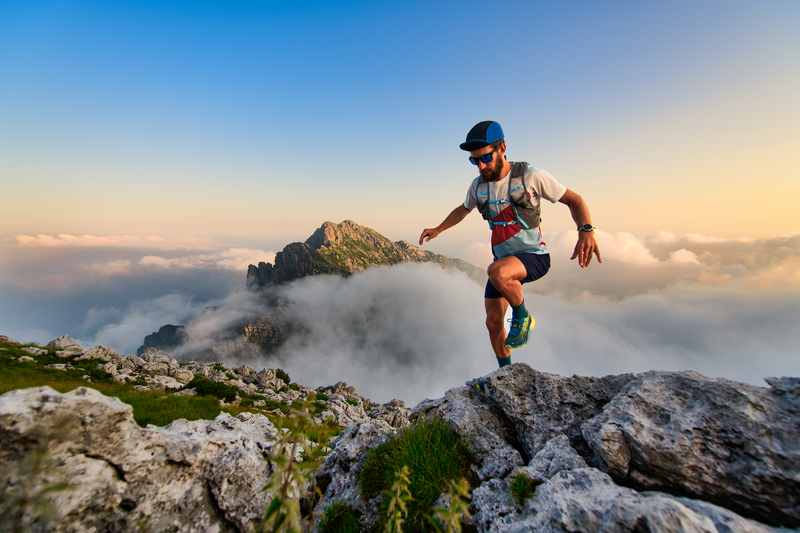
94% of researchers rate our articles as excellent or good
Learn more about the work of our research integrity team to safeguard the quality of each article we publish.
Find out more
ORIGINAL RESEARCH article
Front. Anim. Sci. , 27 January 2023
Sec. Animal Physiology and Management
Volume 3 - 2022 | https://doi.org/10.3389/fanim.2022.1063142
Lameness is a commonly observed disorder in sows and negatively impacts both animal welfare and the profitability of the pig sector. The purpose of this study was to determine anti-inflammatory and/or chondroprotective effects of wheatgrass (WG) on porcine cartilage explants stimulated with lipopolysaccharide (LPS). Explants were aseptically prepared from the intercarpal joints of nine market-weight pigs and placed in culture at 37°C for a total of 120 hours. For the final 96 hours, explants were conditioned with an aqueous extract of WG (0, 5 or 15 μg/mL), and for the final 48 hours explants were stimulated with LPS (0 or 10 µg/mL). Media was removed and replaced every 24 hours. Samples from the final 48 hours were analyzed for biomarkers of cartilage inflammation [prostaglandin E2 (PGE2) and nitric oxide (NO)] and cartilage structure [glycosaminoglycan (GAG)], and cartilage explants were stained for an estimate of cell viability. Stimulation of explants with LPS significantly increased media concentrations of PGE2, GAG and NO compared with that from unstimulated explants. LPS stimulation did not significantly affect cell viability. Conditioning of explants with WG (5 μg/mL) significantly reduced LPS-stimulated cartilage release of PGE2, NO, and GAG (5 and 15 μg/mL), without impairing chondrocyte viability. These data provide evidence for a non-cytotoxic chondroprotective and anti-inflammatory effect of WG extract in cartilage and suggest a role of WG in protection against cartilage breakdown, inflammation, and pain associated with osteoarthritis.
Lameness, characterized by alterations in gait or posture, is commonly observed in sows. It is associated with reduced animal welfare and economic losses, including those associated with increased labour and veterinary costs, decreased slaughter value, and animals that are culled prior to reaching optimal breeding efficiency (Dagorn and Aumaitre, 1979; Anil et al., 2005; Anil et al., 2009; Pluym et al., 2013). Recently, lameness was ranked as the most important measure of welfare in pigs (Whay et al., 2003). Estimates of lameness prevalence vary geographically, but range from 8.8% to 15% for many European countries (Gjein & Larssen, 1995; Bonde et al., 2004; Heinonen et al., 2006; Kilbride et al., 2009; Pluym et al., 2011), 15% in the United States (Lay et al., 2008), and 20% in Canada (Seddon et al., 2013). Rates of lameness may also vary with the productive cycle, with the greatest prevalence of lameness reported after sows were moved to the gestation unit (8.1%) versus 4.1% and 5.5% after being moved to the farrowing pens and insemination cages, respectively (Pluym et al., 2013). Lameness in sows can be attributed to several risk factors, including mixing of individuals resulting in increased aggression (EFSA, 2007), lack of exercise (Fredeen and Sather, 1978), and housing type (stalled or group) coupled with floor/bedding type, such as slatted floors (Heinonen et al., 2006; Anil et al., 2007; Karlen et al., 2007; Spoolder et al., 2017). Causes of lameness include claw and skin lesions, infectious and metabolic disorders, trauma, fractures, osteochondrosis, osteomalacia, and osteoarthritis (Fredeen and Sather, 1978; Wells, 1984; Dewey et al., 1992; Kroneman et al., 1993; Bonde et al., 2004; Kilbride et al., 2009).
Lameness typically results in reduced activity, which may impact social, explorative, and feeding behaviours, including increased lying time and decreased water intake (Madec et al., 1986; Cornou et al., 2008; Weary et al., 2009; Ala-Kurikka et al., 2017). These problems may be exacerbated by group-housing, where lame sows may experience greater degrees of social pressure, be unable to compete for resources, and/or be involved in fighting (Gjein and Larssen, 1995; Heinonen et al., 2013). The stress associated with lameness may also limit the abilities of the immune system to fight infection and disease, which can result in the development of secondary diseases, as well as weight loss and reduced reproductive performance (Bonde et al., 2004; Anil et al., 2009). Inactivity is positively associated with urinary and reproductive infections (Madec et al., 1986; Dee, 1992), which have been reported to account for 6.6 to 8% of all deaths (Chagnon et al., 1991).
Lameness directly impacts longevity, with increased culling and euthanasia or mortality rates of lame sows (Anil et al., 2009), leading to reduced welfare and increased economic costs (Jensen et al., 2010). It is estimated that 6 to 35% of sows are culled due to lameness and claw lesions (Anil et al., 2009). Non-lame sows have a survival time that is more than double that of lame sows after the first farrowing, with a greater reproductive success (lower rates of stillborn or mummified fetuses) in non-lame sows (Anil et al., 2009; Pluym et al., 2011). Furthermore, lame sows tend to have lower litter weights (Fitzgerald et al., 2012) and greater piglet mortality caused by crushing due to increased lying time (Anil et al., 2009). However, lameness itself is not associated with lower rates of pregnancy (Heinonen et al., 2006), instead, lame sows are more likely to be removed from the herd (either due to culling or mortality) earlier than their non-lame counterparts, resulting in fewer overall pregnancies. A study conducted on ten different Danish herds found that of euthanized sows, 72% of cases of euthanasia were associated with the locomotory system, most commonly osteoarthritis (OA; 24%) and fractures (16%), with arthrosis as a secondary diagnosis in 88% of cases (Kirk et al., 2005). Furthermore, OA impacts millions of people worldwide and poses a significant public health concern (Maetzel et al., 2004). Porcine models are considered to be a highly translational model of human OA due to similarities between human and porcine joint sizes, weight-bearing, and cartilage thickness (Cruz et al., 2016). Nonsteroidal anti-inflammatory drugs (NSAIDs) are commonly used in pain management of animals, including swine (Keita et al., 2010; Reiner et al., 2012; Kluivers-Poodt et al., 2013). Using a chemical synovitis model, Pairis-Garcia et al. observed that sows treated with flunixin meglumine and meloxicam had reduced lying posture frequency compared to sows treated with saline, and that pain was mitigated at both 48 and 72 h after lameness induction (Pairis-Garcia et al., 2015). Owing to the absence of a pharmaceutical ‘cure’ for arthritis, and the well-established adverse effects associated with common pharmaceutical pain remedies (Ghanem et al., 2016), feed ingredients targeting maintenance of joint health have emerged on the front-line of OA management in other species. The vast majority of these ‘functional’ feed ingredients with do not have research to support their safety or efficacy in modifying the pathophysiology of OA. A feed ingredient with considerable potential to positively influence clinical outcomes in arthritic animals is wheatgrass (WG; Triticum aestivum). WG is a hardy perennial grass that is a rich source of antioxidant compounds (Durairaj et al., 2014; Parit et al., 2018). Its contemporary popularity as a ‘superfood’ arose initially from a patent in 1930’s which claimed to capture the superior nutritive of sprouted wheatgrass for the purpose of detoxifying the body and providing a concentrated abundance of nutrients. Antioxidant products have demonstrated significant benefit in cases of OA (Ansari et al., 2020), as have extracts rich in polyphenols (Oliviero et al., 2018) such as WG (Durairaj et al., 2014; Benincasa et al., 2015). Therefore, the objective of this study was to characterize the effect of WG on porcine cartilage responses to an inflammatory stimulus (lipopolysaccharide; LPS).
The purpose of this controlled, in-vitro study was to determine anti-inflammatory and/or chondroprotective effects of WG on porcine cartilage explant stimulated with LPS. Briefly, cartilage explants were obtained from the intercarpal joint of nine pigs. From each animal, explants were treated with four conditions, as shown in Figure 1 and described below. Since this study utilized post-mortem tissue from animals slaughtered for reasons unrelated to this study (i.e., for food), animal ethics approval was not required.
Figure 1 Study design for cartilage explants. TSM, tissue culture media; B, Blank (0.28% ethanol in double-distilled water); WG, wheatgrass.
Wheatgrass (WG) extract was prepared by adding WG (190.8 mg) to double distilled water (35mL) and 100% ethanol (100µL). At the same time, an identical blank (B) solution was prepared containing only ethanol and water, without WG. The solutions were incubated at 37°C at 7% CO2 for 2 hours before being centrifuged at 4°C for 20 minutes, and supernatant was separated from the pellet. pH of both supernatants was adjusted to 6.3, before being sterilized through a 0.22µm filter and frozen at -20°C until use.
Explants were prepared and maintained according to the method described by Pearson et al. (2010). Cartilage from nine pigs was aseptically harvested from the intercarpal joints using a 4mm biopsy tool and acclimatized in basal tissue culture media (TCM) for 48 h in 24-well tissue culture plates (two explants per well) at 37°C with 7% CO2. After the initial 48h acclimatization period, TCM was removed and refreshed with one of four conditions: 1. Control (C) – 1000 μL of tissue culture media (TCM); 2. Blank (B) – 970 μL TCM + 30 μL blank solution; 3. T1 – 970 μL TCM + 20 μL B + 10 μL WG (equivalent to a well concentration of 5 μg/mL); 4. T3 –970 μL TCM + 30 μL WG (equivalent to a well concentration of 15 μg/mL). These equate to approximate doses of 18 and 54 g for a 300 kg pig, respectively, assuming a total body water content of approximately 65%.
Media and conditioning were subsequently removed and replaced with fresh solutions every 24 h. After the initial 72 h of culture, half of each of the explant wells were stimulated with LPS (10 ug/mL) for an additional 48h. Explants from each of the nine animals were exposed to each condition.
Media samples were analyzed for biomarkers with importance in cartilage inflammation (PGE2; ELISA and nitric oxide; Griess Reaction) and breakdown (net glycosaminoglycan release; DMB assay) and an assessment of chondrocyte viability (differential live/dead staining).
TCM samples were analyzed for PGE2 using a commercially available ELISA kit (Arbour Assays; cat #K051-H5). Plates were read at absorbance of 450 nm. A best-fit 3rd order polynomial standard curve was developed for each plate (R2 ≥ 0.99), and these equations were used to calculate PGE2 concentrations for samples from each plate.
TCM GAG concentration was determined using a 1,9-Dimethyl Methylene Blue (1,9-DMB) spectrophotometric assay [19]. Samples were added to 96-well plates at 50% dilution, and serially diluted 1:2 up to a final dilution of 1:64. Guanidine hydrochloride (275 mg/mL) was added to each well followed immediately by addition of 150 μL DMB reagent. Absorbance was measured at 530 nm. Sample absorbance was compared to that of a bovine chondroitin sulfate standard (Sigma, Oakville ON). A best-fit linear standard curve was developed for each plate (R2 ≥ 0.99), and these equations used to calculate GAG concentrations for samples on each plate.
Nitrite (NO2-), a stable oxidation product of NO, was analyzed by the Griess reaction [19]. Undiluted TCM samples were added to 96 well plates. Sulfanilamide (0.01 g/mL) and N-(1)-Napthylethylene diamine hydrochloride (1 mg/mL) dissolved in phosphoric acid (0.085 g/L) was added to all wells, and absorbance was read within 5 min at 530 nm. Sample absorbance was compared to a sodium nitrite standard. A best-fit linear standard curve was developed for each plate (R2 ≥ 0.99), and these equations were used to calculate nitrite concentrations for samples from each plate.
Viability of cells within cartilage explants was determined using a Calcein-AM (C-AM)/Ethidium homodimer-1 (EthD-1) cytotoxicity assay kit (Molecular Probes) modified for use in cartilage explants [19]. C-AM and EthD-1 were mixed in sterile distilled water at concentrations of 4 and 8 μM, respectively. Explants were placed one per well into a sterile 96-well microtitre plate and incubated in 200 μL of the C-AM/EthD-1 solution for 40 min at room temperature. The microplate reader (Victor 3 1420 Microplate Reader, Perkin Elmer, Woodbridge ON) was set to scan each well, beginning at the bottom, using 10 horizontal steps at each of 3 vertical displacements set 0.1 mm apart. C-AM and EthD-1 fluorescence in explants were obtained with using excitation/emission filters of 485/530 nm and 530/685 nm, respectively.
All data were analyzed using SigmaPlot (version 14.0) and are reported as mean ± SEM, unless otherwise indicated. Data were analyzed using a 2-way repeated measures ANOVA (with respect to time and treatment) to determine the effect of WG on each outcome measure. A Student t-test was used to determine the effect of treatments on cell viability. When a significant F-ratio was obtained, the Holm Sidak post-hoc test was used to identify differences between treatments. Any treatments stimulated with LPS are designated with + (i.e., C+, B+, T1+ and T3+). Significance was accepted when p < 0.05.
For each outcome measure, C and B explants were initially compared, in order to demonstrate that the vehicle in which WG was extracted did not alter baseline responses of cartilage to LPS. Provided that no differences were identified between C and B explants, effects of WG were only compared with B explants.
There was no significant change in media [NO] in C or B explants across the 48 h sampling time (Figure 2). Stimulation of C+ explants with LPS resulted in a significant increase in media [NO] between 0 (14.7 ± 0.4 μg/mL) and 24 h (18.4 ± 1.3 μg/mL) (p = 0.009), and 0 and 48 h (21.3 ± 1.1 μg/mL) (p < 0.001). Media [NO] was significantly higher in C+ than in C explants at 24 (p = 0.003) and 48 h (p < 0.001).
Figure 2 Media [NO] (µg/mL) from explants cultured in basal media (C) or media conditioned with 1% DD20; Ethanol (0.3%) (B) in the presence (+) or absence of lipopolysaccharide (10µg/mL). *denotes significant change from baseline within treatments. Letters denote significant difference between treatments at individual time points.
Significant increase in [NO] was also observed in B+ explants between 0 (14.9 ± 0.3 μg/mL) and 24 h (19.3 ± 1.1 μg/mL) (p = 0.007), between 0 and 48 h (22.9 ± 2.0 μg/mL) (p < 0.001), and between 24 and 48 h (p = 0.03). Media [NO] was significantly higher in B+ explants than in B explants at 24 (p = 0.007) and 48 h (p < 0.001).
There were no differences in [NO] between C and B (p = 1.0) or C+ and B+ (p = 1.0) at any time point.
There was no change in media [NO] in T1 or T3 explants across the 48 h sampling time course (Figure 3A). Stimulation of T1+ explants with LPS resulted in a significant increase in media [NO] between 0 (14.6 ± 0.2 μg/mL) and 48 h (18.2 ± 1.7 μg/mL) (p < 0.001). Media [NO] was significantly lower in T1+ than in B+ explants at 48 h (p = 0.01) (Figure 3B). Stimulation of T3+ explants with LPS resulted in a significant increase in media [NO] between 0 (14.3 ± 0.5 μg/mL) and 48 h (18.9 ± 1.0 μg/mL) (p < 0.001), and 24 (15.9 ± 1.0 μg/mL) and 48 h (p = 0.007). There were no differences in media [NO] between B+ and T3+ at any time point (Figure 3B).
Figure 3 Media [NO] (µg/mL) from explants cultured in basal media (B) or media conditioned with 5 (T1) or 15 (T3) µg/mL of Wheatgrass Extract. Explants werer maintained without stimulation (A) or were stimulated with lipopolysaccharide (10µg/mL; denoted as’+’) (B). *denotes significant change from baseline within treatments . Letters denote significant difference between treatments at individuals time points.
There was no significant change in media [GAG] in C or B explants across the 48 h sampling time (Figure 4). Stimulation of C+ explants with LPS resulted in a significant increase in media [GAG] between 0 (6.8 ± 0.7 μg/mL) and 24 h (9.9 ± 0.3 μg/mL) (p = 0.05), 24 and 48 h (14.8 ± 1.9 μg/mL) (p < 0.001), and 0 and 48 h (p < 0.001). Media [GAG] was significantly higher in C+ than in C explants at 48 h (p < 0.001). Significant increase in [GAG] was also observed in B+ explants between 0 (6.6 ± 0.7 μg/mL) and 24 h (9.7 ± 1.0 μg/mL) (p = 0.05), 0 and 48 h (14.9 ± 1.9 μg/mL), and between 24 and 48 h (p < 0.001). Media [GAG] was significantly higher in B+ explants than in B explants at 48 h (p < 0.001) (Figure 4). There were no differences in [GAG] between C and B (p = 1.0) or C+ and B+ (p = 1.0) at any time point.
Figure 4 Media [GAG] (µg/mL) from explants cultured in basal media (C) or media conditioned with 1% DD20:Ethanol (0.3%) (B) in the presence (+) or absence of lipopolysaccharide (10 µg/mL). *denotes significant change from baseline within treatments. Letters denote significant difference between treatments at individuals time points.
There was no change in media [GAG] in T1 or T3 explants across the 48 h sampling time course (Figure 5A). Stimulation of T1+ explants with LPS did not result in a significant increase in [GAG] at any time point. Media [GAG] was significantly lower in T1+ (9.1 ± 1.9 μg/mL) than in B+ explants at 48 h (p < 0.001) (Figure 5B). Stimulation of T3+ explants with LPS resulted in a significant increase in media [GAG] between 0 (6.9 ± 0.6 μg/mL) and 48 h (11.8 ± 1.5 μg/mL) (p = 0.04). Media [GAG] was significantly lower in T3+ than in B+ explants at 48 h (p = 0.02) (Figure 5B).
Figure 5 Media [GAG] (µg/mL) from explants cultured in basal media (B) or media conditioned with 5 (T1) or 15 (T3) µg/mL of Wheatgrass Extract. Expalnts were maintained without stimulation (A) or were stimulated with lipopolysaccharide (10 µg/mL;denoted as ‘+’) (B). *denotes significant changefrom baseline within treatments. Letters denote significant difference between treatments at individual time points.
There was no significant change in media [PGE2] in C or B explants across the 48 h sampling time (Figure 6). Stimulation of C+ explants with LPS resulted in a significant increase in media [PGE2] between 0 (204.8 ± 78.4 pg/mL) and 24 h (2133.4 ± 486.4 pg/mL) (p < 0.001), and 0 and 48 h (2160.5 ± 336.9 pg/mL) (p < 0.001). Media [PGE2] was significantly higher in C+ than in C explants at 24 and 48 h (p < 0.001). Significant increase in [PGE2] was also observed in B+ explants between 0 (124.7 ± 57.0 μg/mL) and 24 h (2715.3 ± 480.8 μg/mL) (p < 0.001), 0 and 48 h (2399.1 ± 430.7 μg/mL) (p < 0.001. Media [PGE2] was significantly higher in B+ explants than in B explants at 24 and 48 h (p < 0.001). There were no differences in [PGE2] between C and B (p = 1.0) or C+ and B+ (p = 1.0) at any time point.
Figure 6 Media [PGE2] (µg/mL) from expalnts cultured in basal media (C) or media conditioned with 1% DD20:Ethanol (0.3%) (B) in the presence (+) or absence of lipopolysaccharide (10 µg/mL). *denotes significant change from baseline within treatments. Letters denote significant difference between treatments at individual time points.
There was no change in media [PGE2] in T1 or T3 explants across the 48 h sampling time course (Figure 7A). Stimulation of T1+ explants with LPS resulted in a significant increase in [PGE2] between 0 (254.8 ± 156.9 μg/mL) and 48 h (2159.3 ± 623.9 μg/mL) (p = 0.001). Media [PGE2] was significantly lower in T1+ than in B+ explants at 24 h (p = 0.008). Media [PGE2] was also significantly lower in T1+ than in T3+ explants at 24 h (p = 0.03) (Figure 7B). Stimulation of T3+ explants with LPS resulted in a significant increase in media [PGE2] between 0 (531.0 ± 180.6 μg/mL) and 24 h (2534.2 ± 441.3 μg/mL) (p < 0.001), and 0 and 48 h (2188.2 ± 510.4 μg/mL) (p = 0.009). There were no differences in media [PGE2] between T3+ and B+ explants at any time point (Figure 7B).
Figure 7 Media [PGE2] (µg/mL) from expalnts cultured in basal media (B) or media conditioned with 5 (T1) or 15 (T3) µg/mL of Wheatgrass Extract. Explants were maintained without stimulation (A) or were stimulated with lipopolysaccharide (10 µg/mL; denoted as ‘+’) (B). *denotes significant change from baseline within treatments. Letters denote significant difference between treatments at individual time points.
There was no effect of LPS or WG on cell viability (Figure 8).
Figure 8 Ratio of Calcein-AM to Ethidium homodimer-1 in explants cultured for 96 h For the final 72h, explants were conditioned with wheatgrass extract at 0 (B), 5 (T1) or 15 µg/mL (T3). For the final 48h, explants were stimulated with LPS [0 (unstimulated) or 10 µg/mL (stimulated)].
The purpose of this experiment was to determine the effects of an extract of WG (5 or 15 μg/mL) on porcine cartilage responses to pro-inflammatory stimulation with LPS. We have previously shown that LPS causes an inflammatory response in cartilage, as evidenced by increased production of PGE2, GAG and NO (Pearson et al., 2007; Pearson et al., 2010; Pearson and Kott, 2019; Pearson et al., 2020). The main findings were that conditioning of cartilage explants with both doses of WG extract (5 and 15 μg/mL) for 24 h prior to and 48 h after stimulation with LPS resulted in significantly reduced breakdown of cartilage, as measured by significantly lower GAG release following exposure to LPS in WG-conditioned explants. Furthermore, LPS-induced inflammation was significantly reduced by WG as measured by a significant decrease in LPS-induced PGE2 (5 μg/mL) and NO (5 and 15 μg/mL). These findings provide evidence for a protective effect of WG on cartilage structure and inflammation in the presence of a pro-inflammatory stimulus. Furthermore, WG was found to be safe to cells within cartilage explants, as evidenced by a lack of decline in cell viability after 72 hours of exposure.
Wheatgrass has been suggested as part of the treatment for a variety of degenerative diseases due to its anti-inflammatory and antioxidant properties (Kulkarni et al., 2006; Watzl, 2008; Urbonavičiute et al., 2009; Parit et al., 2018). The effects of WG have primarily been tested in vitro, where it has been observed to be capable of countering all major types of excessive radicals (Watzl, 2008; Durairaj et al., 2014). In rodents, WG supplementation has been found to be beneficial in the treatment and prevention of obesity (Im et al., 2015), protect the liver against alcohol and oxidative stress (Durairaj et al., 2015), and prevent or treat cancer (Zalatnai et al., 2001). In rats with glucocorticoid-induced osteoporosis, 30 days of WG extract, coupled with bisphosphonate and risedronate, were found to increase bone mineral content and decrease bone resporption (Banji et al., 2014). In humans, supplementation of WG alongside standard therapy was found to improve symptoms of rheumatoid arthritis (Bálint et al., 2006). However, to the authors’ knowledge, no evidence exists to date to indicate that WG may play a role in OA specifically.
PGE2 is a key biomarker for pain and inflammation and is the compound that is targeted for inhibition by NSAIDs. PGE2 is directly associated with the pain and inflammation that is the hallmark of arthritis, and its inhibition by WG suggests that sows with clinical signs of OA supplemented with WG will experience a reduction in pain. Similarly, NO is a key biochemical in the pain signaling cascade, as well as a pyrogenic mediator of the periarticular swelling. The strong inhibition of NO by both doses of WG in the current study support a marked anti-inflammatory potential of the plant.
GAGs are highly polarized structural sidechains of aggregating proteoglycans within cartilage. Their high polarity causes them to strongly attract and retain water within cartilage, contributing to the ability of cartilage to resist compressive and shear forces (Volpi, 2006). In the early stages of arthritis, GAGs are cleaved from proteoglycans, contributing to progressive thinning of articular cartilage and impairment of the tissue’s ability to buffer catabolic effects of forces travelling across the articulating joint (Zhou et al., 2018). The mechanism of action of some useful disease modifying agents such as n-acetyl glucosamine derivatives (Cao et al., 2016) and bioactive plant extracts (Pearson et al., 2010; Liu et al., 2015) involves preventing GAG loss during inflammation, resulting in protraction of the disease process and prolonging healthy function of cartilage. Results from the current study provide evidence for a protective effect of WG on cartilage structure during inflammatory stress. The molecular mechanism by which WG exerts this effect is not known but may be associated with the reported inhibitory effect of polyphenols on proteolytic aggrecanases (Cudic et al., 2009) which catalyze breakdown of proteoglycan molecules during catabolic events such as inflammation and degenerative articular diseases. This hypothesis should be explored in future research.
Safety of WG on cartilage cells was determined using fluorescent dye method. We have previously validated and applied this technique for use in cartilage explants to obtain an estimate of the effect of dietary supplements on cartilage explants for which the actual number of cells within the explants is not known (Pearson et al., 2007; Pearson et al., 2010). This technique utilizes Calcein-AM (C-AM) and Ethidium Homodimer-1 (EthD1) to differentially fluoresce in live and dead chondrocytes, respectively. C-AM is transported into live cells across the cell membrane. Once inside, esterases within the cytosol cleave the ‘AM’ portion from calcein, trapping the calcein molecule inside the cell and causing it to fluoresce green. Conversely, EthD1 will only fluoresce when it is able to bind to DNA. Because intact cell membranes are impermeable to EthD1 this binding can only occur when the cell membrane is dead. When explants are submerged in fluid containing C-AM and EthD1 at the appropriate concentration and for the appropriate duration, simultaneous measurements of fluorescence of both C-AM and EthD1 can be obtained, which gives a good estimation of the percent viability of cells within the individual explants. The current study demonstrates that WG is not cytotoxic at the doses evaluated, which approximated 18 and 54 g for a 300 kg sow.
It is concluded that conditioning cartilage explants in the presence of a wheatgrass extract results in protection of cartilage against LPS-stimulated decline in cartilage structure, reduces mediators of inflammation, and is non-cytotoxic. Nutrition of sows has previously been noted as an important predisposing factor of sow lameness (van Riet et al., 2013). Nutritional strategies to support bone remodeling, cartilage metabolism, and horn production are not yet fully understood (van Riet et al., 2013). In addition to ensuring adequate availability of nutrients and appropriate feed intake, there is potential for functional feed ingredients to also aid in supporting musculoskeletal health.
Due to the similarities in joint anatomy, physiology, and biomechanics between humans and pigs, pigs are an excellent translational model to better understanding human OA. Additionally, pigs have large sized litters that mature quickly, making them an excellent non-rodent model (Cruz et al., 2016). Pigs have previously been used as an animal model to study the physiopathology of OA and cartilage repair (Murray and Fleming, 2012; Schlichting et al., 2014; Sieker et al., 2018; Kremen et al., 2020; Zhao et al., 2021). It is possible that similar chondroprotective and anti-inflammatory effects may be associated with WG supplementation in humans. Further research into this area is warranted.
The results of this study provide evidence for a potentially valuable role of WG in preventing joint dysfunction and pain associated with OA, and supports its use for prolonging healthy cartilage in sows. Further research on the effects of this supplementation in live sows is warranted.
The original contributions presented in the study are included in the article/supplementary material. Further inquiries can be directed to the corresponding author/s.
KC - conducted all experiments; analyzed data; critical review and approval of final draft. AG - analyzed data; critical review and approval of final draft. PM - wrote manuscript, critical review and approval of final draft. WP - designed experiment; analyzed data; critical review and approval of final draft. All authors contributed to the article and approved the submitted version.
This research was funded by GreenLife Acres Inc. (St. Clements, Ontario Canada).
The authors declare that this study received funding from GreenLife Acres. The funder was not involved in the study design, collection, analysis, or interpretation of data, the writing of this article or the decision to submit it for publication.
All claims expressed in this article are solely those of the authors and do not necessarily represent those of their affiliated organizations, or those of the publisher, the editors and the reviewers. Any product that may be evaluated in this article, or claim that may be made by its manufacturer, is not guaranteed or endorsed by the publisher.
Ala-Kurikka E., Heinonen M., Mustonen K., Peltoniemi O., Raekallio M., Vainio O., et al. (2017). Behavior changes associated with lameness in sows. Appl. Anim. Behav. Sci. 193, 15–20. doi: 10.1016/j.applanim.2017.03.017
Anil S. S., Anil L., Deen J. (2005). Evaluation of patterns of removal and associations among culling because of lameness and sow productivity traits in swine breeding herds. J. Am. Vet. Med. Assoc. 226, 956–961. doi: 10.2460/javma.2005.226.956
Anil S. S., Anil L., Deen J. (2009). Effect of lameness on sow longevity. J. Am. Vet. Med. Assoc. 235, 734–738. doi: 10.2460/javma.235.6.734
Anil S. S., Anil L., Deen J., Baidoo S. K., Walker R. D. (2007). Factors associated with claw lesions in gestating sows. J. Swine Heal. Prod. 15, 78–83.
Ansari M. Y., Ahmad N., Haqqi T. M. (2020). Oxidative stress and inflammation in osteoarthritis pathogenesis: Role of polyphenols. Biomed. Pharmacother. 129, 110452. doi: 10.1016/j.biopha.2020.110452
Bálint G., Apáthy Á., Gaál M., Telekes A., Resetár Á., Blazsó G., et al. (2006). Effect of avemar® - a fermented wheat germ extract - on rheumatoid arthritis. preliminary data. Clin. Exp. Rheumatol. 24, 325–328.
Banji D., Banji O. J. F., Chiluka V. L., Abbagoni S. (2014). Role of triticum aestivum aqueous extract in glucocorticoid induced osteoporosis in rats. Indian J. Exp. Biol. 52, 153–158.
Benincasa P., Galieni A., Manetta A. C., Pace R., Guiducci M., Pisante M., et al. (2015). Phenolic compounds in grains, sprouts and wheatgrass of hulled and non-hulled wheat species. J. Sci. Food Agric. 95, 1795–1803. doi: 10.1002/jsfa.6877
Bonde M., Rousing T., Badsberg J. H., Sørensen J. T. (2004). Associations between lying-down behaviour problems and body condition, limb disorders and skin lesions of lactating sows housed in farrowing crates in commercial sow herds. Livest. Prod. Sci. 87, 179–187. doi: 10.1016/j.livprodsci.2003.08.005
Cao T., Li Y., Jiang L., Yuan L., Dong L., Li Y., et al. (2016). Novel biologically active series of n-acetylglucosamine derivatives for the suppressive activities on GAG release. Carbohydr. Res. 433, 73–79. doi: 10.1016/j.carres.2016.07.004
Chagnon M., D’Allaire S., Drolet R. (1991). A prospective study of sow mortality in breeding herds. Can. J. Vet. Res. 55, 180–184.
Cornou C., Vinther J., Kristensen A. R. (2008). Automatic detection of oestrus and health disorders using data from electronic sow feeders. Livest. Sci. 118, 262–271. doi: 10.1016/j.livsci.2008.02.004
Cruz R., Ramírez C., Rojas O., Casas-Mejía O., Kouri J., Vega-López M. (2016). The pig as an osteoarthritis translational research model. Int. J. Clin. Rheumatol. 11, 1–2.
Cudic M., Burstein G. D., Fields G. B., Lauer-Fields J. (2009). Analysis of flavonoid-based pharmacophores that inhibit aggrecanases (ADAMTS-4 and ADAMTS-5) and matrix metalloproteinases through the use of topologically constrained peptide substrates. Chem. Biol. Drug Des. 74, 473–482. doi: 10.1111/j.1747-0285.2009.00885.x
Dagorn J., Aumaitre A. (1979). Sow culling: Reasons for and effect on productivity. Livest. Prod. Sci. 6, 167–177.
Dewey C. E., Friendship R. M., Wilson M. R. (1992). Lameness in breeding age swine: a case study. Can. Vet. J. 33, 747–748.
Durairaj V., Hoda M., Shakya G., Babu S. P. P., Rajagopalan R. (2014). Phytochemical screening and analysis of antioxidant properties of aqueous extract of wheatgrass. Asian Pac. J. Trop. Med. 7, S398–S404. doi: 10.1016/S1995-7645(14)60265-0
Durairaj V., Shakya G., Rajagopalan R. (2015). Hepatoprotective role of wheatgrass on alcohol and ΔpUFA-induced oxidative stress in rats. J. Diet. Suppl. 12, 126–137. doi: 10.3109/19390211.2014.902002
EFSA (2007). Scientific opinion of the panel on animal health and welfare: Animal health and welfare aspects of different housing and husbandry systems for adult breeding boars, pregnant, farrowing sows and unweaned piglets. EFSA J. 5, 1–13. doi: 10.2903/j.efsa.2007.572
Fitzgerald R. F., Stalder K. J., Karriker L. A., Sadler L. J., Hill H. T., Kaisand J., et al. (2012). The effect of hoof abnormalities on sow behavior and performance. Livest. Sci. 145, 230–238. doi: 10.1016/j.livsci.2012.02.009
Fredeen H. T., Sather A. P. (1978). Joint damage in pigs reared under confinement. Can. J. Anim. Sci. 58, 759–773.
Ghanem C. I., Pérez M. J., Manautou J. E., Mottino A. D. (2016). Acetaminophen from liver to brain: New insights into drug pharmacological action and toxicity. Pharmacol. Res. 109, 119–131. doi: 10.1016/j.phrs.2016.02.020
Gjein H., Larssen R. B. (1995). The effect of claw lesions and claw infections on lameness in loose housing of pregnant sows. Acta Vet. Scand. 36, 451–459. doi: 10.1186/BF03547660
Heinonen M., Oravainen J., Orro T., Seppä-Lassila L., Ala-Kurikka E., Virolainen J., et al. (2006). Lameness and fetility of sows and gilts in radomly selected loose-housed herds in Finland. Vet. Rec. 159, 383–387. doi: 10.1136/vr.159.12.383
Heinonen M., Peltoniemi O., Valros A. (2013). Impact of lameness and claw lesions in sows on welfare, health and production. Livest. Sci. 156, 2–9. doi: 10.1016/j.livsci.2013.06.002
Im J. Y., Ki H. H., Xin M., Kwon S. U., Kim Y. H., Kim D. K., et al. (2015). Anti-obesity effect of triticum aestivum sprout extract in high-fat-diet-induced obese mice. Biosci. Biotechnol. Biochem. 79, 1133–1140. doi: 10.1080/09168451.2015.1006567
Jensen T. B., Bonde M. K., Kongsted A. G., Toft N., Sørensen J. T. (2010). The interrelationships between clinical signs and their effect on involuntary culling among pregnant sows in group-housing systems. Animal 4, 1922–1928. doi: 10.1017/S1751731110001102
Karlen G. A. M., Hemsworth P. H., Gonyou H. W., Fabrega E., David Strom A., Smits R. J. (2007). The welfare of gestating sows in conventional stalls and large groups on deep litter. Appl. Anim. Behav. Sci. 105, 87–101. doi: 10.1016/j.applanim.2006.05.014
Keita A., Pagot E., Prunier A., Guidarini C. (2010). Pre-emptive meloxicam for postoperative analgesia in piglets undergoing surgical castration. Vet. Anaesth. Analg. 37, 367–374. doi: 10.1111/j.1467-2995.2010.00546.x
Kilbride A. L., Gillman C. E., Green L. E. (2009). A cross-sectional study of the prevalence of lameness in finishing pigs, gilts and pregnant sows and associations with limb lesions and floor types on commercial farms in England. Anim. Welf. 18, 215–224.
Kirk R. K., Svensmark B., Ellegaard L. P., Jensen H. E. (2005). Locomotive disorders associated with sow mortality in Danish pig herds. J. Vet. Med. Ser. A Physiol. Pathol. Clin. Med. 52, 423–428. doi: 10.1111/j.1439-0442.2005.00747.x
Kluivers-Poodt M., Zonderland J. J., Verbraak J., Lambooij E., Hellebreker L. J. (2013). Pain behaviour after castration of piglets; effect of pain relief with lidocaine and/or meloxicam. Animal 7, 1158–1162. doi: 10.1017/S1751731113000086
Kremen T. J., Stefanovic T., Tawackoli W., Salehi K., Avalos P., Reichel D., et al. (2020). A translational porcine model for human cell–based therapies in the treatment of posttraumatic osteoarthritis after anterior cruciate ligament injury. Am. J. Sports Med. 48, 3002–3012. doi: 10.1177/0363546520952353
Kroneman A., Vellenga L., van der Wilt F. J., Vermeer H. M. (1993). Review of health problems in group-housed sows, with special emphasis on lameness. Vet. Q. 15, 26–29. doi: 10.1080/01652176.1993.9694364
Kulkarni S. D., Tilak J. C., Acharya R., Rajurkar N. S., Devasagayam T. P. A., Reddy A. V. R. (2006). Evaluation of the antioxidant activity of wheatgrass (Triticum aestivum l.) as a function of growth under different conditions. Phyther. Res. 20, 218–227. doi: 10.1002/ptr.1838
Lay D. C., Schenck E. L., Mcmunn K. A., Rosenstein D. S., Stroshine R. L., Nielsen B. D., et al. (2008). Exercising stall-housed gestating gilts: Effects on lameness, the musculo-skeletal system, production, and behavior. J. Anim. Sci. 86, 3166–3180. doi: 10.2527/jas.2008-1046
Liu Q., Zhao J., Tan R., Zhou H., Lin Z., Zheng M., et al. (2015). Parthenolide inhibits pro-inflammatory cytokine production and exhibits protective effects on progression of collagen-induced arthritis in a rat model. Scand. J. Rheumatol. 44, 182–191. doi: 10.3109/03009742.2014.938113
Madec F., Cariolet R., Dantzer R. (1986). Relevance of some behavioural criteria concerning the sow (motor activity and water intake) in intensive pig farming and veterinary practice. Ann. Rech. Vet. 17, 177–184.
Maetzel A., Li L. C., Pencharz J., Tomlinson G., Bombardier C. (2004). The economic burden associated with osteoarthritis, rheumatoid arthritis, and hypertension: A comparative study. Ann. Rheumatol. Dis. 63, 395–401. doi: 10.1136/ard.2003.006031
Murray M. M., Fleming B. C. (2012). Use of a bioactive scaffold to stimulate ACL healing also minimizes post-traumatic osteoarthritis after surgery Martha. Bone 23, 1–7. doi: 10.1177/0363546513483446.Use
Oliviero F., Scanu A., Zamudio-Cuevas Y., Punzi L., Spinella P. (2018). Anti-inflammatory effects of polyphenols in arthritis. J. Sci. Food Agric. 98, 1653–1659. doi: 10.1002/jsfa.8664
Pairis-Garcia M. D., Johnson A. K., Stalder K. J., Abell C. A., Karriker L. A., Coetzee J. F., et al. (2015). Behavioural evaluation of analgesic efficacy for pain mitigation in lame sows. Anim. Welf. 24, 93–99. doi: 10.7120/09627286.24.1.093
Parit S. B., Dawkar V. V., Tanpure R. S., Pai S. R., Chougale A. D. (2018). Nutritional quality and antioxidant activity of wheatgrass (Triticum aestivum) unwrap by proteome profiling and DPPH and FRAP assays. J. Food Sci. 83, 2127–2139. doi: 10.1111/1750-3841.14224
Pearson W., Fletcher R. S., Kott L. S., Hurtig M. B. (2010). Protection against LPS-induced cartilage inflammation and degradation provided by a biological extract of mentha spicata. BMC Complement. Altern. Med. 10, 19. doi: 10.1186/1472-6882-10-19
Pearson W., Garland A. E. N., Nixon A., Cant J. P., Hurtig M. B. (2020). Culturing articular cartilage explants in the presence of autologous adipose tissue modifies their inflammatory response to lipopolysaccharide. Mediators Inflamm. 2020, 8811001. doi: 10.1155/2020/8811001
Pearson W., Kott L. S. (2019). A biological extract of turmeric (Curcuma longa) modulates response of cartilage explants to lipopolysaccharide. BMC Complement. Altern. Med. 19, 1–8. doi: 10.1186/s12906-019-2660-z
Pearson W., Orth M. W., Karrow N. A., MacLusky N. J., Lindinger M. I. (2007). Anti-inflammatory and chondroprotective effects of nutraceuticals from sasha’s blend in a cartilage explant model of inflammation. Mol. Nutr. Food Res. 51, 1020–1030. doi: 10.1002/mnfr.200700026
Pluym L., Van Nuffel A., Dewulf J., Cools A., Vangroenweghe F., Van Hoorebeke S., et al. (2011). Prevalence and risk factors of claw lesions and lameness in pregnant sows in two types of group housing. Vet. Med. (Praha). 56, 101–109. doi: 10.17221/3159-VETMED
Pluym L. M., Van Nuffel A., Van Weyenberg S., Maes D. (2013). Prevalence of lameness and claw lesions during different stages in the reproductive cycle of sows and the impact on reproduction results. Animal 7, 1174–1181. doi: 10.1017/S1751731113000232
Reiner G., Schollasch F., Hillen S., Willems H., Piechotta M., Failing K. (2012). Effects of meloxicam and flunixin on pain, stress and discomfort in male piglets during and after surgical castration. Berl. Munch. Tierarztl. Wochenschr. 125, 305–314.
Schlichting N., Dehne T., Mans K., Endres M., Stuhlmüller B., Sittinger M., et al. (2014). Suitability of porcine chondrocyte micromass culture to model osteoarthritis in vitro. Mol. Pharm. 11, 2092–2105. doi: 10.1021/mp5000554
Seddon Y. M., Brown J. A., Seddon Y. (2013). Quantifying the prevalence of lameness and hoof lesions in Canadian nucleus herds 2013–14. Prairie Swine Centre Annual Research Report 11–12.
Sieker J. T., Proffen B. L., Waller K. A., Chin K. E., Karamchedu N. P., Akelman M. R., et al. (2018). Transcriptional profiling of articular cartilage in a porcine model of early post-traumatic osteoarthritis. J. Orthop. Res. 36, 318–329. doi: 10.1002/jor.23644
Spoolder H., Bracke M., Mueller-Graf C., Edwards S. (2017). Preparatory work for the future development of animal based measures for assessing the welfare of pigs - report 1: Preparatory work for the future development of animal based measures for assessing the welfare of sow, boar and piglet including aspects rel. EFSA Support. Publ. 8, 178E. doi: 10.2903/sp.efsa.2011.en-178
Urbonavičiute A., Samuoliene G., Brazaityte A., Duchovskis P., Ruzgas V., Žukauskas A. (2009). The effect of variety and lighting qualityon wheatgrass antioxidant properties. Zemdirbyste 96, 119–128.
van Riet M. M. J., Millet S., Aluwé M., Janssens G. P. J. (2013). Impact of nutrition on lameness and claw health in sows. Livest. Sci. 156, 24–35. doi: 10.1016/j.livsci.2013.06.005
Watzl B. (2008). Anti-inflammatory effects of plant-based foods and of their constituents. Int. J. Vitam. Nutr. Res. 78, 293–298. doi: 10.1024/0300-9831.78.6.293
Weary D. M., Huzzey J. M., Von Keyserlingk M. A. G. (2009). Board-invited review: Using behavior to predict and identify ill health in animals. J. Anim. Sci. 87, 770–777. doi: 10.2527/jas.2008-1297
Whay H. R., Main D. C. J., Green L. E., Webster A. J. F. (2003). Animal-based measures for the assessment of welfare state of diary cattle, pigs and laying hens: Consensus of expert opinion. Anim. Welf. 12, 205–217.
Zalatnai A., Lapis K., Szende B., Rásó E., Telekas A., Resetár Á., et al. (2001). Wheat germ extract inhibits experimental colon carcinogenesis in f-344 rats. Carcinogenesis 22, 1649–1652. doi: 10.1093/carcin/22.10.1649
Zhao R., Dong Z., Wei X., Gu X., Han P., Wu H., et al. (2021). Inflammatory factors are crucial for the pathogenesis of post-traumatic osteoarthritis confirmed by a novel porcine model: “Idealized“. anterior cruciate ligament reconstruction”and gait analysis. Int. Immunopharmacol. 99, 107905. doi: 10.1016/j.intimp.2021.107905
Keywords: inflammation, cartilage, swine, supplements, nutraceuticals
Citation: Cridland K, Garland A, McCrae P and Pearson W (2023) Wheatgrass extract has chondroprotective and anti-inflammatory effects on porcine cartilage. Front. Anim. Sci. 3:1063142. doi: 10.3389/fanim.2022.1063142
Received: 06 October 2022; Accepted: 19 December 2022;
Published: 27 January 2023.
Edited by:
Adem Kara, Erzurum Technical University, TürkiyeReviewed by:
Jorge U. Carmona, University of Caldas, ColombiaCopyright © 2023 Cridland, Garland, McCrae and Pearson. This is an open-access article distributed under the terms of the Creative Commons Attribution License (CC BY). The use, distribution or reproduction in other forums is permitted, provided the original author(s) and the copyright owner(s) are credited and that the original publication in this journal is cited, in accordance with accepted academic practice. No use, distribution or reproduction is permitted which does not comply with these terms.
*Correspondence: Wendy Pearson, d3BlYXJzb25AdW9ndWVscGguY2E=
Disclaimer: All claims expressed in this article are solely those of the authors and do not necessarily represent those of their affiliated organizations, or those of the publisher, the editors and the reviewers. Any product that may be evaluated in this article or claim that may be made by its manufacturer is not guaranteed or endorsed by the publisher.
Research integrity at Frontiers
Learn more about the work of our research integrity team to safeguard the quality of each article we publish.