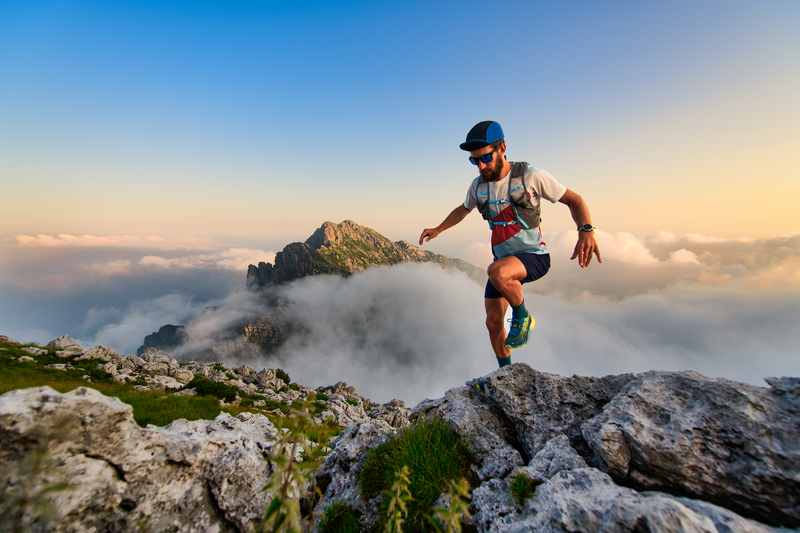
95% of researchers rate our articles as excellent or good
Learn more about the work of our research integrity team to safeguard the quality of each article we publish.
Find out more
ORIGINAL RESEARCH article
Front. Anim. Sci. , 12 January 2023
Sec. Animal Nutrition
Volume 3 - 2022 | https://doi.org/10.3389/fanim.2022.1094314
This article is part of the Research Topic Bio-accessibility of Functional Compounds and Nutrients of Animal Diets View all 5 articles
Dietary modulation in broilers is crucial for the establishment of beneficial microbiota and, subsequently, the promotion of intestinal health. In this trial, a 2 × 2 factorial design was used with two different specifications with respect to dietary metabolizable energy (ME) and crude protein (CP) levels (i.e., 95% and 100% of recommendations) and phytogenic levels (0 and 150 mg/kg). Levels of total bacteria, Bacteroides spp., Lactobacillus spp., and Clostridium cluster XIVa attached to the cecal mucosa and in the cecal digesta were lower in broilers fed the 95% ME and CP specification diets, as was the molar ratio of butyric acid. In addition, the relative activity of autoinducers-2 (AI-2) and the expression levels of TLR4 and AvBD6 were increased. Phytogenic supplementation reduced cecal digesta levels of Escherichia coli and Clostridium cluster I levels, and increased Clostridium cluster IV levels. Moreover, the butyric acid molar ratio and the relative activity of AI-2 were increased, whereas the concentration of branched VFAs and the expression of AvBD6 and LEAP2 were reduced by phytogenic administration. Dietary specifications and phytogenic interactions were shown for the cecal-attached microbiota composition, metabolic activity of digesta microbiota, relative expression of autoinducers-2, and relative expression of toll-like signaling molecules and host antimicrobial peptides. In conclusion, it has been shown that ME and CP dietary specifications, combined or not with phytogenics, modulate multilevel gut biomarkers ranging from microbiota composition and metabolic activity to microbial communications and host signaling, inflammation, and defense.
Good functioning of the gastrointestinal (GI) system is a basic requirement for the optimal productivity and health of broiler chickens. The GI system’s proper function and health depends on the maintenance of a dynamic balance between the microbial populations that colonize the gastrointestinal tract, microbiota, and the cells of the host, such as immune and epithelial cells (Brisbin et al., 2008; Celi et al., 2017). The symbiotic relationship between chickens and their intestinal microbiota results in beneficial effects such as promotion of intestinal mucosa growth (Slawinska et al., 2019), exclusion of pathogenic microorganisms (Baba et al., 1991; Tierney et al., 2004), polysaccharides breakdown (Rehman et al., 2007), energy supply in the form of amino acids or volatile fatty acids (Van Immerseel et al., 2006; Elling-Staats et al., 2021), and homeostasis of the immune system (Brisbin et al., 2010; Brisbin et al., 2015; Hu et al., 2021).
A necessary condition for achieving a balanced symbiosis is the appropriate communication between the host cells and the microbiota and between the prokaryotic cells of the microbiota (Li et al., 2019). The latter communication in particular is considered a mechanism of bacterial homeostasis and cooperation, called quorum sensing (QS), mediated by small diffusible signal molecules termed autoinducers (AIs). For intraspecies communication, Gram-negative bacteria signal using acyl homoserine lactones (AI-1) and Gram-positive bacteria signal using small peptides. For interspecies communication, non-species-specific AIs are employed, such as AI-2. The AI-2 is a family of molecules, and a major signal-type molecule of QS, which is a derivative of 4,5-dihydroxy-2,3-pentanedione that is synthesized by AI-2 synthetase (LuxS) enzymes. AI-2 levels modulate the abundance of the major phyla of intestinal microbiota and could be a new biomarker for monitoring intestinal flora disorders (Li et al., 2019; Fu et al., 2020; Wu and Luo, 2021).
Diet is a determining factor for intestinal microbiota. It is known that energy and protein levels, as well as the overall composition of the diet, can influence the composition of the intestinal microbiota in broilers (Laudadio et al., 2012; Paraskeuas and Mountzouris, 2019b). Nutritional manipulation in broilers for the establishment of beneficial microbiota and/or the existing microbiota modification is a key tool for promoting gut health. More specifically, several studies have reported that the dietary addition of bioactive ingredients (e.g., probiotics, acidifiers, enzymes, phytogenics) can change the profile of bacterial populations in the intestinal tract (Mountzouris et al., 2010; Mountzouris et al., 2015; Palamidi and Mountzouris, 2018; Paraskeuas and Mountzouris, 2019b). In recent years, phytogenics are gaining importance in the scientific community. Phytogenics include a wide range of plant products, such as essential oils, herbs, and oleoresins (Mohammadi Gheisar and Kim, 2018), and act multifacetedly on the intestinal ecosystem, more specifically antimicrobially, with anti-inflammatory effects, and affecting the sensory mechanisms of the gut (Yang et al., 2015). However, their effects depend on both the composition and the level of addition to the final diet (Mountzouris et al., 2011; Mountzouris et al., 2020). It has been reported that plant extracts (α-pinene or carvacrol) in vitro exhibit anti-quorum-sensing effects against pathogens (Šimunović et al., 2020; Wagle et al., 2020). However, reports investigating the in vivo effects of plant extracts on QS on endogenous microbiota in broilers are scarce.
Nowadays, due to the increased cost of feeding broilers and environmental concerns related to high nitrogen excretion, there is a tendency to reduce the energy density and protein level in the diet. Incorporation of a mixture of carvacrol, cinnamaldehyde, and capsicum oleoresin (Bravo et al., 2014) or a phytogenic based on carvacrol, anethol, and limonene (Paraskeuas et al., 2016) appear to compensate for the effects of the reduction in energy and protein levels and restore broiler performance.
The aim of this study was to generate new knowledge on the effects of dietary energy and protein levels with or without phytogenic addition on the cecal microbiota composition and its metabolic activity, signaling of cecal microbiota, and the expression of critical genes relevant for inflammation and defense in the cecal mucosa of broilers.
The experimental protocol was in compliance with the current European Union Directive on the protection of animals used for scientific purposes (Directive, 2007; Directive, 2010) and was approved by the relevant national authority Department of Agriculture and Veterinary Policy, General Directorate of Agriculture, Economy, Veterinary and Fisheries (Approval 1130/290216).
This study forms part of our previous research work (Griela et al., 2021). To avoid excessive repetition, a brief description of the experimental treatments is given below. A total of 540 1-day-old male Cobb 500 broilers vaccinated for Marek disease, infectious bronchitis, and Newcastle disease were acquired from a local hatchery. Birds were arranged according to a 2×2 factorial design in four treatments, with nine (n=9) replicate pens of 15 chicks per treatment for a 42-d study. Depending on diet specifications (i.e., 95% and 100%) and phytogenic supplementation (i.e., 0 and 150 mg/kg), the four experimental treatments were D95 (95% of optimal ME and CP specifications with no phytogenic supplementation), D95+ (95% of optimal ME and CP specifications with phytogenic supplementation), D100 (100% of optimal ME and CP specifications with no phytogenic supplementation), and D100+ (100% of optimal ME and CP specifications with phytogenic supplementation).
A three-phase feeding program with starter (1–10 d), grower (11–22 d), and finisher (23–42 d) diets was followed (Table S1). In particular, for each growth phase, two diets were formulated to meet 95% and 100% of optimal Cobb 500 metabolizable energy (ME) and protein (CP) specifications. The phytogenic used was a commercial product; a blend of bioactive compounds such as carvacrol, thymol, carvone, methyl salicylate, and menthol (Digestarom® Biomin Phytogenics GmbH, Germany), as previously described (Griela et al., 2021).
Each replicate was assigned to a clean floor cage (1 m2), and the birds were raised on rice hull litter. The temperature program was set at 32°C at week 1 and gradually reduced to 23°C by week 6. Heat was provided with a heating lamp per cage. Except for day 1, an 18-h light to 6-h dark lighting program was applied during the experiment to ensure adequate access to feed and water.
The ingredient (g/kg) and calculated chemical composition (g/kg as fed) of the basal diets (i.e., 95% vs 100%) was as follows (Table S1). Starter diet: AMEn (11.97 vs. 12.60 MJ); crude protein (204.3 vs. 215.0 g); lysine (12.5 vs. 13.2 g); methionine + cysteine (9.4 vs. 9.9 g); threonine (8.2 vs. 8.6 g); calcium (9 g); and available phosphorus (4.5 g). Grower diet: AMEn (12.27 vs. 12.92 MJ); crude protein (185.3 vs. 195 g); lysine (11.3 vs. 11.9 g); methionine + cysteine (8.6 vs. 9.0 g); threonine (7.5 vs. 7.9 g); calcium (8.4 g); and available phosphorus (4.2 g). Finisher diet: AMEn (12.59 vs. 13.26 MJ); crude protein (175.8 vs. 185.0 g); lysine (10.0 vs. 10.5 g); methionine + cysteine (7.8 vs. 8.2 g); threonine (6.8 vs. 7.1 g); calcium (7.6 g); and available phosphorus (3.8 g).
For the determination of the luminal- and mucosa-associated microbiota composition and the digesta volatile fatty acids (VFAs) concentration, one bird per replicate pen (i.e., nine birds per treatment) were euthanized via electrical stunning prior to slaughter. Subsequently the abdomen was opened and the ceca removed and immediately snap frozen in liquid nitrogen and stored at –80°C. For the purpose of this study, one cecum was thawed on ice and opened longitudinally. Firstly, cecal digesta were removed carefully and stored at –30°C until further analysis; then, to remove remaining digesta and bacteria not attached to the gut mucosa, ceca tissue was washed three times in ice-cold saline by gentle agitation. Subsequently, cecal-mucosa-attached bacteria were removed from the mucosa following a protocol of 3 × 1 min vigorous hand-shaking washes (15 ml) in saline containing 0.1% (wt/wt) Tween 80, according to Li et al. (2003). Finally, the washes were pooled and centrifuged at 10,000 × g for 30 min at 4°C to precipitate cells (cell pellet).
Cecal digesta and cell pellets from caecum were used for DNA extraction using a manual protocol adopted by the International Human Microbiome Standards project (Dore et al., 2015; http://www.microbiome-standards.org). For each sample, the extracted DNA was eluted in 200 ml of TE buffer, and the quality and quantity of the DNA preparations were determined by spectrophotometry (Q3000, Quawell Technology, Inc.) and stored at –30°C.
For the quantification of total bacteria (domain bacteria), Lactobacillus spp., Escherichia coli, Bacteroides spp., Clostridium cluster I (Clostridium perfringens group), Clostridium cluster XIVa (Clostridium coccoides group), and Clostridium cluster IV (Clostridium leptum group), suitable primers were used targeting the 16S rRNA gene (Table 1). Primer specificity was confirmed using BLAST (NCBI) and the ProbeMatch programs.
Table 1 Primers targeting 16S rRNA gene used for determination of luminal- and mucosa-associated microbiota composition by real-time PCR.
Real-time PCR was performed in microplates with the SaCycler‐96 (Sacace Biotechnologies Srl). Reactions were made to a final volume of 10μl and consisted of a 5μl of 2 × FastGene IC Green universal mix (Nippon Genetics, Tokyo, Japan), forward and reverse primers each at a final concentration of 300– 450 nmol/l, and 1 μl of DNA template (i.e., 20 ng of sample DNA/reaction). The reactions were incubated at 95°C for 5 s, at the primer-specific annealing temperature for 20 s, and at 72°C for 33 s. This was followed by a melt curve analysis to determine the reaction specificity. Each sample was measured in duplicates. Depending on whether the sample was from mucosa or luminal digesta, results were expressed as log-cells/g mucosa-associated cell pellet or as log-cells/g wet digesta contents, respectively.
Reference bacterial strains that were used to control the specificity of the primers and to construct standard curves are shown in Table 2. Each of the reference strains was cultured on selective broth under suitable conditions. Bacterial genomic DNA from each culture was extracted using a manual protocol adopted by the International Human Microbiome Standards project (Dore et al., 2015; http://www.microbiome-standards.org). For each sample, the extracted DNA was eluted in 100 μl of TE buffer, and the quality and quantity of the preparations were determined by spectrophotometry (Q3000, Quawell Technology, Inc) and stored at -30°C.
For the quantification of bacterial species and groups, a quantification method similar to the one described by Joly et al. (2006) was used. In more detail, an appropriate standard curve using 10-fold serial dilutions of a known concentration of genomic DNA was included in each 96-well plate. The concentration of genome copies, from each bacterial species in the initial purified DNA solution used to construct the standard curves, was calculated by assuming an average molecular mass of 660 Da for 1 bp of double-stranded DNA and using the following equation: concentration of genome copies ¼ Quantity of DNA (fg)/Mean mass of the corresponding genome (fg). The amount of genomic copies correspond to an equal amount of bacterial cells. Genome sizes for all bacteria species and groups used in this study are presented in Table 2.
For the determination of cecal VFA concentration, digesta were homogenized following a 10-fold dilution (i.e., 10% wt/vol) in sterile ice-cold phosphate-buffered saline (0.1 mol/l, pH 7.0). Homogenates were subsequently centrifuged at 12,000 × g for 10 min at 4°C and the resulting supernatants were stored at –80°C until their analysis by capillary gas chromatography (GC). Samples of 2 μl were injected into a gas chromatograph (Agilent 6890GC System, Agilent Technologies, Santa Clara, CA, USA) equipped with a Stabilwax®-DA Capillary GC Column (size x I.D. 30m × 0.25 mm, df 0.25 μm) (Restek Corporation, Bellefonte, PA, USA) and a flame ionization detector. The injector and detector temperature were set at 200°C and 220°C, respectively, and the temperature program was run from 140°C to 200°C with a temperature ramp rate of 5°C/min. Helium was the carrier gas with a column flow of 20 ml/min. The VFAs determined were acetic, propionic, isobutyric, butyric, isovaleric, and isohexanoic. Results were expressed as mmol/kg wet digesta for total VFA and as molar ratios (% of total VFA) for acetic, propionic, butyric, and branched VFAs (b-VFA; sum of isobutyric, isovaleric, and isocaproic).
For the determination of gene expression studies, the second cecum was used. In brief, the whole cecum was exposed and the cecal digesta were removed. The cecum was washed completely in 30 ml cold phosphate-buffered saline (PBS)–ethylenediaminetetraacetic acid (EDTA; 10 mmol/l) solution (pH = 7.2). Total RNA was extracted from the cecal tissue or the cecal tonsil using the NucleoZOL reagent, as reported by the manufacturer’s protocol (Macherey-Nagel GmbH & Co. KG, Duren, Germany). The purity and quality of RNA were evaluated using spectrophotometry (Q3000, Quawell Ltd) based on 260/230 nm wavelength ratios. Genomic DNA was removed using DNase I (M0303, New England Biolabs Inc, Ipswich, UK). A concentration of 500 ng of total RNA of each sample was reverse transcribed using the PrimeScript RT reagent kit (Perfect Real Time, Takara Bio Inc., Shiga, Japan), according to the manufacturer’s protocol. cDNAs were stored at –20°C.
For the relative expression of mucin 2 (MUC2), zonula occludens-1 (ZO1), zonula occludens-2 (ZO2), claudin-1 (CLDN1), occludin (OCLN), toll-like receptor 2 type-2 (TLR2B), toll-like receptor 4 (TLR4), interferon gamma (IFNG), nuclear factor kappa B subunit 1 (NFKB1), inducible nitric oxide synthase 2 (NOS2), free fatty acid receptor 2 (FFAR2), free fatty acid receptor 4 (FFAR4) was used produced cDNA from cecal tissue. For the relative expression of avian beta-defensin 1 (AvBD1) and cathelicidin 2 (CATH2), cDNA from the cecal tonsils was used as the relative expression from the cecal tissue cDNA was very low.
Primers not originating from the scientific literature were designed with the PerlPrimer program v.1.1.19 (Marshall, 2004) using the GenBank sequences, and their sequences are shown in Table 3. Primer specificity and efficiency were determined using pooled samples.
The relative expression of the above genes was detected using quantitative real‐time PCR (qPCR) using the SaCycler‐96 (Sacace Biotechnologies Srl) with FastGene IC Green 2x qPCR universal mix (Nippon Genetics, Tokyo, Japan). Each reaction contained 5 ng of RNA equivalents, as well as 200–450 nmol/l of forward and reverse primers for each gene. The reactions were incubated at 95°C for 5 s, 59–65°C (depending on the target gene) for 20 s, and 72°C for 33 s. This was followed by a melt curve analysis to determine the reaction specificity. Each sample was measured in duplicates. Relative expression ratios of target genes were calculated according to Pfaffl (2001), adapted for the multi-reference genes normalization procedure according to Hellemans et al. (2007), using glyceraldehyde-3-phosphate dehydrogenase (GAPDH) and hypoxanthine phosphoribosyltransferase (HPRT) as reference genes (Table 3).
The relative expression of AI-2 was investigated by using the Vibrio harveyi bioluminescence assay (Taga and Xavier, 2011; Hsiao et al., 2014). In brief, previously frozen cecal digesta were suspended in 2034 Autoinducer bioassay (AB) medium, centrifuged, and the supernatant was filtered using a 0.2-μm pore-size syringe filter (Macherey-Nagel GmbH & Co. KG, Duren, Germany) to create cecal cell-free fluids (CCFF). The samples were stored at –30°C.
An overnight culture of V. harveyi BAA-1117 (sensor 1-, sensor 2+) was diluted 1:1.000 with fresh AB. A total of 90 μl of this cell suspension was mixed with 10 μl of the CCFF in a 96-well polystyrene microplate (Nunc® MicroWell™, Merck KGaA, Darmstadt, Germany). A total of 10 μl of sterile growth medium was used as the negative control. In addition, 10 μl of the cell-free culture fluids of V. harveyi BAA-1119 was used as the positive control to verify the bioassays. The microplates were incubated at 30°C with shaking and luminescence measured every 30 min with a Synergy HT multimode microplate reader (BioTek, Winooski, VT, US) for 7 hours. AI-2-like activity is expressed as relative AI-2-like activity, which was calculated as the ratio of luminescence of the test sample (CCFE) to that of the control (negative) sample.
Data were analyzed using a general linear model (GLM): a general factorial ANOVA procedure using dietary ME and CP specifications (i.e., 95% and 100%) and phytogenic inclusion level (i.e., 0 and 150 mg/kg diet) as fixed factors on individual broiler basis. In addition, data were tested for normality using the Kolmogorov–Smirnov test and found to be normally distributed. Moreover, the statistically significant effects were further analyzed, and means were compared using Tukey’s honest significant difference multiple comparison procedure. Statistical significance was determined at a P-value <0.05. All statistical analyses were done using SPSS for Windows Statistical Package Program (SPSS Inc., Chicago, IL, US).
A significant interaction of diet specifications × phytogenic (PD×P ≤ 0.01) was noted for the concentration of Lactobacillus spp. and Clostridium cluster IV. In particular, treatments D100 and D100+ were associated with a higher concentration of Lactobacillus spp. In addition, treatment D100+ was associated with higher Clostridium cluster IV levels than treatment D95+, whereas treatments D100 and D95 were intermediate. Diet specifications were the main factor significantly affecting cecal mucosa‐associated microbiota (Figure 1). Broilers with diets formulated with the 100% ME and CP specifications had significantly higher concentrations of total bacteria (PD ≤ 0.05), Bacteroides spp. (PD ≤ 0.05), Lactobacillus spp. (PD ≤ 0.01), and Clostridium cluster XIVa (PD ≤ 0.01). Phytogenic addition did not significantly affect (P > 0.05) the cecal mucosa‐associated microbiota.
Figure 1 Effect of diet specifications (A) and phytogenic inclusion (B) and interaction effect of diet specifications × phytogenic inclusion (C) in cecal mucosa-associated bacteria of 42-day-old broilers. Columns indicate means + SE and the asterisks denote level of statistical difference *P ≤ 0.05, **P ≤ 0.01. Treatment means with different superscripts a, b differ significantly (P < 0.05).
There were no significant (P > 0.05) interactions of diet specifications × phytogenic at cecal digesta. Diet specifications were the main factor that significantly affected Clostridium cluster XIVa levels in cecal digesta (Figure 2). In particular, broilers fed diets formulated with the 100% specifications had significantly higher concentrations (PD ≤ 0.05) of Clostridium cluster XIVa. Phytogenic addition significantly decreased E. coli (PP ≤ 0.05) and Clostridium cluster I (PP ≤ 0.05) levels and significantly increased the Clostridium cluster IV (PP ≤ 0.01) concentration.
Figure 2 Effect of diet specifications (A) and phytogenic inclusion (B) in cecal digesta microbiota of 42-day-old broilers. Columns indicate means + SE and the asterisks denote level of statistical difference *P ≤ 0.05, **P ≤ 0.01.
A significant interaction of diet specifications × phytogenic was noted for acetic acid (PD×P ≤ 0.01). In particular, the acetic molar ratio was higher in treatments D100 and D95+ than in treatment D95, whereas treatment D100+ was intermediate. Diet specifications significantly affected the cecal digesta VFA pattern (Table 4). In particular, the butyric acid molar ratio was significantly higher (PP ≤ 0.05) in broilers fed diets formulated with the 100% specifications. Phytogenic addition significantly increased the butyric molar ratio (PP ≤ 0.05) and significantly decreased the branched VFA (PP ≤ 0.05) molar ratios.
Table 4 Effects of diet specifications %, phytogenic inclusion, and their interaction on the volatile fatty acid (VFA) concentration and molar ratios in the cecal digesta of 42-day-old broilers.
An interaction of diet specifications × phytogenic (PD×P ≤ 0.01) was noted for the relative expression of TLR2B (PD×P ≤ 0.05) and TLR4 (PD×P ≤ 0.05). The relative expression of TLR2B was lower in treatment D100+ than in the other treatments and the relative expression of TLR4 was lower in treatment D100+ than in treatments D95 and D95+ (Table 5). Diet specifications as a main factor significantly affected (PD ≤ 0.05) TLR4 relative expression. In particular, TLR4 expression was significantly lower in broilers fed diets formulated with the 100% specifications. Phytogenic supplementation did not affect (PP ≤ 0.05) relative gene expressions in the broiler ceca tissue.
Table 5 Effects of diet specifications %, phytogenic inclusion, and their interaction on mRNA expression of, barrier function, immune and nutrient sensing genes in chicken cecal mucosa.
An interaction of diet specifications × phytogenic was noted for relative expression of LEAP2 (PD×P ≤ 0.05) and AVBD6 (PD×P ≤ 0.01). In particular, broilers with a diet formulated with 100% specifications and supplemented with phytogenic (D100+) had lower expression levels of LEAP2 than broilers with a diet formulated with 100% specifications and no phytogenic addition (D100+). Transcripts of AVBD6 were lower in treatments D100 and D100+ than in treatment D95, whereas treatment D95+ received intermediate values and differed from the rest of the treatments. AVBD6 expression was significantly affected (PD ≤ 0.001) by diet specifications. Broilers with a diet formulated with 100% specifications had lower AVBD6 transcripts. Broilers with a diet supplemented with phytogenic had significantly lower values of LEAP2 (PP ≤ 0.05) and AVBD6 (PP ≤0.05) transcripts.
A significant interaction (PD×P ≤ 0.01) of diet specifications × phytogenic was noted for cecal AI-2 relative activity. In particular, treatment D95+ had higher AI-2 relative activity than the other three treatments (Figure 3). Broilers with a diet formulated with 95% specifications had significantly higher (PD ≤ 0.01) AI-2 relative activity. Phytogenic addition significantly increased (PP ≤ 0.05) AI-2 relative activity in the cecal digesta.
Figure 3 Effects of diet specifications %, phytogenic inclusion, and interaction effect of diet specifications × phytogenic inclusion on cecal AI-2 relative activity. Columns indicate means + SE and the asterisks denote level of statistical difference *P ≤ 0.05, **P ≤ 0.01. Treatment means with different superscripts (A, B) differ significantly (P < 0.05).
It has been shown that dietary metabolizable energy (ME) and crude protein (CP) reduction negatively affected broiler performance and that phytogenics inclusion tended to ameliorate this effect (Paraskeuas et al., 2016; Griela et al., 2021). Several mechanisms for the growth enhancement of phytogenics have been proposed, some of which include antioxidant activity, antimicrobial and anti-quorum-sensing activity, and anti-inflammatory and transcription-modulating effects on gut health biomarkers (Pandey et al., 2019; Kikusato, 2021). Although phytogenics are mainly absorbed in the proximal gut, there is evidence that phytogenic addition can also modulate the cecal microbiota and the overall cecal ecosystem (Paraskeuas et al., 2016). The ceca have long been a target of research due to the wide range of microorganisms it harbors, which continuously signal mainly through metabolites to the gut epithelium, affecting bird health and productivity (Shang et al., 2018; Rodrigues et al., 2020). In turn, host receptors play a critical role in host–microbe communication through the recognition of bacteria and the subsequent immune response to preserve homoeostasis (Pan and Yu, 2014). The present study provides new knowledge on the effects of dietary energy and protein levels with or without phytogenic addition on the modulation of microbiota composition and metabolic activity, bacterial communications and host signaling, inflammation, and defense.
Different dietary components can change the structure and function of the gut microbiome. In this study dietary ME and CP specifications in combination with phytogenic administration were shown to affect the mucosa-associated microbiota (Lactobacillus spp. and Clostridium cluster IV). Moreover, diet specification was the main factor to modulate mucosa-associated microbiota, whereas phytogenic administration modulated cecal digesta microbiota. Limitations of nutrients in the host diet are known to shape the structure of gut-associated microbial communities (Luo et al., 2015; Dong et al., 2017; Paraskeuas and Mountzouris, 2019b). In addition, it is already known that phytogenics based on carvacrol, anethol, and limonen (Mountzouris et al., 2011; Paraskeuas and Mountzouris, 2019a) can shape, in a beneficial way, the cecal microbiota profile. In this study phytogenic addition favored the growth of bacteria belonging to Clostridium cluster IV (C. leptum group), a cluster that contains numerous butyrate-producing and fibrolytic species, whose metabolic activities have a positive effect on host gut health (Jeraldo et al., 2016; Guo et al., 2020), whereas the supplementation of phytogenics acted in an antimicrobial manner and decreased the levels of microbiota species (i.e., E. coli and Clostridium cluster I) that could act as pathogens in broilers (Fancher et al., 2020; Murase and Ozaki, 2022).
Most known microbiota-derived metabolites are volatile fatty acids (VFAs; e.g., acetate, propionate, and butyrate), which have a multifaceted effect on the composition of the microbiota and on the host, beyond their contribution as an energy substrate to the intestinal epithelium (van der Hee and Wells, 2021). VFAs directly affect intestinal microbial composition by a cross-feeding mechanism, by their antimicrobial effects or by affecting bacterial gene expression, and indirectly affect intestinal microbial composition by maintaining the integrity of the gut barrier and modulating intestinal immunity (van Der Wielen et al., 2000; Sunkara et al., 2011; Lamas et al., 2019). Hence, dietary strategies leading to increased VFA production may benefit biomarkers of gut health.
In the present study, both diet specifications and phytogenic supplementation individually or in combination modulated the cecal microbial metabolism, as significant changes were noted for the cecal VFA pattern. In particular, an interaction of diet specification × phytogenic supplementation was revealed for the acetic acid molar ratio. In addition, the 95% ME and CP specification diet decreased the molecular ratio of butyric acid, while phytogenic supplementation increased the butyric molar ratio and decreased branched VFAs. The changes in the VFA pattern observed in this work may be influenced by the cecal microbiota composition (Cao et al., 2010) or by the amount and type of feed substrates reaching the ceca (Svihus et al., 2013). The reduced branched-VFA molar ratios observed in this study by dietary phytogenic addition could be considered to be a beneficial feature for chicken gut ecology. Concentrations of branched VFAs may be used as an indicator for protein fermentation (Macfarlane et al., 1992). Excess protein fermentation in the gut is not desirable because harmful metabolites are produced (e.g., ammonia) which, in addition to burdening the environment, have been linked to negative effects on the epithelium (Qaisrani et al., 2015; Naseem and King, 2018)
The crosstalk among bacteria, the immune system, and dietary factors is able to modulate the mucosal barrier function (De Santis et al., 2015). The intestinal epithelium forms a dynamic physicochemical barrier to maintain immune homeostasis. Gut mucosae include physical barriers (i.e., mucus layer and the cell junction) and chemical barriers (i.e., antimicrobial peptides). In this sense, an additional purpose of this study was to assess the effect of the dietary factors examined in the relative quantification of gene transcripts that encode mucin 2, the major component of mucus in the large intestine (Duangnumsawang et al., 2021); zonula occludens-1 and -2; claudin-1 and occludin, tight junction proteins that regulate epithelial barriers (Lee, 2015); toll-like receptors 2 (type 2) and 4, which play a crucial role in the innate immune system by recognizing bacteria (Hug et al., 2018); interferon-γ, nuclear factor-κB, and inducible nitric oxide synthase, which are associated with inflammation (Aktan, 2004); and gallinacin-1 and -6, cathelicidin-2 and liver-expressed antimicrobial peptide 2, which are antimicrobial peptides and are part of the innate immune response (Cuperus et al., 2013). In addition, the gene expression of free fatty acid receptors 2 and 4, which are important biomarkers of intestinal microflora activity (Slawinska et al., 2019; Bartoszek et al., 2020), were also determined.
Tight junction proteins are the most significant feature of gut integrity and make up a barrier in the paracellular space. These proteins are subject to alteration and remodeling in response to external stimuli in the gut environment, such as nutrients and bacteria (commensals or pathogens) (Ulluwishewa et al., 2011). So far it has been shown that in non-challenging conditions the effect of reduced energy and protein levels has little impact on intestinal tight junction gene expression (Barekatain et al., 2019; Paraskeuas and Mountzouris, 2019a) and intestinal permeability (Barekatain et al., 2019) compared with standard full-specification diets. This work confirmed these effects since reduction of CP and ME by 5% did not impact expression of tight junction-related genes. Moreover, the fact that the inflammatory biomarkers studied (i.e., the expression of NFKB1, NOS2, IFNG) were not altered by the reduced ME and CP levels may also be explained by the optimal management and hygiene conditions followed in this study. In another study, reducing CP levels in combination with aflatoxin challenge altered tight junction-related gene expression, exacerbating the effect of aflatoxicosis on intestinal permeability, which was improved with a 10% increase in CP (Chen et al., 2016). Thus, the mucosa barrier can be affected by low-CP diets when a stress factor is present. Further research is needed to determine the effect of reduced ME and CP levels under commercial conditions where chicks are raised in environments with multiple stressors. On the other hand, phytogenic effects on the gene expression of tight junction proteins have been shown to vary from no effects (phytogenic based on menthol and anethole; Paraskeuas and Mountzouris, 2019a), as in this study and a previous study, to positive effects noted by TJ upregulation (phytogenic based on carvacrol, anethol, and limonen; Paraskeuas and Mountzouris, 2019b). It is understood that factors such as phytogenic composition and inclusion level may impact TJ expression, especially under challenging conditions (Ibrahim et al., 2020; Hashem et al., 2022).
The gut is constantly exposed to antigens that can trigger an immune response, which is characterized by activation of the NF-κb pathway. One of the negative regulatory mechanisms of this pathway is the downregulation of the transcription of TLRs and related genes (Villena and Kitazawa, 2014). In the present study, the 95% ME and CP specification diet resulted in upregulation of TLR4, whereas the 100% ME and CP specification diet with phytogenic downregulated both TLR4 and TLR2B expression in the cecal epithelium. In healthy conditions, in intestinal epithelial cells, TLR expression is repressed to maintain homeostasis of the intestine and allow for commensal microbiome development (Villena and Kitazawa, 2014; Bruning et al., 2021). This effect could be attributed to either a bacteria-mediated mechanism due to the changes observed in the cecal microbiota or to the blocking of the binding of TLR ligands to the corresponding receptors, thus interfering with the intracellular signaling pathways (Paraskeuas and Mountzouris, 2019b; Paraskeuas and Mountzouris, 2019a; Rehman et al., 2021), or a combination of both.
Host defense peptides (HDPs), also known as antimicrobial peptides, are a critical component of the animal innate immune system, with direct antimicrobial (against bacteria, fungi, and viruses) and immunomodulatory activities (Hilchie et al., 2013). Avian HDPs have been shown to be modulated by dietary factors (Shao et al., 2016; Tian et al., 2016; Robinson et al., 2018) or by pathogens (Shao et al., 2016; Tian et al., 2016; Su et al., 2017). In this study, the inclusion of phytogenic in the 100% ME and CP specification diet did not affect the expression of AvBD6. However, phytogenic inclusion in the 95% ME and CP specification diet reduced the expression of AvBD6 compared with phytogenic exclusion in this diet. In addition, phytogenic inclusion downregulated the expression of LEAP2 in the 100% ME and CP specification diet. These results highlight the role of diet in the efficacy of phytogenics. Chicken AvBD6 and LEAP2 play an important role in chicken innate host defense (Van Dijk et al., 2007; Su et al., 2017) in ceca. However, a strong immune response is detrimental to the host and performance of animals in a chronic inflammation site such as the gut. In the case of healthy broilers, downregulation of HDPs could point to phytogenics’ potential to contribute towards an improved intestinal environment via an anti-inflammatory mechanism. Downregulation of AvBD6 and LEAP2 will have to be considered in context, considering overall zootechnical performance, which has been shown to tend to be improved by phytogenic addition (Griela et al., 2021).
In the “noisy” environment of the gut, bacteria rely on quorum sensing to regulate survival and to compete with others for spatial dominance (Wu and Luo, 2021). In this study, the inclusion of phytogenic in the 100% ME and CP specifications diet (D100+) did not affect the relative activity of AI-2; however, phytogenic inclusion in the 95% ME and CP specifications diet (D95+) increased the relative expression of AI-2 compared with the non-supplementation of phytogenic. This result indicated that the combination of reduced ME and CP and phytogenic inclusion affected interspecies communication and may determine bacterial group behaviors (Fu et al., 2020). Thompson et al. (2015) showed that, in the mammalian gastrointestinal tract, AI-2 mediated communication among bacteria, thereby shaping the structure of the microbial community. In vitro studies that used pathogens isolated from broilers reported that plant extracts, α-pinene or carvacrol, act as quorum-sensing inhibitors (Šimunović et al., 2020; Wagle et al., 2020). The latter may be through an antagonistic action due to the similarity of their chemical structure to those of quorum-sensing signals or their ability to degrade quorum-sensing receptors such as autoinducer binding domain-containing protein (LuxR)/transcriptional regulator LasR (Kalia, 2013). In the present study, AI-2 relative expression was positively regulated on supplementation of phytogenic and this could be due to the presence of a multitude of AI-2-producing bacteria that could have been differentially affected by phytogenics. Consequently, it could be postulated that, except their bacterial growth-inhibiting ability, phytogenics, through diverse mechanisms of AI-2 regulation, can affect the communication of microbiota and, as a result, further modulate microbiota composition. As there are no other studies investigating the effect of phytogenic inclusion on the role of AI-2 in broiler guts, further metatranscriptomic and metagenomic research will be required to reveal and associate the changes of the bacterial behavior with the microbiota structure (composition) at a detailed level.
In conclusion, it has been shown that ME and CP dietary specifications, combined or not with phytogenic, modulate multilevel gut biomarkers, from microbiota composition and metabolic activity to microbial communications and host signaling, inflammation, and defense. Further exploration using metatranscriptomic analysis combined with metagenomic analysis of microbiota will provide important details about the changes in bacterial behavior in the complex gut environment.
The raw data supporting the conclusions of this article will be made available by the authors, without undue reservation.
The animal study was reviewed and approved by the Department of Agriculture and Veterinary Policy, General Directorate of Agriculture, Economy, Veterinary and Fisheries.
KM and IP conceived the original idea. KM and IP conceived and planned the experiments. IP performed the analytical assays. IP wrote the manuscript with support from KM and VP. KM supervised the project. All authors provided critical feedback and helped shape the research, analysis, and manuscript. All authors contributed to the article and approved the submitted version.
This research is co-financed by Greece and the European Union (European Social Fund- ESF) through the Operational Programme «Human Resources Development, Education and Lifelong Learning» in the context of the project “Reinforcement of Postdoctoral Researchers - 2nd Cycle” (MIS-5033021), implemented by the State Scholarships Foundation (IKY).
The authors would like to thank Prof. Nychas from the Laboratory of Food Microbiology and Biotechnology, Department of Food Science and Human Nutrition, Department of Biotechnology, Agricultural University of Athens, for free disposition of the V. harveyi BAA-1117 and V. harveyi BAA-1119.
The authors declare that the research was conducted in the absence of any commercial or financial relationships that could be construed as a potential conflict of interest.
All claims expressed in this article are solely those of the authors and do not necessarily represent those of their affiliated organizations, or those of the publisher, the editors and the reviewers. Any product that may be evaluated in this article, or claim that may be made by its manufacturer, is not guaranteed or endorsed by the publisher.
The Supplementary Material for this article can be found online at: https://www.frontiersin.org/articles/10.3389/fanim.2022.1094314/full#supplementary-material
Aktan F. (2004). iNOS-mediated nitric oxide production and its regulation. Life Sci. 75, 639–653. doi: 10.1016/j.lfs.2003.10.042
Baba E., Nagaishi S., Fukata T., Arakawa A. (1991). The role of intestinal microflora on the prevention of salmonella colonization in gnotobiotic chickens. Poult Sci. 70, 1902–1907. doi: 10.3382/ps.0701902
Barekatain R., Nattrass G., Tilbrook A. J., Chousalkar K., Gilani S. (2019). Reduced protein diet and amino acid concentration alter intestinal barrier function and performance of broiler chickens with or without synthetic glucocorticoid. Poult Sci. 98, 3662–3675. doi: 10.3382/ps/pey563
Bartoszek A., Moo E., Binienda A., Fabisiak A., Krajewska J. B., Mosińska P., et al. (2020). Free fatty acid receptors as new potential therapeutic target in inflammatory bowel diseases. Pharmacol. Res. 152, 104604. doi: 10.1016/j.phrs.2019.104604
Bravo D., Pirgozliev V., Rose S. P. (2014). A mixture of carvacrol, cinnamaldehyde, and capsicum oleoresin improves energy utilization and growth performance of broiler chickens fed maize-based diet. J. Anim Sci. 92, 1531–1536. doi: 10.2527/jas.2013-6244
Brisbin J. T., Davidge L., Roshdieh A., Sharif S. (2015). Characterization of the effects of three lactobacillus species on the function of chicken macrophages. Res. Vet. Sci. 100, 39–44. doi: 10.1016/j.rvsc.2015.03.003
Brisbin J. T., Gong J., Parvizi P., Sharif S. (2010). Effects of lactobacilli on cytokine expression by chicken spleen and cecal tonsil cells. Clin. Vaccine Immunol. 17, 1337–1343. doi: 10.1128/cvi.00143-10
Brisbin J. T., Gong J., Sharif S. (2008). Interactions between commensal bacteria and the gut-associated immune system of the chicken. Anim Heal. Res. Rev. 9, 101–110. doi: 10.1017/s146625230800145x
Bruning E., Coller J., Wardill H., Bowen J. (2021). Site-specific contribution of toll-like receptor 4 to intestinal homeostasis and inflammatory disease. J. Cell Physiol. 236, 877–888. doi: 10.1002/jcp.29976
Cao P. H., Li F. D., Li Y. F., Ru Y. J., Péron A., Schulze H., et al. (2010). Effect of essential oils and feed enzymes on performance and nutrient utilization in broilers fed a corn/soy-based diet. Int. J. Poult Sci. 9, 749–755. doi: 10.3923/ijps.2010.749.755
Celi P., Cowieson A. J., Fru-Nji F., Steinert R. E., Kluenter A. M., Verlhac V. (2017). Gastrointestinal functionality in animal nutrition and health: New opportunities for sustainable animal production. Anim Feed Sci. Technol. 234, 88–100. doi: 10.1016/j.anifeedsci.2017.09.012
Chen X., Naehrer K., Applegate T. J. (2016). Interactive effects of dietary protein concentration and aflatoxin B1 on performance, nutrient digestibility, and gut health in broiler chicks. Poult Sci. 95, 1312–1325. doi: 10.3382/ps/pew022
Clifford R. J., Milillo M., Prestwood J., Quintero R., Zurawski D. V., Kwak Y. I., et al. (2012). Detection of bacterial 16S rRNA and identification of four clinically important bacteria by real-time PCR. PloS One 7, e48558. doi: 10.1371/journal.pone.0048558
Cuperus T., Coorens M., van Dijk A., Haagsman H. P. (2013). Avian host defense peptides. Dev. Comp. Immunol. 41, 352–369. doi: 10.1016/j.dci.2013.04.019
Delroisse J.-M., Boulvin A.-L., Parmentier I., Dauphin R. D., Vandenbol M., Portetelle D. (2008). Quantification of bifidobacterium spp. and lactobacillus spp. in rat fecal samples by real-time PCR. Microbiol. Res. 163, 663–670. doi: 10.1016/j.micres.2006.09.004
De Santis S., Cavalcanti E., Mastronardi M., Jirillo E., Chieppa M. (2015). Nutritional keys for intestinal barrier modulation. Front. Immunol. 6. doi: 10.3389/fimmu.2015.00612
Directive C. (2007). 43/EC of 28 June 2007 laying down minimum rules for the protection of chickens kept for meat production. Off. J. Eur. Union L 182, 19–28.
Directive E. U. (2010). 63/EU of the European parliament and of the council of 22 September 2010 on the protection of animals used for scientific purposes. Off. J. Eur. Union 276, 33–79.
Dong X. Y., Azzam M. M. M., Zou X. T. (2017). Effects of dietary threonine supplementation on intestinal barrier function and gut microbiota of laying hens. Poult Sci. 96, 3654–3663. doi: 10.3382/ps/pex185
Dore J., Ehrlich S. D., Levenez F., Pelletier E., Alberti A., Bertrand L., et al. (2015). HMS_SOP 06 V1: Standard operating procedure for fecal samples DNA extraction. Protocol Q. Int. Hum. Microbiome Stand.
Duangnumsawang Y., Zentek J., Goodarzi Boroojeni F. (2021). Development and functional properties of intestinal mucus layer in poultry. Front. Immunol. 12. doi: 10.3389/fimmu.2021.745849
Elling-Staats M. L., Gilbert M. S., Smidt H., Kwakkel R. P. (2021). Caecal protein fermentation in broilers: A review. Worlds Poult Sci J. 78, 103–123. doi: 10.1080/00439339.2022.2003170
Fancher C. A., Zhang L., Kiess A. S., Adhikari P. A., Dinh T. T. N., Sukumaran A. T. (2020). Avian pathogenic escherichia coli and clostridium perfringens: Challenges in no antibiotics ever broiler production and potential solutions. Microorganisms 8, 1–27. doi: 10.3390/microorganisms8101533
Fu C. Y., Li L. Q., Yang T., She X., Ai Q., Wang Z. L. (2020). Autoinducer-2 may be a new biomarker for monitoring neonatal necrotizing enterocolitis. Front. Cell. Infect. Microbiol. 10. doi: 10.3389/fcimb.2020.00140/bibtex
Goodarzi Boroojeni F., Vahjen W., Mader A., Knorr F., Ruhnke I., Röhe I., et al. (2014). The effects of different thermal treatments and organic acid levels in feed on microbial composition and activity in gastrointestinal tract of broilers. Poult Sci. 93, 1440–1452. doi: 10.3382/ps.2013-03763
Griela E., Paraskeuas V., Mountzouris K. C. (2021). Effects of diet and phytogenic inclusion on the antioxidant capacity of the broiler chicken gut. Anim an Open Access J. MDPI 11, 1–15. doi: 10.3390/ani11030739
Guo P., Zhang K., Ma X., He P. (2020). Clostridium species as probiotics: Potentials and challenges. J. Anim Sci. Biotechnol. 11, 1–10. doi: 10.1186/s40104-019-0402-1
Hashem Y. M., Abd El-Hamid M. I., Awad N. F. S., Ibrahim D., Elshater N. S., El-Malt R. M. S., et al. (2022). Insights into growth-promoting, anti-inflammatory, immunostimulant, and antibacterial activities of toldin CRD as a novel phytogenic in broiler chickens experimentally infected with mycoplasma gallisepticum. Poult Sci. 101, 102154. doi: 10.1016/j.psj.2022.102154
Hellemans J., Mortier G., De Paepe A., Speleman F., Vandesompele J. (2007). qBase relative quantification framework and software for management and automated analysis of real-time quantitative PCR data. Genome Biol. 8, R19. doi: 10.1186/gb-2007-8-2-r19
Hilchie A. L., Wuerth K., Hancock R. E. W. (2013). Immune modulation by multifaceted cationic host defense (antimicrobial) peptides. Nat. Chem. Biol. 9, 761–768. doi: 10.1038/nchembio.1393
Hsiao A., Ahmed A. M. S., Subramanian S., Griffin N. W., Drewry L. L., Petri W. A., et al. (2014). Members of the human gut microbiota involved in recovery from vibrio cholerae infection. Nature 515, 423–426. doi: 10.1038/nature13738
Hug H., Mohajeri M. H., La Fata G. (2018). Toll-like receptors: Regulators of the immune response in the human gut. Nutrients 10, 203. doi: 10.3390/nu10020203
Hu R., Lin H., Wang M., Zhao Y., Liu H., Min Y., et al. (2021). Lactobacillus reuteri-derived extracellular vesicles maintain intestinal immune homeostasis against lipopolysaccharide-induced inflammatory responses in broilers. J. Anim Sci. Biotechnol. 12, 1–18. doi: 10.1186/s40104-020-00532-4
Ibrahim D., Sewid A. H., Arisha A. H., El-fattah A. H., Abdelaziz A. M., Al-Jabr O. A., et al. (2020). Influence of glycyrrhiza glabra extract on growth, gene expression of gut integrity, and campylobacter jejuni colonization in broiler chickens. Front. Vet. Sci. 7. doi: 10.3389/fvets.2020.612063
Jeraldo P., Hernandez A., Nielsen H. B., Chen X., White B. A., Goldenfeld N., et al. (2016). Capturing one of the human gut microbiome’s most wanted: Reconstructing the genome of a novel butyrate-producing, clostridial scavenger from metagenomic sequence data. Front. Microbiol. 7. doi: 10.3389/fmicb.2016.00783
Joly P., Falconnet P.-A., Andre J., Weill N., Reyrolle M., Vandenesch F., et al. (2006). Quantitative real-time legionella PCR for environmental water samples: Data interpretation. Appl. Environ. Microbiol. 72, 2801–2808. doi: 10.1128/aem.72.4.2801-2808.2006
Kalia V. C. (2013). Quorum sensing inhibitors: An overview. Biotechnol. Adv. 31, 224–245. doi: 10.1016/j.biotechadv.2012.10.004
Kikusato M. (2021). Phytogenics to improve health and production of broiler chickens: Functions beyond the antioxidant activity. Anim Biosci. 34, 345. doi: 10.5713/ab.20.0842
Lamas A., Regal P., Vázquez B., Cepeda A., Franco C. M. (2019). Short chain fatty acids commonly produced by gut microbiota influence salmonella enterica motility, biofilm formation, and gene expression. Antibiotics 8, 265. doi: 10.3390/antibiotics8040265
Laudadio V., Passantino L., Perillo A., Lopresti G., Passantino A., Khan R. U., et al. (2012). Productive performance and histological features of intestinal mucosa of broiler chickens fed different dietary protein levels. Poult Sci. 91, 265–270. doi: 10.3382/ps.2011-01675
Lee S. H. (2015). Intestinal permeability regulation by tight junction: Implication on inflammatory bowel diseases. Intest Res. 13, 11. doi: 10.5217/ir.2015.13.1.11
Li M., Gong J., Cottrill M., Yu H., de Lange C., Burton J., et al. (2003). Evaluation of QIAamp DNA stool mini kit for ecological studies of gut microbiota. J. Microbiol. Methods 54, 13–20. doi: 10.1016/s0167-7012(02)00260-9
Li Q., Ren Y., Fu X. (2019). Inter-kingdom signaling between gut microbiota and their host. Cell. Mol. Life Sci. 76, 2383–2389. doi: 10.1007/s00018-019-03076-7
Luo Z., Li C., Cheng Y., Hang S., Zhu W. (2015). Effects of low dietary protein on the metabolites and microbial communities in the caecal digesta of piglets. Arch. Anim. Nutr. 69, 212–226. doi: 10.1080/1745039x.2015.1034521
Macfarlane G. T., Gibson G. R., Beatty E., Cummings J. H. (1992). Estimation of short-chain fatty acid production from protein by human intestinal bacteria based on branched-chain fatty acid measurements. FEMS Microbiol. Ecol. 10, 81–88. doi: 10.1111/j.1574-6941.1992.tb00002.x
Marshall O. J. (2004). PerlPrimer: Cross-platform, graphical primer design for standard, bisulphite and real-time PCR. Bioinformatics 20, 2471–2472. doi: 10.1093/bioinformatics/bth254
Matsuki T., Watanabe K., Fujimoto J., Takada T., Tanaka R. (2004). Use of 16S rRNA gene-targeted group-specific primers for real-time PCR analysis of predominant bacteria in human feces. Appl. Environ. Microbiol. 70, 7220–7228. doi: 10.1128/aem.70.12.7220-7228.2004
Mohammadi Gheisar M., Kim I. H. (2018). Phytogenics in poultry and swine nutrition – a review. Ital J. Anim Sci. 17, 92–99. doi: 10.1080/1828051X.2017.1350120
Mountzouris K. C., Palamidi I., Tsirtsikos P., Mohnl M., Schatzmayr G., Fegeros K. (2015). Effect of dietary inclusion level of a multi-species probiotic on broiler performance and two biomarkers of their caecal ecology. Anim Prod. Sci. 55, 484. doi: 10.1071/AN13358
Mountzouris K. C., Paraskeuas V. V., Fegeros K. (2020). Priming of intestinal cytoprotective genes and antioxidant capacity by dietary phytogenic inclusion in broilers. Anim Nutr. 6, 305–312. doi: 10.1016/j.aninu.2020.04.003
Mountzouris K. C., Paraskevas V., Tsirtsikos P., Palamidi I., Steiner T., Schatzmayr G., et al. (2011). Assessment of a phytogenic feed additive effect on broiler growth performance, nutrient digestibility and caecal microflora composition. Anim Feed Sci. Technol. 168, 223–231. doi: 10.1016/j.anifeedsci.2011.03.020
Mountzouris K. C., Tsitrsikos P., Palamidi I., Arvaniti A., Mohnl M., Schatzmayr G., et al. (2010). Effects of probiotic inclusion levels in broiler nutrition on growth performance, nutrient digestibility, plasma immunoglobulins, and cecal microflora composition. Poult Sci. 89, 58–67. doi: 10.3382/ps.2009-00308
Murase T., Ozaki H. (2022). Relationship between phylogenetic groups of escherichia coli and pathogenicity among isolates from chickens with colibacillosis and healthy chickens. Poult Sci. 101, 102007. doi: 10.1016/j.psj.2022.102007
Naseem S., King A. J. (2018). Ammonia production in poultry houses can affect health of humans, birds, and the environment–techniques for its reduction during poultry production. Environ. Sci. pollut. Res. 25, 15269–15293. doi: 10.1007/s11356-018-2018-y
Palamidi I., Mountzouris K. C. (2018). Diet supplementation with an organic acids-based formulation affects gut microbiota and expression of gut barrier genes in broilers. Anim Nutr. 4, 367–377. doi: 10.1016/j.aninu.2018.03.007
Pandey A. K., Kumar P., Saxena M. J., Gupta R. C., Srivastava A., Lall R. (2019). “Feed additives in animal health BT - nutraceuticals in veterinary medicine,” ed. Gupta R. C., Srivastava A., Lall R. (Cham: Springer International Publishing), 345–362. doi: 10.1007/978-3-030-04624-8_23
Pan D., Yu Z. (2014). Intestinal microbiome of poultry and its interaction with host and diet. Gut Microbes 5, 108–119. doi: 10.4161/gmic.26945
Paraskeuas V., Fegeros K., Palamidi I., Theodoropoulos G., Mountzouris K. C. (2016). Phytogenic administration and reduction of dietary energy and protein levels affects growth performance, nutrient digestibility and antioxidant status of broilers. J. Poult Sci. 53, 264–273. doi: 10.2141/jpsa.0150113
Paraskeuas V., Mountzouris K. C. (2019a). Broiler gut microbiota and expressions of gut barrier genes affected by cereal type and phytogenic inclusion. Anim Nutr. 5, 22–31. doi: 10.1016/j.aninu.2018.11.002
Paraskeuas V. V., Mountzouris K. C. (2019b). Modulation of broiler gut microbiota and gene expression of toll-like receptors and tight junction proteins by diet type and inclusion of phytogenics. Poult Sci. 98, 2220–2230. doi: 10.3382/ps/pey588
Peinado M. J., Ruiz R., Echávarri A., Aranda-Olmedo I., Rubio L. A. (2013). Garlic derivative PTS-O modulates intestinal microbiota composition and improves digestibility in growing broiler chickens. Anim Feed Sci. Technol. 181, 87–92. doi: 10.1016/j.anifeedsci.2013.03.001
Pfaffl M. W. (2001). A new mathematical model for relative quantification in real-time RT-PCR. Nucleic Acids Res. 29, E45. doi: 10.1093/nar/29.9.e45
Qaisrani S. N., Van Krimpen M. M., Kwakkel R. P., Verstegen M. W. A., Hendriks W. H. (2015). Dietary factors affecting hindgut protein fermentation in broilers: a review. Worlds Poult Sci. J. 71, 139–160. doi: 10.1017/S0043933915000124
Rehman M. S., Rehman S., Yousaf W., Hassan F., Ahmad W., Liu Q., et al. (2021). The potential of toll-like receptors to modulate avian immune system: Exploring the effects of genetic variants and phytonutrients. Front. Genet. 12. doi: 10.3389/fgene.2021.67123
Rehman H. U., Vahjen W., Awad W. A., Zentek J. (2007). Indigenous bacteria and bacterial metabolic products in the gastrointestinal tract of broiler chickens. Arch. Anim Nutr. 61, 319–335. doi: 10.1080/17450390701556817
Robinson K., Ma X., Liu Y., Qiao S., Hou Y., Zhang G. (2018). Dietary modulation of endogenous host defense peptide synthesis as an alternative approach to in-feed antibiotics. Anim Nutr. 4, 160–169. doi: 10.1016/j.aninu.2018.01.003
Rodrigues D. R., Briggs W., Duff A., Chasser K., Murugesan R., Pender C., et al. (2020). Cecal microbiome composition and metabolic function in probiotic treated broilers. PloS One 15, e0225921. doi: 10.1371/journal.pone.0225921
Schwiertz A., Taras D., Schäfer K., Beijer S., Bos N. A., Donus C., et al. (2010). Microbiota and SCFA in lean and overweight healthy subjects. Obesity 18, 190–195. doi: 10.1038/oby.2009.167
Shang Y., Kumar S., Oakley B., Kim W. K. (2018). Chicken gut microbiota: Importance and detection technology. Front. Vet. Sci. 5. doi: 10.3389/fvets.2018.00254
Shao Y., Wang Z., Tian X., Guo Y., Zhang H. (2016). Yeast β-d-glucans induced antimicrobial peptide expressions against salmonella infection in broiler chickens. Int. J. Biol. Macromol 85, 573–584. doi: 10.1016/j.ijbiomac.2016.01.031
Silkie S. S., Nelson K. L. (2009). Concentrations of host-specific and generic fecal markers measured by quantitative PCR in raw sewage and fresh animal feces. Water Res. 43, 4860–4871. doi: 10.1016/j.watres.2009.08.017
Šimunović K., Sahin O., Kovač J., Shen Z., Klančnik A., Zhang Q., et al. (2020). (-)-α-Pinene reduces quorum sensing and campylobacter jejuni colonization in broiler chickens. PloS One 15, e0230423. doi: 10.1371/journal.pone.0230423
Slawinska A., Dunislawska A., Plowiec A., Radomska M., Lachmanska J., Siwek M., et al. (2019). Modulation of microbial communities and mucosal gene expression in chicken intestines after galactooligosaccharides delivery in ovo. PloS One 14, e0212318. doi: 10.1371/journal.pone.0212318
Su S., Dwyer D. M., Miska K. B., Fetterer R. H., Jenkins M. C., Wong E. A. (2017). Expression of host defense peptides in the intestine of eimeria-challenged chickens. Poult Sci. 96, 2421–2427. doi: 10.3382/ps/pew468
Sunkara L. T., Achanta M., Schreiber N. B., Bommineni Y. R., Dai G., Jiang W., et al. (2011). Butyrate enhances disease resistance of chickens by inducing antimicrobial host defense peptide gene expression. PloS One 6, e27225. doi: 10.1371/journal.pone.0027225
Svihus B., Choct M., Classen H. L. (2013). Function and nutritional roles of the avian caeca: A review. Worlds Poult Sci. J. 69, 249–264. doi: 10.1017/s0043933913000287
Taga M. E., Xavier K. B. (2011). “Methods for analysis of bacterial autoinducer-2 production,” in Current protocols in microbiology (Hoboken, NJ, USA: John Wiley & Sons, Inc), 1C.1.1–1C.1.15. doi: 10.1002/9780471729259.mc01c01s23
Thompson J. A., Oliveira R. A., Djukovic A., Ubeda C., Xavier K. B. (2015). Manipulation of the quorum sensing signal AI-2 affects the antibiotic-treated gut microbiota. Cell Rep. 10, 1861–1871. doi: 10.1016/j.celrep.2015.02.049
Tian X., Shao Y., Wang Z., Guo Y. (2016). Effects of dietary yeast β-glucans supplementation on growth performance, gut morphology, intestinal clostridium perfringens population and immune response of broiler chickens challenged with necrotic enteritis. Anim Feed Sci. Technol. C 215, 144–155. doi: 10.1016/j.anifeedsci.2016.03.009
Tierney J., Gowing H., Van Sinderen D., Flynn S., Stanley L., McHardy N., et al. (2004). In vitro inhibition of eimeria tenella invasion by indigenous chicken lactobacillus species. Vet. Parasitol. 122, 171–182. doi: 10.1016/j.vetpar.2004.05.001
Ulluwishewa D., Anderson R. C., McNabb W. C., Moughan P. J., Wells J. M., Roy N. C. (2011). Regulation of tight junction permeability by intestinal bacteria and dietary components. J. Nutr. 141, 769–776. doi: 10.3945/jn.110.135657
van der Hee B., Wells J. M. (2021). Microbial regulation of host physiology by short-chain fatty acids. Trends Microbiol. 29, 700–712. doi: 10.1016/j.tim.2021.02.001
van Der Wielen P. W., Biesterveld S., Notermans S., Hofstra H., Urlings B. A., van Knapen F. (2000). Role of volatile fatty acids in development of the cecal microflora in broiler chickens during growth. Appl. Environ. Microbiol. 66, 2536–2540. doi: 10.1128/aem.66.6.2536-2540.2000
Van Dijk A., Veldhuizen E. J. A., Kalkhove S. I. C., Tjeerdsma-Van Bokhoven J. L. M., Romijn R. A., Haagsman H. P. (2007). The beta-defensin gallinacin-6 is expressed in the chicken digestive tract and has antimicrobial activity against food-borne pathogens. Antimicrob. Agents Chemother. 51, 912–922. doi: 10.1128/aac.00568-06
Van Immerseel F., Russell J. B., Flythe M. D., Gantois I., Timbermont L., Pasmans F., et al. (2006). The use of organic acids to combat salmonella in poultry: a mechanistic explanation of the efficacy. Avian Pathol. 35, 182–188. doi: 10.1080/03079450600711045
Villena J., Kitazawa H. (2014). Modulation of intestinal TLR4-inflammatory signaling pathways by probiotic microorganisms: Lessons learned from lactobacillus jensenii TL2937. Front. Immunol. 4. doi: 10.3389/fimmu.2013.00512
Wagle B. R., Donoghue A. M., Shrestha S., Upadhyaya I., Arsi K., Gupta A., et al. (2020). Carvacrol attenuates campylobacter jejuni colonization factors and proteome critical for persistence in the chicken gut. Poult Sci. 99, 4566–4577. doi: 10.1016/j.psj.2020.06.020
Wu L., Luo Y. (2021). Bacterial quorum-sensing systems and their role in intestinal bacteria-host crosstalk. Front. Microbiol. 12. doi: 10.3389/fmicb.2021.611413/xml/nlm
Keywords: phytogenic, host defense peptides, microbiota, autoinducers-2, volatile fatty acids, gut barrier
Citation: Palamidi I, Paraskeuas VV and Mountzouris KC (2023) Dietary and phytogenic inclusion effects on the broiler chicken cecal ecosystem. Front. Anim. Sci. 3:1094314. doi: 10.3389/fanim.2022.1094314
Received: 09 November 2022; Accepted: 30 November 2022;
Published: 12 January 2023.
Edited by:
Luciana Rossi, University of Milan, ItalyReviewed by:
Carlotta Giromini, University of Milan, ItalyCopyright © 2023 Palamidi, Paraskeuas and Mountzouris. This is an open-access article distributed under the terms of the Creative Commons Attribution License (CC BY). The use, distribution or reproduction in other forums is permitted, provided the original author(s) and the copyright owner(s) are credited and that the original publication in this journal is cited, in accordance with accepted academic practice. No use, distribution or reproduction is permitted which does not comply with these terms.
*Correspondence: Irida Palamidi, SW5wYWxhbWlkaUBhdWEuZ3I=; Konstantinos C. Mountzouris, a21vdW50em91cmlzQGF1YS5ncg==
Disclaimer: All claims expressed in this article are solely those of the authors and do not necessarily represent those of their affiliated organizations, or those of the publisher, the editors and the reviewers. Any product that may be evaluated in this article or claim that may be made by its manufacturer is not guaranteed or endorsed by the publisher.
Research integrity at Frontiers
Learn more about the work of our research integrity team to safeguard the quality of each article we publish.