- 1Department of Animal Science, North Dakota State University, Fargo, ND, United States
- 2Department of Animal Biosciences, University of Guelph, Guelph, ON, Canada
- 3Zoetis Inc., Parsippany-Troy Hills, NJ, United States
To determine the effects of leucine supplementation on body weight (BW), tissue mass, nutrient digestibility, the concentration of serum amino acids (AAs) and metabolites, and protein abundance of elongation initiation factor 4E (eIF4E) in skeletal muscle, 23 Holstein bull calves (43. 3 ± 1.16 kg; 11.3 ± 0.57 days of age) fed milk replacer at 2.5% of body weight (BW; dry matter basis) were used in a randomized complete block design. Leucine was supplemented at 0, 0.4, 0.6, or 0.8 g Leu/kg BW per day for 28 d. Data were analyzed using the MIXED procedure of SAS. Leucine supplementation did not affect calf BW (P = 0.73), and digestibility of nitrogen (P = 0.21), organic matter (P = 0.28), and dry matter (P = 0.28). Masses proportional to BW of the pancreas (P = 0.04), omasum (P < 0.01), and spleen (P = 0.01) were quadratically affected by treatment where tissue mass decreased at 0.4 g Leu/kg BW and increased at 0.6 and 0.8 g Leu/kg BW. Semitendinosus mass proportional to BW tended (P = 0.07) to be quadratically affected, as tissue mass increased at 0.4 g Leu/kg BW, and decreased at 0.6 and 0.8 g Leu/kg BW. Serum Leu concentration increased linearly (P = 0.002; day × time × treatment) across days and after feedings with increased supplemental Leu. Increasing supplemental Leu linearly decreased serum Ala (P < 0.01), Arg (P = 0.04), Ile (P = 0.02), Met (P < 0.01), and Pro (P = 0.05) concentrations, and quadratically affected serum Glu (P = 0.04) and Lys (P = 0.03) concentrations where serum Glu and Lys concentrations were decreased at 0.4 g Leu/kg BW and increased at 0.6 and 0.8 g Leu/kg BW. There was no effect of treatment on protein abundance of eIF4E in semitendinosus or longissimus dorsi. These data indicate that supplemental Leu did not influence ADG and nitrogen retention in calves fed milk replacer. However, changes in serum AA concentrations and tissue masses proportional to BW suggest that supplementation of Leu at lower levels could increase the use of AA for non-visceral tissue growth.
Introduction
During early life, milk or milk replacer is the primary component of the diet for calves. Because the rumen is not yet developed there is limited pregastric fermentation resulting in nutrients bypassing the rumen and digestion occurring in the abomasum and intestines (Abe et al., 1979). Soberon et al. (2012) reported that increased pre-weaning plane of nutrition in Holstein heifers during the pre-weaning period was associated with an increase in future milk production. These results suggest a developmental programming effect on long-term productivity. Skeletal muscle protein synthesis and growth is rapid in the pre-weaning period (Wray-Cahen et al., 1998; Zheng et al., 2019) and requires adequate amino acid (AA) supply. Also, pre-weaned lambs are sensitive to dietary imbalances of AA (Rogers and Egan, 1975), and oversupply of non-limiting AA results in increased catabolism leading to a delay in muscle growth. Therefore, AA supplementation strategies should be explored to improve pre-weaning growth, and thus potentially lead to improvements in lifetime productivity.
Leucine is an essential AA as sufficient quantities are not able to be synthesized within the body to meet metabolic needs. However, Leu is not typically considered limiting in diets, but it is thought to be a functional AA because Leu has numerous effects on metabolism (Alfalah et al., 2009; Dodd and Tee, 2012). Leucine is believed to be the primary AA that signals for increasing skeletal muscle protein synthesis (Pedroso et al., 2015). Leucine also influences plasma EAA concentrations in weaned piglets with lower concentrations observed when Leu was supplemented at 150–175% of requirements than when supplemented at 100 or 200% of requirements (Wiltafsky et al., 2010) and increases AA transport into cells (Broer, 2008) allowing for greater protein synthesis. In pre-weaning pigs, Leu has been shown to increase protein synthesis in skeletal muscle (Escobar et al., 2010) and increase BW and mass of the longissimus dorsi (LD) muscle (Columbus et al., 2015). More research on the effects of Leu in pre-weaned ruminants is needed.
We hypothesized that increasing supplemental Leu in milk replacer fed to Holstein bull calves would increase nitrogen retention and muscle mass, and influence factors regulating tissue growth and protein synthesis. Therefore, the objectives of this study were to determine the effects of increasing supplemental Leu in milk replacer on pre-weaned calf growth and tissue masses, nutrient balance, serum AA and metabolite concentrations, and protein abundance of elongation initiation factor 4E (eIF4E) in skeletal muscle.
Materials and Methods
Animals, Facilities, and Experimental Design
All the procedures involving the use of animals were approved by the North Dakota State University Institutional Animal Care and Use Committee. A total of 23 Holstein bull calves (43.3 ± 1.16 kg; 11.3 ± 0.57 days of age) were used. Calves were sourced from one commercial dairy. Calves were individually housed (0.91 × 1.2 m pens) in a temperature-controlled room (14°C) on Tenderfoot flooring with rubber stall mats and no bedding, and fed twice daily (07:30 and 16:30 h) for 28 d. Calves received commercial milk replacer (Nurture, Cargill Incorporation, Minneapolis, Minnesota, USA) formulated to meet or exceed CP and AA requirements at 2.5% (dry matter basis) of their initial BW split evenly between daily feedings. Milk replacer was reconstituted with 1.5 L warm tap water at each feeding. Water was offered for ad libitum intake. No other feed, such as starter or hay, was provided to calves because we did not want observed changes to be influenced by possible differences in starter intake between treatment groups.
Calves were blocked by arrival date (n = 4 per block except for 1 block with n = 3) into one of four treatment groups: milk replacer with no supplemental Leu or with 0.4, 0.6, or 0.8 g/kg initial BW of added Leu (99.7% purity; Ajinomoto, Raleigh, NC, USA; n = 6 for 0, 0.6, and 0.8 g Leu/kg BW treatments and n = 5 for 0.4 g Leu/kg BW treatments). The nutrient and AA composition of the treatment milk replacers is given in Table 1. Daily Leu allotments were split evenly between feedings (2 per day) and mixed with milk replacer before feeding. Amounts of supplemental Leu were chosen to include values above and below the amount (0.63 g/kg BW) provided parenterally by Boutry et al. (2013) to piglets that resulted in increased skeletal muscle protein synthesis.
Sample Collection
Calves were weighed for 2 d at the beginning of the experiment (day 0 and day 1), and on days 18, 22, 28, and immediately before tissue collection on day 29. Blood samples were collected via jugular venipuncture before and 2 h post-feeding on d 1, 18, 22, and 28 for analyses of serum metabolites and AA. Blood samples were allowed to sit at room temperature to clot for 30 min before being placed on ice and transferred to the laboratory. Serum was separated by centrifugation (3,000 × g at 4°C) for 20 min and stored at −20°C until analyses.
To determine the effects of increasing supplemental Leu on nutrient digestibility and nitrogen retention, calves were adapted to metabolism crates from days 19 to 21; one calf from the 0.6 g Leu/kg BW treatment was removed due to not adapting to the crate. Milk replacer (100 ml) was sampled from days 21 to 28 and the total collection of feces and urine occurred from days 21 to 28 as described previously (Swanson et al., 2000, 2004). Daily fecal output was recorded, composited across days within animals, and stored at −20°C until laboratory analyses. Urine was collected into 10-L jugs containing 100 ml of 6 N HCl to prevent nitrogen volatilization. A total of 10% of daily urine output was retained, composited across days within the animal, and stored at −20°C until laboratory analyses. Nitrogen retention was calculated as nitrogen intake minus nitrogen output in urine and feces.
On day 29, calves were removed from metabolism crates, weighed, and stunned via captive bolt before exsanguination. Contents of the digestive tract were removed and masses of reticulorumen, omasum, abomasum, small intestine, colon, cecum, liver, pancreas, spleen, visceral fat, kidneys, heart, lungs, semimembranosus, semitendinosus, and LD were recorded. Tissue masses are reported on an absolute (g) and proportional (g/kg BW) basis.
Sample Analyses
Feces and milk replacer were thawed at room temperature and subsequently dried at 60°C in a forced-air oven for 48 h, ground to pass a 1-mm screen, and analyzed (AOAC, 1990) for dry matter, organic matter, and nitrogen (Kjeldahl method). Urine was also analyzed for nitrogen (Kjeldahl method). Crude protein concentration was calculated as 6.25 × N. AA concentration of milk replacer was analyzed by high-performance liquid chromatography at the University of Missouri Agricultural Experiment Station Chemistry Laboratory (AOAC, 1990).
Serum glucose concentration was measured using the hexokinase/glucose-6-phosphate dehydrogenase method (Farrance, 1987) using the infinity glucose hexokinase kit (Thermo Trace, Louisville, Kentucky, USA). Urea nitrogen concentration in serum was measured (Jung et al., 1975) using the QuantiChrom urea assay kit (BioAssay Systems, Hayward, California, USA). Serum insulin concentrations were analyzed using a bovine insulin ELISA kit (ABclonal Technology, Woburn, Massachusetts, USA). Glucose, urea N, and insulin were analyzed using a Synergy H1 microplate reader (BioTek Instruments, Winooski, Vermont, USA). Serum-free AA concentrations were analyzed by reversed-phase ultraperformance liquid chromatography after pre-column derivatization of AA with aminoquinolyl-n-hydroxysuccinimidyl carbamate (Salazar et al., 2012; Lemley et al., 2013). Chromatography was achieved on an ethylene bridged hybrid C18 column (2.1 × 150 mm; 1.7 μm; Waters Corporation, Milford, Massachusetts, USA). Concentrations of AA were quantified in reference to norvaline and measured at 260 nm (Acquity UPLC; Waters Corporation, Milford, Massachusetts, USA).
For immunoblot analysis, ~500 mg of frozen semitendinosus or LD were homogenized in 4 ml RIPA buffer (Sigma-Aldrich Corporation, St Louis, Mosby, USA) which included a protease inhibitor cocktail (1%; Cell Signaling Technology, Danvers, Massachusetts, USA) and a phosphatase inhibitor solution (1%; Cell Signaling Technology) using a polytron (Brinkmann Instruments Incorporation, Westbury, New York, USA). The samples were centrifuged (13,000 × g, 4°C, 15 min) and the supernatant was stored at −20°C. The concentration of protein was evaluated using the bicinchoninic acid procedure with bovine serum albumin as the standard (Smith et al., 1985) and measured on a Synergy H1 microplate reader (BioTek Instruments). From each homogenate, 112.5 μg protein was separated on 5% stacking and 15% separating sodium dodecyl sulfate-polyacrylamide (SDS-PAGE) gels, and electro-transferred to polyvinylidene fluoride membranes (0.2 μm; Bio-Rad Life Science, Hercules, CA) using a Trans-Blot Turbo transfer system (Bio-Rad Life Science). The amount of protein selected for loading was within the linear range of band intensity (Figure 1). A molecular weight marker (10–250 kDa; Bio-Rad Life Science) was loaded on each gel as well as a pooled control to allow for comparison between blots. Blocking was done in 5% goat serum (VWR, Radnor, PA) in phosphate-buffered saline containing Tween-20 (PBST) for 1 h at room temperature. Membranes were incubated with a primary antibody against eIF4E (#9742, Cell Signaling Technology; source = rabbit, specific for eIF4E in several species including bovine) at a dilution of 1:5,000 in PBST with 5% heat-inactivated goat serum at 4°C overnight. A horseradish peroxidase (HRP)-linked goat anti-rabbit IgG secondary antibody (Cell Signaling Technology) at a dilution of 1:10,000 and a StrepTactin-HRP conjugate (Bio-Rad Life Science) at a dilution of 1:100,000 for 1 h in PBST containing 5% heat-inactivated goat serum. Membranes were washed 3 times for 10 min in PBST, incubated with the Amersham ECL Prime western blotting detection reagent (Cytiva Life Sciences, Marlborough, Massachusetts, USA) for 5 min at room temperature, and the intensities of bands were detected with a FluorChem FC2 system (Protein Simple, San Jose, CA, USA) after an exposure time of 1.5 times the recommended exposure time, and quantified by AlphaEaseFC (Protein Simple) software.
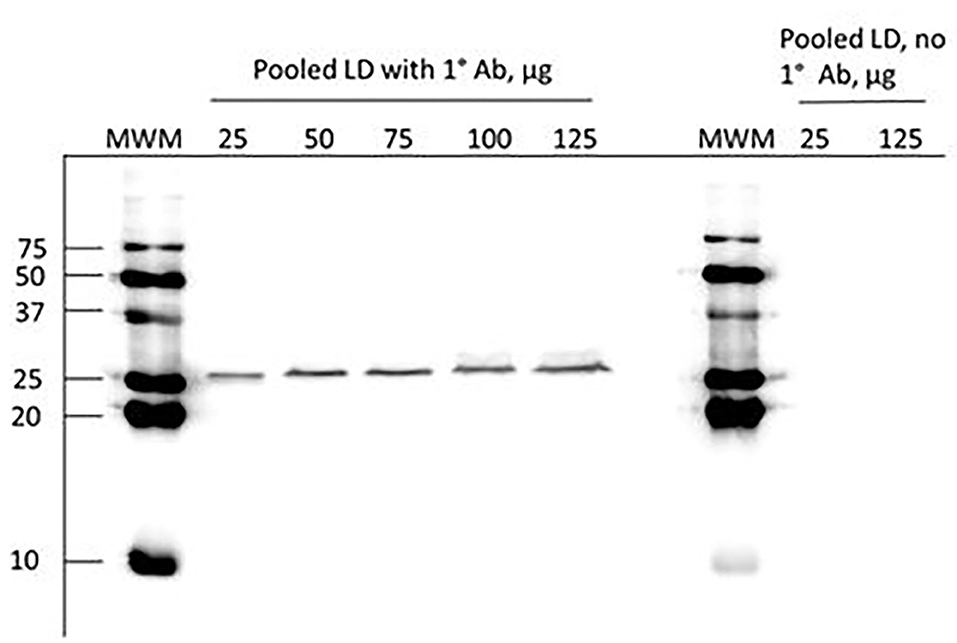
Figure 1. Representative immunoblot of eIF4E in a pooled longissimus dorsi (LD) sample. Increasing amounts of protein were loaded and subjected to immunoblotting as described in the materials and methods. The image indicates that the amount of protein selected for loading was within the linear range of band intensity and that the detected protein has an apparent molecular weight of ~26 kD when comparing the molecular weight marker (MWM). Immunoblotting was also conducted on lanes with low and high protein loading in the absence of primary antibody (1° Ab) and the lack of visible bands indicates the lack of non-specific binding.
Statistical Analysis
Data were analyzed as a randomized complete block design (block = arrival date group; n = 6 blocks). Nutrient intake, excretion, disappearance, digestibility, and nitrogen retention, and tissue masses were analyzed using the MIXED procedure of SAS (SAS 9.4, SAS Institute Incorporation, Cary, North Carolina, USA). The experimental unit was calf and the model included effects of block and treatment. Linear and quadratic effects of treatment were determined using orthogonal contrast statements. Because of unequal spacing of supplemental Leu treatments, the IML procedure of SAS was used to develop contrast coefficients. Serum AA and metabolites were analyzed using the MIXED procedure in SAS with repeated measures. The experimental unit was calf, the repeated effect was day, arrival date served as block, and the model included effects of the day, treatment, time, and all interactions. Linear and quadratic effects of treatment were determined by orthogonal contrasts. Appropriate (minimize information criterion) covariance structures were used (Wang and Goonewardene, 2004). Significance was declared at P ≤ 0.05 and a tendency at 0.05 < P ≤ 0.10.
Results
Initial and final BW and ADG were not influenced by supplemental Leu treatment (Table 2). Dry matter and organic matter intake, excretion, disappearance, and digestibility were not influenced by supplemental Leu treatment (Table 3). Intake, excretion, and digestibility of nitrogen were not influenced by supplemental Leu treatment, but there was a linear increase (P = 0.04) in nitrogen disappearance. Nitrogen retention also did not differ among treatments.
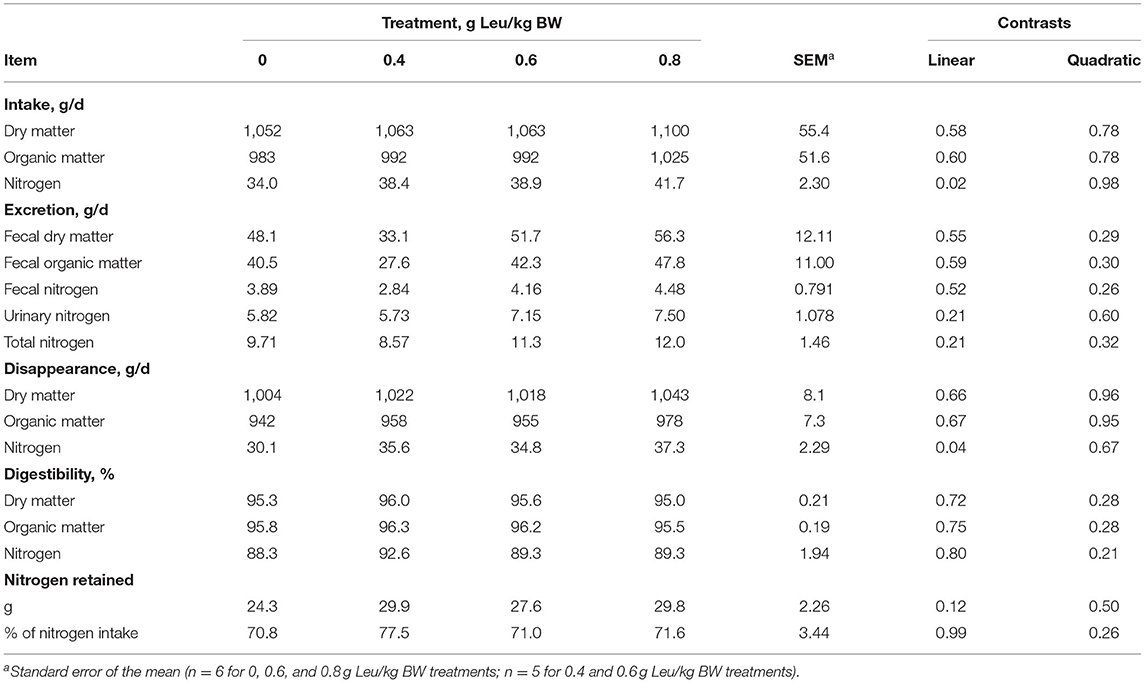
Table 3. Nutrient intake, excretion, disappearance, digestibility, and nitrogen retention of calves-fed milk replacer with increasing amounts of supplemental leucine.
Increasing supplemental Leu did not influence mass (g and g/kg BW; Table 4) of the small intestine, reticulorumen, or abomasum. Mass of omasum (g and g/kg BW) responded quadratically (P = 0.01) to supplemental Leu treatment where Leu supplemented at 0.4 g/kg BW decreased tissue mass compared with other treatments. Tissue mass (g and g/kg BW) of the colon, cecum, and liver were not influenced by supplemental Leu. Pancreas mass (g) increased linearly (P = 0.05) with increasing Leu supplementation and was affected quadratically (P = 0.04) when expressed proportional to BW (g/kg BW) as proportional mass decreased with Leu supplementation of 0.4 g Leu/kg BW and then increased with increasing supplemental Leu. Mass of the spleen (g and g/kg BW) responded quadratically (P = 0.02) to increasing supplemental Leu as tissue mass decreased at Leu supplementation of 0.4 g Leu/kg BW and then increased with increasing supplemental Leu. Mass (g and g/kg BW) of visceral fat, kidney, heart, lung, semitendinosus, and LD were not affected by treatment. Proportional mass (g/kg BW) of the semitendinosus tended (P = 0.07) to respond quadratically with increasing supplemental Leu as proportional tissue mass increased in calves on the 0.4 g Leu/kg BW treatment, and then decreased with greater amounts of supplemental Leu.
There were no interactions between treatment, day, and time for serum urea nitrogen or glucose concentration. Increasing supplemental Leu did not affect serum urea nitrogen concentration (Table 5). Serum glucose concentration tended (P = 0.10) to be affected quadratically by treatment where glucose concentration was lower in calves supplemented with 0.4 and 0.6 g Leu/kg BW than calves supplemented with the control and 0.8 g Leu/kg BW treatments. There were no interactions between treatment, day, and time for serum insulin concentration. Serum insulin concentration tended (P = 0.10) to decrease linearly with increasing Leu supplementation. Serum urea nitrogen concentration decreased (P = 0.05) over time, serum glucose concentration increased (P < 0.01) up to day 22 and then decreased on day 28, and tended (P = 0.10) to increase over time (Supplementary Table 1). Serum glucose concentration increased (P < 0.01) after feeding and serum urea N and insulin concentrations were not influenced by sampling time (data not shown).
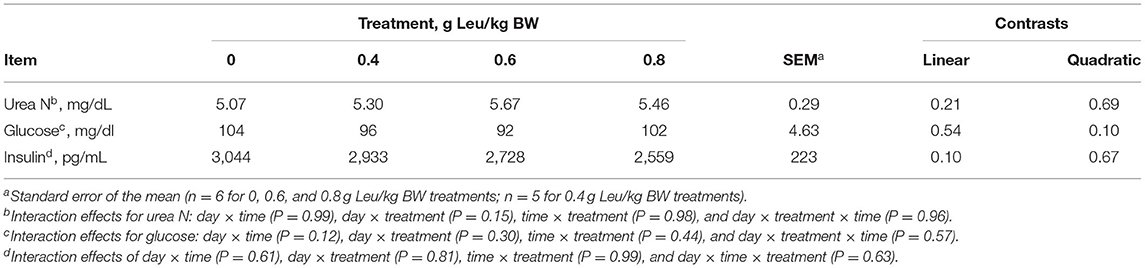
Table 5. Serum metabolites of calves-fed milk replacer with increasing amounts of supplemental leucine.
A treatment × day × time interaction was observed (P = 0.002) for serum Leu concentration (Figure 2). Serum Leu concentrations increased after feeding for all treatments and serum Leu increased linearly across treatments post-feeding, with day 18 having the greatest serum Leu concentrations. No interactions were observed for treatment × day × time, treatment × time, or day × time for serum AA concentrations. Day × treatment interactions (Supplementary Table 2) were observed for serum Glu (P = 0.03) and Ser (P = 0.04) concentrations, but these interactions were largely driven by differences in variability within specific days more so than changes in the order of response to supplemental Leu. Serum Arg concentration linearly decreased (P = 0.04) with increasing supplemental Leu (Table 6). Serum His concentration was unaffected by increasing supplemental Leu. Serum Ile concentration linearly decreased (P = 0.02) with increasing supplemental Leu, and serum Lys concentration was affected quadratically (P = 0.03) with Lys concentration decreasing in calves supplemented with 0.4 g Leu/kg BW and then increasing with greater amounts of supplemental Leu. Serum Met concentration linearly decreased (P < 0.01) with increasing supplemental Leu, and serum Phe concentration tended (P = 0.06) to be affected quadratically where Phe concentration increased at lower levels of supplemental Leu. There was a tendency (P = 0.07) for a quadratic effect for serum Thr concentration, where Thr concentration decreased at lower levels of supplemental Leu. Serum Trp concentration was unaffected by increasing supplemental Leu. There was a tendency (P = 0.09) for a quadratic effect for serum Val concentration where Val concentration increased at lower levels of supplemental Leu. Also, quadratic effects (P = 0.02) of increasing supplemental Leu were observed for serum essential AA (EAA) concentrations with EAA concentrations decreasing with lower levels of supplemental Leu and then increasing at greater levels of supplemental Leu. Serum Ala concentration linearly decreased (P < 0.01) with increasing supplemental Leu, and serum concentration of Asn was unaffected by increasing supplemental Leu. Serum Asp concentration tended (P = 0.07) to linearly decrease with increasing supplemental Leu. Serum Gly concentration was unaffected by increasing supplemental Leu, and serum Glu concentration was affected quadratically (P = 0.04) with Glu concentration decreasing in calves supplemented with 0.4 g Leu/kg BW and then increasing with greater amounts of supplemental Leu. Increasing supplemental Leu had no effect on serum Gly concentration. Serum Pro concentration linearly decreased (P = 0.05) with increasing supplemental Leu, and serum Ser concentration was unaffected by increasing supplemental Leu. There was a tendency (P = 0.08) for a linear effect for serum Tyr concentration, where Tyr concentration decreased as supplemental Leu increased. However, serum total nonessential AA (NEAA) concentration was not influenced by supplemental Leu treatment. Serum total AA concentration was affected quadratically (P = 0.05) where total AA concentration decreased at lower levels of supplemental Leu and increased at greater levels of supplemental Leu. There were also effects of day on serum AA concentrations, where serum concentration of Ala, Arg, Asp, Glu, Gly, His, Ile, Lys, Pro, Ser, Thr, and Val decreased (P < 0.03) and Asn, Gln, Met, Phe, Trp, and Tyr increased (P < 0.02) over the experimental period (Supplementary Table 3). Serum concentrations postfeeding were elevated (P < 0.04) for Ala, Arg, Asn, Asp, Gln, His, Ile, Lys, Met, Pro, Ser, Thr, Trp, Tyr, and Val concentrations, but serum Gly concentration decreased (P < 0.01) after feeding (data not shown). There was no effect of increasing supplemental Leu on protein abundance of eIF4E (Table 7) in semitendinosus or LD.
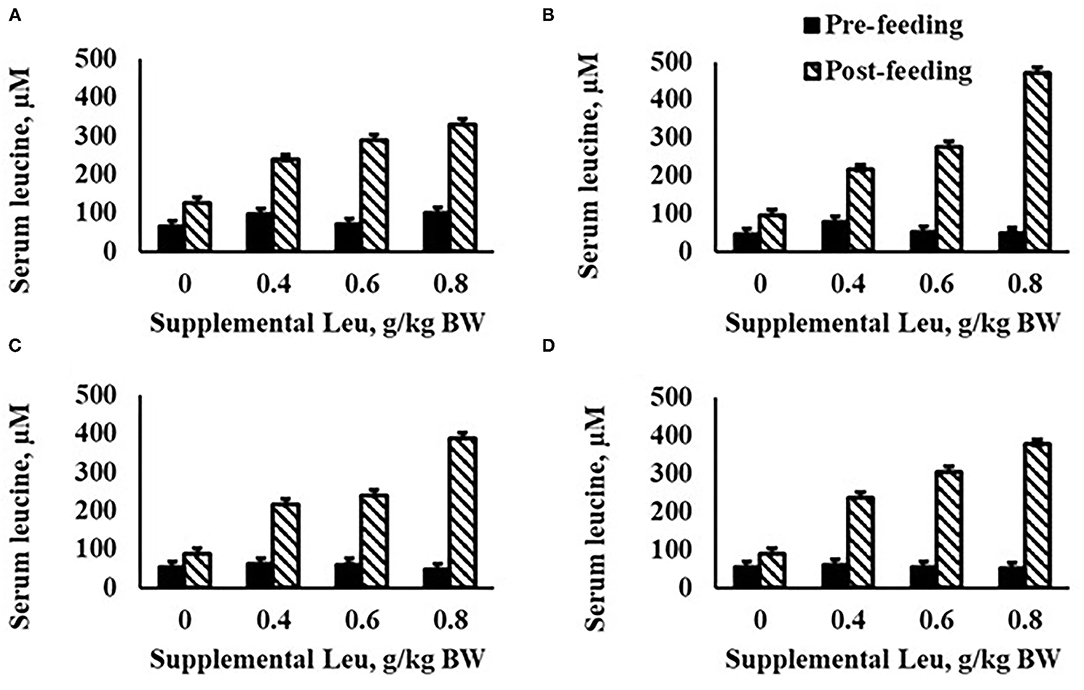
Figure 2. Serum leucine concentration in calves fed milk replacer with increasing amounts of supplemental leucine on day 1 (A), 18 (B), 22 (C), and 28 (D). Treatments were 0, 0.4, 0.6, or 0.8 g/kg BW supplemental leucine to milk replacer. Effects for serum leucine were day × time × treatment (P = 0.002), time × treatment (P < 0.001), day × treatment (P = 0.03), treatment (P < 0.001), day × time (P = 0.22), time (P < 0.001), and day (P = 0.20).
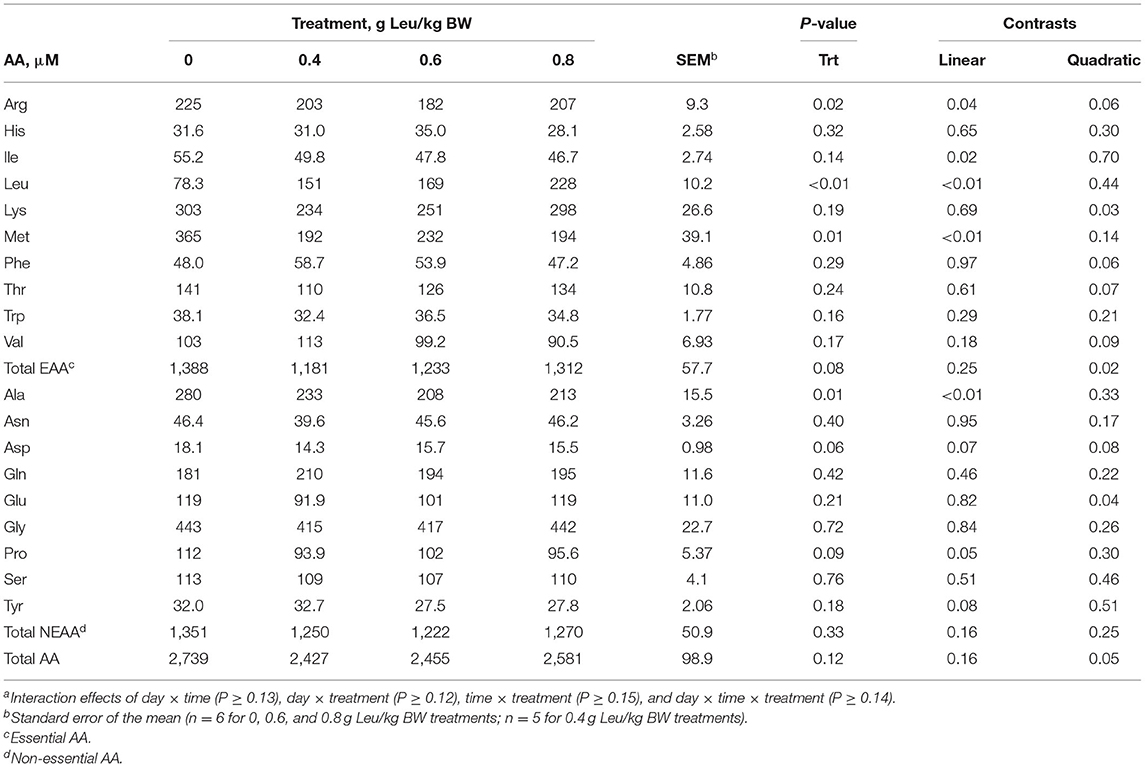
Table 6. Serum amino acid (AA) concentrations of calves-fed milk replacer with increasing amounts of supplemental leucinea.
Discussion
Better meeting the AA needs of pre-weaning ruminants should result in more efficient utilization of milk replacer and increased growth, and potentially could improve lifetime productivity. More research is needed on supplemental Leu in calves-fed milk replacer. In this study, supplemental Leu did not influence calf BW or ADG, although measuring BW changes over a period of 28 days is difficult because of the variation in BW within animals from day to day. Our results are similar to results from Cao et al. (2018) who reported that the final BW of 8-week-old Holstein calves was not influenced by Leu supplementation at 1.435 g Leu/L milk (~0.2 g Leu/kg BW). It should be pointed out that calves were not offered starter feed which may have resulted in delayed gastrointestinal development and lower ADG than if calves were offered starter feed.
Data are also limited on the effects of the supplemental Leu on digestibility and nitrogen retention in calves. Blaxter and Wood (1951) reported dry matter digestibility of skim milk with lard of 94%, similar to our result of 95%. More recent data are limited on nutrient digestibility in calves-fed diets of milk replacer without starter feed. As the digestibility of the milk replacer was high, it might not be surprising that no differences in digestibility were observed with supplemental Leu feeding. Similarly, nitrogen retention was not influenced by supplemental Leu which suggests that Leu did not promote an increase in overall nitrogen retention by calves. Gerrits (2019) reported that nitrogen retention of pre-weaned calves is around 70%, which is similar to our result of 73%. It is unknown why increasing supplemental Leu did not influence ADG, nutrient digestibility, or nitrogen balance. However, differences in ADG are difficult to detect with the limited number of experimental observations (n = 6) and study duration (28 days) used in this study, and the sensitivity of approaches used for measuring digestion and nitrogen balance used in the present experiment may not have been great enough to measure treatment differences. In support of the concept that alternative measures other than growth or nitrogen balance may be more appropriate for examining subtle dietary effects on nitrogen utilization, Pencharz and Ball (2003) reported that the use of nitrogen balance and growth measurements in humans is less sensitive than measuring plasma AA levels to assess AA and protein status. However, it should be noted that nitrogen retention numerically increased in the 0.4 g/kg BW treatment and then plateaued with increasing supplemental Leu. This may suggest that the effects of supplemental Leu could be observed by utilizing greater experimental units or more sensitive approaches for measuring whole animal nitrogen metabolism.
Proportional tissue mass (g/kg BW) will be discussed because of the effect that overall BW has on absolute tissue mass (g). Pancreatic proportional mass was decreased at 0.4 g/kg BW of supplemental Leu and increased with greater supplemental Leu. Differences in the pancreatic mass could potentially influence the production of pancreatic digestive enzymes (Swanson et al., 2002) or insulin (Baumgard et al., 2016). Changes in enzyme activity could alter the ability to degrade and absorb nutrients from the diet, and alterations in insulin production could influence signaling for protein synthesis (Baumgard et al., 2016) and energy utilization (Van Den Borne et al., 2006). Similar to the pancreas, spleen and omasum masses proportional to BW were quadratically affected by increasing supplemental Leu which could suggest a shift in proportional tissue growth from visceral tissues to skeletal muscle, at least at lower amounts of supplemental Leu.
Serum glucose concentration tended to be quadratically affected with an observed decrease in serum glucose in calves supplemented with 0.4 and 0.6 g Leu/kg BW. Leucine is a ketogenic AA (Brosnan and Brosnan, 2006; Voet and Voet, 2008) and does not have a direct role in glucose production. However, the tendency for an increase in semitendinosus proportional mass could be associated with increased demand for glucose, resulting in increased blood glucose utilization (Saxton and Sabatini, 2017). Others have reported no effects of Leu supplementation on blood glucose concentrations (Manjarín et al., 2016; Cao et al., 2018) in preweaned calves and piglets. The reasons for the discrepancies between studies may be because only a single sample collected on the day of tissue collection was analyzed in the calf study (Cao et al., 2018) and the piglet diet was restricted in CP which could have decreased the magnitude of response in tissue growth (Manjarín et al., 2016).
Besides being important in regulating blood glucose concentrations, insulin is also believed to upregulate mTORC1 activity through the Akt pathway. Furthermore, it has also been reported that Leu increases insulin secretion from the pancreas through the mTOR pathway (Yang et al., 2010, 2012). Serum insulin concentrations in this study decreased with increasing supplemental Leu. Other studies have reported no effect of supplemental Leu on serum insulin concentrations in humans or piglets (Nair et al., 1992; Boutry et al., 2013; Manjarín et al., 2016). Reasons for the discrepancies between studies are unknown. It is possible that Leu supplementation may not have been great enough to elicit an increase in serum insulin concentrations. Interestingly, serum urea N concentrations were not influenced by Leu supplementation. This could be because Leu was not degraded to the extent that results in increased urea N concentrations. Alternatively, and more likely as serum urea N concentrations were greater in calves receiving supplemental Leu, the lack of observed effect on serum urea N could be because the number of experimental units used in the experiment did not allow for differences to be detected.
As expected, serum Leu concentration increased as supplemental Leu increased, with greater increases following feeding (Figure 2). Our results are similar to those of Cao et al. (2018) who reported increasing plasma Leu in calves supplemented with Leu. Increased concentrations of serum Leu have been reported to be associated with decreased plasma concentrations of the other BCAA, Val, and Ile in piglets (Boutry et al., 2013). Similarly, as Leu supplementation increased in our study, both Val and Ile decreased. Loest et al. (2001) reported that in growing steers fed a soybean hull-based diet and abomasally infused with AA with or without specific BCAA, removal of Leu from the AA infusate increased plasma Ile and Val concentration and suggested that this may have occurred because of the stimulatory effect of Leu on BCAA oxidation. Alternatively, decreased concentrations of serum Ile and Val concentrations could be because Leu is transported by the same AA transport system as Ile and Val in the small intestine (Broer, 2008) resulting in decreased absorption of Ile and Val.
Serum concentrations of EAA decreased at 0.4 and 0.6 g Leu/kg BW, but increased at 0.8 g Leu/kg BW. This quadratic effect was likely driven by the decreases observed in Arg, Lys, and Met. These results disagree with the results of Cao et al. (2018) who reported no effects of Leu on plasma concentrations of Arg or Met. Boutry et al. (2013) reported lower concentrations of serum Met in piglets supplemented Leu compared with control piglets. Zheng et al. (2019) also reported no effects of supplemental Leu on serum concentrations of Lys in calves, but reported an increase in serum Met and a decrease in serum Arg with supplemental Leu. Reasons for the discrepancy between Zheng et al. (2019) and our results could be because of serum collection occurring during a fasted state before morning feedings, whereas in this study samples pre- and post-feeding were analyzed. In addition, L-Lys and DL-Met were included in the commercial milk replacer used in this experiment. Milk replacer and serum AA analyses in this experiment did not differentiate separate isoforms (D or L) of AA and supplemental Leu could potentially have different effects on the utilization of these isoforms. For example, it has been reported that cattle can utilize D-Met after conversion to L-Met but the conversion of D-Met to L-Met is slow (Lapierre et al., 2012), potentially decreasing the efficiency of utilization. Regardless, our results may suggest that lower levels of supplemental Leu altered serum EAA concentrations. The physiological effects of the observed changes in serum AA concentrations in this experiment are unknown, but the lack of observed effects in ADG and nitrogen balance suggest that there were no negative effects on growth.
Translation initiation is thought to be the rate-limiting step of protein synthesis (Sonenberg and Hinnebusch, 2009) and eIF4E plays a central role in regulating translation initiation (Romagnoli et al., 2021). However, in this study, no effect of supplemental Leu on eIF4E protein expression in semitendinosus or LD was observed. It is unknown if supplemental Leu downregulated protein degradation within the muscle, though it seems unlikely because of the lack of change in ADG and only a tendency for differences in semitendinosus proportional weight.
Conclusion
Our results showed that supplemental Leu did not influence the gross measurements of calf growth, nutrient digestibility, or nitrogen balance. However, supplemental Leu decreased the concentration of some EAA, with some EAA having the greatest decrease in calves supplemented with the lowest amount of supplemental Leu (0.4 g/kg BW). Furthermore, at lower levels of supplemental Leu, the proportional mass of some visceral tissues decreased, the proportional mass of semitendinosus tended to increase, and serum glucose concentration tended to decrease. Future research to examine effects on protein synthesis and turnover are needed to better define the potential for Leu supplementation to increase muscle protein synthesis in the pre-weaned calf, and if Leu supplementation pre-weaning improves post-weaning productivity. Future research also should examine other nutritional factors that could influence the response to supplemental Leu, such as examining different ratios of Leu to other AAs at similar crude protein intake, providing milk replacer differing in the concentration of other dietary nutrients, or energy, or providing starter feed.
Data Availability Statement
The raw data supporting the conclusions of this article will be made available by the authors, without undue reservation.
Ethics Statement
The animal study was reviewed and approved by North Dakota State University Institutional Animal Care and Use Committee.
Author Contributions
KS, KV, MS, and KM designed the experiment. KS and JR conducted the experiment and analyzed the data. JR drafted the manuscript with KS. All the authors reviewed the manuscript. All authors contributed to the article and approved the submitted version.
Funding
This study was supported by Animal Health and Production and Animal Products Project No. 2016-08395 from the USDA National Institute of Food and Agriculture.
Conflict of Interest
KV is employed by Zoetis.
The remaining authors declare that the research was conducted in the absence of any commercial or financial relationships that could be construed as a potential conflict of interest.
The handling editor declared a past co-authorship with one of the authors KS.
Publisher's Note
All claims expressed in this article are solely those of the authors and do not necessarily represent those of their affiliated organizations, or those of the publisher, the editors and the reviewers. Any product that may be evaluated in this article, or claim that may be made by its manufacturer, is not guaranteed or endorsed by the publisher.
Acknowledgments
The authors thank Cargill (Minneapolis, MN) for supplying milk replacer and Ajinomoto (Chicago, IL) for providing leucine used in this study. The authors thank the employees of the NDSU Animal Nutrition and Physiology Center (Fargo, ND), the Nutrition, Physiology, and Muscle Biology Laboratories in the Department of Animal Sciences at North Dakota State University (Fargo, ND), and students who assisted with this project.
Supplementary Material
The Supplementary Material for this article can be found online at: https://www.frontiersin.org/articles/10.3389/fanim.2022.817173/full#supplementary-material
References
Abe, M., Iriki, T., Kondoh, K., and Shibui, H. (1979). Effects of nipple or bucket feeding of milk-substitute on rumen by-pass and on rate of passage in calves. Br. J. Nutr. 41, 175–181. doi: 10.1079/BJN19790024
Alfalah, M., Keiser, M., Leeb, T., Zimmer, K. P., and Naim, H. Y. (2009). Compound heterozygous mutations affect protein folding and function in patients with congenital sucrase-isomaltase deficiency. Gastroenterology 136, 883–892. doi: 10.1053/j.gastro.2008.11.038
Baumgard, L. H., Hausman, G. J., and Sanz Fernandez, M. V. (2016). Insulin: pancreatic secretion and adipocyte regulation. Domest. Anim. Endocrinol. 54, 76–84. doi: 10.1016/j.domaniend.2015.07.001
Blaxter, K. L., and Wood, W. A. (1951). The nutrition of the young ayrshire calf. Br. J. Nutr. 5, 11–25. doi: 10.1079/BJN19510004
Boutry, C., El-Kadi, S. W., Suryawan, A., Wheatley, S. M., Orellana, R. A., Kimball, S. R., et al. (2013). Leucine pulses enhance skeletal muscle protein synthesis during continuous feeding in neonatal pigs. Am. J. Physiol. Endocrinol. Metab. 305, E620–E631. doi: 10.1152/ajpendo.00135.2013
Broer, S. (2008). Amino acid transport across mammalian intestinal and renal epithelia. Physiol. Rev. 88, 249–286. doi: 10.1152/physrev.00018.2006
Brosnan, J. T., and Brosnan, M. E. (2006). Branched-chain amino acids: enzyme and substrate regulation. J. Nutr. 136, 207S−211S. doi: 10.1093/jn/136.1.207S
Cao, Y. C., Yang, X. J., Guo, L., Zheng, C., Wang, D. D., Cai, C. J., et al. (2018). Effects of dietary leucine and phenylalanine on pancreas development, enzyme activity, and relative gene expression in milk-fed Holstein dairy calves. J. Dairy Sci. 101, 4235–4244. doi: 10.3168/jds.2017-13987
Columbus, D. A., Fiorotto, M. L., and Davis, T. A. (2015). Leucine is a major regulator of muscle protein synthesis in neonates. Amino Acids 47, 259–270. doi: 10.1007/s00726-014-1866-0
Dodd, K. M., and Tee, A. R. (2012). Leucine, and mTORC1: a complex relationship. Am. J. Physiol. Endocrinol. Metab. 302, E1329–E1342. doi: 10.1152/ajpendo.00525.2011
Escobar, J., Frank, J. W., Suryawan, A., Nguyen, H. V., Van Horn, C. G., Hutson, S. M., et al. (2010). Leucine and alpha-ketoisocaproic acid, but not norleucine, stimulate skeletal muscle protein synthesis in neonatal pigs. J. Nutr. 140, 1418–1424. doi: 10.3945/jn.110.123042
Gerrits, W. J. J. (2019). Symposium review: macronutrient metabolism in the growing calf. J. Dairy Sci. 102, 3684–3691. doi: 10.3168/jds.2018-15261
Jung, D., Biggs, H., Erikson, J., and Ledyard, P. U. (1975). New colorimetric reaction for end-point, continuous-flow, and kinetic measurement of urea. Clin. Chem. 21, 1136–1140. doi: 10.1093/clinchem/21.8.1136
Lapierre, H., Holtrop, G., Calder, A. G., Renaud, J., and Lobley, G. E. (2012). Is d-methionine bioavailable to the dairy cow? J. Dairy Sci. 95, 353–362. doi: 10.3168/jds.2011-4553
Lemley, C. O., Camacho, L. E., Meyer, A. M., Kapphahn, M., Caton, J. S., and Vonnahme, K. A. (2013). Dietary melatonin supplementation alters uteroplacental amino acid flux during intrauterine growth restriction in ewes. Animal 7, 1500–1507. doi: 10.1017/S1751731113001006
Loest, C. A., Titgemeyer, E. C., Lambert, B. D., and Trater, A. M. (2001). Branched-chain amino acids for growing cattle limit-fed soybean hull- based diets. J. Anim Sci. 79, 2747–2753. doi: 10.2527/2001.79102747x
Manjarín, R., Columbus, D. A., Suryawan, A., Nguyen, H. V., Hernandez-García, A. D., Hoang, N.-M., et al. (2016). Leucine supplementation of a chronically restricted protein and energy diet enhances mTOR pathway activation but not muscle protein synthesis in neonatal pigs. Amino Acids 48, 257–267. doi: 10.1007/s00726-015-2078-y
Nair, K. S., Matthews, D. E., Welle, S. L., and Braiman, T. (1992). Effect of leucine on amino acid and glucose metabolism in humans. Metabolism 41, 643–648. doi: 10.1016/0026-0495(92)90057-H
Pedroso, J. A., Zampieri, T. T., and Donato, J. Jr. (2015). Reviewing the effects of L-leucine supplementation in the regulation of food intake, energy balance, and glucose homeostasis. Nutrients 7, 3914–3937. doi: 10.3390/nu7053914
Pencharz, P. B., and Ball, R. O. (2003). Different approaches to define individual amino acid requirements. Annu. Rev. Nutr. 23, 101–116. doi: 10.1146/annurev.nutr.23.011702.073247
Rogers, Q. R., and Egan, A. R. (1975). Amino acid imbalance in the liquid-fed lamb. Aust. J. Biol. Sci. 28, 169–181. doi: 10.1071/BI9750169
Romagnoli, A., D'Agostino, M., Ardiccioni, C., Maracci, C., Motta, S., La Teana, A., et al. (2021). Control of the eIF4E activity: structural insights and pharmacological implications. Cell. Mol. Life Sci. 78, 6869–6885. doi: 10.1007/s00018-021-03938-z
Salazar, C., Armenta, J. M., and Shulaev, V. (2012). An UPLC-ESI-MS/MS assay using 6-aminoquinolyl-N-hydroxysuccinimidyl carbamate derivatization for targeted amino acid analysis: application to screening of arabidopsis thaliana mutants. Metabolites 2, 398–428. doi: 10.3390/metabo2030398
Saxton, R. A., and Sabatini, D. M. (2017). mTOR signaling in growth, metabolism, and disease. Cell 168, 960–976. doi: 10.1016/j.cell.2017.02.004
Smith, P. K., Krohn, R. I., Hermanson, G. T., Mallia, A. K., Gartner, F. H., Provenzano, M. D., et al. (1985). Measurement of protein using bicinchoninic acid. Anal. Biochem. 150, 76–85. doi: 10.1016/0003-2697(85)90442-7
Soberon, F., Raffrenato, E., Everett, R. W., and Van Amburgh, M. E. (2012). Preweaning milk replacer intake and effects on long-term productivity of dairy calves. J. Dairy Sci. 95, 783–793. doi: 10.3168/jds.2011-4391
Sonenberg, N., and Hinnebusch, A. G. (2009). Regulation of translation initiation in eukaryotes: mechanisms and biological targets. Cell 136, 731–745. doi: 10.1016/j.cell.2009.01.042
Swanson, K. C., Freetly, H. C., and Ferrell, C. L. (2004). Nitrogen balance in lambs fed a high-concentrate diet and infused with differing proportions of casein in the rumen and abomasum. J. Anim. Sci. 82, 495–501. doi: 10.2527/2004.822495x
Swanson, K. C., Matthews, J. C., Matthews, A. D., Howell, J. A., Richards, C. J., and Harmon, D. L. (2000). Dietary carbohydrate source and energy intake influence the expression of pancreatic alpha-amylase in lambs. J.Nutr. 130, 2157–2165. doi: 10.1093/jn/130.9.2157
Swanson, K. C., Matthews, J. C., Woods, C. A., and Harmon, D. L. (2002). Postruminal administration of partially hydrolyzed starch and casein influences pancreatic alpha-amylase expression in calves. J. Nutr. 132, 376–381. doi: 10.1093/jn/132.3.376
Van Den Borne, J. J. G. C., Verstegen, M. W. A., Alferink, S. J. J., Giebels, R. M. M., and Gerrits, W. J. J. (2006). Effects of feeding frequency and feeding level on nutrient utilization in heavy preruminant calves. J. Dairy Sci. 89, 3578–3586. doi: 10.3168/jds.S0022-0302(06)72397-9
Voet, D., and Voet, J. G. (2008). Amino acid metabolism, in Fundamentals of Biochemistry, 3rd edn (Hoboken, NJ: John Wiley & Sons), 732–790.
Wang, Z., and Goonewardene, L. A. (2004). The use of MIXED models in the analysis of animal experiments with repeated measures data. Can. J. Anim. Sci. 84, 1–11. doi: 10.4141/A03-123
Wiltafsky, M. K., Pfaffl, M. W., and Roth, F. X. (2010). The effects of branched-chain amino acid interactions on growth performance, blood metabolites, enzyme kinetics and transcriptomics in weaned pigs. Br. J. Nutr. 103, 964–976. doi: 10.1017/S0007114509992212
Wray-Cahen, D., Nguyen, H. V., Burrin, D. G., Beckett, P. R., Fiorotto, M. L., Reeds, P. J., et al. (1998). Response of skeletal muscle protein synthesis to insulin in suckling pigs decreases with development. Am. J. Physiol. 275, E602–E609. doi: 10.1152/ajpendo.1998.275.4.E602
Yang, J., Chi, Y., Burkhardt, B. R., Guan, Y., and Wolf, B. A. (2010). Leucine metabolism in regulation of insulin secretion from pancreatic beta cells. Nutr. Rev. 68, 270–279. doi: 10.1111/j.1753-4887.2010.00282.x
Yang, J., Dolinger, M., Ritaccio, G., Mazurkiewicz, J., Conti, D., Zhu, X., et al. (2012). Leucine stimulates insulin secretion via down-regulation of surface expression of adrenergic α2A receptor through the mTOR (mammalian target of rapamycin) pathway. J. Biol. Chem. 287, 24795–24806. doi: 10.1074/jbc.M112.344259
Keywords: amino acids, digestion, leucine, muscle, nitrogen balance, pre-ruminant, visceral tissues
Citation: Reiners JN, Steele MA, Vonnahme KA, Maddock Carlin KR and Swanson KC (2022) Effects of Supplemental Leucine on Growth, Nutrient Use, and Muscle and Visceral Tissue Mass in Holstein Bull Calves Fed Milk Replacer. Front. Anim. Sci. 3:817173. doi: 10.3389/fanim.2022.817173
Received: 17 November 2021; Accepted: 28 January 2022;
Published: 02 March 2022.
Edited by:
David L. Harmon, University of Kentucky, United StatesReviewed by:
Jon Schoonmaker, Purdue University, United StatesBrittany Harlow, Forage-Animal Production Research Unit, Agricultural Research Service (USDA), United States
Copyright © 2022 Reiners, Steele, Vonnahme, Maddock Carlin and Swanson. This is an open-access article distributed under the terms of the Creative Commons Attribution License (CC BY). The use, distribution or reproduction in other forums is permitted, provided the original author(s) and the copyright owner(s) are credited and that the original publication in this journal is cited, in accordance with accepted academic practice. No use, distribution or reproduction is permitted which does not comply with these terms.
*Correspondence: Kendall C. Swanson, a2VuZGFsbC5zd2Fuc29uQG5kc3UuZWR1