
Keywords: birds, lungs, air sacs, structure, function
Citation: Maina JN (2022) Perspectives on the Structure and Function of the Avian Respiratory System: Functional Efficiency Built on Structural Complexity. Front. Anim. Sci. 3:851574. doi: 10.3389/fanim.2022.851574
Received: 25 January 2022; Accepted: 01 March 2022;
Published: 13 April 2022.
Edited by:
Mohan Mondal, ICAR-National Dairy Research Institute, IndiaReviewed by:
Lynn Weber, University of Saskatchewan, CanadaCopyright © 2022 Maina. This is an open-access article distributed under the terms of the Creative Commons Attribution License (CC BY). The use, distribution or reproduction in other forums is permitted, provided the original author(s) and the copyright owner(s) are credited and that the original publication in this journal is cited, in accordance with accepted academic practice. No use, distribution or reproduction is permitted which does not comply with these terms.
*Correspondence: John N. Maina, am1haW5hQHVqLmFjLnph
Disclaimer: All claims expressed in this article are solely those of the authors and do not necessarily represent those of their affiliated organizations, or those of the publisher, the editors and the reviewers. Any product that may be evaluated in this article or claim that may be made by its manufacturer is not guaranteed or endorsed by the publisher.
Research integrity at Frontiers
Learn more about the work of our research integrity team to safeguard the quality of each article we publish.