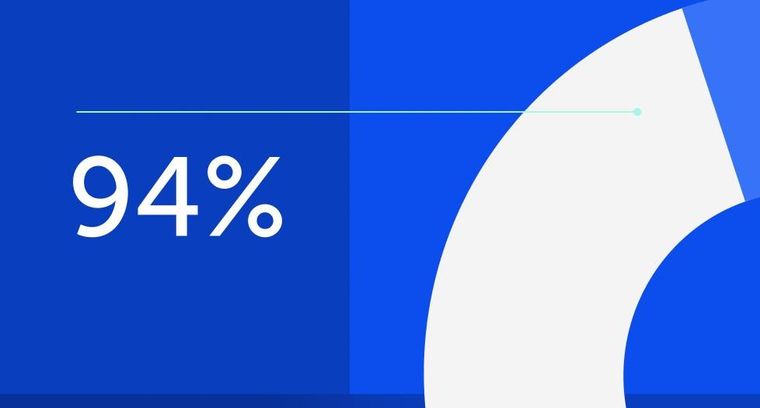
94% of researchers rate our articles as excellent or good
Learn more about the work of our research integrity team to safeguard the quality of each article we publish.
Find out more
ORIGINAL RESEARCH article
Front. Anim. Sci., 06 June 2022
Sec. Animal Nutrition
Volume 3 - 2022 | https://doi.org/10.3389/fanim.2022.837279
This article is part of the Research TopicNatural Products in Animal Feed and Production SystemsView all 5 articles
Aerobic metabolism produces reactive oxygen species (ROS) as a natural by-product that can play a significant role in cell signaling and homeostasis. Excessive and uncontrolled production of ROS, however, can lead to oxidative stress that causes damage to immune cells and is related to several diseases in dairy cattle. Endothelial cells are essential for optimal immune and inflammatory responses but are especially sensitive to the damaging effects of ROS. Accordingly, investigating antioxidant strategies that can mitigate the detrimental impact of ROS on endothelial functions could impact compromised host defenses that lead to increased disease susceptibility. The objective of this study was to test the antioxidant effect of different concentrations (20, 40, 60, 80 μg/ml) of pomegranate by-product extract (PBE) on bovine aortic endothelial cells (BAECs). A model of oxidative stress was developed using in vitro exposure of BAEC to 2,2′-azobis (2-amidinopropane) dihydrochloride (AAPH) to induce the formation of ROS. The BAEC were then analyzed for cell viability, ROS production, fatty acids profile, and oxylipids formation. The BAECs viability did not change after different concentrations of PBE and remained up to 80% over control; whereas, intracellular ROS showed a reduction passing from 20 to 50% with increasing PBE concentration from 20 to 80 μg/ml, respectively. The PBE extract clearly demonstrated efficacy in reducing the concentrations of pro-inflammatory oxylipids with a concomitant enhancement of anti-inflammatory oxylipids. In particular, the pro-inflammatory 13-hydroxyoctadecadienoic acid and its derived anti-inflammatory 13-hydroperoxoctadecaienoic acid were found lower and higher, respectively, in PBE+AAPH treated cells than AAPH treatment. Data from the present study support in vivo future experimental use of pomegranate by-product extract to study its potential beneficial effect against oxidative stress conditions in dairy cattle.
The production of cellular reactive oxygen species (ROS) during aerobic metabolism is a normal process; however, the effect on cellular functions depends on the delicate equilibrium between ROS accumulation during the time of enhanced metabolism and the availability of enzymatic/non-enzymatic scavenging antioxidants present in the tissues. Moderate increases of ROS are crucial for activating signaling cascades that are essential for host defenses, while an excessive production induces oxidative stress conditions resulting in a damage to DNA, protein, or lipids (Schieber and Chandel, 2014). Some of these alterations are related to the formation of secondary compounds that can regulate all aspects of the inflammatory response, among which there are oxylipids. They are generally produced during oxidative stress conditions, and their function is related to the different fatty acids substrate from which they are metabolized as well as the biosynthetic pathways involved. Common substrates for oxylipid synthesis are n-6 polyunsaturated fatty acids (PUFA) such as arachidonic and linoleic acid and n-3 PUFA such as α-linolenic (ALA), eicosapentaenoic acid (EPA), and docosahexaenoic acid (DHA) (Raphael and Sordillo, 2013). Certain oxylipids are recognized as biomarkers of oxidative stress and they play a key role in the onset of inflammation or in its resolution (Serhan et al., 2008). A dysfunctional inflammatory response is crucial in the pathophysiology of several health disorders in dairy cows, such as ketosis, fatty liver, mastitis, and retained placenta; indeed, the production of some oxylipids is activated during tissue damage to control the magnitude and duration of the inflammatory responses (Sordillo, 2018). Thus, the control of oxylipid biosynthesis could be crucial to modulate the inflammatory responses. The development of nutritional-based strategies, such as antioxidant supplementation, can modify the substrate availability for oxylipid biosynthesis during health and disease (Sordillo, 2016).
Endogenous antioxidants in dairy cattle that are needed to mitigate oxidative stress are primarily derived from the diet and are localized transiently in various cells type and tissues throughout the body. One of the primary sources of antioxidants is in the form of vitamins and minerals with known antioxidant capacities; for example, vitamin E and selenium that are associated with enhanced resistance to mastitis during the transition period when dairy cattle experience increased oxidative stress (Baldi et al., 2000; Raphael and Sordillo, 2013). A number of reports have shown that the supplementation of dairy cows with various trace minerals could also reduce metritis and mastitis during the transition period (Sordillo and Aitken, 2009). In the last decades, there has been an interest focused on the development of alternative feed ingredients to the commercial and synthetic additives, with anti-microbial, antioxidant, and immunomodulation effects for animals (Yang et al., 2015). Furthermore, the effects of plant secondary compounds, such as tannins, saponin, and essential oils, as alternative feed additives have been found able to modify ruminal fermentation, antimicrobial activity, improve animal productivity, and mitigate CH4 production (Min et al., 2020). An active area of interest is the possibility to recover the bioactive compounds from agri-food industry waste, which represents innovative and sustainable reuse of this matrix. These recovered bioactive molecules are considered a new class of feed additives for livestock with demonstrated several beneficial responses in animal production in terms of growth performance, nutrient utilization, immunity with an enhancement of antioxidant status in blood and tissues (Van Bibber-Krueger et al., 2016; Kumar et al., 2017; Szczechowiak et al., 2018). Among them, tannins are probably the most studied compounds for farm ruminants (Huang et al., 2018).
The by-product of pomegranate juice production can be derived by peels, seeds, membranes, and the portion of arils, a rich source of polyphenols, that can be recovered and extracted. Pomegranate contains polyphenols including ellagitannins, ellagic acid, and other flavonoids with antimicrobial, anti-inflammatory, antioxidant, and immunomodulatory properties both in vivo and in vitro (Adams et al., 2006; Seeram et al., 2006). Therefore, the hypothesis of the present research was that pomegranate extracts could modulate cellular oxidative status and influence the composition of cell membrane fatty acids and derived oxidative secondary products.
The objective of this study was to evaluate the antioxidant effect of pomegranate by-product extract on cell viability, ROS production, cell membrane fatty acids profile, and oxylipids formation after oxidative stress challenge in BAECs in vitro model.
The PBE (Punica granatum L. var. Wonderful) extract was kindly provided by Dr. Antonio Natalello (the University of Catania, Department of Agriculture, Food and Environment, Via Valdisavoia 5, 95123 Catania, Italy). The residual part of pomegranate fruits containing peels, seeds, membranes, and the portion of arils was collected and subsequently dried in a ventilated oven at 40 °C until constant weight. The phenolic compounds were determined following Makkar et al. (1993) with some modifications. Briefly, 100 g of milled (1 mm) pomegranate by-product was mixed with 700 ml petroleum ether and the mixture was sonicated in a water bath for 15 min. After 2 h of maceration under continuous stirring at 4 °C, petroleum ether was removed, and the leftover solid residue was sonicated with 1 L of acetone/water (70/30, vol/vol) for 15 min in a water bath. After an overnight at 4 °C, the liquid phase was collected, and the acetone was removed by rotary evaporation at 40 °C. The remaining water was washed twice with an equal volume of hexane in a separating funnel, in order to remove lipids still present. The washed water was freeze-dried, and the extract obtained was stored at −30 °C. The composition of PBE was conducted as described by Natalello et al. (2020); briefly, tannin extract concentration resulted in 0.25% of dry matter (g/g), and the main fatty acids present were punicic acid (51.71%), linoleic acid (6.21%), oleic acid (5.60%), and palmitic acid (4.08%).
Bovine aortic endothelial cells (BAEC) were collected from the aorta of healthy Holstein dairy cows based on techniques previously described by Sordillo et al. (1998). The BAECs were purified by dilution cloning techniques and then cultured in Ham's F-12K media containing 10% FBS, 20 mM HEPES, 2 mM L-glutamine, 1% (vol/vol) antibiotic-antimycotic, heparin (100 μg/ml), insulin (10 μg/ml), transferrin (5 μg/ml), and sodium selenite (10 ng/ml). Then, cells were immediately frozen using Ham's F-12K media containing 10% DMSO. Cells were revived from liquid nitrogen at passage 4, used from 8 to 11 passage. After thawing, the BAECs were cultured in a different culture flask until T300 cm2 and detached with trypsin 0.05% at 80–90% of confluence to perform the assays. Trypan blue exclusion was used to evaluate cell death.
For viability and intracellular ROS production cells were seeded the day before at a concentration of 4 × 104 cells/ml in 96 well plate, for oxylipids determination 1 × 106 cells/ml in 100 mm petri dish were used. At the time of the challenge, the BAECs were pre-treated with different concentrations of PBE (20, 40, 60, 80 μg/ml) and incubated for 6 h at 37 °C. Subsequently, the oxidative stress condition was induced by 5 mM of 2,2′-azobis (2-amidinopropane) dihydrochloride (AAPH) for 6 h, the generation of ROO·radical is obtained through decomposition at 37 °C of AAPH that produce two homolytic breaks of C–N bond.
Cell viability was measured using the Promega Cell Titer-Glo Viability Assay (Promega Corp., Madison, WI), which quantifies the amount of ATP, an indicator of metabolically active cells. A mixture of substrate and buffer results in cell lysis and the generation of a luminescent signal proportional to the amount of ATP. Briefly, BAECs were seeded overnight, treated with the experimental extract, and then, the kit solution mix was added (99 μl/well). Luminescence was measured using a Wallac Victor3 1420 Multilabel Plate Reader (PerkinElmer Inc., Waltham, MA). The amount of ATP directly reflects the number of viable cells.
The intracellular ROS levels were measured using the OxiSelect Intracellular ROS Assay Kit (Cell Biolabs, San Diego, CA, USA) following the manufacturers' instructions.
Briefly, BAECs were seeded in a black 96 well plate overnight. The media was then changed to 0% FBS, 20 mM HEPES, 2 mM L-glutamine, 1% (vol/vol) antibiotic-antimycotic, heparin (100 μg/ml), insulin (10 μg/ml), transferrin (5 μg/ml), and sodium selenite (10 ng/ml) for the reading. Fluorescence was measured using a Wallac Victor3 1420 Multilabel Plate Reader (PerkinElmer Inc., Waltham, MA).
Based on results of cell viability and ROS production, the PBE concentration of 80 μg/ml was selected for the subsequent evaluation of fatty acids profile and oxylipids formation.
Media samples were extracted and analyzed using modified methods previously published by Mavangira et al. (2015). Briefly, 1 ml of flash-frozen media was thawed on ice, diluted with 1 ml of 4% formic acid, mixed with an antioxidant reducing agent mixture at 4 μl/ml to prevent degradation of pre-formed oxylipids and that of ex vivo lipid peroxidation (O'Donnell et al., 2009). The antioxidant reducing agent mixture consisted of 50% methanol, 25% ethanol, and 25% water with 0.9 mM of BHT, 0.54 mM EDTA, 3.2 mM TPP, and 5.6 mM indomethacin. Samples were combined with a 15 μl mixture of internal standards containing 0.25 uM 5(S)-HETE-d8, 0.25 uM 15(S)-HETE-d8, 0.5 uM 8(9)-EET-d11, 0.5 uM PGE2-d9, and 0.25 uM 8,9-DHET-d11. Solid-phase extraction utilizing Waters (Waters, Melford Mass) Oasis Prime HLB 3 cc (150 mg) cat#: 186008717 solid-phase extraction columns was performed. Supernatants were loaded into the columns, pushed through with nitrogen, and then the columns were washed with 3 ml of 5% methanol. Samples were eluted with a 2.5 ml of 90:10 acetonitrile:methanol. Volatile solvents were removed using a Savant SpeedVac and residues were reconstituted in methanol, mixed at a 2:1 ratio with HPLC water, and stored in chromatography vials at −80 °C until analysis. A 6-point standard curve was created with a mix of standards and the internal standards mentioned above for quantification.
The quantification of metabolites was performed on a Waters Xevo-TQ-S tandem quadrupole mass spectrometer using multiple reaction monitoring. Chromatography separation was performed with an Ascentis® Express C18 HPLC column, held at 50 °C and autosampler held at 10 °C. Mobile phase bottle A was water containing 0.1% formic acid and mobile phase bottle B was acetonitrile, the flow rate was 0.3 ml/min. Liquid chromatography separation took 15 min per sample. All oxylipids were detected using electrospray ionization in negative-ion mode; cone voltages and collision voltages were optimized for each analyte using Waters QuanOptimize software and data analysis was carried out with Waters MassLynx software.
The reverse-phase LC was obtained on a Waters Acquity UPLC utilizing a BEH C18 1.7 μM (2.1 × 100 mm) column with a flow rate of 0.6 ml/min at 50 °C. The quadrupole MS was in electrospray negative ionization mode and voltage was −3 kV with the turbo ion spray source temperature at 450 °C. The gradient mobile phase was programmed in the following manner (A/B/D ratio): time 0 to 0.5 min (30/5/65), to (65/5/30) at 1.0 min, to (85/10/5) at 5.50 min, to (89/10/1) at 7.0 min, and held until 11.5 min, then return to (30/5/65) at 11.01 min, and held at this condition until 15.0 min. In this gradient mobile phase, A = acetonitrile, B = methanol, and D = 0.1% formic acid. Fatty acids were quantified by matching mass-1 and retention time with corresponding deuterated internal standard abundance and calibrated to a linear 7-point standard curve (R2 > 0.99) using Waters Empower 3 software.
Statistical analysis was conducted using SAS, University Edition (SAS Institute Inc., Cary, NC, 2013). Differences in BAECs viability, intracellular ROS production, fatty acids profile, oxylipids biosynthesis, and their ratio were evaluated between PBE at different concentrations and negative or AAPH control. Comparisons of the means were made by ANOVA one-way followed by Tukey's post-hoc correction test. Data are shown as least squares means ± standard error of the mean. Differences with P < 0.05 were considered statistically significant.
Figure 1 depicted the viability percentage of BAECs after 12 h exposure to different concentrations of PBE (20, 40, 60, and 80 μg/ml). In the present experiment, BAECs exposed to a concentration of 5 mM AAPH showed mean values of cell viability of about 89% over negative control. All PBE treatments showed significant reduction (p < 0.05) of cell viability with respect to negative control; in particular, cells treated with 20 μg/ml showed about 90% viability, while treatments with 40, 60, and 80 μg/ml showed about 86%.
Figure 1. Data of BAECs viability are expressed as percentage (%) calculated on negative control. Percentage changes in BAECs viability treated with 20, 40, 60, and 80 μg/ml concentration of PBE after 6 h of 5 mM AAPH stimulation. Dark gray bars represent negative control. Light gray bars represent AAPH control and different treatments (PBE 20 = pomegranate 20 μg/ml, PBE 40 = pomegranate 40 μg/ml, PBE 60 = pomegranate 60 μg/ml, PBE 80 = pomegranate 80 μg/ml). Different letters (a, b) represent significant difference (p < 0.05). Error bars represent SEM.
The intracellular ROS production was measured to evaluate the protective effect of PBE at 20, 40, 60, and 80 μg/ml on ROS generation following AAPH exposure for 6 h (Figure 2). As expected, AAPH exposure induced higher amounts of ROS compared to untreated cells (P < 0.01) and to BAECs treated with different concentrations of PBE (P < 0.01; P < 0.001). Cells pretreated with PBE showed a decrease of intracellular ROS in a concentration-dependent manner following exposure to AAPH, with a reduction of ROS passing from 20 to 50% with increasing PBE concentration from 20 to 80 μg/ml, respectively.
Figure 2. Mean changes in bovine aortic endothelial cell production of reactive oxygen species (ROS) after exposure to different concentrations of pomegranate by-product extract (PBE) and AAPH, expressed as relative fluorescence unit (RFU). Dark gray bars represent the mean of controls; negative control is untreated cell and AAPH treatment. Light gray bars represent different treatments (PBE 20 = pomegranate 20 μg/ml, PBE 40 = pomegranate 40 μg/ml, PBE 60 = pomegranate 60 μg/ml, PBE 80 = pomegranate 80 μg/ml). Different letters (a−d) represent significant difference (p < 0.05). Error bars represent SEM.
Data on ROS production and cell viability, after PBE exposure, demonstrated that the 80 μg/ml concentration induced an acceptable level of reduction of cell viability, and the concomitant best ROS reduction compared to other PBE concentrations tested. Based on these results, the PBE at a concentration of 80 μg/ml was selected for the evaluation of fatty acids profile and oxylipids formation.
The fatty acids profile detected in BAECs treated with PBE80 after AAPH stimulation are presented in Table 1. Significant differences (P < 0.05) were found in arachidonic acid (AA), linoleic acid (LA), eicosapentaenoic acid (EPA), and docosahexaenoic acid (DHA) showing higher levels in PBE80+AAPH treated cells than AAPH.
Table 1. Fatty acids profile (nM) in bovine aortic endothelial cells pre-treated with pomegranate by-product extract 80 μg/ml after 6 h of 5 mM AAPH exposure.
The type and concentration of oxylipids detected in BAECs treated with PBE80 after AAPH stimulation was presented in Table 2. Among pro-inflammatory oxylipids, significant differences were found for 9-hydroxyoctadecadienoic acid (9-HODE), 13-hydroperoxoctadecaienoic acid (13-HODE), 5-hydroxyeicosatetraenoic acid (5-HETE), 15-HETE, 11-HETE, 9-HETE, Prostaglandin E2 (PGE2) with higher concentrations found in AAPH than in PBE80+AAPH; whereas 9,10-dihydroxyoctadec-12-enoic acid (9, 10 DiHOME) were higher in PBE80+AAPH than AAPH. Among anti-inflammatory oxylipids, significant differences were found for 11, 12-epoxyeicosatrienoic acid (11,12-EET), 14,15-EET, 9, 10 epoxyoctadecamonoenoic acid (9,10-EpOME), 13-oxo-octadecadienoic acid (13-oxoODE), 15-Oxo-eicosatetraenoic acid (15-oxoETE), prostaglandin D2 (PGD2), in particular, 9,10-EpOME and 13-oxoODE levels were higher in PBE80+AAPH than AAPH treatment. Finally, two oxylipids (17,18-dihydroxyarachidonic acid (17,18-DiHETE) and 14,15-DiHETE were found with unknown action, deriving from EPA, with no significant differences among treatments.
Table 2. Concentration of oxylipids (nM) in bovine aortic endothelial cells pre-treated with pomegranate by-product extract 80 μg/ml after 6 h of 5mM AAPH exposure.
To better focus on the balance between the pro- and anti-oxidant metabolites in the cultured BAECs, some oxylipids ratios were studied. In Table 3, the oxylipids ratios among 5-HETE:5-oxoETE, 15-HETE:15-oxoETE, 13-HODE:13-oxoODE, and 9-HODE:9-oxoODE were shown. The ratios 5-HETE:5-oxoETE, 13-HODE:13-oxoODE, and 9-HODE:9-oxoODE were found significantly higher in AAPH than PBE80+AAPH-treated BAECs.
Table 3. Ratio values of pro-inflammatory oxylipids and their corresponding anti-inflammatory forms.
Animal nutrition is a key factor to assist health and welfare, especially during critical phases of the production cycle when animals experience oxidative stress and are more susceptible to diseases. Plant-based feed plays an essential role in optimizing animal health; in particular, polyphenols showed a positive effect on both cell-mediated and humoral immune response (Pathak, 2013), on antioxidant status, and reducing inflammatory responses (Gessner et al., 2017). In this study, the antioxidant effect of PBE on BAECs during oxidative stress challenge was evaluated. In particular, the PBE abilities to reduce ROS production, to modify the cellular fatty acids profile, and to produce different pro- and anti-inflammatory oxylipids were studied.
In our study, the BAECs viability exceeded values of 85% over control after 12 h of exposure to PBE extract apart from concentration, denoting a limited cytotoxic effect. Accordingly, pomegranate peel extract tested at concentrations of 0.1, 1.0, and 10 μg/ml did not display cytotoxic effect on bovine mammary epithelial cells (BME-UV1) and on bovine peripheral blood mononuclear cells (Mastrogiovanni et al., 2019, 2020). On the contrary, Felice et al. (2013) found that the polyphenols extracted by grape seeds tested at concentrations ranging from 2 μg/ml up to 150 μg/ml, exerted a dose-dependent cytotoxic effect on endothelial cells in which a reduction of about 55% cell viability was observed as compared to the untreated cells. Motterlini et al. (2000) found a negative effect on BAECs viability after curcumin treatment (30 μM) with an inhibition of the heme oxygenase pathway, suggesting an impairment of the antioxidant genes activation when cellular damage occurs.
Several studies tested different methods for in vitro evaluation of antioxidant properties of phenolic compounds (Antolovich et al., 2002; Sánchez-Moreno, 2002), demonstrating higher antioxidant activity than vitamin E, vitamin C, and carotenoids (Rice-Evans et al., 1997). Based on best ROS reduction and cell viability, the PBE at 80 μg/ml (PBE80) was further tested for the definition of fatty acids profile and oxylipids formation. In a study by Kuresh et al. (2000), the cytotoxic effect of elderberry extract on BAECs after exposure to different oxidative stressors at different concentrations was evaluated. The elderberry extract realized significant protection against all hydrogen peroxide concentrations was examined, while it was not effective against high concentrations of AAPH-induced damage. This result demonstrated that the antioxidant effect and related cell protection of natural extracts can change depending on the type of stressor. The antioxidant mechanisms of natural extracts, enriched in polyphenols and tannins, is based on the ability to donate an electron to free radicals and delocalize the unpaired electron within the aromatic structure (Fernandez-Panchon et al., 2008); this mechanism can protect biological molecules, such as lipids, from the oxidation process.
The n-6 or n-3 PUFA present in cell membrane phospholipids are precursors of oxylipids, through several different oxidation and reduction pathways (Raphael and Sordillo, 2013). In general, oxylipids n-3 PUFAs derived have lesser biological potency compared with those formed from n-6 PUFAs, and often compete for the same receptor, further mitigating the biological effect (Hawcroft et al., 2010). Diet plays a key role in the alteration and modification of cell membrane phospholipids, and the concentration of oxylipids is strongly affected by the cell membrane fatty acids composition. However, it is worthy noting that PUFAs composition cannot be used to reliably predict the oxylipids content of tissues because the oxylipid biosynthesis processes are complex and not clearly defined (Gabbs et al., 2015). Many scientific reports showed that the transition period is associated with an increased ROS production establishing an oxidative stress condition (Bernabucci et al., 2005); thus, predispose periparturient cows to dysregulated inflammatory conditions, including mastitis (Sordillo and Mavangira, 2014). Furthermore, the possibility of using dietary strategies to reduce the PUFAs substrate availability for oxylipid biosynthesis, during health and disease, has received some research interest. Mavangira et al. (2015) demonstrated that the oxylipid generation, during mastitis in dairy cattle, is influenced by the PUFAs substrate availability in plasma. Present data on cultured BAECs evidenced that the AAPH treatment-induced as expected cell damage with a consequent release of PUFAs from cell membrane phospholipids; however, the PBE polyphenols were able to partly neutralize the harmful effect of excessive ROS production.
Based on the biosynthetic pathway and PUFAs involved in their formation, oxylipids can increase inflammatory conditions or assist in its resolution (Mavangira and Sordillo, 2017). The non-enzymatic pathway of oxylipid biosynthesis is regarded as less specific and leads to the formation of an assortment of oxylipids (Milne et al., 2015), that control a wide range of biological functions, many of which have yet to be defined in dairy cattle. In a human study, it has been demonstrated that the oxylipids profile was altered after increasing dietary concentration of LA or ALA; indeed, oxylipids derived from LA and ALA were present more than one-half of the total oxylipids content measured in the blood (Ramsden et al., 2012). Oxylipids were grouped according to their signaling cell function into pro- and anti-inflammatory; some of them could not be attributed to a specific function and were classified as unknown.
In general, the n-6 PUFA oxy-metabolites have pro-inflammatory activities while n-3 PUFA oxidation products are associated with anti-inflammatory and resolving activities (Gabbs et al., 2015). In our study, AA is one of the most abundant n-6 PUFAs in cultured BAECs, this causes the shift in favor of pro-inflammatory oxylipids formation in this cell model (Table 2). However, pro-inflammatory compounds can be further converted in their corresponding anti-inflammatory oxylipids. Cell membranes usually contain a large amount of AA, compared with other potential oxylipids precursors, which is the principal precursor for biosynthesis (Calder, 2002). The cytochrome P450 (CYP) metabolizes AA to epoxyeicosatrienoic acids (EETs), 20-HETEs, and corresponding DiHETEs (Miyata and Roman, 2005); however, no significant change was found in 20 HETE and DiHETEs oxylipids, probably the absence of differences was related to the CYP activity that results lower in cell culture system compared to in vivo model. Also, PGE2 from AA, derives from the COX-2 pathway with pro-inflammatory activities, and it is in general expressed during the onset of inflammation; whereas an enhancement of COX-2 expression during the resolution of inflammation is associated with the presence of the other COX-2 derived AA metabolites including PGD2. In our study, the higher concentration of PGE2 confirmed the action of AAPH in inflammatory effect induced by oxidative stress; however, PBE80 helped the cell system to switch the production into PGD2 anti-inflammatory compound. This anti-inflammatory oxylipid interacts directly with blood vessels in the mammary gland, able to alter the vascular tone and blood flow within the affected tissues and increase vascular permeability needed for the migration of blood leukocytes to the site of injury (Ryman et al., 2015). Furthermore, the PGD2 oxylipid has the capacity to suppress various pro-inflammatory signaling pathways (Ricciotti and Fitz-Gerald, 2011). Collectively, these results suggest the key role of PBE in the resolution of inflammation in BAECs culture enhancing the anti-inflammatory oxylipids biosynthesis. The oxylipid 5-HETE is one of several oxidative stress-induced metabolites of AA in B-lymphocytes (Grant et al., 2011); even if, in the study of Erlemann et al. (2007) was found that four different epithelial cell lines can synthesize 5-oxo-ETE from 5-HETE in amounts comparable to leukocytes. The hydroperoxides are considered biomarkers of oxidative stress condition and they can contribute to the onset of the pathological condition. In the present study, the BAECs pretreated with pomegranate and subsequently exposed to free-radical resulted in lower 5-HETE oxylipid formation, suggesting a rescue capacity of pomegranate to scavenge ROS and contribute to cellular defense from oxidative damage.
The oxylipids produced from EPA are less biologically potent than the analogs synthesized from AA (Calder, 2002), and many biological activities still need to be investigated. However, in this study, the oxy-metabolite derived from EPA was the 9,10 DiHOME, and showed a higher level in PBE80+AAPH compared with the AAPH control.
Ryman et al. (2015) previously reported that linoleic acid-derived hydroxyoctadecadienoic acid (13-HODE) was formed by an enzymatic pathway during Streptococcus uberis exposure of bovine mammary endothelial cells in vitro. The oxylipid 13-HODE was found the most abundant plasma oxylipid in early lactation dairy cows and was correlated with gene expression of IL-12 and inducible nitric oxide synthase in peripheral blood leukocytes (Raphael et al., 2014). The 15-HETE is one of the primary oxylipids recognized as potent ROS able to cause severe damage to cellular macromolecules. In addition, 15-HETE derived from AA may be involved in oxidative stress-dependent endothelial cell apoptosis, relevant in the pathogenesis of mastitis and other systemic inflammatory-based diseases in dairy cattle (Sordillo et al., 2005; Ryman et al., 2016). Both 13-HODE and 15-HETE, produced as primary oxygenation metabolites, exert similar effects to hydrogen peroxide and are directly involved in the impairment of oxidative stress status. Furthermore, the pro-inflammatory 13-HODE can be converted into its corresponding antioxidant form 13-oxooctadecadienoic acid (13-oxoODE), being the latter produced in higher concentration in presence of PBE80. Changes in the redox status of cells can influence the degree to which initial oxygenation products of PUFA are metabolized to form downstream lipid mediators (Sordillo, 2018). It is reported that the initial product 13-hydroperoxoctadecaienoic acid (13-HPODE), can be reduced to 13-HODE and then can be metabolized further to 13-oxoODE; therefore, pro-inflammatory 13-HPODE is required for the subsequent generation of the anti-inflammatory 13-oxoODE. The effect that oxylipids may have on coordinating inflammatory responses depend upon the timing and expression of certain oxylipids profiles during disease processes. PBE extract clearly demonstrated efficacy in reducing the concentration of primary oxylipids; therefore, it could be hypothesized its possible role in modulating the pro-oxidant pathways by reducing hydroperoxide formation.
Furthermore, both 11-HETE and 9-HODE, classified as pro-inflammatory oxylipid, were found higher in BAECs exposed to AAPH than in the same cells treated with PBE80 (Table 2). It is reported that these metabolites are produced non-enzymatically and can be used as proxies during times of oxidative stress (Sordillo, 2018). This result suggests that PBE moved the balance in favor of anti-inflammatory oxylipids. In particular, the 13-HODE oxylipid is one of the biggest markers expressed during oxidative stress conditions and inflammatory state-related. Indeed, 13-HODE was present in higher concentrations than other LA-derived oxylipids. The LA oxylipids derived are usually present in tissues and blood in higher amounts than oxylipids derived from any other PUFAs (Gabbs et al., 2015). In this study, BAECs exposed to AAPH, the PBE80 sustained the production of the corresponding 13-oxoODE against 13-HODE (Table 3). Moreover, it has been hypothesized that the excessive 13-HPODE or 13-HODE accumulation in periparturient cows may exacerbate the dysfunctional inflammatory responses (Sordillo, 2018), demonstrating that PBE could be a useful dietary intervention to control the inflammatory status.
This study suggests that natural compounds from pomegranate, such as polyphenols and tannins, can exert a protective effect on BAECs membrane phospholipids from free radical damage and consequently can modify the oxylipids profile after an oxidative stress challenge. In particular, the PBE sustains the secretion of the anti-inflammatory oxylipid 13-oxoODE. Potential implications of the use of pomegranate supplementation in lactating dairy cows may help mitigate dysfunctional inflammatory responses due to changes in oxylipids concentration at local and systemic levels. Therefore, further study is needed on the administration of pomegranate by-products in dairy cows to evaluate in vivo effect on oxylipid biosynthesis especially during exposition to oxidative stress condition or peripartum period.
The raw data supporting the conclusions of this article will be made available by the authors, without undue reservation.
ASa and FC conceived the study's original design. FC and JG managed the on-farm trial and analyzed data. ASa, FC, and JG were responsible for analytical procedures. ASa, FC, and MGC wrote the manuscript. ASe and MA revised the first draft of the manuscript. All authors have read, critically revised, and approved the final version of the manuscript. All authors contributed to the preparation of the manuscript.
The authors declare that the research was conducted in the absence of any commercial or financial relationships that could be construed as a potential conflict of interest.
All claims expressed in this article are solely those of the authors and do not necessarily represent those of their affiliated organizations, or those of the publisher, the editors and the reviewers. Any product that may be evaluated in this article, or claim that may be made by its manufacturer, is not guaranteed or endorsed by the publisher.
We would thank Prof. Lorraine Sordillo, who passed away prematurely, for her immense scientific contribution to the project. Thanks to you and your commitment and devotion we have carried out on three-years work, proud to have shared this path with you.
Adams, L. S., Seeram, N. P., Aggarwal, B. B., Takada, Y., Sand, D., and Heber, D. (2006). Pomegranate juice, total pomegranate ellagitannins, and punicalagin suppress inflammatory cell signaling in colon cancer cells. J. Agric. Food Chem. 54, 980.−985. doi: 10.1021/jf052005r
Antolovich, M., Prenzler, P. D., Patsalides, E., McDonald, S., and Robards, K. (2002). Methods for testing antioxidant activity. Analyst 127, 183–198. doi: 10.1039/b009171p
Baldi, A., Savoini, G., Pinotti, L., Monfardini, E., Cheli, F., and Orto, V. D. (2000). Effects of vitamin E and different energy sources on vitamin E status, milk quality and reproduction in transition cows. J. Vet. Med. Series A. 47, 599–608. doi: 10.1046/j.1439-0442.2000.00323.x
Bernabucci, U., Ronchi, B., Lacetera, N., and Nardone, A. (2005). Influence of body condition score on relationships between metabolic status and oxidative stress in periparturient dairy cows. J. Dairy Sci. 88, 2017–2026. doi: 10.3168/jds.S0022-0302(05)72878-2
Calder, P. C. (2002). Dietary modification of inflammation with lipids. Proc. Nutr. Soc. 61, 345–358. doi: 10.1079/PNS2002166
Erlemann, K. R., Cossette, C., Gravel, S., Lesimple, A., Lee, G. J., Saha, G., et al. (2007). Airway epithelial cells synthesize the lipid mediator 5-oxo-ete in response to oxidative stress. Free Radic. Biol. Med. 42:654–664. doi: 10.1016/j.freeradbiomed.2006.12.006
Felice, F., Zambito, Y., Belardinelli, E., D'Onofrio, C., Fabiano, A., Balbarini, A., et al. (2013). Delivery of natural polyphenols by polymeric nanoparticles improves the resistance of endothelial progenitor cells to oxidative stress. Eur. J. Pharmac. Sci. 50, 393–399. doi: 10.1016/j.ejps.2013.08.008
Fernandez-Panchon, M. S., Villano, D., Troncoso, A. M., and Garcia-Parrilla, M. C. (2008). Antioxidant activity of phenolic compounds: from in vitro results to in vivo evidence. Food Sci. Nutr. 48, 649–671. doi: 10.1080/10408390701761845
Gabbs, M., Leng, S., Devassy, J. G., Monirujjaman, M., and Aukema, H. M. (2015). Advances in our understanding of oxylipins derived from dietary PUFAs. Adv. Nutr. 6, 513–540. doi: 10.3945/an.114.007732
Gessner, D. K., Ringseis, R., and Eder, K. (2017). Potential of plant polyphenols to combat oxidative stress and inflammatory processes in farm animals. J. Anim. Physiol. Anim. Nutr. 101, 605–628. doi: 10.1111/jpn.12579
Grant, G. E., Gravel, S., Guay, J., Patel, P., Mazer, B. D., Rokach, J., et al. (2011). 5-Oxo-ETE is a major oxidative stress-induced arachidonate metabolite in B lymphocytes. Free Radic. Biol. Med. 50:1297–1304. doi: 10.1016/j.freeradbiomed.2011.02.010
Hawcroft, G., Loadman, P. M., Belluzzi, A., and Hull, M. A. (2010). Effect of eicosapentaenoic acid on E-type prostaglandin synthesis and EP4 receptor signaling in human colorectal cancer cells. Neoplasia 12, 618–627. doi: 10.1593/neo.10388
Huang, Q., Liu, X., Zhao, G., Hu, T., and Wang, Y. (2018). Potential and challenges of tannins as an alternative to in-feed antibiotics for farm animal production. Anim. Nutr. 4:137–150. doi: 10.1016/j.aninu.2017.09.004
Kumar, P., Patra, A. K., Mandal, G. P., Samanta, I., and Pradhan, S. (2017). Effect of black cumin seeds on growth performance, nutrient utilization, immunity, gut. health and nitrogen excretion in broiler chickens. J. Sci. Food Agric. 97, 3742–3751. doi: 10.1002/jsfa.8237
Kuresh, A. Y., Martin, A., and Joseph, J. A. (2000). Incorporation of the elderberry anthocyanins by endothelial cells increases protection against oxidative stress. Free Radic. Biol. Med. 29, 51–60. doi: 10.1016/S0891-5849(00)00329-4
Makkar, H. P., Blümmel, M., Borowy, N. K., and Becker, K. (1993). Gravimetric determination of tannins and their correlations with chemical and protein precipitation methods. J. Sci. Food Agric. 61, 161–165. doi: 10.1002/jsfa.2740610205
Mastrogiovanni, F., Bernini, R., Basiric,ò, L., Bernabucci, U., Campo, M., Romani, A., et al. (2020). Antioxidant and anti-inflammatory effects of pomegranate peel extracts on bovine mammary epithelial cells BME-UV1. Nat. Prod. Res. 34, 1465–1469. doi: 10.1080/14786419.2018.1508149
Mastrogiovanni, F., Mukhopadhya, A., Lacetera, N., Ryan, M. T., Romani, A., Bernini, R., et al. (2019). Anti-inflammatory effects of pomegranate peel extracts on in vitro human intestinal Caco-2 cells and ex vivo porcine colonic tissue explants. Nutrients. 11, 548–562. doi: 10.3390/nu11030548
Mavangira, V., Gandy, J. C., Zhang, C., Ryman, V. E., Jones, A. D., and Sordillo, L. M. (2015). Polyunsaturated fatty acids influence differential biosynthesis of oxylipids and other lipid mediators during bovine coliform mastitis. J. Dairy Sci. 98, 6202–6215. doi: 10.3168/jds.2015-9570
Mavangira, V., and Sordillo, L. M. (2017). Role of lipid mediators in the regulation of oxidative stress and inflammatory responses in dairy cattle. Res. Vet. Sci. 116, 4–14. doi: 10.1016/j.rvsc.2017.08.002
Milne, G. L., Dai, Q., and Roberts, L. J. (2015). The isoprostanes-25 years later. Biochim. Biophys. Acta. 4:433–445. doi: 10.1016/j.bbalip.2014.10.007
Min, B. R., Solaiman, S., Waldrip, H. M., Parker, D., Todd, R. W., and Brauer, D. (2020). Dietary mitigation of enteric methane emissions from ruminants: A review of plant tannin mitigation options. Anim. Nutr. 6(3): 231–246. doi: 10.1016/j.aninu.2020.05.002
Miyata, N., and Roman, R. J. (2005). Role of 20-hydroxyeicosatetraenoic acid (20-HETE) in vascular system. J. Smooth Muscle Res. 41, 175–193. doi: 10.1540/jsmr.41.175
Motterlini, R., Foresti, R., Bassi, R., and Green, C. J. (2000). Curcumin, an antioxidant and anti-inflammatory agent, induces heme oxygenase-1 and protects endothelial cells against oxidative stress. Free Radic. Biol. Med. 28, 1303–1312. doi: 10.1016/S0891-5849(00)00294-X
Natalello, A., Hervás, G., Toral, P. G., Luciano, G., Valenti, B., Mendoza, A. G., et al. (2020). Bioactive compounds from pomegranate by-products increase the in vitro ruminal accumulation of potentially health promoting fatty acids. Anim. Feed Sci. Technol. 259, 114355. doi: 10.1016/j.anifeedsci.2019.114355
O'Donnell, V. B., Maskrey, B., and Taylor, G. W. (2009). Eicosanoids: generation and detection in mammalian cells. Methods Mol. Biol. 462, 5–23. doi: 10.1007/978-1-60327-115-8_1
Pathak, A. K. (2013). Potential of using condensed tannins to control gastrointestinal nematodes and improve small ruminant performance. Int. J. Molec. Veter. Res. 3, 36–50. doi: 10.5376/ijmvr.2013.03.00
Ramsden, C. E., Ringel, A., Feldstein, A. E., Taha, A. Y., Macintosh, B. A., Hibbeln, J. R., et al. (2012). Lowering dietary linoleic acid reduces bioactive oxidized linoleic acid metabolites in humans. Prostaglandins Leukot. Essent. Fatty Acids. 87:135–141. doi: 10.1016/j.plefa.2012.08.004
Raphael, W., Halbert, L., Contreras, G. A., and Sordillo, L. M. (2014). Association between polyunsaturated fatty acid-derived oxylipid biosynthesis and leukocyte inflammatory marker expression in periparturient dairy cows. J. Dairy Sci. 97, 3615–3625. doi: 10.3168/jds.2013-7656
Raphael, W., and Sordillo, L. M. (2013). Dietary polyunsaturated fatty acids and inflammation: the role of phospholipid biosynthesis. Intern. J. Molecular Sci. 14, 21167–21188. doi: 10.3390/ijms141021167
Ricciotti, E., and Fitz-Gerald, G. A. (2011). Prostaglandins and inflammation. Arterioscler. Thromb. Vasc. Biol. 31, 986–1000. doi: 10.1161/ATVBAHA.110.207449
Rice-Evans, C., Miller, N., and Paganga, G. (1997). Antioxidant properties of phenolic compounds. Trends Plant Sci. 2, 152–159. doi: 10.1016/S1360-1385(97)01018-2
Ryman, V. E., Packiriswamy, N., and Sordillo, L. M. (2016). Apoptosis of endothelial cells by 13-hpode contributes to impairment of endothelial barrier integrity. Mediat. Inflamm. 13. doi: 10.1155/2016/9867138
Ryman, V. E., Pighetti, G. M., Lippolis, J. D., Gandy, J. C., Applegate, C. M., and Sordillo, L. M. (2015). Quantification of bovine oxylipids during intramammary Streptococcus uberis infection. Prostaglandins Other Lipid Mediat. 121, 207–217. doi: 10.1016/j.prostaglandins.2015.09.006
Sánchez-Moreno, C. (2002). Review: methods used to evaluate the free radical scavenging activity in foods and biological systems. Food Sci. Technol. Int. 8, 121–137. doi: 10.1177/1082013202008003770
SAS Institute Inc., Cary, NC. (2013). SAS Enterprise Guide: Statistics. Version 6, 1. ed. Cary, NC: SAS Inst. Inc.
Schieber, M., and Chandel, N. S. (2014). ROS function in redox signaling and oxidative stress. Current Biol. 24, 453–462. doi: 10.1016/j.cub.2014.03.034
Seeram, N. P., Schulman, R. N., and Heber, D. (2006). Pomegranates: Ancient Roots to Modern Medicine. ed. CRC Press, Taylor and Francis Group, Boca Raton, FL.
Serhan, C. N., Yacoubian, S., and Yang, R. (2008). Anti-inflammatory and proresolving lipid mediators. Annu. Rev. Pathol. Mech. Dis. 3, 279–312. doi: 10.1146/annurev.pathmechdis.3.121806.151409
Sordillo, L. M. (2018). Symposium review: oxilipids and the regulation of bovine mammary inflammatory responses. J. Dairy Sci. 101, 5629–5641. doi: 10.3168/jds.2017-13855
Sordillo, L. M., and Aitken, S. L. (2009). Impact of oxidative stress on the health and immune function of dairy cattle. Vet. Immunol. Immunopathol. 128, 104–109. doi: 10.1016/j.vetimm.2008.10.305
Sordillo, L. M., and Mavangira, V. (2014). The nexus between nutrient metabolism, oxidative stress and inflammation in transition cows. Anim. Prod. Sci. 54, 1204–1214. doi: 10.1071/AN14503
Sordillo, L. M., SooHoo, H., Aherne, K. M., Reddy, C. C., and Hogan, J. S. (1998). A method to reduce glutathione peroxidase levels in primary endothelial cell cultures. Methods Cell Sci. 19, 243–253. doi: 10.1023/A:1009759132250
Sordillo, L. M., Weaver, J. A., Cao, Y. Z., Corl, C., Sylte, M. J., and Mullarky, I. K. (2005). Enhanced 15-HpETE production during oxidant stress induces apoptosis of endothelial cells. Prostaglandins Other Lipid Mediat. 76, 19–34. doi: 10.1016/j.prostaglandins.2004.10.007
Sordillo, L. M. (2016). Nutritional strategies to optimize dairy cattle immunity. J. Dairy Sci. 99, 4967–4982. doi: 10.3168/jds.2015-10354
Szczechowiak, J., Szkudelska, K., Szumacher-Strabel, M., Sadkowski, S., Gwozdz, K., El-Sherbiny, M., et al. (2018). Blood hormones, metaBolic parameters and fatty acid proportion in dairy cows fed condensed tannins and oils Blend. Ann. Anim. Sci. 18, 155–166. doi: 10.1515/aoas-2017-0039
Van Bibber-Krueger, C. L., Miller, K. A., Aperce, C. C., Alvarado-Gilis, C. A., Higgins, J. J., and Drouillard, J. S. (2016). Effects of crystalline menthol on blood metabolites in Holstein steers and in vitro volatile fatty acid and gas production. J. Anim. Sci. 94, 1170–1178. doi: 10.2527/jas.2015-8779
Keywords: bovine aortic endothelial cell, pomegranate extract, oxylipid, dairy cattle, oxidative stress
Citation: Ciampi F, Gandy J, Ciliberti MG, Sevi A, Albenzio M and Santillo A (2022) Pomegranate (Punica granatum) By-Product Extract Influences the Oxylipids Profile in Primary Bovine Aortic Endothelial Cells in a Model of Oxidative Stress. Front. Anim. Sci. 3:837279. doi: 10.3389/fanim.2022.837279
Received: 16 December 2021; Accepted: 15 March 2022;
Published: 06 June 2022.
Edited by:
James Levi Klotz, United States Department of Agriculture, United StatesReviewed by:
Vincenzo Lopreiato, Catholic University of the Sacred Heart, ItalyCopyright © 2022 Ciampi, Gandy, Ciliberti, Sevi, Albenzio and Santillo. This is an open-access article distributed under the terms of the Creative Commons Attribution License (CC BY). The use, distribution or reproduction in other forums is permitted, provided the original author(s) and the copyright owner(s) are credited and that the original publication in this journal is cited, in accordance with accepted academic practice. No use, distribution or reproduction is permitted which does not comply with these terms.
*Correspondence: Antonella Santillo, YW50b25lbGxhLnNhbnRpbGxvQHVuaWZnLml0
Disclaimer: All claims expressed in this article are solely those of the authors and do not necessarily represent those of their affiliated organizations, or those of the publisher, the editors and the reviewers. Any product that may be evaluated in this article or claim that may be made by its manufacturer is not guaranteed or endorsed by the publisher.
Research integrity at Frontiers
Learn more about the work of our research integrity team to safeguard the quality of each article we publish.