- 1Ruminant Nutrition Unit, Department of Animal Science, University of Goettingen, Goettingen, Germany
- 2Institute of Animal Science, University of Bonn, Bonn, Germany
- 3Institute for Physiology and Cell Biology, University of Veterinary Medicine Hannover Foundation, Hanover, Germany
- 4Clinic for Zoo Animals, Exotic Pets and Wildlife, University Zurich, Zurich, Switzerland
This study investigated the impact of carbohydrate source and fluid passage rate (dilution rate) on ruminal fermentation characteristics and microbial crude protein (MCP) formation. Three commonly used feeds (barley grain [BG], beet pulp [BP], and soybean hulls [SBH]), which differ considerably in their carbohydrate composition, were incubated together with a mixture of grass hay and rapeseed meal in two identical Rusitec apparatuses (each 6 vessels). Differences in fluid passage rate were simulated by infusing artificial saliva at two different rates (1.5% [low] and 3.0% [high] of fermenter volume per h). This resulted in six treatments (tested in 3 runs): BGhigh, BGlow, BPhigh, BPlow, SBHhigh and SBHlow. The system was adapted for 7 d, followed by 4 d of sampling. Production of MCP (mg/g degraded organic matter [dOM]; estimated by 15N analysis) was greater with high dilution rate (DL; p < 0.001) and was higher for SBH compared to both BG and BP (p < 0.001). High DL reduced OM degradability (OMD) compared to low DL (p < 0.001), whereas incubation of BG resulted in higher OMD compared to SBH (p < 0.002). Acetate:propionate ratio decreased in response to high DL (p < 0.001). Total gas and methane production (both /d and /g dOM) were lower with high DL (p < 0.001). In our study increasing liquid passage rate showed the potential to increase MCP and decrease methane production simultaneously. Results encourage further studies investigating these effects on the rumen microbial population.
Introduction
Sufficient post-ruminal supply of microbial crude protein (MCP) is crucial especially for high-yielding dairy cows, which have particularly high amino acid requirements. Numerous factors such as energy, nitrogen and mineral supply, pH, and passage rate affect the synthesis of MCP in the rumen (Owens and Goetsch, 1986).
An increase in fluid passage rate commonly leads to greater formation of microbial biomass through a stimulation of fast-growing bacteria and a higher washout rate from the rumen, which is expected to result in an increase in post-ruminal MCP supply (Hungate, 1966; Van Soest, 1994). The formation of microbial cells is more efficient in response to higher passage rates of the fluid phase, since a fast-growing microbial population utilizes more of the available energy for growth than a slower-growing, which will use more energy for maintenance of cell functions (Hespell and Bryant, 1979). Furthermore, the efficiency of microbial cell formation is decreased by higher cell lysis at longer retention time (Nolan and Leng, 1983). The N-efficiency may be further improved since faster growing microorganisms have been found to contain more nitrogen per unit of microbial biomass (Hespell and Bryant, 1979).
In addition to the factors outlined above, the carbohydrate composition of feeds has also been reported to impact rumen fermentation and MCP. In vitro experiments used different carbohydrates (sugar, starch, pectin and cellulose) as energy source for rumen microbes and showed variations in MCP formation, organic matter degradability (OMD) and short chain fatty acid (SCFA) production (Hall and Herejk, 2001; Pfau and Hummel, 2019). At the time point of half-maximal gas production, Pfau and Hummel (2019) detected higher MCP yield from cellulose compared to sucrose and pectin. In addition, a higher MCP was found for starch compared to sucrose. Similarly, Hall and Herejk (2001) reported higher MCP yield for starch compared to sucrose and pectin.
The effects of ruminal fluid passage rate and carbohydrate source and their interaction are not extensively understood to date; a more detailed understanding must be regarded a prerequisite to more accurate predictions of post-ruminal MCP supply. Therefore, the objective of this study was to investigate the impact of three commonly used feeds (barley grain, beet pulp, soybean hulls), which differ considerably in their carbohydrate composition, and differences in fluid passage rate on fermentation characteristics and MCP formation.
Inducing defined changes to ruminal fluid passage rates in-vivo is challenging, since a number and complexity of factors such as level of feed intake, diet composition, saliva flow rate, rumination and mastication behavior affect ruminal passage rate. For that reason, we chose to employ the rumen simulation technique (Rusitec) in this study and induced two different fluid passage or dilution rates (DL) by infusing artificial saliva (i.e., buffer) at two different rates, while keeping the retention of feed particles and therefore the energy supply of microbial populations constant.
Materials and Methods
Experimental Design and Treatments
The experiment was conducted as completely randomized block design with two blocks (Rusitec apparatuses). The treatment factors were carbohydrate source (barley grain [BG], beet pulp [BP], and soybean hulls [SBH]) and DL (high and low), resulting in six treatments: BGhigh, BGlow, BPhigh, BPlow, SBHhigh, and SBHlow. For each fermenter and day, the incubated basal substrates consisted of a mixture of 5 g grass hay and 2 g solvent-extracted rapeseed meal (RSM; dry matter [DM] basis). The basal substrate was incubated together with either 4 g of BG, BP, or SBH (DM basis).
The hay was ground through a 10-mm screen aperture (Retsch SM 200, Retsch GmbH, Haan, Germany). After grinding, fine particles were separated and discarded by sieving the hay manually through a 1.18-mm screen aperture. The RSM, BG, BP, and SBH were ground through a 3-mm screen aperture (Retsch SM 200, Retsch GmbH, Haan, Germany). The hay and RSM were weighed into one set of nylon bags and the respective carbohydrate sources (BG, BP, or SBH) in a second set of nylon bags (all bags: 6.5 × 12 cm; 50 ± 10 μm mean pore size; R 712 Forage Bags, Ankom Technology Corp., Macedon, NY, USA). The targeted DL of the infused buffer were 1.50 and 3.00%/h (400 and 800 ml/d). Actual mean infusion rates were 1.56 and 2.90%/h (411 and 764 ml/d) for low and high DL, respectively. The experiment consisted of three separate Rusitec runs carried out under identical conditions. The system was adapted for 7 d, followed by 4 d of sampling.
Rumen Simulation Technique and Sampling
The rumen fluid and solid digesta used in the experiment were obtained from two rumen fistulated Holstein-Friesian heifers housed at the Institute for Physiology and Cell Biology, University of Veterinary Medicine Hannover (Germany). The heifers were housed and sampled in accordance with the German Animal Welfare Act. Housing and sampling protocols were reviewed and approved by the State Office for Consumer Protection and Food Safety of the state of Lower Saxony (reference number: AZ 33.4-42505-04-13A373).
Both heifers were fed 9 kg mixed grass hay, 600 g compound feed and 75 g mineral supplement per heifer and day (as fed basis). Forestomach contents were collected manually 2 h after the morning feeding from three sites (reticulum, dorsal, and ventral sac) within the reticulo-rumen of each heifer. The contents were strained through two layers of gauze and the resulting fluid was transferred into two pre-warmed plastic canisters. The canisters containing rumen fluid and rumen digesta were placed in a pre-warmed (39°C) polystyrene box for transportation to the Department of Animal Science at the University of Goettingen (ca. 1 h of drive). Two different buffer solutions were used for the high and low DL; both supplied 3.27 g/d of NaHCO3, 3.10 g/d of Na2HPO4 × 12 H2O, 0.155 g/d of NaCl, 0.188 g/d of KCl, 0.017 g/d of CaCl × 2 H2O, 0.042 g/d of MgCl × 6 H2O (McDougall, 1948). Buffer solutions had a pH of 8.25–8.27. They were modified to supply 0.210 g NH4Cl/d to increase the minimum ruminal nitrogen balance (which was present in BG) to 20 g N/kg DM. In addition, 0.002 g 15NH4Cl/d were infused as microbial marker to estimate MCP. The amount of infused salts per day and fermenter was identical for both buffer infusion rates (high and low) to ensure equal mineral supply and buffering capacity among treatments.
Two Rusitec apparatuses (Czerkawski and Breckenridge, 1977), each equipped with six identical fermenters (1.1 l volume), were used in this study. Before the onset of the experiment, each fermenter was filled with 690 ml strained rumen fluid and 410 ml pre-warmed buffer solution. To promote the inoculation with particle associated microorganisms one reusable nylon bag containing 80 g of solid rumen digesta (wet weight) was placed in each fermenter. In addition, each fermenter was equipped with one nylon bag containing hay+RSM and a second nylon bag containing one of the three carbohydrate sources. On the following morning (900 h), the nylon bag containing the solid digesta was replaced by two fresh nylon bags containing hay + RSM and the carbohydrate source, respectively. Every following day at 900 h, the pair of substrate bags which was incubated for 48 h was exchanged with a new pair of substrate bags. The bags withdrawn from each fermenter were placed in a polypropylene bag and washed manually with 60 ml of pre-warmed buffer for 1 min, to remove some of the microbial attachment. The buffer solution was added back into the fermenter. The effluent from each individual fermenter was collected in graduated flasks, which were cooled permanently/24 h using ice packs to stop further microbial activity. The quantity of effluent from each fermenter was measured once per day during the exchange of substrate bags. On sampling days (d 8 to 11), the effluent was subsampled for analysis of SCFA, ammonium-N and MCP (15N). During the daily exchange of substrate bags, the pH of the fermenter fluid was measured (pH-Meter CG 825, SCHOTT Instruments, Mainz, Germany) to ensure that pH conditions were stable and near neutral. The pH data were not recorded.
The fermentation gas was collected in gas-tight bags (5L TECOBAG, Tesseraux Spezialverpackungen GmbH, Bürstadt, Germany) attached to the flasks. The volume of total gas was measured once per day using a water displacement apparatus. The total gas volume was corrected for differences in air pressure and temperature among sampling days. Before the gas volume was measured, 15 ml gas samples (3 repetitions) were collected directly from each gas bag using a 25 ml syringe and injected into evacuated exetainer vials (Labco Ltd., Lampeter, United Kingdom) for methane analysis.
Chemical Analysis of the Substrates and Residues
All feed samples were analyzed prior to incubation according to VDLUFA (2012) for DM (method 3.1), ash (method 8.1), ether extract (method 5.1.1), CP (method 4.1.1, Kjeldahl, N × 6.25), neutral detergent fiber (aNDFom, method 6.5.1, assayed with heat-stable amylase, exclusive residual ash) and acid detergent fiber (ADFom, method 6.5.2, exclusive residual ash). Both, aNDFom and ADFom were analyzed using an Ankom 200 Fiber Analyzer (ANKOM Technology, Macedon, NY, United States). The starch content of the barley grain was analyzed polarimetrically (VDLUFA, 2012; method 7.2.1). The sugar content of the dried beet pulp was analyzed according to method 7.1.3 (VDLUFA, 2012). After removing the substrate bags from the fermenter after 48 h of incubation, the bags were freeze-dried. For each fermenter, residues were pooled separately for hay+RSM and carbohydrate source for 4 d to ensure sufficient sample for chemical analysis. Unfortunately, we lost two sampling days in the first run. Therefore, residues from that run were only pooled over two sampling days. The residues of the hay+RSM bags were ground through a 2-mm screen aperture using a laboratory mill (Polymix PX-MFC 90 D, Kinematica AG, Luzern, Switzerland) designed to handle small volumes, which matched the Retch mill grinding through a 1-mm screen aperture closely. The residues of the carbohydrate sources were manually crumbled and not ground because of the small quantities and the fact that they were already ground through a 3-mm screen prior incubation. All feed residues were analyzed for DM (VDLUFA, 2012; method 3.1), ash (VDLUFA, 2012; method 8.1) and NDFom (VDLUFA, 2012; method 6.5.3; Ankom 2000 Fiber Analyzer, ANKOM Technology Corp., Macedon, NY, USA). Analysis of NDFom was conducted to remove the feed associated microorganisms and obtain the truly degraded substrate.
The OMD and the degradability of NDFom (NDFD) were calculated as the proportion of OM or NDFom that disappeared during incubation from the amount of these components in the substrate before incubation. Results were expressed for the degradability of the hay and RSM mixture (OMDhrsm and NDFDhrsm), of the carbohydrate source (OMDcs and NDFDcs), and of all feeds combined (OMDtot and NDFDtot).
Microbial Crude Protein Synthesis
Microbial crude protein synthesis was estimated using three different methods: (1) 15N analysis (MCP1), (2) an N-balance approach comparable to that used in the modified Hohenheim gas test (MCP2; Leberl et al., 2007; Edmunds et al., 2012), and (3) solely based on the N content of the effluent after centrifugation (MCP3). The effluent samples (50 ml) for analysis were acidified with 1% (wt/vol) H2SO4 (10 mL).
For method (1), a steam distillation of 10 ml effluent was conducted (VAPODEST® 300, C. Gerhardt GmbH & Co.KG, Königswinter, Germany) with 2.5 ml 1 M NaOH solution to obtain samples for 15N analyses. The distillates were vaporized at 40°C. Aliquots of the remainder and sub-samples of the microbial pellets (see below), substrates and substrate residues after incubation were weighed into tin cups for analyzes of 15N. These were carried out at the Center for Stable Isotope Research and Analysis of the University Goettingen (KOSI). Isotope ratios of solid samples were determined by elemental analysis isotope ratio mass spectrometry.
For method (2), ammonium-N content of the effluent was analyzed; an aliquot (10 ml) of the daily effluent was subjected to steam distillation (VAPODEST® 300, C. Gerhardt GmbH & Co.KG, Königswinter, Germany) with 2.5 ml 1 molar NaOH solution and subsequent titration (TitroLine® 6000/7000 Titrator, SI Analytics GmbH, Mainz, Germany) with 0.05 molar HCl solution. The N contents of feed residues were available from 15N analyses.
For method (3), aiming to quantify MCP synthesis in the fluid phase/effluent, an aliquot of 40 ml of the daily effluent was centrifuged at 500 × g and 4°C for 10 min to separate the feed particles. The supernatant was then centrifuged again at 20,000 × g and 4°C for 30 min. The resulting supernatant was discarded and the remaining microbial pellet was washed three times with NaCl solution (9 g/l) followed by centrifugation (20,000 × g, 4°C for 30 min; Romero-Pérez et al., 2015). The resulting microbial pellet was freeze-dried and weighed.
For the three methods, MCP was calculated according to the following equations:
(1) MCP1 = [(15N intake – 15Nfeedresidues – 15Neffluent)/ enrichment of microbial N] × 6.25 (modifed after Nolan and Leng, 1983).
with 15N intake being the input from the buffer solution and the naturally occurring traces of 15N in the substrates, 15Nfeedresidues being the amount of 15N in the incubation residuals (after NDF boiling), 15Neffluent being the amount of 15N in the effluent and enrichment of microbial N being the 15N enrichment in the microbial pellet from the effluent
(2) MCP2 = (N intake – Nfeedresidues – – Neffluent) × 6.25
with N intake being the N input from buffer solution and substrates, Nfeedresidues being the N amount in incubation residues (after NDF boiling) and – Neffluent being the N covered in in the effluent (and therefore the N not used by microbes).
(3) MCP3 = Microbial mass × effluent volume × N contentmicrobialpellet ×6.25
with microbial mass being the weight of the centrifugation pellet in the centrifuged effluent volume, effluent volume being the 24 h amount of effluent and N contentmicrobialpellet being the N concentration in the centrifugation pellet.
Short Chain Fatty Acids
For SCFA analysis, 1.5 ml of the effluent was centrifuged at 16.600 × g and 4°C for 10 min. The resulting supernatant (1 ml) was acidified with 150 μl meta-phosphoric acid (25%) and 50 μl formic acid which contained 2-methylpentanoic acid (4%) and centrifuged again (16.600 × g, ambient temperature, 10 min). The 2-methylpentanoic acid was used as the internal standard. Short chain fatty acids were analyzed using a gas chromatograph (model GC-14B, Shimadzu Corporation, Kyoto, Japan) equipped with flame ionization detector. The injector temperature was 170°C, the detector temperature was 220°C. The oven temperature was 130°C. The carrier gas was hydrogen. Peak recording and area calculation were conducted by an integrator (D-2000, Merck Hitachi, Tokyo, Japan).
Methane
The methane content of the gas samples was measured using an infrared analyzer (Advanced Gasmitter®, PRONOVA Analysentechnik GmbH & Co. KG, Berlin, Germany). Since the infrared analyzer required a minimum of 10 ml of total gas for accurate measurements, 8 ml of gas was withdrawn from each of the three exetainers taken per fermenter and sampling day. The gas was pooled in one syringe and injected twice (12 ml) into the analyzer (Wild et al., 2019).
Statistical Analyses
Yield of MCP, OMD and NDFD, SCFA, total gas production and methane were analyzed using the mixed model procedure of SAS (version 9.4) with substrate, DL and substrates × DL as fixed and Rusitec run as random effect. Sampling day was considered as repeated measurement. All data are presented as least squares means. Separation of treatment means was accomplished using the Tukey-Kramer procedure. For the repeated measurements, several covariance structures were tested (unstructured, variance components banded, autoregressive, heterogeneous autoregressive, compound symmetry, and heterogeneous compound symmetry). The best-fitting covariance structure (variance components banded) was chosen based on the lowest Akaike's information and Bayesian information criteria. Statistical significance was declared at p < 0.05 and trends are discussed at 0.05 ≥ p ≤ 0.10.
Results
Substrate Composition
The treatments contained between 151 g (BPlow and BPhigh) and 177 g (SBHlow and SBHhigh) crude protein/kg DM (Table 1). The aNDFom and ADFom content of the incubated substrate mixtures were between 380 and 528 g aNDFom/kg and 200 and 331 g ADFom/kg (DM basis).
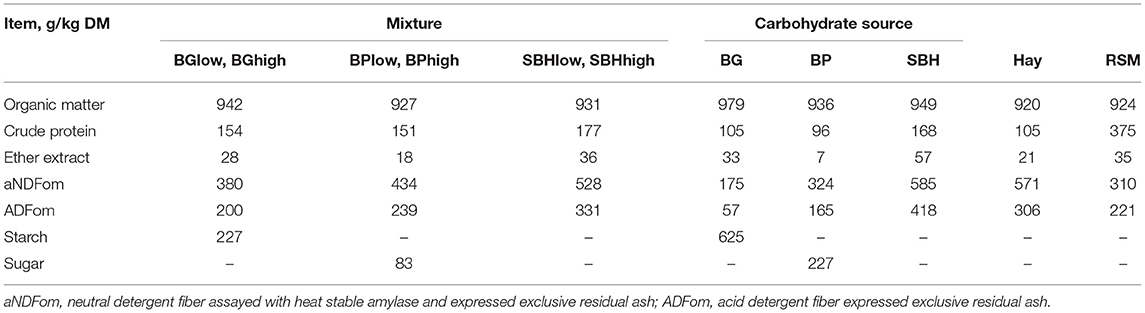
Table 1. Chemical composition of the substrates containing a mixture of 5 g grass hay, 2 g rapeseed meal (RSM) and 4 g barley grain (BG), beet pulp (BP) or soybean hulls (SBH) as carbohydrate source (on DM basis).
Organic Matter and Neutral Detergent Fiber Degradability
Overall, OMDtot was high and on a range of 68–78% (Table 2). Among the three carbohydrate sources, BG had a higher OMDtot compared to SBH, no matter which DL was applied (p = 0.002). Considering OMDcs, SBHlow (74.9%) was degraded less extensively compared to BGlow (90.0%) and BPlow (90.0%; p < 0.001). Within the high DL treatments, OMDcs from all three carbohydrate sources differed from each other (SBHhigh [68.1%] < BPhigh [81.6%] < BGhigh [88.7%]; p < 0.01). A significant interaction was present between the factorscs and DL; in fact, dilution rate had a decreasing effect on OMDcs for BP and SBH (by about 10%), but not for BG.
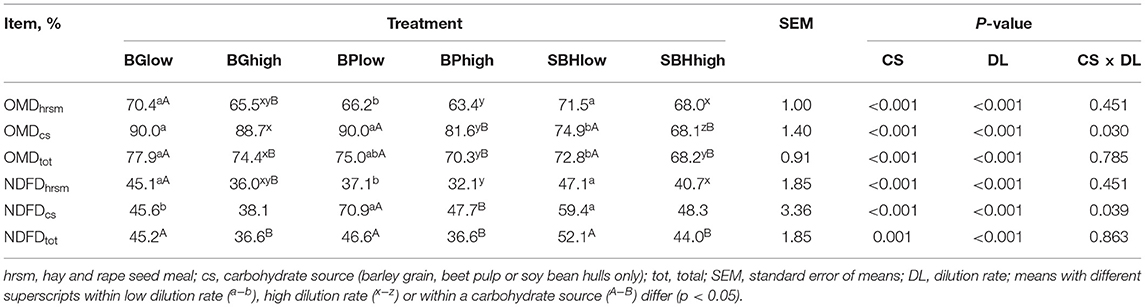
Table 2. In vitro organic matter degradability (OMD) and neutral detergent degradability (NDFD) of different carbohydrate sources incubated at high and low dilution rate for 48 h.
The differences in NDFDhrsm among treatments were the same as for OMDhrsm (Table 2). Considering only the carbohydrate source, incubation of BGlow resulted in lower NDFDcs compared to BPlow (p < 0.001) and SBHlow (p = 0.026). There was no difference in NDFDcs among BGhigh, BPhigh and SBHhigh.
Microbial Crude Protein
Due to the differences in DOM, values are given in mg/d and mg/g dOM. Total MCP1 formation per day was only affected by carbohydrate source (Table 3). Incubation of BPlow resulted in a reduction of MCP1 (mg/d) compared to SBHlow; while BPhigh reduced MCP1 (mg/d) compared to SBHhigh and BGhigh (p < 0.001). Yield of MCP1 per unit degraded organic matter (dOM) was affected by carbohydrate source (p < 0.001) and DL (p < 0.001), with a trend for an interaction (p = 0.092). Incubation of SBH resulted in higher MCP1 (mg/g dOM) compared to BG and BP no matter which DL was applied (p < 0.001). High DL resulted in higher MCP per gram dOM compared to low DL for BGhigh (p = 0.006) and SBHhigh (p = 0.020) but not for BPhigh.
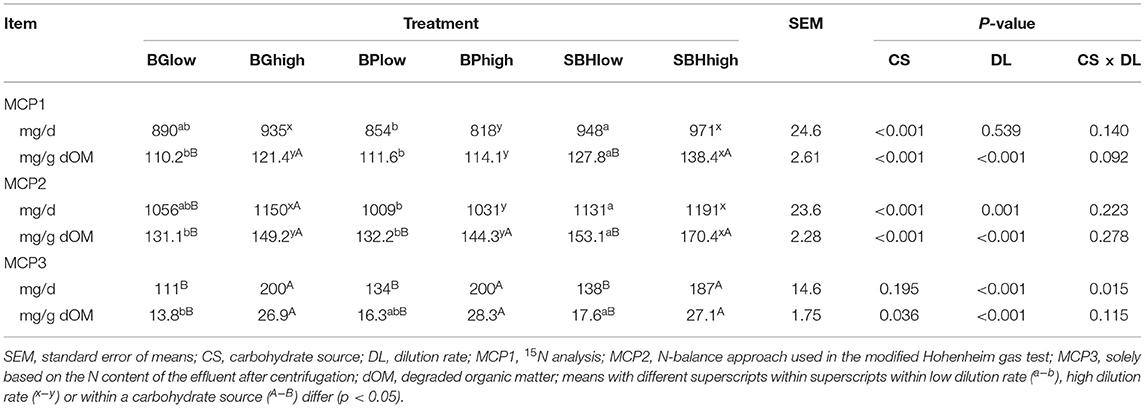
Table 3. In vitro microbial crude protein (MCP) formation of different carbohydrate sources incubated at high and low dilution rate estimated using three different methods.
Estimation of MCP2 resulted in ~15% higher values than for MCP1, but the pattern of the effects of carbohydrate source and dilution rate was basically comparable (Table 3).
The amount of MCP3 (mg/d and mg/g dOM) in the effluent was foremost affected by DL (Table 3). The MCP3 yield (mg/g dOM) from the different carbohydrate sources differed only within low DL treatments, with higher values for SBHlow (17.6 mg/g dOM) compared to BGlow (13.6 mg/g dOM; p = 0.040).
Short Chain Fatty Acids
For total SCFA, DL had an decreasing effect on mmol/d values (p < 0.001; Table 4) while CS had an effect on mmol/g dOM values (p = 0.009). There was a decreasing effect of DL on acetate production (mmol/d) (p < 0.001), which was less prominent for mmol/g dOM values. Acetate production was higher for SBH and BP compared to BG (p = 0.001) (and for high DL also for SBH compared to BP). For propionate (in mmol/d and mmol/g dOM), there was an interaction of CS and DL; in fact, the increasing effect of DL was only present in BG. Changes were also reflected in the acetate to propionate ratio, which decreased in response to high DL for all carbohydrate sources (p < 0.001), with a particularly prominent effect in BG. For butyrate (both, mmol/d and mmol/g dOM) we observed an interaction between carbohydrate source and DL (p < 0.001). Among the CS, dilution rate had a significantly decreasing effect in BG; concerning an effect of CS, butyrate was lowest in SBH.
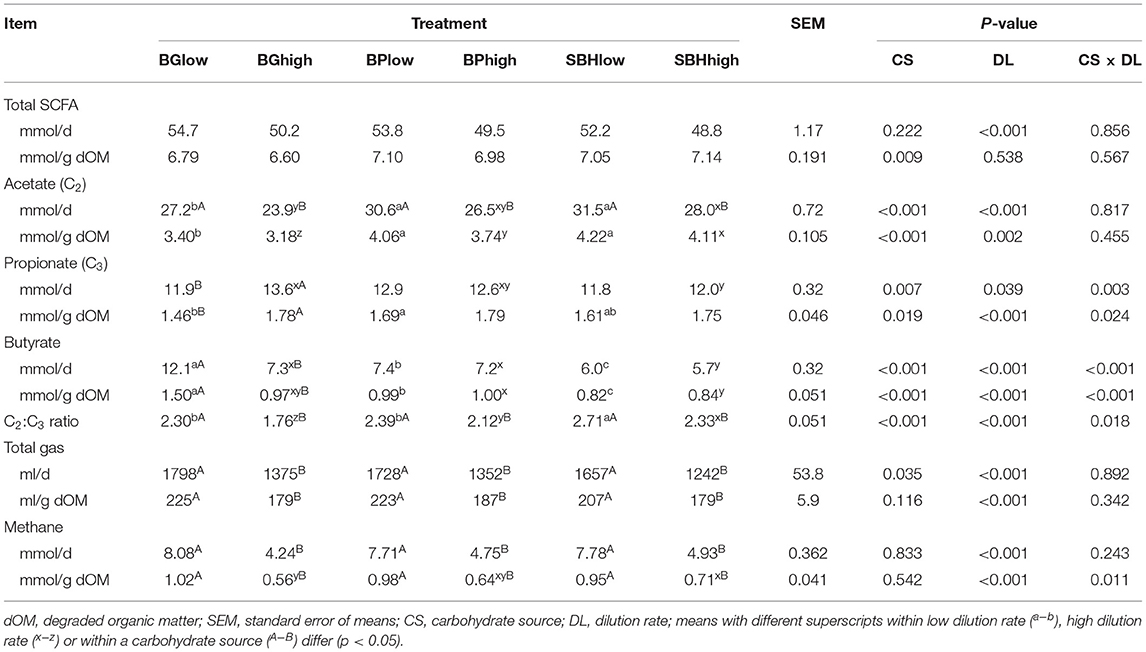
Table 4. In vitro short chain fatty acid (SCFA), methane and total gas production of different carbohydrate sources incubated at high and low dilution rate.
Total Gas and Methane
Total gas production (ml/d and ml/g dOM) was affected by DL (p < 0.001; Table 4).
Methane production (ml/d and ml/g dOM) was influenced by DL with considerably lower values at the higher dilution rate. No effect of CS was present in the ANOVA but an interaction between CS and DL for the ml/g dOM values. In fact, the influence of DL appeared more prominent in BG compared to BP and SBH.
Discussion
Influence of Carbohydrate
For rumen microbial growth, fermentable energy is generally considered a very relevant factor. In a further step, the origin of this energy may also become relevant. Since carbohydrates are the main energy source for rumen microbes, even minor differences in their efficiency for microbial growth will result in relevant changes in MCP yield. A link between type of carbohydrate and microbial growth can be explained in different ways, which are not exclusive. For example, differences in microbial yield could be primarily due to the microbial group supported by the particular carbohydrate, e.g., amylolytes vs. cellulolytes (Van Soest, 1994). Different carbohydrate sources can also provide varying amounts of energy (ATP) to microbes; acetate formation has been described to result in higher microbial ATP gain than propionate (Bergner and Hoffmann, 1996). In higher fermenting carbohydrates such as sucrose, some ATP will need to be spent on glycogen synthesis for temporary storage of oversupply, leading to lower overall efficiency (Hall and Weimer, 2016). Microbial yield can also be affected by differences in ruminal pH, which may explain beneficial effects of pectin compared to starch (Van Soest et al., 1991).
Hall and Herejk (2001) reported that the in vitro MCP yield from starch was the highest, followed by pectin, sucrose, and NDF. All values in that study are based on MCP/g OM and therefore include differences due to fermentation rate, which explains the low MCP from NDF. To some degree in contrast to this, a ranking of cellulosea, starcha, b, pectinsb, and sucrosec (different superscripts = statistical differences p < 0.05) in terms of MCP/g DM was found when MCP was measured at half-maximal gas production (time points of 17.7, 8.2, 8.1, and 5.7 h for the respective carbohydrate) (Pfau and Hummel, 2019). In the present study, using feeds rich in particular carbohydrates, MCP yields of cellulose (SBH) and starch (BG) were superior to pectin + NDF (BP) when related to DM, while cellulose (SBH) was superior to both others when related to dOM. In summary, under the given conditions (e.g., particle incubation for 48 h), this study supports the finding of a particularly efficient MCP production from cellulose, producing 10–15% more MCP from SBH compared to BP (but not BG) when expressed per unit of incubated DM. When expressed per unit of dOM, SBH produced app. 10–15% more MCP than both BG and BP. It should added that the ranking of pectins may be influenced by the high buffering capacity in in vitro fermentations, not reacting to differences of carbohydrates in this trait, which was considered a major reason for a beneficial effect of pectins on MCP (Van Soest et al., 1991).
The C2:C3 ratio was the highest for SBH, followed by BP, and BG, respectively. This supports the general view that fermentation of cell wall components favors the production of acetate, while the fermentation of grains favors propionate as fermentation product. The fact that methane yield was higher in SBH (rich in cellulose) compared to BG (rich in starch) also fits in with expectations.
Of the components used, SBH was characterized by a lower OMDcs compared to BP or BG after 48 h of incubation, which was expected due to the considerably lower fermentation rate of its major carbohydrate cellulose of ~4%/h (Hall et al., 1998). Surprisingly, some interaction between degradability of forage and protein supplements and the type of component used was present. In treatments containing BP, a lower OMDhrsm was measured.
Dilution Rate and Microbial Crude Protein
The major question of this study was the effect of DL (fluid passage) on fermentation variables, in particular MCP, when feed retention is kept constant at the same time. This approach helps to learn about variables relevant in steering fermentation, which can become important to understand the phenotype of individual animals with a particularly high microbial production. It is also relevant to understand differences between ruminant species in this regard. The mean retention time (MRT) of fluid and particles for example differs between sheep and cattle, the latter being characterized by a significantly higher ratio of MRTparticle/MRTfluid (Udén et al., 1982; Colucci et al., 1990).
Increasing the DL of the buffer resulted in higher MCP formation (MCP/g dOM) with MCP1+2, both covering total MCP. These findings are consistent with earlier studies conducted in continuous culture systems with DL between 2 and 20%/h (Isaacson et al., 1975; Meng et al., 1999; Eun et al., 2004). Efficiency of MCP production (MCP/g dOM) was greater with higher DL in these studies as well as in our experiment. A likely explanation is the shift in microbial metabolism from maintenance to expedited growth as stated by Isaacson et al. (1975). While this explanation is in line with fundamentals in microbiology (Herbert et al., 1956), other studies reported conflicting results. Using DL of 3.8 and 5.4 %/h in a Rusitec, Martínez et al. (2009) found no effect of higher DL on the total microbial N flow (MCP/d) and the daily N flow of the liquid-associated microorganisms (LAM), but the N content of LAM increased with increasing DL. Regarding the N flow of the solid-associated microorganisms (SAM) and the efficiency of microbial growth, the opposite effect to our study was observed. The SAM and the efficiency of microbial growth decreased with increasing DL. Also, the proportion of SAM of the total microbial N flow decreased with increased DL (Martínez et al., 2009).
The cellular mechanisms through which an increase in DL would enhance MCP yield are likely of some complexity. One potential factor might be the increased circulation around microbes creating a microclimate of increased nutrient supply and an efficient removal of fermentation end products and potential deterrents. Additionally, the efficiency of MCP formation is increased with increasing DL due to less microbial cell lysis and intraruminal N-recycling (Nolan and Leng, 1983).
While in general, OMD was high in this experiment (68–78%), an unexpected effect of higher DL was the lowered OMD, in particular for BP and SBH (please note that retention of solids was not different between DL and can be excluded as an explanation). It appears that the substrates rich in cell wall components were more affected. A potential explanation could be that attachment of microbes to the fibrous substrate was hindered by increased washout due to the higher DL. The fact that total SCFA production per day was lower with high DL is a logic consequence of these results. In fact, DL had no impact on total SCFA when SCFA were adjusted for differences in dOM. In contrast to our study, Martínez et al. (2009) reported higher total SCFA production and higher apparently fermented OM (estimated from SCFA production) in response to an increase in DL.
Impact of Dilution Rate on Methane Production
Besides the 10–15% increase in MCP yield, high DL also was linked to a reduction in methane production by 35% (CH4/g dOM). This was one of the most striking results of this study. While Isaacson et al. (1975) also found a decrease in methane formation (CH4/unit fermented glucose) by 20% when DL increased, others report no influence (Martínez et al., 2009) or even an increase in methane production when DL was augmented (Eun et al., 2004).
When trying to explain a link between DL and methane in this study, the first and most obvious argument must be the reduction in dOM observed with high DL. However, while this explains a part of the difference in methane formation, the effect of DL is still detectable after expressing methane per g dOM. A further explanation could be a shift in SCFA toward propionate (as observed in this study) with its known decreasing effect on methane synthesis due to less development of reduction equivalents in its metabolic pathway. An increasing effect of DL on propionate proportion was also found by Isaacson et al. (1975); Martínez et al. (2009) and Hoover et al. (1984), while Eun et al. (2004) report the opposite. Increased propionate amounts have been associated with shifts in the microbial population, like e.g., for protozoa depleted populations (Mobashar et al., 2019). As a third potential mechanism linked to methane reduction, fatty acid synthesis of growing microbes can be considered (Hackmann and Firkins, 2015; Cabezas-Garcia et al., 2017). Cell membranes contain considerable amounts of fatty acids [total fat content of bacterial cells being 8-14% (Czerkawski, 1986)], which need to be synthesized for bacterial cell proliferation and which can be considered a relevant sink for carbon and reduction equivalents. Estimations by Mills et al. (2001) assume 0.41 moles [2H] (metabolic hydrogen) being used per gram microbial biomass produced. This level already explains the differences in methane production seen between dilution rates in the present study. This is true even if a potentially larger effect by a change in composition of fatty acids in microbial membranes from unsaturated to saturated at higher [2H] pressures (Ungerfeld, 2015; Guyader et al., 2017) is not considered yet.
A strategy of high fluid turnover and constant particle retention may have the potential to maximize MCP production, while decreasing ruminal methane production to some extent. While some support for this concept has been found in this study, further research is necessary to confirm its relevance in vivo, e.g., as a potential physiological explanation for individuals emitting less methane. If this concept proves to be valid, a likely limit for a strategy of increasing fluid turnover to increase ruminal efficiency will be a concomitant reduction of the ruminal barrier function against anti-nutritive substances ranging from mycotoxins to plant toxins (Müller et al., 2011).
Considerations on Methods
While the Rusitec is obviously a long- and well-established method, methodological refinements to further evolve the fermentation system and involved measurements still appear justified. The following points may deserve to be mentioned here. The first is the approach to estimate microbial production in the system, a measure that is of high importance, but notoriously challenging, since quantifiable only indirectly via a marker. While the 15N method (MCP1) is well established, few studies appear to have used content (MCP2) as a measure of N fixation by microbes. Its principle is straightforward. The difference between daily N input [N from substrates + N from buffer] and microbially unused N [ in effluent + undegradable N in substrate] was used to estimate microbial production. This approach was adapted from a method to estimate utilizable CP (CP available at the small intestine) from N input and changes of concentration in the modified Hohenheim Gas Test (Leberl et al., 2007; Edmunds et al., 2012). Both MCP estimation methods led to concordant results, the 15N method arriving at approx. 15% lower numbers (e.g., average of 121 vs. 147 mg MCP/g dOM). In our study, growth efficiency (MCP/dOM) was close to a value of 156 mg/g dOM suggested in official German recommendations (GfE 2001), albeit the latter being based on in vivo apparent digestibility experiments (and not ruminal degradability). But our values also fit well into the range of 142 to 173 mg MCP/g fermented OM based on theoretical considerations (Czerkawski, 1986; p. 142) and a value of 148 mg/g fermented OM (SD ± 33) as estimated in a more recent meta-analysis (Cabezas-Garcia et al., 2017). Due to the lack of a direct quantitative measure of microbial growth in the Rusitec, a final evaluation of methods appears challenging; based on the largely similar reaction of MCP1 and MCP2, the closeness of MCP2 with published values and the straightforwardness of its principle, we consider this approach to be a valid alternative for MCP measurements in Rusitec.
It is well established that the major part of microbial activity and growth is directly linked to the biofilm of substrate surfaces; however, the fluid phase also contributes substantially to microbial growth. In this study, the value of MCP in the effluent represented 16% of total microbial production. This is considerably lower than values of 29-41% estimated by Guyader et al. (2017) and than ~50% of total MCP in the effluent as measured by Ribeiro et al. (2015), who used a similar method to estimate MCP in the soluble phase. While MCP3 is an interesting measure, it surely represents an additional measure to MCP1 or MCP2 only.
In contrast to the standard Rusitec procedure (Czerkawski and Breckenridge, 1977), a N source (NH4Cl) was added to the artificial saliva/buffer solution in the present study, like e.g., also done by Romero-Pérez et al. (2015). In our view, this modification represents an important modification of the Rusitec, since a continuous supply of rapidly available N resembles an important aspect of ruminant physiology, where some supply of urea N via saliva and the rumen mucosa is constantly present and contributes to level out diurnal fluctuations in non-protein N supply in response to feeding. This is of particular importance in a study investigating effects of carbohydrates on microbial growth, which, according to the principle of synchrony (Sinclair et al., 1993), will benefit from simultaneous availability of N and energy.
Conclusions
Both dilution rate and carbohydrate source were shown to be relevant factors for microbial production in the Rusitec. Among the carbohydrate sources, SBH (rich in fermentable cellulose) lead to a higher microbial production per unit of dOM than BG and BP. Higher fluid dilution rate (1.5 vs. 3.0 %/h) increased production of MCP per unit dOM by about 10%, the shift in microbial metabolism from maintenance to growth probably playing some role. Among the concomitant changes in fermentation was a substantial reduction in methane [ml/g dOM] at high DL. This can be explained by changes in SCFA production partly, but increased microbial production may also be involved. The significance of microbes as hydrogen sink deserves further attention.
Data Availability Statement
The raw data supporting the conclusions of this article will be made available by the authors, without undue reservation.
Ethics Statement
The animal study was reviewed and approved by the State Office for Consumer Protection and Food Safety of the state of Lower Saxony (reference number: AZ 33.4-42505-04-13A373).
Author Contributions
FP, JH, and MH designed the experiment. FP and MH conducted the experiment and analyzed the data. FP prepared the tables and drafted the manuscript together with JH and MH. K-HS facilitated the CH4 analysis and contributed in data interpretation and manuscript. GB gave access to the donor cows needed for the inoculum and contributed in data interpretation and manuscript. MC contributed in project concept, data interpretation, and manuscript.
Funding
We acknowledge support by the Open Access Publication Funds of the Göttingen University.
Conflict of Interest
The authors declare that the research was conducted in the absence of any commercial or financial relationships that could be construed as a potential conflict of interest.
Publisher's Note
All claims expressed in this article are solely those of the authors and do not necessarily represent those of their affiliated organizations, or those of the publisher, the editors and the reviewers. Any product that may be evaluated in this article, or claim that may be made by its manufacturer, is not guaranteed or endorsed by the publisher.
Acknowledgments
We would like to thank Anke von Gaza, Rolf Jeromin, Colette Kramer and Ingeborg Zumbrägel for sophisticated lab work.
References
Bergner, H., and Hoffmann, L. (1996). Biochemical and Energetic Processes in the Rumen of the Ruminant. Amsterdam: Harwood Academic Publishers.
Cabezas-Garcia, E. H., Krizsan, S. J., Shingfield, K. J., and Huhtanen, P. (2017). Between-cow variation in digestion and rumen fermentation variables associated with methane production. J. Dairy Sci. 100, 4409–4424. doi: 10.3168/jds.2016-12206
Colucci, P. E., Macleod, G. K., Grovum, W. L., McMillan, I., and Barney, D. J. (1990). Digesta kinetics in sheep and cattle fed diets with different forage to concentrate ratios at high and low intakes. J. Dairy Sci. 73, 2143–2156. doi: 10.3168/jds.S0022-0302(90)78895-9
Czerkawski, J. W., and Breckenridge, G. (1977). Design and development of a long-term rumen simulation technique (Rusitec). Br. J. Nutr. 38, 371–383. doi: 10.1079/BJN19770102
Edmunds, B., Südekum, K.-H., Spiekers, H., Schuster, M., and Schwarz, F. J. (2012). Estimating utilisable crude protein at the duodenum, a precursor to metabolisable protein for ruminants, from forages using a modified gas test. Anim. Feed Sci. Technol. 175, 106–113. doi: 10.1016/j.anifeedsci.2012.05.003
Eun, J.-S., Fellner, V., and Gumpertz, M. L. (2004). Methane production by mixed ruminal cultures incubated in dual-flow fermentors. J. Dairy Sci. 87, 112–121. doi: 10.3168/jds.S0022-0302(04)73148-3
Guyader, J., Ungerfeld, E. M., and Beauchemin, K. A. (2017). Redirection of metabolic hydrogen by inhibiting methanogenesis in the rumen simulation technique (RUSITEC). Front. Microbiol. 8:393. doi: 10.3389/fmicb.2017.00393
Hackmann, T. J., and Firkins, J. L. (2015). Maximizing efficiency of rumen microbial protein production. Front. Microbiol. 6:465. doi: 10.3389/fmicb.2015.00465
Hall, M. B., and Herejk, C. (2001). Differences in yields of microbial crude protein from in vitro fermentation of carbohydrates. J. Dairy Sci. 84, 2486–2493. doi: 10.3168/jds.S0022-0302(01)74699-1
Hall, M. B., Pell, A. N., and Chase, L. E. (1998). Characteristics of neutral detergent-soluble fiber fermentation by mixed ruminal microbes. Anim. Feed Sci. Technol. 70, 23–39. doi: 10.1016/S0377-8401(97)00068-0
Hall, M. B., and Weimer, P. J. (2016). Divergent utilization patterns of grass fructan, inulin, and other nonfiber carbohydrates by ruminal microbes. J. Dairy Sci. 99, 245–257. doi: 10.3168/jds.2015-10417
Herbert, D., Elsworth, R., and Telling, R. C. (1956). The continuous culture of bacteria; a theoretical and experimental study. J. Gen. Microbiol. 14, 601–622. doi: 10.1099/00221287-14-3-601
Hespell, R. B., and Bryant, M. P. (1979). Efficiency of rumen microbial growth : influence of some theoretical and experimental factors on Y ATP. J. Anim. Sci. 49, 1640–1659. doi: 10.2527/jas1979.4961640x
Hoover, W. H., Kincaid, C. R., Varga, G. A., Thayne, W. V., and Junkins Jr, L. L. (1984). Effects of solids and liquid flows on fermentation in continuous cultures. IV. pH and dilution rate. J. Anim. Sci. 58, 692–699. doi: 10.2527/jas1984.583692x
Isaacson, H. R., Hinds, F. C., Bryant, M. P., and Owens, F. N. (1975). Efficiency of energy utilization by mixed rumen bacteria in continuous culture. J. Dairy Sci. 58, 1645–1659. doi: 10.3168/jds.S0022-0302(75)84763-1
Leberl, P., Gruber, L., Steingass, H., and Schenkel, H. (2007). Valuation of the utilizable crude protein (uCP) of concentrates in vitro by the modified Hohenheimer gas test in comparison with the fractionation of protein by the Cornell-system (German). VDLUFA-Schriftenreihe 63, 429–431. doi: 10.1016/s0168-8510(97)00077-8
Martínez, M. E., Ranilla, M. J., Ramos, S., Tejido, M. L., and Carro, M. D. (2009). Effects of dilution rate and retention time of concentrate on efficiency of microbial growth, methane production, and ruminal fermentation in Rusitec fermenters. J. Dairy Sci. 92, 3930–3938. doi: 10.3168/jds.2008-1975
McDougall, E. I. (1948). Studies on ruminant saliva. 1. The composition and output of sheep's saliva. Biochem. J. 43, 99–109. doi: 10.1042/bj0430099
Meng, Q., Kerley, M. S., Ludden, P. A., and Belyea, R. L. (1999). Fermentation substrate and dilution rate interact to affect microbial growth and efficiency. J. Anim. Sci. 77, 206–214. doi: 10.2527/1999.771206x
Mills, J. A. N., Dijkstra, J., Bannink, A., Cammell, S. B., Kebreab, E., and France, J. (2001). A mechanistic model of whole-tract digestion and methanogenesis in the lactating dairy cow: model development, evaluation, and application. J. Anim. Sci. 79, 1584–1597. doi: 10.2527/2001.7961584x
Mobashar, M., Hummel, J., Blank, R., and Südekum, K.-H. (2019). Contribution of different rumen microbial groups to gas, short-chain fatty acid and ammonium production from different diets—an approach in an in vitro fermentation system. J. Anim. Physiol. Anim. Nutr. 103, 17–28. doi: 10.1111/jpn.12996
Müller, D. W. H., Caton, J., Codron, D., Schwarm, A., Lentle, R., Streich, W. J., et al. (2011). Phylogenetic constraints on digesta separation: variation in fluid throughput in the digestive tract in mammalian herbivores. Comp. Biochem. Physiol. A 160, 207–220. doi: 10.1016/j.cbpa.2011.06.004
Nolan, V. J., and Leng, A. R. (1983). “Nitrogen metabolism in the rumen and its measurement,” in Nuclear techniques for assessing and improving ruminant feeds (Vienna: International atomic energy agency), 43–65.
Owens, F. N., and Goetsch, A. L. (1986). “Digesta passage and microbial protein synthesis,” in Control of digestion and metabolism in ruminants, eds. L. P. Milligan, W. L. Grovum, and A. Dobson (Alberta (Canada): Reston Book Englewood Cliffs), 196–223.
Pfau, F., and Hummel, J. (2019). Microbial protein formation of different carbohydrates in vitro. J. Anim. Physiol. Anim. Nutr. 103, 1739–1746. doi: 10.1111/jpn.13204
Ribeiro, G. O., Gonçalves, L. C., Pereira, L. G. R., Chaves, A. V., Wang, Y., Beauchemin, K. A., et al. (2015). Effect of fibrolytic enzymes added to a Andropogon gayanus grass silage-concentrate diet on rumen fermentation in batch cultures and the artificial rumen (Rusitec). Animal 9, 1153–1162. doi: 10.1017/S1751731115000221
Romero-Pérez, A., Okine, E. K., Guan, L. L., Duval, S. M., Kindermann, M., and Beauchemin, K. A. (2015). Effects of 3-nitrooxypropanol on methane production using the rumen simulation technique (Rusitec). Anim. Feed Sci. Technol. 209, 98–109. doi: 10.1016/j.anifeedsci.2015.09.002
Sinclair, L. A., Garnsworth, P. C., Newbold, J. R., and Buttery, P. J. (1993). Effect of synchronizing the rate of dietary energy and nitrogen release on rumen fermentation and microbial protein synthesis in sheep. J. Agric. Sci. 120, 251–263. doi: 10.1017/S002185960007430X
Udén, P., Rounsaville, T. R., Wiggans, G. R., and Van Soest, P. J. (1982). The measurement of liquid and solid digesta retention in ruminants, equines and rabbits given timothy (Phleum pratense) hay. Br. J. Nutr. 48, 329–339. doi: 10.1079/BJN19820117
Ungerfeld, E. M. (2015). Shifts in metabolic hydrogen sinks in the methanogenesis-inhibited ruminal fermentation: a meta-analysis. Front. Microbiol. 6:37. doi: 10.3389/fmicb.2015.00037
Van Soest, P. J. (1994). Nutritional Ecology of the Ruminant. 2nd ed. Ithaca, NY, USA: Cornell University Press.
Van Soest, P. J., Robertson, J. B., and Lewis, B. A.(1991). Methods for dietary fiber, neutral detergent fiber, and nonstarch polysaccharides in relation to animal nutrition. J. Dairy Sci. 74, 3583–3597. doi: 10.3168/jds.S0022-0302(91)78551-2
VDLUFA (2012). VDLUFA-Methodenbuch, Bd. III. Die chemische Untersuchung von Futtermitteln. Darmstadt: VDLUFA-Verlag.
Keywords: fermentation products, microbial protein synthesis, fluid passage rate, methane, carbohydrate source
Citation: Pfau F, Hünerberg M, Südekum K-H, Breves G, Clauss M and Hummel J (2021) Effects of Dilution Rate on Fermentation Characteristics of Feeds With Different Carbohydrate Composition Incubated in the Rumen Simulation Technique (RUSITEC). Front. Anim. Sci. 2:715142. doi: 10.3389/fanim.2021.715142
Received: 26 May 2021; Accepted: 23 August 2021;
Published: 14 September 2021.
Edited by:
Ibukun Michael Ogunade, West Virginia University, United StatesReviewed by:
Atef M. Saleem, South Valley University, EgyptXiaoxia Dai, Royal Veterinary College (RVC), United Kingdom
Copyright © 2021 Pfau, Hünerberg, Südekum, Breves, Clauss and Hummel. This is an open-access article distributed under the terms of the Creative Commons Attribution License (CC BY). The use, distribution or reproduction in other forums is permitted, provided the original author(s) and the copyright owner(s) are credited and that the original publication in this journal is cited, in accordance with accepted academic practice. No use, distribution or reproduction is permitted which does not comply with these terms.
*Correspondence: Jürgen Hummel, amh1bW1lbCYjeDAwMDQwO2d3ZGcuZGU=
†These authors share senior authorship