- 1Division of Animal Sciences, University of Missouri, Columbia, MO, United States
- 2United States Department of Agriculture-Agricultural Research Service (USDA-ARS), Plant Genetics Research Unit, Columbia, MO, United States
- 3National Swine Resource and Research Center, University of Missouri, Columbia, MO, United States
Generating porcine embryos in vitro is a critical process for creating genetically modified pigs as agricultural and biomedical models; however, these embryo technologies have been scarcely applied by the swine industry. Currently, the primary issue with in vitro-produced porcine embryos is low pregnancy rate after transfer and small litter size, which may be exasperated by micromanipulation procedures. Thus, in this review, we discuss improvements that have been made to the in vitro porcine embryo production system to increase the number of live piglets per pregnancy as well as abnormalities in the embryos and piglets that may arise from in vitro culture and manipulation techniques. Furthermore, we examine areas related to embryo production and transfer where improvements are warranted that will have direct applications for increasing pregnancy rate after transfer and the number of live born piglets per litter.
Introduction
In vitro-produced (IVP) embryos are essential for employing assisted reproductive technologies to establish porcine models. Recent development of genome editing techniques has fueled considerable interest in expanding porcine models for biomedical and agricultural purposes. As pigs have similar physiology and more relevant body and organ sizes to humans compared to other laboratory species, porcine models can be used to understand disease progression and to test relevant doses of therapeutics or medical devices. To date, several porcine biomedical models have been generated, including those for cystic fibrosis (Rogers et al., 2008), cardiovascular disease (Turk et al., 2005), cancer (Schook et al., 2015; Hendricks-Wenger et al., 2021), phenylketonuria (Koppes et al., 2020), immunodeficiency (Suzuki et al., 2012; Lee et al., 2014), viral infection (Lei et al., 2016), and xenotransplantation (Lai et al., 2002). Moreover, gene editing has been used to improve carcass traits (Lai et al., 2006) of pigs and to confer resistance to viruses that plague the swine industry (Whitworth et al., 2016, 2019).
The majority of porcine embryo production systems are comprised of three main steps: oocyte maturation, in vitro fertilization, and embryo culture. Initially, oocytes are aspirated from ovaries (gilt or sow) and go through the in vitro maturation process. The oocytes that reach the metaphase II (MII) stage are selected for in vitro fertilization (IVF). Afterwards, presumptive zygotes are cultured in vitro prior to embryo transfer. Intensive studies have been conducted to develop porcine embryo culture media (Lee et al., 2013; Spate et al., 2015; Redel et al., 2016b; Chen et al., 2018), and the development of different culture systems has dramatically impacted when embryo transfers are able to be performed. Moreover, the embryo production system is essential for designing porcine models. To create genetically modified pigs, manipulation of oocytes and embryos is required. The most common methods for introducing mutations into target genes or transgenes are microinjection of the CRISPR/Cas9 system (mRNA or ribonucleoprotein complex) into the porcine zygote or transfection of fetal fibroblasts to generate donor cells for somatic cell nuclear transfer (SCNT) (Figure 1). Both methods are routinely used to produced genetically modified pigs; however, SCNT is technically demanding which can lower the efficiency of obtaining live piglets. After the manipulation procedures, embryos are cultured and subsequently transferred into a surrogate gilt. Gene editing of embryos by microinjection results in pigs with heterogeneous mutations, while SCNT results in pigs with the same mutation as the donor cell (Figure 1).
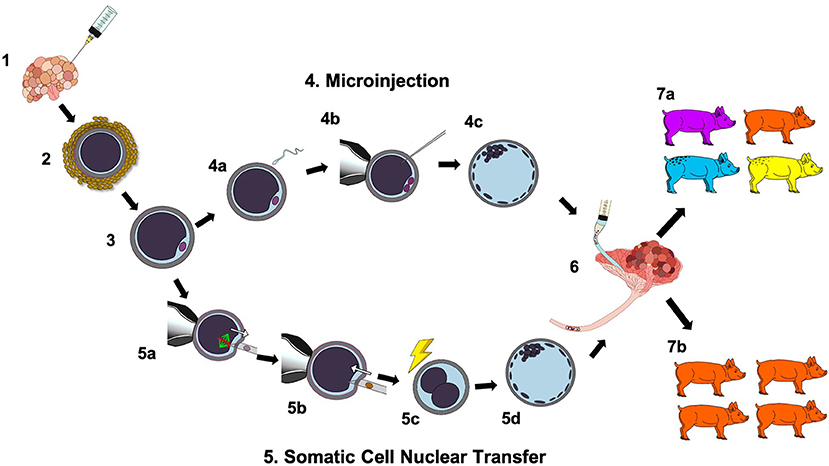
Figure 1. Schematic of in vitro production of porcine embryos, manipulation procedures, and surgical transfer to obtain live-born piglets. (1) Follicular contents are aspirated from follicles (3–6 mm in diameter) by using an 18-gauge needle attached to a 10-mL disposable syringe. (2) Cumulus-oocyte complexes (COCs) with at least two layers of cumulus cells to be placed in maturation medium. (3) Oocytes are denuded and are inspected for extrusion of the first polar body (metaphase II), as an indicator of maturation. (4) Microinjection: (4a) Matured oocytes are fertilized in vitro and (4b) zygotes are injected with the CRISPR/Cas9 system. (4c) Zygotes are cultured for 5–6 days to reach the blastocyst stage. (5) Somatic cell nuclear transfer (SCNT): (5a) The first polar body and metaphase plate are extracted from the oocyte. (5b) An edited donor cell is injected into the perivitelline space. (5c) The oocyte and donor cell are electrically fused followed by artificial activation. (5d) The cloned embryo is cultured for 5–6 days to reach the blastocyst stage. (6) The embryos are surgically transferred into the ampullary-isthmic junction of a gilt. (7a) Piglets derived from microinjected embryos have varying edits to the target gene(s) as denoted by different colors. (7b) Cloned piglets have the same mutation(s) as the donor cells used for SCNT as denoted by the same color.
Although IVP of embryos has proven to be beneficial and has been adopted for species, such as cattle, small ruminants, and horses, the swine industry has been reluctant to adopt these technologies. Similar to other species, in vitro-produced porcine embryos exhibit reduced quality and ability to establish a pregnancy after transfer compared to their in vivo-derived counterparts (Macháty et al., 1998; Bauer et al., 2010). Thus, a considerable amount of effort has been devoted to improving each step of the IVP process, which is the main focus of this review. Furthermore, developmental and epigenetic aberrations have been noted after in vitro culture (IVC) of porcine embryos, possibly resulting in abnormalities during gestation and after birth. Other issues, including polyspermy, embryo transfer procedures, and cryopreservation, are discussed as barriers to widespread use of IVP porcine embryos.
Advances in In Vitro Maturation
Oocyte maturation is a complex process that involves the coordination of events that together allow the oocyte to resume meiosis, undergo successful fertilization, and support normal embryo development and subsequent development to term (Gilchrist and Thompson, 2007). Detailed underlying mechanisms and signaling events that promote oocyte development in vitro are not fully understood. In vivo, the immature oocyte and surrounding cumulus cells (CCs) maintain an intimate relationship which allow the oocyte to gradually acquire meiotic and developmental competence (Gilchrist et al., 2008). The bi-directional communication between the oocyte and its surrounding CCs is critical in activating the necessary signaling pathways needed for competence to be attained. Starting the in vitro embryo production (IVP) process with the most competent oocytes is critical as the oocyte's intrinsic quality sets the stage for proper embryo development (Lonergan et al., 2003).
Oocytes remain under meiotic arrest because of high levels of oocyte secreted cyclic adenosine monophosphate (cAMP) (Mehlmann et al., 2004). The act of removing a cumulus oocyte complex (COC) from its follicular environment and placing it into culture will lead to spontaneous resumption of meiosis. This is likely due to the physical disruption of gap junctions, which leads to a reduced influx of cyclic guanosine monophosphate (cGMP) from the CCs. Cyclic GMP plays an important role as an inhibitor of cAMP hydrolysis (Norris et al., 2009; Vaccari et al., 2009), and the reduction of cGMP in oocytes prematurely removed from follicles may cause oocytes to resume meiosis and complete nuclear maturation. However, the premature progression of meiosis often results in defective cytoplasmic maturation, therefore compromising development (Sela-Abramovich et al., 2006). Phosphodiesterases are a group of enzymes that hydrolyze cyclic nucleotides which can lead to a decrease in cGMP and cAMP levels and results in the resumption of meiosis. Treatment of COCs removed from follicles with phosphodiesterase inhibitors, such as milrinone, has shown promising results in improving oocyte nuclear and cytoplasmic competence (Roy et al., 2021). Furthermore, cAMP negatively regulates maturation promoting factor (MPF), and decreases in cAMP levels with concomitant increases in MPF is critical for successful meiosis (Yu et al., 2008). Upon fertilization, intracellular calcium oscillations in the oocyte cytoplasm promote degradation of MPF and allow meiosis to be completed (Madgwick et al., 2004).
Another issue often encountered during in vitro maturation (IVM) is improper activation of the mitogen activated protein kinase-3 and−1 (MAPK3/1) signaling pathway, which is a direct result of a decreased response to luteinizing hormone (LH). Oocytes derived from small or medium sized antral follicles often respond poorly to LH as the CCs surrounding oocytes from immature follicles do not possess adequate LH receptors (Eppig et al., 1997). In vivo, acquisition of an appropriate numbers of LH receptors in CCs followed by the LH surge activates a signaling cascade that promotes oocyte maturation, which incorporates actions of epidermal growth factor (EGF)-like factors and the downstream MAPK3/1 pathway (Shimada et al., 2003, 2006; Hsieh et al., 2007). Activation of MAPK3/1 plays an integral role in successful oocyte maturation and is needed for optimal cumulus cell expansion (Su et al., 2003; Fan et al., 2009; Yuan et al., 2017). To improve the response of COCs to LH in vitro, follicle stimulating hormone (FSH) is added to the medium to increase in LH receptor numbers, and EGF is supplemented to promote CC expansion and maturation (Prochazka et al., 2003; Kawashima et al., 2008). Recently, addition of three cytokines, fibroblast growth factor 2 (FGF2), leukemia inhibitory factor (LIF), and insulin like growth factor 1 (IGF1) together (termed “FLI”) during maturation improved oocyte competency by influencing the induction of MAPK3/1 activation in the cumulus cells (Yuan et al., 2017). Supplementation of FLI into porcine oocyte maturation medium dramatically increased the number of porcine oocytes that completed nuclear maturation, doubled the number of embryos that reached the blastocyst stage, and quadrupled the number of piglets born compared to the traditional form of oocyte maturation (Yuan et al., 2017). The FLI matured COCs showed a dramatic increase in cumulus cell expansion, decreased the number of transzonal projections as time in culture increased, and demonstrated a difference in the timing of MAPK3/1 activation in cumulus cells. Effectiveness of this novel maturation system has been validated for pigs (Procházka et al., 2021) and has been incorporated into maturation systems for cattle and sheep (Stoecklein et al., 2021; Tian et al., 2021).
A recent study also suggests that FLI provides necessary signals to promote oocyte maturation without the presence of gonadotropins, specifically LH and FSH. Porcine oocytes placed into a maturation medium supplemented with FLI and without LH and FSH were able to complete nuclear maturation at an equivalent frequency but with only negligible CC expansion (Redel et al., 2021). The findings go against a conventional dogma that CC expansion is necessary or a marker for successful oocyte maturation. Results in the study suggest that FLI can drive oocyte competence in the absence of gonadotropins and has a downstream role in enhancing CC expansion when gonadotropins are present (Redel et al., 2021). More research is needed to completely understand the mechanisms by which FLI is promoting oocyte maturation and if other players can be supplemented to continue to improve our IVM system.
Outcomes of IVM are highly influenced by the oocyte source, specifically depending on whether the oocytes are derived from either prepubertal gilts or sexually mature sows. Bagg et al. (2007) observed that ovaries from gilts have increased numbers of follicles that are 3 mm in diameter, whereas ovaries from sows have more follicles ranging from 4 to 8 mm. Oocytes derived from small follicles (3 mm) gave rise to blastocyst-stage embryos with decreased cell numbers compared to larger follicles of either source, indicating lower developmental quality (Bagg et al., 2007). Although both oocyte sources have been used to generate embryos that result in live piglets, oocytes from gilts have also been shown to be less responsive to FSH during maturation, in turn decreasing progression to MII, and exhibit decreased development to the blastocyst stage compared to sow-derived oocytes (Marchal et al., 2001). However, improvements in porcine maturation systems, such as the addition of FLI, have dramatically increased oocyte quality and subsequent developmental competence of gilt-derived oocytes (Yuan et al., 2017). Understanding these key pathways will assist us in designing an optimal IVM system that enhances availability of in vitro-matured porcine oocytes.
History of Porcine Embryo Culture
The process of embryo transfer in pigs necessitates embryo culture, if even for a brief period. The ability to maintain viable gametes or embryos in vitro is one of the enabling technologies that facilitates genetic manipulation of pigs and is central to advancements in our understanding of reproductive biology. Prior to the 1990s, zygotes, 2-cell stage embryos, compact morula, and blastocyst-stage embryos could be recovered from a donor sow and transferred into a surrogate to produce offspring. Culture from the zygote to the blastocyst stage followed by production of offspring was a rare occurrence (Davis and Day, 1978). While there were a few reports of development through the 4-cell stage (e.g., Menino and Wright, 1982), there was a so-called in vitro “block” to development that occurred during the 4-cell stage. A similar “block” to development was observed in most mammalian species that corresponded to the stage at which significant amounts of RNA synthesis first began after fertilization. Embryos could be cultured before and after this critical stage while retaining viability but could not be cultured through it and maintain viability. Researchers working on mice screened every available strain and discovered that the C57 BL/6 strain could develop in vitro through this stage (block occurs at the two-cell stage). Likely a result of a unique tolerance to hyperosmolarity (Hadi et al., 2005), the C57 BL/6 became the standard for embryo culture and manipulation experiments. For those of us who worked on other mammals, such as pigs, achieving development from the zygote to blastocyst stage required approaches whereby zygotes were placed in a co-culture environment (White et al., 1989), medium supplemented with oviductal fluid (Archibong et al., 1989), embedded in agar cylinders and cultured in a sheep oviduct (Prather et al., 1991), or in vitro in an organ culture system (Krisher et al., 1989).
Gradually, different formulations were described whereby embryos could be routinely cultured from the zygote to blastocyst stage, such as NCSU23 (Reed et al., 1992), Whitten's Medium (Beckmann and Day, 1993), porcine zygote medium (PZM) (Yoshioka et al., 2002). One such culture medium was modified Tyrode's Lactate without bicarbonate and buffered with HEPES (TL-HEPES) (Hagen et al., 1991). The discovery that TL-HEPES would support development from the zygote stage to the blastocyst stage was stumbled upon quite serendipitously. One Friday afternoon, zygotes were collected from a gilt that was supposed to provide 4-cell stage embryos. The zygotes were flushed from the oviduct by using TL-HEPES. Since the wrong stage of embryos was collected and there was no use for them, the zygotes were left in the flushing medium and placed in a humidified warm air incubator. The next week when old culture dishes were being removed from the incubator, it was noticed that the zygotes had become blastocyst stage embryos (RSP, personal observation). About that time, there was much debate regarding which components of the culture system were either inadequate or toxic to the developing embryo (reviewed by Petters and Wells, 1993). So, it was shown with TL-HEPES as the base medium that, contrary to some reports, neither glucose nor glutamine inhibited development (Hagen et al., 1991). Since TL-HEPES does not require a CO2 atmosphere for buffering the pH, it has been widely used as a holding or embryo transfer medium for porcine embryos over the past 30 years (Redel et al., 2019). It was not until the 1990s that the first reports of embryo culture in pigs, whereby zygotes were rinsed from one sow's oviduct, identified microscopically, cultured to the blastocyst stage, and then transferred to the oviduct of another sow with resulting pregnancies, were published (Beckmann and Day, 1993).
While the “block” to embryo development had been a major topic of scientific papers for over a half century, the “block” seems to have disappeared (Prather, 2010). In retrospect, the fine tuning of the culture system by altering the osmolarity (Baltz and Tartia, 2010) and using highly purified water may have caused the “block” to “disappear.” The “block” that was once a major hurdle to advancements in our understanding of early embryonic events. While culture systems are certainly not ideal, they have evolved to the state of functionality that permit widespread repeatability and application of embryo related technologies.
Incorporation of powerful technologies such as RNA-sequencing now allow us to understand embryo requirements in vitro and improve standard medium formulations. For instance, the transcriptional profiles of in vivo-matured and -fertilized, in vitro-cultured blastocyst stage porcine embryos predominantly exhibited an upregulation of transcripts related to amino acid transport and metabolism pathways compared with their in vivo-derived counterparts (Bauer et al., 2010). Transcript abundance of the arginine transporter, solute carrier 7A1 (SLC7A1), was increased in vitro-cultured embryos, indicating a potential deficiency of arginine, and supplementation of arginine to embryo culture increased developmental parameters and resulted in the birth of live piglets (Redel et al., 2016b). Similarly, RNA-sequencing data let to supplementing glutamine to current culture system, and improved embryo development in vitro and led to successful term development after transfer (Chen et al., 2018). The same notion was used to supplement glycine in culture, and benefits of the supplementation was observed in vitro. (Redel et al., 2016a). However, 11 surrogates failed to become pregnant after receiving embryos cultured in supplemental glycine, indicating that in vitro measures of quality do not necessarily translate into in vivo developmental competence. These studies demonstrate that the adaptation of new technologies enable us to interpret the status of porcine embryos under culture conditions and assist us to continuously advance the culture systems.
Pregnancy Rate with In Vitro-Produced Embryos
In vitro-produced embryos are known to possess reduced quality and viability compared to their in vivo-derived counterparts. Before a reliable embryo culture system was developed, embryos were transferred at the one-cell stage into a gilt on days 0 or 1 after standing estrus (Lee et al., 2014; Whitworth et al., 2014). Improvements in the culture system have allowed for transfer of IVP blastocyst-stage embryos into a surrogate pig without compromising viability (Lee et al., 2013; Redel et al., 2016b; Chen et al., 2018). Morula- and blastocyst-stage embryos are typically transferred into a surrogate gilt on days 3, 4, or 5 after standing estrus. This asynchronized transfer strategy accounts for the developmental delay of IVP embryos (Bauer et al., 2010). Most term pregnancies are allowed to farrow; however, Cesarean section (C-section) can also be successfully performed. If a pregnancy is lost during gestation, the majority of loss occurs between days 25 to 45 by resorption, and in rare cases, the surrogate will abort the pregnancy after day 45 (Lai and Prather, 2003).
Since 2017, our group has performed four transfers of unmanipulated IVF embryos cultured in vitro that went to term with an 100% pregnancy rate and average of 10.3 ± 0.3 live piglets per litter. Based on the fact that 40–60 blastocyst-stage embryos were transferred in each case, ~1 in 5 embryos developed to term, indicating a significant improvement in the culture system. However, reports of transfers by using in vivo-derived embryos indicate that ~1 in 3 blastocyst-stage embryos develop to term (Yoshioka et al., 2002; Martinez et al., 2015). Considering these rates, improvements in the developmental potential of IVP embryos can still be made to reduce the number of embryos that need to be transferred.
As discussed above, IVP embryos are essential for the application of ART. However, application of embryo manipulation or SCNT often compromises embryo viability, resulting in a low term development. The full-term pregnancy rate after transferring IVF embryos injected with CRISPR/Cas9 systems (microinjection) ranges from 40 to 47% (187 transfers since 2017) with an average of 5.7 ± 0.7 live piglets per litter. The full-term pregnancy rate after transferring SCNT-derived embryos generated from numerous cell lines was 20–30% (264 transfers since 2017) with an average of 3.2 ± 0.4 live piglets per litter. The full-term development from these embryos is typically lower compared to unmanipulated IVF embryos due to accumulated damage during embryo manipulation or gene editing itself. In addition, efficacy of SCNT also highly depends upon the type of donor cells used for the process (Hao et al., 2009; Richter et al., 2012; Whitworth et al., 2014). Examples between in vivo-, IVF-, and SCNT-derived embryo transfers and resulting litter sizes of live-born piglets can be found in Table 1, and the success rates of in vivo-derived pregnancies vs. pregnancies from different in vitro manipulations are depicted in Figure 2.
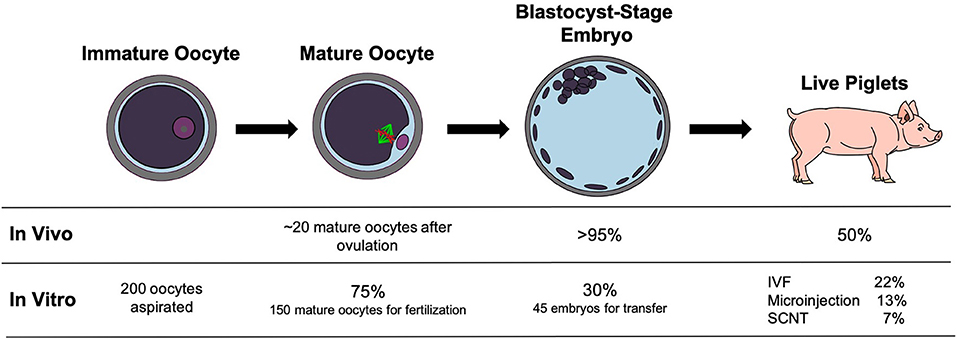
Figure 2. Success rates of pregnancies from in vivo-derived embryos and in vitro-produced embryos by different manipulation procedures. Under in vivo conditions, ~20 oocytes are ovulated each cycle with rates of fertilization and development to the blastocyst stage over 95%. About 50% of the embryos will produce live piglets. Under in vitro conditions, if 200 oocytes are aspirated from ovaries, ~150 oocytes will mature to metaphase II (75%). After IVF or SCNT, roughly 30% will develop to the blastocyst stage for transfer into a surrogate gilt. Different manipulations, IVF with no manipulation, microinjection, and SCNT, result in decreased percentages of embryos that produce live piglets compared to in vivo conditions.
Previous embryo transfer reports certainly indicate that the current IVP system in pigs (both IVM and IVC) has been improved; blastocyst transfers can be practically applied, and the number of live piglets is closer to transferring in vivo-derived embryos. However, embryos and piglets originating from the IVP system can present abnormalities that are additional barriers to consider.
Abnormalities Observed in In Vitro-Produced Preimplantation Embryos
Development of the embryo culture system now successfully supports the production of porcine embryos in vitro, and the embryos are essential for studying early developmental processes as well as for the application of genetic engineering in pigs. However, the quality of embryos (e.g., cell number, apoptosis, epigenetic status) originating in vitro remains inferior to in vivo-derived embryos. Currently, development of IVF embryos to the blastocyst stage is ~40% on day 6 (Redel et al., 2016a; Yuan et al., 2017; Chen et al., 2020), with significant embryo loss or arrest at earlier stages. Blastocyst-stage embryos produced in vitro contain about 40 to 50 cells on day 6 (Redel et al., 2016a; Yuan et al., 2017; Chen et al., 2018, 2020), which is an improvement compared to the previous IVP systems (Macháty et al., 1998; Bauer et al., 2010). However, a significantly lower number of cells are detected in IVP day 7.5 blastocyst-stage embryos compared to in vivo counterparts, 50 vs. 80, respectively (Canovas et al., 2017), presumably contributing to the low term development. In addition, the ratio of inner cell mass (ICM) to trophectoderm (TE) cell numbers, a predictive marker of embryo quality (Tao et al., 1995), is often skewed by IVP. The ICM:TE ratio of day 6 blastocyst-stage embryos cultured under the previous IVP system (~0.15–0.2) was lower than in vivo counterparts (~0.4–0.5) (Macháty et al., 1998; Yoshioka et al., 2002). Optimization of the culture system led to an improvement of the ICM:TE ratio of IVP embryos (~0.35–0.4) (Redel et al., 2016a; Jeong et al., 2017), which is close to the level of in vivo-derived blastocyst-stage embryos.
Regulation of DNA methylation is a major epigenetic event that occurs during preimplantation development in mammals and is critical for normal embryo development (Reik et al., 2001). Fertilized oocytes undergo a massive decrease in global DNA methylation during the transition from gametes to the blastocyst stage, except for imprinted genes and some repetitive elements (Messerschmidt et al., 2014). Artificial intrusions on natural embryo development, including in vitro maturation of oocytes, in vitro embryo culture, and varying oxygen tensions, are expected to influence to the epigenome of IVP embryos (El Hajj and Haaf, 2013; Gaspar et al., 2015; Sirard, 2017). Porcine IVP embryos are reported to possess a high level of DNA methylation compared to in vivo-derived counterparts (Deshmukh et al., 2011). The global level of 5-methylcytosine, measured through immunocytochemistry (ICC), was higher in IVF zygotes than in vivo-derived zygotes, and the higher DNA methylation level was maintained in cleavage and blastocyst-stage embryos, suggesting that IVP embryos may experience impaired post-fertilization demethylation. A recent study conducted an analysis of the whole genome DNA methylation status of in vivo-derived and IVP pig blastocyst-stage embryos at single nucleotide resolution (Canovas et al., 2017). Consistent with the results of the ICC assay, whole genome DNA methylation analyses revealed that global methylation levels, including CpG islands, promoters, intergenic regions, and repetitive elements, are higher in IVP blastocyst-stage embryos (~15%) than in vivo-derived blastocyst-stage embryos (~12%). In vitro culture also affects the expression and methylation of imprinted genes, which is critical for normal embryo development (Reik et al., 2001). Transcript abundance of imprinted genes, imprinting related genes, and X-linked genes differ between IVP and in vivo-derived blastocyst-stage embryos (Park et al., 2011, 2012; Canovas et al., 2017). Although the methylation status of differentially methylated regions (DMRs) is expected to be stable during embryogenesis, IVP pig blastocyst-stage embryos exhibit aberrant methylation patterns in several imprinted genes, such as ZAC1, PEG10, and NNAT, compared to their in vivo counterparts (Canovas et al., 2017). Interestingly, supplementation of oviductal or uterine fluid to fertilization and culture media induces shifts in the DNA methylation profiles of IVF embryos to become more similar to the epigenome of in vivo-derived embryos, indicating suboptimal culture conditions contributes to the epigenetic aberrations in porcine embryos produced in vitro (Canovas et al., 2017).
Incomplete epigenetic reprogramming of donor cell nuclei has been considered one of the main causes for developmental failure in cloned animals (Rideout et al., 2001; Mann and Bartolomei, 2002; Bonk et al., 2007). An early study on the DNA methylation status of cloned pig embryos found that genome-wide post-fertilization demethylation also occurs during preimplantation development (Kang et al., 2001). However, like IVF embryos, cloned embryos retain relatively higher DNA methylation levels compared to in vivo-derived embryos during preimplantation development (Kang et al., 2001; Bonk et al., 2008; Deshmukh et al., 2011). Suboptimal IVC could be a source for the incomplete epigenetic reprogramming of cloned embryos as shown in IVF embryos; however, the abnormal epigenetic status is likely related to the SCNT process because cloned embryos possess a higher global DNA methylation level compared to IVF embryos (Kwon et al., 2008; Huan et al., 2014) and impairments in maintaining methylation imprints (Wei et al., 2011). Indeed, somatic driven DNA methyltransferase 1 (DNMT1) is known to interfere with DNA methylation reprogramming in cloned pig embryos (Song et al., 2017). Reducing DNMT1 abundance by siRNA or epigenetic modification agents improves DNA methylation reprogramming and developmental competency of cloned pig embryos (Xu et al., 2013; Huan et al., 2014, 2015a,b; Song et al., 2017), supporting the fact that a high level of DNMT1 is a barrier to the reprogramming of DNA methylation marks in cloned embryos. Furthermore, treatment of cloned porcine embryos with histone deacetylase inhibitors promotes the DNA demethylation process by reducing DNMT1 expression levels (Liang et al., 2015), leading to improved developmental competency (Zhang et al., 2007; Whitworth et al., 2011; Zhao et al., 2013).
Histone modifications establish molecular landmarks that differentiate active and inactive chromatin states (Rideout et al., 2001). Moreover, histone modifications are important factors that determine successful epigenetic reprogramming of cloned embryos as an open chromatin state must be achieved in the somatic donor cell to mimic the early embryo (Whitworth and Prather, 2010; Ogura et al., 2013). Acetylation and methylation of lysine residues on the amino-terminal tails of histone H3, such as H3K9ac, H3K27ac, and H3K4me3, are markers of an open chromatin state, correlating with the developmental potential of cloned embryos (Santos et al., 2003; Li et al., 2008). Cloned porcine embryos possess lower H3K9ac and H3K4me3 levels than normal fertilized embryos at early developmental stages, which represents a relatively inactive chromatin state (Zhai et al., 2018a,b). Conversely, the repressive histone marker, H3K9me3, is more abundant at early stages in cloned porcine embryos than those derived by IVF (Zhai et al., 2018a; Jeong et al., 2020). Patterns of histone modifications representing repressive chromatic structures serve as a barrier to successful epigenetic reprogramming of donor cells and correspond to a lower developmental potential. Indeed, induction of an open chromatin state in cloned porcine embryos by treatment with histone deacetylase inhibitors or histone-lysine methyltransferases of H3K9 leads to improvement of nuclear reprogramming and the developmental potential (Zhang et al., 2007; Whitworth et al., 2011; Song et al., 2014; Huang et al., 2016; Jeong et al., 2020; Weng et al., 2020).
Abnormalities Observed in Neonatal Piglets Derived From IVF OR SCNT
Different abnormalities have been detected in piglets derived by the IVP system and manipulation procedures, although predominantly the reports are related to cloned piglets. High rates of stillbirths have been noted for pregnancies carrying clones (Estrada et al., 2007; Schmidt et al., 2015; Ao et al., 2017; Cecil et al., 2020), and increased mortality within the first few days after farrowing is often observed with cloned pigs (Ao et al., 2017). Birth weight may be a predictor of survival probability for cloned piglets as those that died within 4 days after birth weighed ~28% less than those that survived for more than 4 days (Ao et al., 2017). As a contributing factor to perinatal mortality, abnormal umbilical cord vasculature and low placental weight as well as placental malformations have been observed for cloned fetuses and piglets, likely impairing nutrient transport and gas exchange (Lee et al., 2007; Ao et al., 2017). Regarding placentas of cloned neonatal piglets, presence of infarcts and villous hypoplasia may be the result of upregulation of apoptotic signaling pathways, which were detected by proteomic analyses (Lee et al., 2007). To overcome issues with stillbirths, lack of mammary development in surrogates carrying clones, and delayed initiation of parturition, a C-section is typically performed on day 116 of gestation (Carter et al., 2002; Whyte et al., 2011). Delivery by C-section has been shown to decrease post-natal mortality over vaginal delivery by 20% (Schmidt et al., 2011).
Anatomical abnormalities can also be present in cloned piglets and are occasionally seen in piglets derived by IVF. Examples of abnormalities that can be observed by external examination include macroglossia, cleft palate, macrocephaly, flexor tendon contracture, polydactyly, kyphosis, and umbilical hernia, among others (Carter et al., 2002; Schmidt et al., 2015). Some of the abnormalities, such as flexor tendon contractures, can be corrected with physical therapy (Carter et al., 2002), but most abnormalities often lead to loss of the affected piglet. Internal abnormalities of the digestive tract include short or absent intestines and small or absent gall bladder (Schmidt et al., 2015). Cloned piglets can also have various heart and circulatory system defects that may lead to congestive heart failure (Carter et al., 2002; Schmidt et al., 2015). Lastly, issues of the reproductive system have been observed in cloned piglets, such as absent gonads, cryptorchidism, and enlarged gubernaculum (Schmidt et al., 2015).
Analysis of muscle tissues from normal and abnormal cloned piglets revealed numerous differentially expressed genes, such as those for the MAPK signaling pathway, the hypertrophic cardiomyopathy pathway, and imprinting, as well as altered DNA methylation patterns that could be involved in abnormality manifestation (Li et al., 2014). Although developmental abnormalities observed in SCNT-derived piglets are potentially linked to incomplete or faulty reprogramming of the donor cell nucleus after SCNT, the overall IVP system is suggested to contribute to the abnormalities as well.
Improvements Required to Expand the Use of In Vitro-Produced Porcine Embryos
Application of ART, such as IVF, embryo transfer, and SCNT, facilitates rapid genetic improvements and are heavily used parts of the livestock industry. Regarding the cattle industry, the number of transfers by using IVP cattle embryos increased 7.3% from 2018 to 2019, pointing to a heavy reliance on these technologies (Viana, 2020). However, application of ART is limited in the swine industry, partially due to difficulties in embryo production. In vitro production of porcine embryos has not been adopted by this industry for several reasons. Since pigs have litters and a short generation interval, there is less incentive to incorporate ART to facilitate genetic improvements as compared to cattle who have single offspring and longer generation intervals. In pigs, establishment of pregnancy is only possible when at least two embryos are present in each uterine horn (Dziuk, 1968). Thus, a large number of IVP embryos must be transferred into a surrogate for a pregnancy to be established. Conventionally, a minimum of 150 one-cell stage embryos or 30 blastocyst-stage embryos are transferred into a single surrogate pig. Other barriers hindering incorporation of IVP embryos in the swine industry include high rates of polyspermy, lack of non-surgical oocyte recovery or embryo transfer procedures, and unsuccessful cryopreservation.
Reducing Polyspermy
In vitro fertilization of porcine oocytes matured in vitro results in high rates of polyspermy that decreases development to the blastocyst stage and the number of cells in the ICM (Han et al., 1999b). The penetration of more than one spermatozoon often leads to developmental arrest of IVF embryos, thus impeding the use of IVF for pig production purposes. In pigs, the block to polyspermy is a two-step mechanism; the fast block occurs at the oocyte plasma membrane and the slow block occurs at the zona pellucida after cortical granule exocytosis. Specifically, the fast block is associated with shedding of folate receptor 4 (JUNO) from the oocyte plasma membrane which acts as the receptor for Izumo sperm-egg fusion 1 (IZUMO1) on the sperm head (Bianchi et al., 2014). The slow block is triggered by sperm-induced release of ions (i.e., calcium and zinc) followed by cortical granule exocytosis (Abbott and Ducibella, 2001; Tokuhiro and Dean, 2018). The released cortical granules induce cleavage of zona protein 2 (ZP2), leading to increased zona hardening (Boccaccio et al., 2012; Burkart et al., 2012). Moreover, zinc released after sperm penetration can bind ZP1, which contributes to further crosslinking of the zona pellucida (Nishimura et al., 2019). Zona hardening has also been shown to be influenced by lectins and glycosidases in the mouse (Dolci et al., 1991), and treatment of porcine oocytes with different lectins decreased sperm binding to the zona pellucida (Hwang et al., 2002). Factors responsible for proper zona hardening are present within the oviductal fluid of pigs, partially explaining why this process is diminished during IVF (Mondéjar et al., 2013). Although polyspermy generally results in developmental arrest during preimplantation stages, accessory sperm heads have been detected within phagolysosomes of porcine embryos, suggesting that embryos possess a mechanism to degrade unnecessary spermatozoa for survival of the embryo (Xia et al., 2001). In fact, polyspermic porcine embryos could develop to term after transfer, and the piglets had the correct number of chromosomes (Han et al., 1999a).
Oocyte quality is known to be correlated with incidence of polyspermy and in vitro-matured oocytes present lower quality and higher rates of polyspermy. Components of porcine oviductal fluid, such as oviductal glycoprotein 1, osteopontin, and plasminogen, have been shown to reduce the number of penetrating sperm (Hao et al., 2006; Coy et al., 2008, 2012). A recently developed porcine IVF system increased the pH from 7.4 to 8.0, which is physiological for the oviductal ampulla, added oviductal fluid, and installed a barrier between gametes to promote sperm chemotaxis (Soriano-Úbeda et al., 2017). The system decreased sperm penetration by 21.8%; however, monospermy of the fertilized oocytes increased from 26.4 to 88.7% in the new system. Similarly, a porcine IVM system with the presence of C-X-C motif chemokine ligand 12 (CXCL12), vascular endothelial growth factor A (VEGFA), and Wingless-type MMTV integration site family member 5A (WNT5A), increased nuclear maturation, development to the blastocyst stage, and retraction of transzonal projections with decreased incidence of polyspermy (Liu et al., 2020). Retraction of transzonal projections can promote cortical granule migration and exocytosis (Galeati et al., 1991).
As previously mentioned, addition of FLI to the porcine oocyte maturation system improved developmental competence of subsequent embryos. Moreover, oocytes matured in the presence of FLI presented decreased transzonal projections during IVM (Yuan et al., 2017). It is not clear whether the improved efficiency of IVM by using FLI is related to its ability to reduce polyspermy by decreasing transzonal projections as this has yet to be evaluated.
Non-surgical Embryo Transfer
The ability to conveniently transfer IVP embryos into surrogates is warranted for broad incorporation of IVF and SCNT technologies into the swine industry. However, unlike other livestock species, such as cows, routine non-surgical transfers in pigs have been limited due to the complexity of the female reproductive tract. Since the spiral shape of the cervix complements the male anatomy, passing a catheter through the cervix to reach the uterus is challenging as well as the fact that the uterine horns are long and narrow compared to other species. For transfer of IVP embryos in the research setting, a midventral laparotomy is performed to expose one ovary and oviduct, and the embryos are deposited in the ampullary-isthmic junction. Successful pregnancies have been established when morula and blastocyst-stage embryos are surgically transferred into a surrogate gilt (Chen et al., 2018, 2021; Koppes et al., 2020; Pfeiffer et al., 2020); however, this procedure is time-consuming and requires specialized skills, thus limiting the use of IVP embryos in the swine industry.
Non-surgical embryo transfers have been accomplished in pigs (averaging 2.5 min per procedure) by using an artificial insemination spirette to guide a modified flexible catheter for deposition of the embryos in the uterus (Li et al., 1996; Martinez et al., 2004). After 24 transfers of in vivo-derived embryos, 17 surrogates became pregnant (71%) and farrowed an average of 6.9±0.7 piglets. The type of female, gilt or sow, did not impact success of the transfer, but more insertion force was needed to pass through the cervical canal of the gilts compared to the sows, as expected (Martinez et al., 2004). One major risk factor associated with this method is the possibility of puncturing the uterine wall with the catheter which could lead to infection. Furthermore, the number of embryos transferred is important as non-surgical transfer of 30 in vivo-derived blastocyst-stage embryos resulted in a farrowing rate of 38.9% (5.7 ± 2.4 piglets per litter) while transfer of 40 blastocyst-stage embryos resulted in a farrowing rate of 72.7% (9.9 ± 2.1 piglets per litter) (Martinez et al., 2015). Although the reports certainly encourage the use of non-surgical embryo transfer in pigs, only in vivo-derived embryos have been used in these studies. As pointed out previously, developmental potential of in vivo-derived embryos far surpasses IVP embryos, and the need for non-surgical transfers is primarily related to IVP embryos. Reports on the effectiveness of non-surgical embryo transfer using IVP embryos should clarify whether the technology can be effectively used in the industry.
Cryopreservation
While cryopreservation of cells from pigs, such as fibroblast cells, is straightforward, cryopreservation of porcine gametes and embryos has been a challenge. Successful cryopreservation of boar sperm is season-, breed-, boar- and ejaculate-specific. Because of this variability, most artificial insemination in the swine industry is performed with fresh semen. For those ejaculates where sperm survive the cryopreservation, frozen semen results in a 20–30% decrease in farrowing rate with reduced litter sizes as compared to fresh semen (Silva et al., 2015).
Successful cryopreservation of embryos is even more challenging. The most consistent success is achieved after centrifugation of the early embryo and micromanipulation removal of the lipids prior to culture to the blastocyst stage and subsequent cryopreservation (Nagashima et al., 1995). Counterintuitively, removal of the lipids enhances development of the embryo rather than hinders it (Li et al., 2006), questioning the role of lipids in porcine embryo development. Transferring 163 delipidized, vitrified blastocyst-stage embryos into two surrogates resulted in a total of 10 piglets between the two pregnancies, confirming that these procedures are compatible with development in vivo (Li et al., 2006). Since the lipid removal procedure by micromanipulation is very labor intensive, a similar strategy was developed that separated the lipids through centrifugation but did not remove them from within the zona pellucida as a high-throughput method of cryopreservation for IVP embryos (Spate et al., 2013). An alternative method uses solid surface vitrification, whereby 50 oocytes or zygotes are moved through a series of equilibration and vitrification media and placed onto a cooling surface, such as aluminum foil, sitting on top of liquid nitrogen before being transferred to cryovials (Somfai and Kikuchi, 2021).
While there are a few reports of more conventional cryopreservation, those reports of successful cryopreservation of early pig embryos have not been widely repeatable, and the industry has not adopted the transfer of frozen embryos as a method of improving genetics or moving genetics around the world. In vivo-derived embryos survive cryopreservation better than IVP embryos. As discussed previously, this difference in survival may be a result of inherent developmental competence as oocytes derived from sexually mature animals are more developmentally competent than from sexually immature animals. Tajima et al. (2020) reported 35 embryo transfers that used 553 embryos resulting in 14 litters and 59 piglets (59/553 = 10%), and Hirayama et al. (2020) reported 12 embryo transfers with 180 embryos resulting in 8 sows farrowing and 37 piglets (20%). However, in both cases, it is not clear how many embryos were cryopreserved as only the number transferred is reported. Thus, the percentages may overestimate the efficiency based on the number of embryos frozen. Maturation in the presence of FLI increased the quality of oocytes derived from prepubertal gilts, and similar improvements on the maturation and culture conditions may enhance the survivability of pig embryos after cryopreservation as has been reported for cattle (Stoecklein et al., 2021). Improvements in cryopreservation for porcine gametes and embryos will considerably enhance the applicability of in vitro technologies for the swine industry, allowing for rapid transfer of genetics at the national and international levels.
Conclusion
Over the years, considerable improvements have been made for IVP of porcine embryos. Pigs produced through these methods have been instrumental for advancements in biomedicine and animal agriculture. However, the success of porcine embryo IVP has not yet reached a level for this technology to be adopted by the swine industry as embryo viability is still decreased compared to in vivo-derived embryos. There have been several advances in porcine oocyte maturation and embryo culture, but certain areas require more attention to improve the IVP process as a whole. Lowering rates of polyspermy in IVP embryos, developing reliable methods of non-surgical transfer, and enhancing cryopreservation success of gametes and embryos are among some of the areas where improvements can be made and would be critical for use of IVP by the swine industry. Altogether, improvements in each step of IVP of porcine embryos will lead to the ultimate goal of increasing the pregnancy rate and number of live piglets from each pregnancy.
Author Contributions
PC, RP, and KL created an outline for the review. PC, BR, KU, RP, and KL wrote the review. ER prepared the figure. All authors revised the manuscript. All authors contributed to the article and approved the submitted version.
Funding
Funding was provided by the Cooperative Research Project (Project Number PJ013695) from the Rural Development Administration (Republic of Korea) and by the National Institute of Allergy and Infectious Disease, the National Institute of Heart, Lung and Blood, and the Office of the Director (U42OD011140) for the National Swine Resource and Research Center.
Conflict of Interest
The authors declare that the research was conducted in the absence of any commercial or financial relationships that could be construed as a potential conflict of interest.
The handling editor VM declared a past co-authorship with the author KL.
Publisher's Note
All claims expressed in this article are solely those of the authors and do not necessarily represent those of their affiliated organizations, or those of the publisher, the editors and the reviewers. Any product that may be evaluated in this article, or claim that may be made by its manufacturer, is not guaranteed or endorsed by the publisher.
References
Abbott, A. L., and Ducibella, T. (2001). Calcium and the control of mammalian cortical granule exocytosis. Front. Biosci. 6, D792–D806. doi: 10.2741/Abbott
Ao, Z., Liu, D., Zhao, C., Yue, Z., Shi, J., Zhou, R., et al. (2017). Birth weight, umbilical and placental traits in relation to neonatal loss in cloned pigs. Placenta 57, 94–101. doi: 10.1016/j.placenta.2017.06.010
Archibong, A. E., Petters, R. M., and Johnson, B. H. (1989). Development of porcine embryos from one- and two-cell stages to blastocysts in culture medium supplemented with porcine oviductal fluid. Biol. Reprod. 41, 1076–1083. doi: 10.1095/biolreprod41.6.1076
Bagg, M. A., Nottle, M. B., Armstrong, D. T., and Grupen, C. G. (2007). Relationship between follicle size and oocyte developmental competence in prepubertal and adult pigs. Reprod. Fertil. Dev. 19, 797–803. doi: 10.1071/RD07018
Baltz, J. M., and Tartia, A. P. (2010). Cell volume regulation in oocytes and early embryos: connecting physiology to successful culture media. Hum. Reprod. Update 16, 166–176. doi: 10.1093/humupd/dmp045
Bauer, B. K., Isom, S. C., Spate, L. D., Whitworth, K. M., Spollen, W. G., Blake, S. M., et al. (2010). Transcriptional profiling by deep sequencing identifies differences in mrna transcript abundance in in vivo-derived versus in vitro-cultured porcine blastocyst stage embryos. Biol. Reprod. 83, 791–798. doi: 10.1095/biolreprod.110.085936
Beckmann, L. S., and Day, B. N. (1993). Effects of media NaCl concentration and osmolarity on the culture of early-stage porcine embryos and the viability of embryos cultured in a selected superior medium. Theriogenology 39, 611–622. doi: 10.1016/0093-691X(93)90248-4
Bianchi, E., Doe, B., Goulding, D., and Wright, G. J. (2014). Juno is the egg Izumo receptor and is essential for mammalian fertilization. Nature 508, 483–487. doi: 10.1038/nature13203
Boccaccio, A., Frassanito, M. C., Lamberti, L., Brunelli, R., Maulucci, G., Monaci, M., et al. (2012). Nanoscale characterization of the biomechanical hardening of bovine zona pellucida. J. R. Soc. Interface 9, 2871. doi: 10.1098/rsif.2012.0269
Bonk, A. J., Cheong, H. T., Li, R., Lai, L., Hao, Y., Liu, Z., et al. (2007). Correlation of developmental differences of nuclear transfer embryos cells to the methylation profiles of nuclear transfer donor cells in swine. Epigenetics 2, 179. doi: 10.4161/epi.2.3.4844
Bonk, A. J., Li, R., Lai, L., Hao, Y., Liu, Z., Samuel, M., et al. (2008). Aberrant DNA methylation in porcine in vitro-, parthenogenetic-, and somatic cell nuclear transfer-produced blastocysts. Mol. Reprod. Dev. 75, 250. doi: 10.1002/mrd.20786
Burkart, A. D., Xiong, B., Baibakov, B., Jiménez-Movilla, M., and Dean, J. (2012). Ovastacin, a cortical granule protease, cleaves ZP2 in the zona pellucida to prevent polyspermy. J. Cell Biol. 197, 37. doi: 10.1083/jcb.201112094
Canovas, S., Ivanova, E., Romar, R., García-Martínez, S., Soriano-Úbeda, C., García-Vázquez, F. A., et al. (2017). DNA methylation and gene expression changes derived from assisted reproductive technologies can be decreased by reproductive fluids. Elife 6:e23670. doi: 10.7554/eLife.23670.027
Carter, D. B., Lai, L., Park, K. W., Samuel, M., Lattimer, J. C., Jordan, K. R., et al. (2002). Phenotyping of transgenic cloned piglets. Cloning Stem Cells 4, 131–145. doi: 10.1089/153623002320253319
Cecil, R. F., Chen, P. R., Benne, J. A., Hord, T. K., Spate, L. D., Samuel, M. S., et al. (2020). Chemical simulation of hypoxia in donor cells improves development of somatic cell nuclear transfer-derived embryos and increases abundance of transcripts related to glycolysis. Mol. Reprod. Dev. 87, 763–772. doi: 10.1002/mrd.23392
Chen, P. R., Lucas, C. G., Cecil, R. F., Pfeiffer, C. A., Fudge, M. A., Samuel, M. S., et al. (2021). Disrupting porcine glutaminase does not block preimplantation development and elongation nor decrease mTORC1 activation in conceptuses. Biol. Reprod. 105:1104–1113. doi: 10.1093/biolre/ioab165
Chen, P. R., Redel, B. K., Spate, L. D., Ji, T., Salazar, S. R., and Prather, R. S. (2018). Glutamine supplementation enhances development of in vitro-produced porcine embryos and increases leucine consumption from the medium. Biol. Reprod. 99, 938–948. doi: 10.1093/biolre/ioy129
Chen, P. R., Spate, L. D., Leffeler, E. C., Benne, J. A., Cecil, R. F., Hord, T. K., et al. (2020). Removal of hypotaurine from porcine embryo culture medium does not impair development of in vitro-fertilized or somatic cell nuclear transfer-derived embryos at low oxygen tension. Mol. Reprod. Dev. 87, 773–782. doi: 10.1002/mrd.23393
Coy, P., Cánovas, S., Mondéjar, I., Saavedra, M. D., Romar, R., Grullón, L., et al. (2008). Oviduct-specific glycoprotein and heparin modulate sperm–zona pellucida interaction during fertilization and contribute to the control of polyspermy. Proc. Natl. Acad. Sci. U. S. A. 105, 15809. doi: 10.1073/pnas.0804422105
Coy, P., Jiménez-Movilla, M., García-Vázquez, F. A., Mondéjar, I., Grullón, L., and Romar, R. (2012). Oocytes use the plasminogen-plasmin system to remove supernumerary spermatozoa. Hum. Reprod. 27, 1985–1993. doi: 10.1093/humrep/des146
Davis, D. L., and Day, B. N. (1978). Cleavage and blastocyst formation by pig eggs in vitro. J. Anim. Sci. 46, 1043–1053. doi: 10.2527/jas1978.4641043x
Deshmukh, R. S., Østrup, O., Østrup, E., Vejlsted, M., Niemann, H., Lucas-Hahn, A., et al. (2011). DNA methylation in porcine preimplantation embryos developed in vivo and produced by in vitro fertilization, parthenogenetic activation and somatic cell nuclear transfer. Epigenetic 6, 177–187. doi: 10.4161/epi.6.2.13519
Dolci, S., Bertolani, M., Canipari, R., and De Felici, M. (1991). Involvement of carbohydrates in the hardening of the zona pellucida of mouse oocytes. Cell Biol. Int. Rep. 15, 571–579. doi: 10.1016/0309-1651(91)90004-3
Dziuk, P. J. (1968). Effect of number of embryos and uterine space on embryo survival in the pig. J. Anim. Sci. 27, 673–676. doi: 10.2527/jas1968.273673x
El Hajj, N., and Haaf, T. (2013). Epigenetic disturbances in in vitro cultured gametes and embryos: implications for human assisted reproduction. Fertil. Steril. 99, 632–641. doi: 10.1016/j.fertnstert.2012.12.044
Eppig, J. J., Wigglesworth, K., Pendola, F., and Hirao, Y. (1997). Murine oocytes suppress expression of luteinizing hormone receptor messenger ribonucleic acid by granulosa cells. Biol. Reprod. 56, 976–984. doi: 10.1095/biolreprod56.4.976
Estrada, J., Sommer, J., Collins, B., Mir, B., Martin, A., York, A., et al. (2007). Swine generated by somatic cell nuclear transfer have increased incidence of intrauterine growth restriction (IUGR). Cloning Stem Cells 9, 229–236. doi: 10.1089/clo.2006.0079
Fan, H.-Y., Liu, Z., Shimada, M., Sterneck, E., Johnson, P. F., Hedrick, S. M., et al. (2009). MAPK3/1 (ERK1/2) in ovarian granulosa cells are essential for female fertility. Science 324, 938. doi: 10.1126/science.1171396
Galeati, G., Modina, S., Lauria, A., and Mattioli, M. (1991). Follicle somatic cells influence pig oocyte penetrability and cortical granule distribution. Mol. Reprod. Dev. 29, 40–46. doi: 10.1002/mrd.1080290107
Gaspar, R. C., Arnold, D. R., Corrêa, C. A. P., da Rocha, C. V., Penteado, J. C. T., del Collado, M., et al. (2015). Oxygen tension affects histone remodeling of in vitro-produced embryos in a bovine model. Theriogenology 83, 1408–1415. doi: 10.1016/j.theriogenology.2015.01.002
Gilchrist, R. B., Lane, M., and Thompson, J. G. (2008). Oocyte-secreted factors: regulators of cumulus cell function and oocyte quality. Hum. Reprod. Update 14, 159–177. doi: 10.1093/humupd/dmm040
Gilchrist, R. B., and Thompson, J. G. (2007). Oocyte maturation: emerging concepts and technologies to improve developmental potential in vitro. Theriogenology 67, 6–15. doi: 10.1016/j.theriogenology.2006.09.027
Hadi, T., Hammer, M.-A., Algire, C., Richards, T., and Baltz, J. M. (2005). Similar effects of osmolarity, glucose, and phosphate on cleavage past the 2-cell stage in mouse embryos from outbred and F1 hybrid females. Biol. Reprod. 72, 179–187. doi: 10.1095/biolreprod.104.033324
Hagen, D., Prather, R., Sims, M., and First, N. (1991). Development of one-cell porcine embryos to the blastocyst stage in simple media. J Anim Sci 69, 1147–1150. doi: 10.2527/1991.6931147x
Han, Y.-M., Wang, W.-H., Abeydeera, L. R., Petersen, A. L., Kim, J.-H., Murphy, C., et al. (1999a). Pronuclear location before the first cell division determines ploidy of polyspermic pig embryos. Biol. Reprod. 61, 1340–1346. doi: 10.1095/biolreprod61.5.1340
Han, Y. M., Abeydeera, L. R., Kim, J. H., Moon, H. B., Cabot, R. A., Day, B. N., et al. (1999b). Growth retardation of inner cell mass cells in polyspermic porcine embryos produced in vitro. Biol. Reprod. 60, 1110–1113. doi: 10.1095/biolreprod60.5.1110
Hao, Y., Mathialagan, N., Walters, E., Mao, J., Lai, L., Becker, D., et al. (2006). Osteopontin reduces polyspermy during in vitro fertilization of porcine oocytes. Biol. Reprod. 75, 726–733. doi: 10.1095/biolreprod.106.052589
Hao, Y., Wax, D., Zhong, Z., Murphy, C., Ross, J. W., Rieke, A., et al. (2009). Porcine skin-derived stem cells can serve as donor cells for nuclear transfer. Cloning Stem Cells 11, 101. doi: 10.1089/clo.2008.0063
Hendricks-Wenger, A., Aycock, K. N., Nagai-Singer, M. A., Coutermarsh-Ott, S., Lorenzo, M. F., Gannon, J., et al. (2021). Establishing an immunocompromised porcine model of human cancer for novel therapy development with pancreatic adenocarcinoma and irreversible electroporation. Sci. Rep. 11, 1–14. doi: 10.1038/s41598-021-87228-5
Hirayama, Y., Takishita, R., Misawa, H., Kikuchi, K., Misumi, K., Egawa, S., et al. (2020). Non-surgical transfer of vitrified porcine embryos using a catheter designed for a proximal site of the uterus. Anim. Sci. J. 91, 1–9. doi: 10.1111/asj.13457
Hsieh, M., Lee, D., Panigone, S., Horner, K., Chen, R., Theologis, A., et al. (2007). Luteinizing hormone-dependent activation of the epidermal growth factor network is essential for ovulation. Mol. Cell. Biol. 27, 1914. doi: 10.1128/MCB.01919-06
Huan, Y., Wang, H., Wu, Z., Zhang, J., Zhu, J., Liu, Z., et al. (2015a). Epigenetic modification of cloned embryos improves nanog reprogramming in pigs. Cell. Reprogram. 17, 191. doi: 10.1089/cell.2014.0103
Huan, Y., Wu, Z., Zhang, J., Zhu, J., Liu, Z., and Song, X. (2015b). Epigenetic modification agents improve gene-specific methylation reprogramming in porcine cloned embryos. PLoS ONE 10, e0129803. doi: 10.1371/journal.pone.0129803
Huan, Y. J., Zhu, J., Wang, H. M., Wu, Z. F., Zhang, J. G., Xie, B. T., et al. (2014). Epigenetic modification agents improve genomic methylation reprogramming in porcine cloned embryos. J. Reprod. Dev. 60, 377. doi: 10.1262/jrd.2014-062
Huang, J., Zhang, H., Yao, J., Qin, G., Wang, F., Wang, X., et al. (2016). BIX-01294 increases pig cloning efficiency by improving epigenetic reprogramming of somatic cell nuclei. Reproduction 151, 39–49. doi: 10.1530/REP-15-0460
Hwang, I. S., Kim, C. I., Cheong, H. T., Yang, B. K., and Park, C. K. (2002). Binding of lectins to the zona pellucida on sperm-oocytes interaction in the pig. Korean J. Fertil. Steril. 29, 179–186.
Jeong, P.-S., Yoon, S.-B., Choi, S.-A., Song, B.-S., Kim, J.-S., Sim, B.-W., et al. (2017). Iloprost supports early development of in vitro-produced porcine embryos through activation of the phosphatidylinositol 3-kinase/AKT signalling pathway. Reprod. Fertil. Dev. 29, 1306–1318. doi: 10.1071/RD15391
Jeong, P. S., Sim, B. W., Park, S. H., Kim, M. J., Kang, H. G., Nanjidsuren, T., et al. (2020). Chaetocin improves pig cloning efficiency by enhancing epigenetic reprogramming and autophagic activity. Int. J. Mol. Sci. 21, 1–19. doi: 10.3390/ijms21144836
Kang, Y. K., Koo, D. B., Park, J. S., Choi, Y. H., Kim, H. N., Chang, W. K., et al. (2001). Typical demethylation events in cloned pig embryos: clues on species-specific differences in epigenetic reprogramming of a cloned donor genome. J. Biol. Chem. 276, 39980–39984. doi: 10.1074/jbc.M106516200
Kawashima, I., Okazaki, T., Noma, N., Nishibori, M., Yamashita, Y., and Shimada, M. (2008). Sequential exposure of porcine cumulus cells to FSH and/or LH is critical for appropriate expression of steroidogenic and ovulation-related genes that impact oocyte maturation in vivo and in vitro. Reproduction 136, 9–21. doi: 10.1530/REP-08-0074
Koppes, E. A., Redel, B. K., Johnson, M. A., Skvorak, K. J., Ghaloul-Gonzalez, L., Yates, M. E., et al. (2020). A porcine model of phenylketonuria generated by CRISPR/Cas9 genome editing. JCI Insight 5, 1–18. doi: 10.1172/jci.insight.141523
Krisher, R., Petters, R., Johnson, B., Bavister, B., and Archibong, A. (1989). Development of porcine embryos from the one-cell stage to blastocyst in mouse oviducts maintained in organ culture. J. Exp. Zool. 249, 235–239. doi: 10.1002/jez.1402490217
Kwon, D.-N., Lee, K., Kang, M.-J., Choi, Y.-J., Park, C., Whyte, J. J., et al. (2013). Production of biallelic CMP-Neu5Ac hydroxylase knock-out pigs. Sci. Rep. 3, 1981. doi: 10.1038/srep01981
Kwon, D. J., Park, C. K., Yang, B. K., and Cheong, H. T. (2008). Control of nuclear remodelling and subsequent in vitro development and methylation status of porcine nuclear transfer embryos. Reproduction 135, 649–656. doi: 10.1530/REP-06-0387
Lai, L., Kang, J., Li, R., Wang, J., Witt, W., Yong, H., et al. (2006). Generation of cloned transgenic pigs rich in omega-3 fatty acids. Nat. Biotechnol. 24, 435–436. doi: 10.1038/nbt1198
Lai, L., Kolber-Simonds, D., Park, K., Cheong, H., Greenstein, J., Im, G., et al. (2002). Production of alpha-1,3-galactosyltransferase knockout pigs by nuclear transfer cloning. Science 295, 1089–1092. doi: 10.1126/science.1068228
Lai, L., and Prather, R. S. (2003). Production of cloned pigs by using somatic cells as donors. Cloning Stem Cells 5, 233–241. doi: 10.1089/153623003772032754
Lee, K., Davis, A., Zhang, L., Ryu, J., Spate, L. D., Park, K.-W., et al. (2015). Pig oocyte activation utilizing a Zn2+ chelator, TPEN. Theriogenology 84, 1024. doi: 10.1016/j.theriogenology.2015.05.036
Lee, K., Kwon, D.-N., Ezashi, T., Choi, Y.-J., Park, C., Ericsson, A. C., et al. (2014). Engraftment of human iPS cells and allogeneic porcine cells into pigs with inactivated RAG2 and accompanying severe combined immunodeficiency. Proc. Natl. Acad. Sci. U. S. A. 111, 7260–7265. doi: 10.1073/pnas.1406376111
Lee, K., Redel, B. K., Spate, L., Teson, J., Brown, A. N., Park, K., et al. (2013). Piglets produced from cloned blastocysts cultured in vitro with GM-CSF. Mol. Reprod. Dev. 80, 145. doi: 10.1002/mrd.22143
Lee, S. Y., Park, J. Y., Choi, Y. J., Cho, S. K., Jong, D. A., Kwon, D. N., et al. (2007). Comparative proteomic analysis associated with term placental insufficiency in cloned pig. Proteomics 7, 1303–1315. doi: 10.1002/pmic.200601045
Lei, S., Ryu, J., Wen, K., Twitchell, E., Bui, T., Ramesh, A., et al. (2016). Increased and prolonged human norovirus infection in RAG2/IL2RG deficient gnotobiotic pigs with severe combined immunodeficiency. Sci. Rep. 6, 1–12. doi: 10.1038/srep25222
Li, G., Jia, Q., Zhao, J., Li, X., Yu, M., Samuel, M. S., et al. (2014). Dysregulation of genome-wide gene expression and DNA methylation in abnormal cloned piglets. BMC Genomics 15, 811. doi: 10.1186/1471-2164-15-811
Li, J., Rieke, A., Day, B. N., and Prather, R. S. (1996). Technical note: porcine non-surgical embryo transfer. J. Anim. Sci. 74, 2263–2268. doi: 10.2527/1996.7492263x
Li, J., Svarcova, O., Villemoes, K., Kragh, P. M., Schmidt, M., Bøgh, I. B., et al. (2008). High in vitro development after somatic cell nuclear transfer and trichostatin A treatment of reconstructed porcine embryos. Theriogenology 70, 800–808. doi: 10.1016/j.theriogenology.2008.05.046
Li, R., Lai, L., Wax, D., Hao, Y., Murphy, C. N., Rieke, A., et al. (2006). Cloned transgenic swine via in vitro production and cryopreservation. Biol. Reprod. 75, 226–230. doi: 10.1095/biolreprod.106.052514
Liang, S., Zhao, M.-H., Choi, J., Kim, N.-H., and Cui, X.-S. (2015). Scriptaid treatment decreases DNA methyltransferase 1 expression by induction of microRNA-152 expression in porcine somatic cell nuclear transfer embryos. PLoS ONE 10, e0134567. doi: 10.1371/journal.pone.0134567
Liu, X., Hao, Y., Li, Z., Zhou, J., Zhu, H., Bu, G., et al. (2020). Maternal ytokines CXCL12, VEGFA, and WNT5A promote porcine oocyte maturation via MAPK activation and canonical WNT inhibition. Front. Cell Dev. Biol. 8, 578. doi: 10.3389/fcell.2020.00578
Lonergan, P., Rizos, D., Gutierrez-Adan, A., Fair, T., and Boland, M. (2003). Oocyte and embryo quality: effect of origin, culture conditions and gene expression patterns. Reprod. Domest. Anim. 38, 259–267. doi: 10.1046/j.1439-0531.2003.00437.x
Macháty, Z., Day, B. N., and Prather, R. S. (1998). Development of early porcine embryos in vitro and in vivo. Biol. Reprod. 59, 451–455. doi: 10.1095/biolreprod59.2.451
Madgwick, S., Nixon, V. L., Chang, H. Y., Herbert, M., Levasseur, M., and Jones, K. T. (2004). Maintenance of sister chromatid attachment in mouse eggs through maturation-promoting factor activity. Dev. Biol. 275, 68–81. doi: 10.1016/j.ydbio.2004.07.024
Mann, M. R. W., and Bartolomei, M. S. (2002). Epigenetic reprogramming in the mammalian embryo: struggle of the clones. Genome Biol. 3, REVIEWS1003. doi: 10.1186/gb-2002-3-2-reviews1003
Marchal, R., Feugang, J. M., Perreau, C., Venturi, E., Terqui, M., and Mermillod, P. (2001). Meiotic and developmental competence of prepubertal and adult swine oocytes. Theriogenology 56, 17–29. doi: 10.1016/S0093-691X(01)00539-8
Martinez, E. A., Angel, M. A., Cuello, C., Sanchez-Osorio, J., Gomis, J., Parrilla, I., et al. (2014). Successful non-surgical deep uterine transfer of porcine morulae after 24 hour culture in a chemically defined medium. PLoS ONE 9, e104696. doi: 10.1371/journal.pone.0104696
Martinez, E. A., Caamaño, J. N., Gil, M. A., Rieke, A., McCauley, T. C., Cantley, T. C., et al. (2004). Successful nonsurgical deep uterine embryo transfer in pigs. Theriogenology 61, 137–146. doi: 10.1016/S0093-691X(03)00190-0
Martinez, E. A., Martinez, C. A., Nohalez, A., Sanchez-Osorio, J., Vazquez, J. M., Roca, J., et al. (2015). Nonsurgical deep uterine transfer of vitrified, in vivo-derived, porcine embryos is as effective as the default surgical approach. Sci. Rep. 5, 1–9. doi: 10.1038/srep10587
Mehlmann, L. M., Saeki, Y., Tanaka, S., Brennan, T. J., Evsikov, A. V., Pendola, F. L., et al. (2004). The Gs-linked receptor GPR3 maintains meiotic arrest in mammalian oocytes. Science 306, 1947–1950. doi: 10.1126/science.1103974
Menino, A. R., and Wright, R. W. (1982). Development of one-cell porcine embryos in two culture systems. J. Anim. Sci. 54, 583–588. doi: 10.2527/jas1982.543583x
Messerschmidt, D. M., Knowles, B. B., and Solter, D. (2014). DNA methylation dynamics during epigenetic reprogramming in the germline and preimplantation embryos. Genes Dev. 28, 812. doi: 10.1101/gad.234294.113
Mondéjar, I., Martínez-Martínez, I., Avilés, M., and Coy, P. (2013). Identification of potential oviductal factors responsible for zona pellucida hardening and monospermy during fertilization in mammals. Biol. Reprod. 89, 67–68. doi: 10.1095/biolreprod.113.111385
Nagashima, H., Kashiwazaki, N., Ashman, R., Grupen, V. G., and Nottle, M. B. (1995). Cryopreservation of porcine embryos. Nature 374, 416. doi: 10.1038/374416a0
Nishimura, K., Dioguardi, E., Nishio, S., Villa, A., Han, L., Matsuda, T., et al. (2019). Molecular basis of egg coat cross-linking sheds light on ZP1-associated female infertility. Nat. Commun. 10, 1–15. doi: 10.1038/s41467-019-10931-5
Norris, R. P., Ratzan, W. J., Freudzon, M., Mehlmann, L. M., Krall, J., Movsesian, M. A., et al. (2009). Cyclic GMP from the surrounding somatic cells regulates cyclic AMP and meiosis in the mouse oocyte. Development 136, 1869. doi: 10.1242/dev.035238
Ogura, A., Inoue, K., and Wakayama, T. (2013). Recent advancements in cloning by somatic cell nuclear transfer. Philos. Trans. R. Soc. B Biol. Sci. 368, 20110329. doi: 10.1098/rstb.2011.0329
Park, C.-H., Jeong, Y. H., Jeong, Y.-I., Lee, S.-Y., Jeong, Y.-W., Shin, T., et al. (2012). X-linked gene transcription patterns in female and male in vivo, in vitro and cloned porcine individual blastocysts. PLoS ONE 7, e51398. doi: 10.1371/journal.pone.0051398
Park, C.-H., Uh, K.-J., Mulligan, B. P., Jeung, E.-B., Hyun, S.-H., Shin, T., et al. (2011). Analysis of imprinted gene expression in normal fertilized and uniparental preimplantation porcine embryos. PLoS ONE 6, e22216. doi: 10.1371/journal.pone.0022216
Park, K.-E., Kaucher, A. V., Powell, A., Waqas, M. S., Sandmaier, S. E. S., Oatley, M. J., et al. (2017). Generation of germline ablated male pigs by CRISPR/Cas9 editing of the NANOS2 gene. Sci. Rep. 7, 40176. doi: 10.1038/srep40176
Petters, R. M., and Wells, K. D. (1993). Culture of pig embryos. J. Reprod. Fertil. Suppl. 48, 61–73.
Pfeiffer, C. A., Meyer, A. E., Brooks, K. E., Chen, P. R., Milano-Foster, J., Spate, L. D., et al. (2020). Ablation of conceptus PTGS2 expression does not alter early conceptus development and establishment of pregnancy in the pig. Biol. Reprod. 102, 475–488. doi: 10.1093/biolre/ioz192
Prather, R. S. (2010). Whatever happened to the “cell-block” during mammalian embryogenesis? Mol. Reprod. Dev. 77. doi: 10.1002/mrd.21182
Prather, R. S., Sims, M. M., and First, N. L. (1991). Culture of porcine embryos from the one- and two-cell stage to the blastocyst stage in sheep oviducts. Theriogenology 35, 1147–1151. doi: 10.1016/0093-691X(91)90361-G
Procházka, R., Bartková, A., Němcová, L., Murín, M., Gad, A., Marcollová, K., et al. (2021). The role of MAPK3/1 and AKT in the acquisition of high meiotic and developmental competence of porcine oocytes cultured in vitro in FLI medium. Int. J. Mol. Sci. 22, 11148. doi: 10.3390/ijms222011148
Prochazka, R., Kalab, P., and Nagyova, E. (2003). Epidermal growth factor-receptor tyrosine kinase activity regulates expansion of porcine oocyte-cumulus cell complexes in vitro. Biol. Reprod. 68, 797–803. doi: 10.1095/biolreprod.102.005520
Redel, B. K., Spate, L. D., Lee, K., Mao, J., Whitworth, K. M., and Prather, R. S. (2016a). Glycine supplementation in vitro enhances porcine preimplantation embryo cell number and decreases apoptosis but does not lead to live births. Mol. Reprod. Dev. 83, 246–258. doi: 10.1002/mrd.22618
Redel, B. K., Spate, L. D., and Prather, R. S. (2019). In vitro maturation, fertilization, and culture of pig oocytes and embryos. Methods Mol. Biol. 2006, 93–103. doi: 10.1007/978-1-4939-9566-0_6
Redel, B. K., Spate, L. D., Yuan, Y., Murphy, C. N., Roberts, R. M., and Prather, R. S. (2021). Neither gonadotropin nor cumulus cell expansion is needed for the maturation of competent porcine oocytes in vitro†. Biol. Reprod. 105, 533–542. doi: 10.1093/biolre/ioab090
Redel, B. K., Tessanne, K. J., Spate, L. D., Murphy, C. N., and Prather, R. S. (2016b). Arginine increases development of in vitro produced porcine embryos and affects the PRMT-DDAH-NO Axis. Reprod. Ferti.l Dev. 27, 655–666. doi: 10.1071/RD14293
Reed, M., Illera, M., and Petters, R. (1992). In vitro culture of pig embryos. Theriogenology 37, 95–190. doi: 10.1016/0093-691X(92)90249-Q
Reik, W., Dean, W., and Walter, J. (2001). epigenetic reprogramming in mammalian development. Science 293, 1089–1093. doi: 10.1126/science.1063443
Richter, A., Kurome, M., Kessler, B., Zakhartchenko, V., Klymiuk, N., Nagashima, H., et al. (2012). Potential of primary kidney cells for somatic cell nuclear transfer mediated transgenesis in pig. BMC Biotechnol. 12, 84. doi: 10.1186/1472-6750-12-84
Rideout, W., Eggan, K., and Jaenisch, R. (2001). Nuclear cloning and epigenetic reprogramming of the genome. Science 293, 1093–1098. doi: 10.1126/science.1063206
Rogers, C. S., Hao, Y., Rokhlina, T., Samuel, M., Stoltz, D. A., Li, Y., et al. (2008). Production of CFTR-null and CFTR-DeltaF508 heterozygous pigs by adeno-associated virus-mediated gene targeting and somatic cell nuclear transfer. J. Clin. Invest. 118, 1571–1577. doi: 10.1172/JCI34773
Roy, P. K., Qamar, A. Y., Tanga, B. M., Fang, X., Kim, G., Bang, S., et al. (2021). Enhancing oocyte competence with milrinone as a phosphodiesterase 3A inhibitor to improve the development of porcine cloned embryos. Front. Cell Dev. Biol. 9, 647616. doi: 10.3389/fcell.2021.647616
Santos, F., Zakhartchenko, V., Stojkovic, M., Peters, A., Jenuwein, T., Wolf, E., et al. (2003). Epigenetic marking correlates with developmental potential in cloned bovine preimplantation embryos. Curr. Biol. 13, 1116–1121. doi: 10.1016/S0960-9822(03)00419-6
Schmidt, M., Winter, K. D., Dantzer, V., Li, J., Kragh, P. M., Du, Y., et al. (2011). Maternal endometrial oedema may increase perinatal mortality of cloned and transgenic piglets. Reprod. Fertil. Dev. 23, 645–653. doi: 10.1071/RD10220
Schmidt, M., Winther, K. D., Secher, J. O., and Callesen, H. (2015). Postmortem findings in cloned and transgenic piglets dead before weaning. Theriogenology 84, 1014–1023. doi: 10.1016/j.theriogenology.2015.05.037
Schook, L. B., Collares, T. V., Hu, W., Liang, Y., Rodrigues, F. M., Rund, L. A., et al. (2015). A genetic porcine model of cancer. PLoS ONE 10, e0128864. doi: 10.1371/journal.pone.0128864
Sela-Abramovich, S., Edry, I., Galiani, D., Nevo, N., and Dekel, N. (2006). Disruption of gap junctional communication within the ovarian follicle induces oocyte maturation. Endocrinology 147, 2280–2286. doi: 10.1210/en.2005-1011
Sheets, T. P., Park, K.-E., Park, C.-H., Swift, S. M., Powell, A., Donovan, D. M., et al. (2018). Targeted mutation of NGN3 gene disrupts pancreatic endocrine cell development in pigs. Sci. Rep. 8, 3582. doi: 10.1038/s41598-018-22050-0
Shimada, M., Hernandez-Gonzalez, I., Gonzalez-Robayna, I., and Richards, J. (2006). Paracrine and autocrine regulation of epidermal growth factor-like factors in cumulus oocyte complexes and granulosa cells: key roles for prostaglandin synthase 2 and progesterone receptor. Mol. Endocrinol. 20, 1352–1365. doi: 10.1210/me.2005-0504
Shimada, M., Nishibori, M., Isobe, N., Kawano, N., and Terada, T. (2003). Luteinizing hormone receptor formation in cumulus cells surrounding porcine oocytes and its role during meiotic maturation of porcine oocytes. Biol. Reprod. 68, 1142–1149. doi: 10.1095/biolreprod.102.010082
Silva, C. G., Cunha, E. R., Blume, G. R., Malaquias, J. V., Báo, S. N., and Martins, C. F. (2015). Cryopreservation of boar sperm comparing different cryoprotectants associated in media based on powdered coconut water, lactose and trehalose. Cryobiology 70, 90–94. doi: 10.1016/j.cryobiol.2015.01.001
Sirard, M. A. (2017). The influence of in vitro fertilization and embryo culture on the embryo epigenetic constituents and the possible consequences in the bovine model. J. Dev. Orig. Health Dis. 8, 411–417. doi: 10.1017/S2040174417000125
Somfai, T., and Kikuchi, K. (2021). Vitrification of porcine oocytes and zygotes in microdrops on a solid metal surface or liquid nitrogen. Methods Mol. Biol. 2180, 455–468. doi: 10.1007/978-1-0716-0783-1_21
Song, X., Liu, Z., He, H., Wang, J., and Li, H. (2017). Dnmt1s in donor cells is a barrier to SCNT-mediated DNA methylation reprogramming in pigs. Oncotarget 8, 34980–34991. doi: 10.18632/oncotarget.16507
Song, Y., Hai, T., Wang, Y., Guo, R., Li, W., Wang, L., et al. (2014). Epigenetic reprogramming, gene expression and in vitro development of porcine SCNT embryos are significantly improved by a histone deacetylase inhibitor—m-carboxycinnamic acid bishydroxamide (CBHA). Protein Cell 5, 382. doi: 10.1007/s13238-014-0034-3
Soriano-Úbeda, C., García-Vázquez, F. A., Romero-Aguirregomezcorta, J., and Matás, C. (2017). Improving porcine in vitro fertilization output by simulating the oviductal environment. Sci. Rep. 7, 1–12. doi: 10.1038/srep43616
Spate, L. D., Brown, A. N., Redel, B. K., Whitworth, K. M., and Prather, R. S. (2015). PS48 can replace bovine serum albumin in pig embryo culture medium, and improve in vitro embryo development by phosphorylating AKT. Mol. Reprod. Dev. 82, 315. doi: 10.1002/mrd.22474
Spate, L. D., Murphy, C. N., and Prather, R. S. (2013). High-throughput cryopreservation of in vivo-derived swine Embryos 8, 1–5. doi: 10.1371/journal.pone.0065545
Stoecklein, K. S., Sofia Ortega, M., Spate, L. D., Murphy, C. N., and Prather, R. S. (2021). Improved cryopreservation of in vitro produced bovine embryos using FGF2, LIF, and IGF1. PLoS ONE 16, e0243727. doi: 10.1371/journal.pone.0243727
Su, Y. Q., Denegre, J. M., Wigglesworth, K., Pendola, F. L., O'Brien, M. J., and Eppig, J. J. (2003). Oocyte-dependent activation of mitogen-activated protein kinase (ERK1/2) in cumulus cells is required for the maturation of the mouse oocyte–cumulus cell complex. Dev. Biol. 263, 126–138. doi: 10.1016/S0012-1606(03)00437-8
Suzuki, S., Iwamoto, M., Saito, Y., Fuchimoto, D., Sembon, S., Suzuki, M., et al. (2012). Il2rg gene-targeted severe combined immunodeficiency pigs. Cell Stem Cell 10, 753–758. doi: 10.1016/j.stem.2012.04.021
Tajima, S., Motoyama, S., Wakiya, Y., Uchikura, K., Misawa, H., Takishita, R., et al. (2020). Piglet production by non-surgical transfer of vitrified embryos, transported to commercial swine farms and warmed on site. Anim. Sci. J. 91, 1–8. doi: 10.1111/asj.13476
Tao, T., Reichelt, B., and Niemann, H. (1995). Ratio of inner cell mass and trophoblastic cells in demi- and intact pig embryos. Reproduction 104, 251–258. doi: 10.1530/jrf.0.1040251
Tian, H., Qi, Q., Yan, F., Wang, C., Hou, F., Ren, W., et al. (2021). Enhancing the developmental competence of prepubertal lamb oocytes by supplementing the in vitro maturation medium with sericin and the fibroblast growth factor 2 - leukemia inhibitory factor - insulin-like growth factor 1 combination. Theriogenology 159, 13–19. doi: 10.1016/j.theriogenology.2020.10.019
Tokuhiro, K., and Dean, J. (2018). Glycan-independent gamete recognition triggers egg zinc sparks and ZP2 cleavage to prevent polyspermy. Dev. Cell 46, 627. doi: 10.1016/j.devcel.2018.07.020
Turk, J. R., Henderson, K. K., Vanvickle, G. D., Watkins, J., and Laughlin, M. H. (2005). Arterial endothelial function in a porcine model of early stage atherosclerotic vascular disease. Int. J. Exp. Pathol. 86, 335. doi: 10.1111/j.0959-9673.2005.00446.x
Vaccari, S., Weeks, J. L. II., Hsieh, M., Menniti, F. S., and Conti, M. (2009). Cyclic GMP signaling is involved in the luteinizing hormone-dependent meiotic maturation of mouse oocytes. Biol. Reprod. 81, 595. doi: 10.1095/biolreprod.109.077768
Viana, J. (2020). 2019 Statistics of embryo production and transfer in domestic farm animals Divergent trends for IVD and IVP embryos. IETS Embryo Technol. Newsl. 38:1–15.
Wei, Y., Huan, Y., Shi, Y., Liu, Z., Bou, G., Luo, Y., et al. (2011). Unfaithful maintenance of methylation imprints due to loss of maternal nuclear Dnmt1 during somatic cell nuclear transfer. PLoS ONE 6, e20154. doi: 10.1371/journal.pone.0020154
Weng, X. G., Cai, M. M., Zhang, Y. T., Liu, Y., Liu, C., and Liu, Z. H. (2020). Improvement in the in vitro development of cloned pig embryos after kdm4a overexpression and an H3K9me3 methyltransferase inhibitor treatment. Theriogenology 146, 162–170. doi: 10.1016/j.theriogenology.2019.11.027
White, K. L., Hehnke, K., Rickords, L. F., Southern, L. L., Thompson, D. L., and Wood, T. C. (1989). Early embryonic development in vitro by coculture with oviductal epithelial cells in pigs. Biol. Reprod. 41, 425–430. doi: 10.1095/biolreprod41.3.425
Whitworth, K. M., Lee, K., Benne, J. a, Beaton, B. P., Spate, L. D., Murphy, S. L., et al. (2014). Use of the CRISPR/Cas9 system to produce genetically engineered pigs from in vitro-derived oocytes and embryos. Biol. Reprod. 91, 78. doi: 10.1095/biolreprod.114.121723
Whitworth, K. M., and Prather, R. S. (2010). Somatic cell nuclear transfer efficiency: how can it be improved through nuclear remodeling and reprogramming? Mol. Reprod. Dev. 77, 1001–1015. doi: 10.1002/mrd.21242
Whitworth, K. M., Rowland, R. R. R., Ewen, C. L., Trible, B. R., Kerrigan, M. A., Cino-Ozuna, A. G., et al. (2016). Gene-edited pigs are protected from porcine reproductive and respiratory syndrome virus. Nat. Biotechnol. 34, 20–22. doi: 10.1038/nbt.3434
Whitworth, K. M., Rowland, R. R. R., Petrovan, V., Sheahan, M., Cino-Ozuna, A. G., Fang, Y., et al. (2019). Resistance to coronavirus infection in amino peptidase N-deficient pigs. Transgenic Res. 28, 21–32. doi: 10.1007/s11248-018-0100-3
Whitworth, K. M., Zhao, J., Spate, L. D., Li, R., and Prather, R. S. (2011). Scriptaid corrects gene expression of a few aberrantly reprogrammed transcripts in nuclear transfer pig blastocyst stage embryos. Cell Reprogram. 13, 191–204. doi: 10.1089/cell.2010.0087
Whyte, J. J., Samuel, M., Mahan, E., Padilla, J., Simmons, G. H., Arce-Esquivel, A. A., et al. (2011). Vascular endothelium-specific overexpression of human catalase in cloned pigs. Transgenic Res. 20, 989–1001. doi: 10.1007/s11248-010-9473-7
Xia, P., Wang, Z., Yang, Z., Tan, J., and Qin, P. (2001). Ultrastructural study of polyspermy during early embryo development in pigs, observed by scanning electron microscope and transmission electron microscope. Cell Tissue Res. 303, 271–275. doi: 10.1007/s004410000315
Xu, W., Li, Z., Yu, B., He, X., Shi, J., Zhou, R., et al. (2013). Effects of DNMT1 and HDAC inhibitors on gene-specific methylation reprogramming during porcine somatic cell nuclear transfer. PLoS ONE 8, e64705. doi: 10.1371/journal.pone.0064705
Yoshioka, K., Suzuki, C., Tanaka, A., Anas, I. M.-K., and Iwamura, S. (2002). Birth of piglets derived from porcine zygotes cultured in a chemically defined medium. Biol. Reprod. 66, 112–119. doi: 10.1095/biolreprod66.1.112
Yu, A., Zhang, Z., Bi, Q., Sun, B., Su, W., Guan, Y., et al. (2008). Regulation of cAMP on the first mitotic cell cycle of mouse embryos. Mol. Reprod. Dev. 75, 489–495. doi: 10.1002/mrd.20782
Yuan, Y., Spate, L. D., Redel, B. K., Tian, Y., Zhou, J., and Prather, R. S. (2017). Quadrupling efficiency in production of genetically modified pigs through improved oocyte maturation. Proc. Natl. Acad. Sci. U. S. A. 114, E5796–E5804. doi: 10.1073/pnas.1703998114
Zhai, Y., Li, W., Zhang, Z., Cao, Y., Wang, Z., Zhang, S., et al. (2018a). Epigenetic states of donor cells significantly affect the development of somatic cell nuclear transfer (SCNT) embryos in pigs. Mol. Reprod. Dev. 85, 26–37. doi: 10.1002/mrd.22935
Zhai, Y., Zhang, Z., Yu, H., Su, L., Yao, G., Ma, X., et al. (2018b). Dynamic methylation changes of DNA and H3K4 by RG108 improve epigenetic reprogramming of somatic cell nuclear transfer embryos in pigs. Cell. Physiol. Biochem. 50, 1376–1397. doi: 10.1159/000494598
Zhang, Y., Li, J., Villemoes, K., Pedersen, A. M., Purup, S., and Vajta, G. (2007). An epigenetic modifier results in improved in vitro blastocyst production after somatic cell nuclear transfer. Cloning Stem Cells 9, 357–363. doi: 10.1089/clo.2006.0090
Keywords: pig, porcine embryo, in vitro, pregnancy rate, micromanipulation
Citation: Chen PR, Uh K, Redel BK, Reese ED, Prather RS and Lee K (2022) Production of Pigs From Porcine Embryos Generated in vitro. Front. Anim. Sci. 3:826324. doi: 10.3389/fanim.2022.826324
Received: 30 November 2021; Accepted: 21 February 2022;
Published: 16 March 2022.
Edited by:
Vitor R. G. Mercadante, Virginia Tech, United StatesReviewed by:
Joao Henrique Moreira Viana, Brazilian Agricultural Research Corporation (EMBRAPA), BrazilWeinan Zhou, University of Illinois at Urbana-Champaign, United States
Copyright © 2022 Chen, Uh, Redel, Reese, Prather and Lee. This is an open-access article distributed under the terms of the Creative Commons Attribution License (CC BY). The use, distribution or reproduction in other forums is permitted, provided the original author(s) and the copyright owner(s) are credited and that the original publication in this journal is cited, in accordance with accepted academic practice. No use, distribution or reproduction is permitted which does not comply with these terms.
*Correspondence: Kiho Lee, a2lob2xlZSYjeDAwMDQwO21pc3NvdXJpLmVkdQ==