- 1Department of Oceanography, Dalhousie University, Halifax, NS, Canada
- 2Marine Affairs Program, Dalhousie University, Halifax, NS, Canada
The health and welfare of farmed fish are highly dependent on environmental conditions. Under suboptimal conditions, the negative impact on welfare can cause changes in fish behaviour. Acoustic tags can provide high resolution and high frequency data to monitor fish positioning within the cage, which can be used to infer swimming behaviour. In this study, implanted acoustic tags were used to monitor the three-dimensional positioning of Atlantic salmon (Salmo salar) at a commercial farm in Nova Scotia, Canada. The one-month study period allowed the characterisation of background behaviour and changes in behaviour in relation to different environmental conditions, namely, water characteristics in terms of dissolved oxygen and temperature caused by the fall overturn, storm conditions, and feeding activity. The three-dimensional position of 15 fish was recorded using high temporal resolution (3 s). Fish movement was characterised by calculating four fish variables: distance from the centre of the cage [m], depth [m], velocity [ms−1], and turning angle [°]. The population swam in a counterclockwise swimming direction around 4 ± 2 m depth at an average speed of 0.61 ± 0.38 ms−1. After the fall overturn, the population moved significantly towards cage centre while decreasing velocity, and non-significant differences in depth and turning angle were observed. During feeding periods, a significant increase in depth and velocity, as well as a reduction in turning angle were observed. The storm event did not cause any significant change in the four fish variables. While some of the behavioural changes were difficult to assess with respect to causation, the high resolution, high frequency data provide unique detailed positioning information to further our understanding of the swimming behaviour of farmed fish.
Introduction
Farmed Atlantic salmon are subject to a variety of environmental stressors, including diseases, marine heatwaves, harmful algal blooms, and anoxic events. For example, the high stocking density in net pens can lead to ideal conditions for bacterial infections and diseases (Sindermann, 1984). Beyond disease and parasites, temperature and water quality can be highly variable in net pens due to vertical stratification and diffusion-advection of water masses due to tides and winds. Since fish are highly mobile, their ability to change positioning within the net pen can be critical in stress avoidance. Accordingly, fish movement can provide insights on behaviour, defined as a “representation of a reaction to the environment as a fish perceives it” (sensu Martins et al., 2011).
Water temperature has long been studied with respect to fish behaviour (Ogilvie and Anderson, 1965; Javaid and Anderson, 1967; Elliott and Elliott, 2010; Oppedal et al., 2011). For example, Atlantic salmon (Salmo salar) are known to be temperature sensitive, and when a gradient is present, e.g., a thermocline, the fish will distribute themselves according to preferred temperature ranges (Coutant, 1977; Jobling, 1981). Water temperatures below 8°C led to active behavioural thermoregulation via vertical migration (Johansson et al., 2006, 2009). The upper preferred temperature range for Atlantic salmon is 16–18°C, with avoidance behaviour occurring above the threshold (Oppedal et al., 2011). As warming climate has led to an increase in marine heatwaves, which are defined as an extended period of abnormally high sea surface temperature (Oliver et al., 2018), cultured salmon will be increasingly exposed to temperature stress. While warming waters can trigger thermal stress on farmed salmon, cold winter temperatures can also lead to significant mortality events, i.e., super chill (Hargrave et al., 2005).
Dissolved oxygen (DO) also has a major effect on the growth and performance of cultured fish. For example, at 15°C, Atlantic salmon require a dissolved oxygen level above 6.0 mgL−1 before their feed intake is reduced, while below 4.0 mgL−1 salmon are forced to switch to anaerobic metabolism (Burt et al., 2012; Remen et al., 2012; Dempster et al., 2016; Oldham et al., 2018). Some short-term effects of low DO are a decrease in swimming speed, indicative of conservation of energy, and a reduction in feeding activity (Martins et al., 2011; Oldham et al., 2018). Physiological effects of long-term low DO and elevated temperatures are decreased appetite and growth, and ultimately, increased mortality (Johansson et al., 2006; Remen et al., 2012, 2016; Gamperl et al., 2020). As with unfavourable temperatures, salmon can avoid low oxygen conditions via vertical migration within net pens, but other factors such as fish density, have been reported to override the effect of DO (Johansson et al., 2006; Stehfest et al., 2017; Oldham et al., 2018). Due to continued ocean warming, solubility of dissolved oxygen will decrease leading to more hypoxic events (Keeling et al., 2009; IPCC, 2018).
Environmental conditions that lead to behavioural changes may be relevant in the context of farmed fish welfare, to understand welfare in a “function-based” perspective changes in biological functioning in response to physiological stress are indicated by behaviour (Martins et al., 2011). Increasing use of technology on fish farms has led to a greater capability in collecting data on environmental conditions as well as fish behaviour. For example, real-time oxygen and temperature sensors are commonly deployed in salmon farming (e.g., Burke et al., 2021). Observations of fish behaviour are generally of two types: visual and acoustic. For example, feed cameras are routinely used to monitor satiation and regulate feed input. A sophisticated analysis of size, biomass, and disease status have been derived from these types of video (e.g., Williams et al., 2006; Pinkiewicz et al., 2011). Acoustic observations generally involve echo-sounding of net pen populations or individual fish via implanted tags, providing information about their positioning and movement (e.g., Cubitt et al., 2003; Cooke et al., 2005; Broell et al., 2013; Thorstad et al., 2013; Macaulay et al., 2021). Acoustic fish tracking provides an individual-based, objective sampling of position allowing for high spatial and temporal resolution (Føre et al., 2017). Although previous studies have evaluated the feasibility of acoustic telemetry to assess individual fish movement (e.g., Juell and Westerberg, 1993; Cubitt et al., 2003; Clark et al., 2010; Broell et al., 2013; Thorstad et al., 2013; Kupilik and Petersen, 2014; Føre et al., 2017) not much attention has been paid to applying acoustic telemetry in aquaculture compared to ecological studies (e.g., Childs et al., 2008; Crossin et al., 2017; Haulsee et al., 2018; Kraus et al., 2018; Rothermel et al., 2020).
Acoustic telemetry techniques have been used in aquaculture for chinook salmon (e.g., Cubitt et al., 2003), Atlantic salmon (e.g., Føre et al., 2011, 2017), Atlantic cod (e.g., Rillahan et al., 2011; Ward et al., 2012), and gilthead seabream (e.g., Muñoz et al., 2020; Palstra et al., 2021). These studies have focused on monitoring fish positioning and behaviour in commercial sea pen settings. The accuracy and reliability of a three-dimensional system was tested on chinook salmon (Cubitt et al., 2003). Further, acoustic telemetry has been used to infer behaviour, including vertical positioning and diel swimming patterns in farmed fish (e.g., Føre et al., 2011, 2017; Rillahan et al., 2011; Ward et al., 2012; Muñoz et al., 2020; Palstra et al., 2021). Individual fish tags do not capture the entire pen population, but they provide access to data on individual fish (e.g., swimming speed) that is not available when using population-level echo-sounding. Continuous monitoring of fish positioning can provide a more comprehensive view of the effects of environmental conditions on swimming behaviour and consequently, on welfare. In the present study, implanted acoustic tags were used to monitor the three-dimensional positioning of salmon for one month during the fall at a commercial farm in Nova Scotia, Canada. The main research questions of this study were:
(i) Can high resolution 3D fish location be used to infer swimming behaviour in the cage environment?
(ii) Can 3D location be used to develop metrics related to fish behaviour?
(iii) What is the relationship of behavioural indicators to environmental conditions in the net pen?
Methods
Study Site
Shelburne Bay, located in southwest Nova Scotia (Canada), is 7.4 km wide at the open mouth, narrowing landward and then branching to Shelburne Harbour over a distance of 15.5 km. The outer bay is somewhat protected by McNutt's Island. Seawater temperature has a typical north temperate range of 0–3°C in February to a maximum of 14–18°C in August (seatemperature.org). Temperature changes can be rapid due to wind-induced upwelling of cold shelf water. The small Shelburne River enters the bay at the head of the harbour, but the outer bay has salinity values (30 PSU) typical of inshore Nova Scotia.
Atlantic salmon are farmed on the northeast side of McNutts Island (Kelly Cove Salmon), a farm area of about 50,000 m2 (Figure 1A). During the study period, the site contained 19 active net pens, each of them with a diameter of ~32 m, a depth of 11 m, and an average stocking density of 12.09 kgm−3 (~20,000 individuals). The site has a feed barge on the north end, and net pens in two equal columns of 10, with pen 20 being empty (Figure 1B).
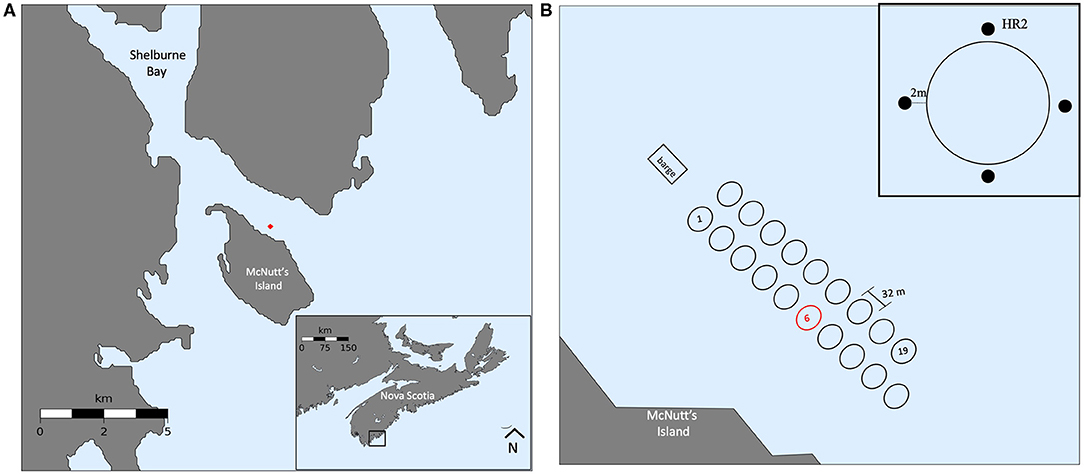
Figure 1. Study site map. (A) Regional map of Shelburne Bay with site location marked in red, and inset showing location in Nova Scotia, Canada. (B) Zoomed site map with farm orientation and net pen schematic; study net pen highlighted in red, inset showing schematic of acoustic receivers.
Acoustic Tags
Implanted acoustic tags (Vemco V9P-180, InnovaSea Systems Inc, Bedford, Nova Scotia, Canada) were 9 mm in diameter, 26 mm in length, and 4 g in air, transmitting at a frequency of 180 kHz. Tags were surgically implanted in 15 Atlantic salmon on day of year 229, and receivers ran out of battery on day of the year 361. Although 15 fish are a small percentage of the net pen population, studies show individual salmon response to environmental factors reflects group behaviour in aquaculture conditions (Oppedal et al., 2011; Stehfest et al., 2017). In addition, a higher density of tags causes interference in acoustic transmission and potential loss of data (Al-Dharrab et al., 2013).
Adult salmon (73–78 cm length and 3 kg weight) were retrieved from net pen 6, located in the centre of the farm, and implanted with acoustic tags by a Cooke Aquaculture veterinarian in compliance with the Dalhousie University Animal Care Council. Before each surgery, all surgical instruments were disinfected using a Vikron solution, and activated V9P acoustic tags were immersed in a chemical disinfectant bath of Beta iodine for a minimum of 20 min. Each fish was collected from the cage using a dip net and placed in a 40 mgL−1 bath of TMS (MS-222) 3-aminobenzoic acid ethyl. After 5–10 min, when visual signs of sedation (loss of equilibrium and no reaction to touch; Moore et al., 1990) fish were transferred to a cradle with fresh seawater bathed over the gills. A 1–1.5 cm incision was made slightly offset from the linea alba and anterior to the pelvic girdle, parallel to the midline. The tag was inserted into the coelomic cavity and closed with an absorbable 3–0 monofilament sterilised suture. An interrupted surgical knot was applied to the incision every 5 mm. Once the incision was closed, the fish was transferred to a 350-gallon recovery tank with fresh seawater constantly supplied. Recovery averaged 40 min, and release was determined when opercular movement, equilibrium, and locomotor movements were observed (Moore et al., 1990).
Each tag was set to transmit a 2D position [x, y] every 3 s for the duration of the experiment. A pressure sensor within the tags was used to measure depth (z dimension). Eight HR2 receivers (InnovaSea Systems Inc.) were deployed on the NSEW axes outside of net pen in a two-layer array and used to triangulate horizontal position (Figure 1B). The top 4 HR2s were deployed at 2 m depth in the net pen and the bottom 4 HR2s were deployed at 9 m depth. Among the 15 tagged fish, data were unusable from 5 tags due to tag malfunction or fish mortality. Three tags malfunctioned, noted by the lack of response of the pressure sensor (constant depth over time), and two tags were ascribed to dead individuals. The data from these 5 tags were not used in the analysis. Over the tagging period (132 days) mortality was 13%, which is lower than average long-term (100+ days) studies (Macaulay et al., 2021). Data from the HR2 receivers were downloaded upon retrieval but fish tags were discarded in processing following harvest.
Additional Data
Additional fish data, such as feeding time and mortality rate, were collected from farm logs. Feeding was completed twice a day, morning and evening, by an automated feed system with submerged cameras to inform when the population was satiated. Weather data, including wind speed and direction, were retrieved from the nearby Sandy Point weather station, about 7 km from the study site (Environment and Climate Change Canada). Temperature and DO were recorded every 5 min by real-time, wireless sensors (Aqua Measure, InnovaSea Systems Inc.). The data were stored onto cloud-based storage in real-time. Sensors were deployed within the study cage with 4 sensors at 2 m depth in NSEW axes, one sensor in cage centre at 4 m depth, and 4 sensors at 8 m depth in NSEW axes.
Data Processing
Raw data collected from the receivers were downloaded and sent to InnovaSea for processing. Data were returned in an [x, y, z] format with a time stamp out to 10−6 s. The positioning was then normalised by representing [0, 0, 0] as the approximate cage centre at the surface. Absolute error values for horizontal positioning is about 1 fish length, ~1 m, and absolute error for depth is about 7.5 cm. An error value was provided with each location via the horizontal error position (HPE), which is the theoretical horizontal error calculated using 3 receivers that create an array. The HPE value can range from 0 to 2, where 2 is higher error sensitivity, and 0 is less error sensitivity. In this dataset, HPE ranged from 0.06 to 0.6 with a mean of 0.31, after filtering the top 10% out.
The three-dimensional distance travelled by the fish between two consecutive time records (d12) was calculated based on [x, y, z] positioning following:
where [x1, y1, z1] to [x2, y2, z2] are the positions for the two records. Velocity between those two consecutive acoustic records (v12) was calculated as:
where d12 is the distance travelled by the fish between times t1 and t2. Distance from the centre of the net pen (dcentre) was calculated for each acoustic record following:
where x1 and y1 are the x and y positions of the first acoustic record. Turning angle from the fish's point-of-view (turn13) was calculated considering three consecutive acoustic records as:
where d12 is the distance from [x1, y1, z1] to [x2, y2, z2], d23 the distance from [x2, y2, z2] to [x3, y3, z3], and d13 the distance from [x1, y1, z1] to [x3, y3, z3]. Due to the way acoustic tags transmit data, a time philtre was applied to use only acoustic records with time stamps within 5 s of each other to ensure that only consecutive records were used in the calculations. This filtering reduced the number of detections but ensured the high quality of the data. Given the high temporal resolution of the tags, ~3 s, discarding detections is acceptable when the outcome is reducing the uncertainty of the dataset.
Statistical Analyses
Although the tagging period lasted 132 days (day of the year 229–361), the study period was reduced to one-month (day of year 274–304). This was done both to ensure that the fish had enough time to recover after the surgery and for statistical consistency (in comparing periods of similar lengths around key environmental changes). This one-month period captured two environmental changes, (1) fall overturn and disruption of water column stratification, and (2) a storm. The abrupt change in temperature and DO caused by the fall overturn allowed the study period to be split into two periods (see section Results). A storm event was identified on 298–299 when wind speeds averaged 30 ± 8 kmh−1. The fish positioning variables during the storm period were compared to the observations 2 days before the storm (296–297) and 2 days after the storm (300–301). The dataset was also divided into two categories regarding feeding activity: feeding and non-feeding times.
The four fish positioning variables, distance from centre, swimming depth, swimming speed, and turning angle, were compared (a) before and after the fall overturn event and (b) between feeding and non-feeding periods using a Wilcoxon signed-ranks test. The storm event required the use of a Friedman test to detect differences across multiple groups (before, during, after). Spectral analysis was performed to examine periodicity in depth and velocity. A Wilcoxon signed-ranks test was used to compare periodicity of day against night to test the significance of those cycles. All data processing was completed using Python Spyder 3.7 (Python Software Foundation, https://www.python.org/).
Results
Fish Survival
Other than survivorship, the effects of surgery and the tags on fish behaviour are difficult to assess. Although there is inter-individual variation in the different measures of fish position/swimming, the spread is not large, and no fish stand out as being obviously incapacitated. In addition, it is expected that poorly recovered fish would not persist in the population. Therefore, it could be concluded that the 10 remaining fish recovered and displayed normal swimming responses.
Background Swimming Movement
Four variables were examined in individual fish including (a) distance from centre of the net pen [m], (b) swimming depth [m], (c) swimming velocity [ms−1], and (d) turning angle [°] (Equations 1–4; Figure 2). The tagged population averaged a distance of 9.1 ± 3.5 m from the cage centre during the study period, with a maximum of 20.6 m and a minimum of <1 m (Figure 2A). Based on cage diameter, the maximum distance from the centre should be ~16 m, but the net is flexible, and some distention due to flow is expected. Fish 5 and 9 are highlighted in Figure 2 to focus on individual responses. Fish 5 is the most extreme case for three of the four fish variables. Fish 9 is a representation of the majority of the population and therefore was chosen to show a more detailed view of an “average” fish. Heatmaps of horizontal fish position based on % of observations in a given location (Figure 3) show examples of the two emphasised individuals. In both cases, the maximum density of observations is <0.5%, indicating that the fish evenly occupied most of the net pen. Fish 5 showed the highest density of observations at distances of about 10–15 m from the net pen centre (Figure 3A). Fish 9 occupied more centred positions than Fish 5, with regions of higher relative density at distances of about 5–10 m from the centre (Figure 3B). The directionality of the swimming vectors demonstrates that both fish 5 and 9 fish swam in a counter-clockwise direction (Figures 3C,D).
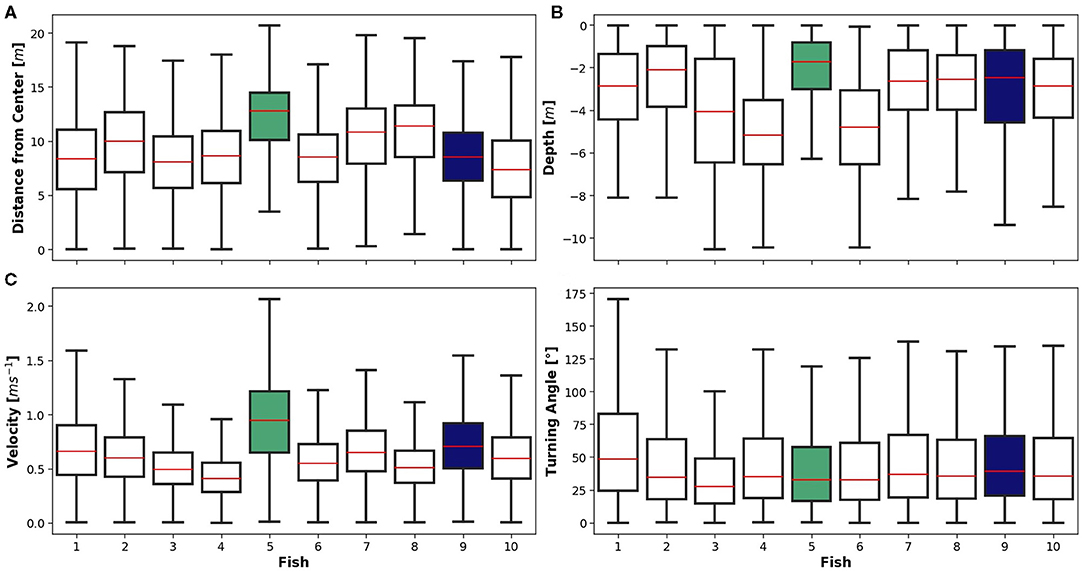
Figure 2. Boxplots of four behavioural variables for individual fish during the study period where the red line is median, box incorporates 25–75th percentile, and error bars mark maxima and minima. Green shaded box represents fish 5 and blue shaded represents fish 9. (A) Distance from centre [m]. (B) Depth [m]. (C) Velocity [ms−1]. (D) Turning Angle [°].
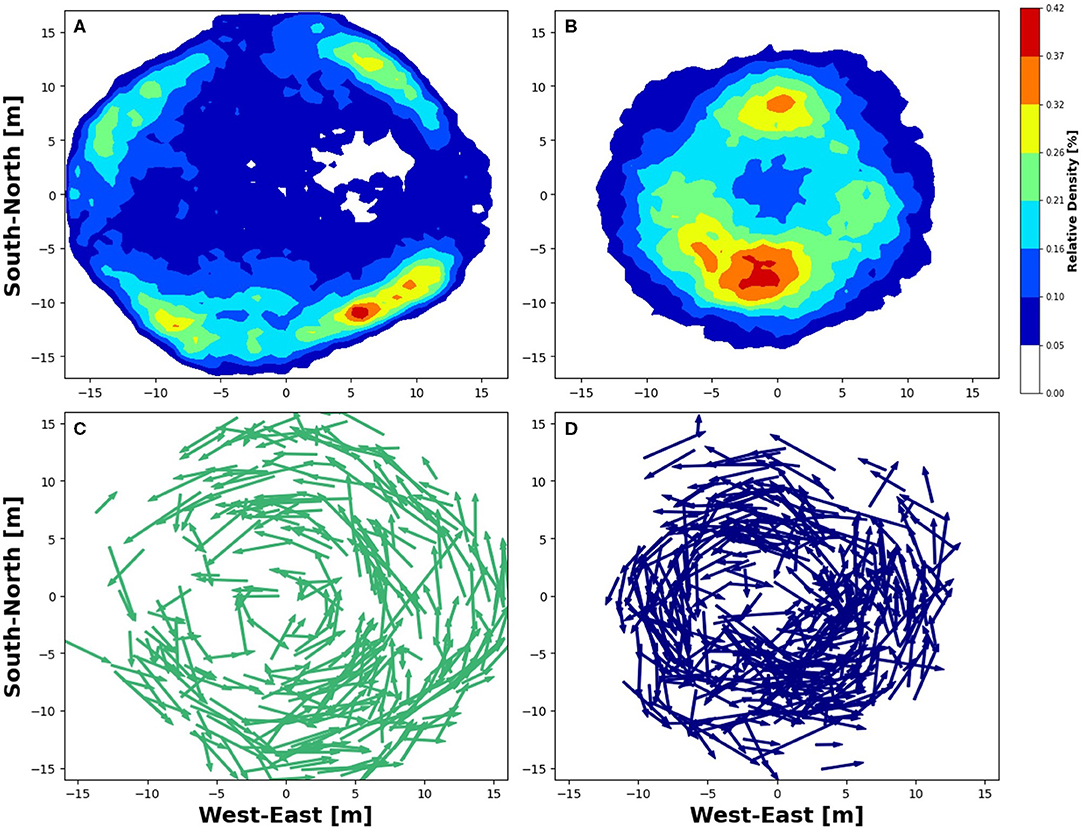
Figure 3. Horizontal plots of two tagged fish. (A) Heatmap of fish 5 during October where blue shows low relative density [% occupancy] and warmer colours represent higher relative density. (B) Heatmap of fish 9 during October. (C) Vector plot of fish 5 during a 12-h period in October. (D) Vector plot of fish 9 during a 6-h period in October.
The tagged population averaged 3.6 ± 2.2 m swimming depth below the water surface with a maximum of 10 m and minimum at the water surface (Figure 2B). Jumping out of the water was not recorded since the tags only operate in water. A time series of Fish 5 and 9 showed a clear periodicity related to time of day with greater depths during the day, and shallower depths at night (Figure 4A). Despite the temporal similarity between the vertical position of these two fish, Fish 9 migrated distinctly deeper in the net pen than Fish 5. Spectral density analysis (1-h rolling mean) of each individual identified a strong peak at 24 h for 70% of the tagged fish, with the other 30% having two peaks on either side of the 24-h mark, ranging between 22.5 and 25.5 h (Figure 4B). Fish 9 also showed a slight peak at 8 h. In general, the tagged population depth was deeper during daylight, 2.95 ± 0.03 m, than at night, 2.05 ± 0.07 m (Wilcoxon signed-ranks, p = 0.00).
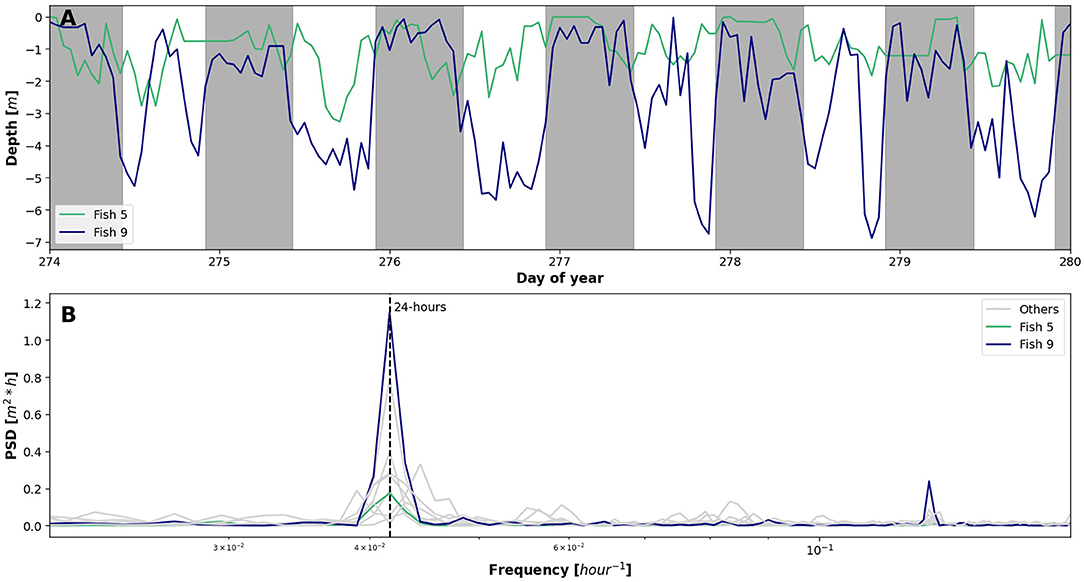
Figure 4. Spectral analysis of tagged fish depth [m]. (A) One-week one hour rolling mean time series of two individuals: Fish 5 (green) and Fish 9 (blue) with night highlighted in grey. (B) Power spectral density plot using 1 h rolling mean of all individuals highlighting fish 5 and 9 where frequency is in hours.
Swimming velocity [ms−1] averaged 0.61 ± 0.38 ms−1 with a maximum of 2.0 ms−1 and a minimum remaining almost still (Figure 2C). A time series of velocity for Fish 9 showed the highest velocity occurred during evening, while for Fish 5 no obvious pattern was observed (Figure 5A). Similar to the results obtained for depth, spectral density analysis (1-h rolling mean) of velocity identified a strong peak at 24 h for 50% of the individuals (Figure 5B). Swimming speed among the tagged population was significantly higher during daylight than at night (Wilcoxon signed-ranks, p = 0.00). The other half of individuals show peaks on either side of 24 h ranging between 23 and 25.75 h. Turning angle [°] was used to measure the change in direction of the fish trajectory (Figure 2D). The population averaged 46.82 ± 38.2° deviation from their trajectory with a maximum of 179.9°, indicating a U-turn, and a minimum of 0°, indicating no change in swimming direction. Mean turning angle was highly consistent among the tagged fish.
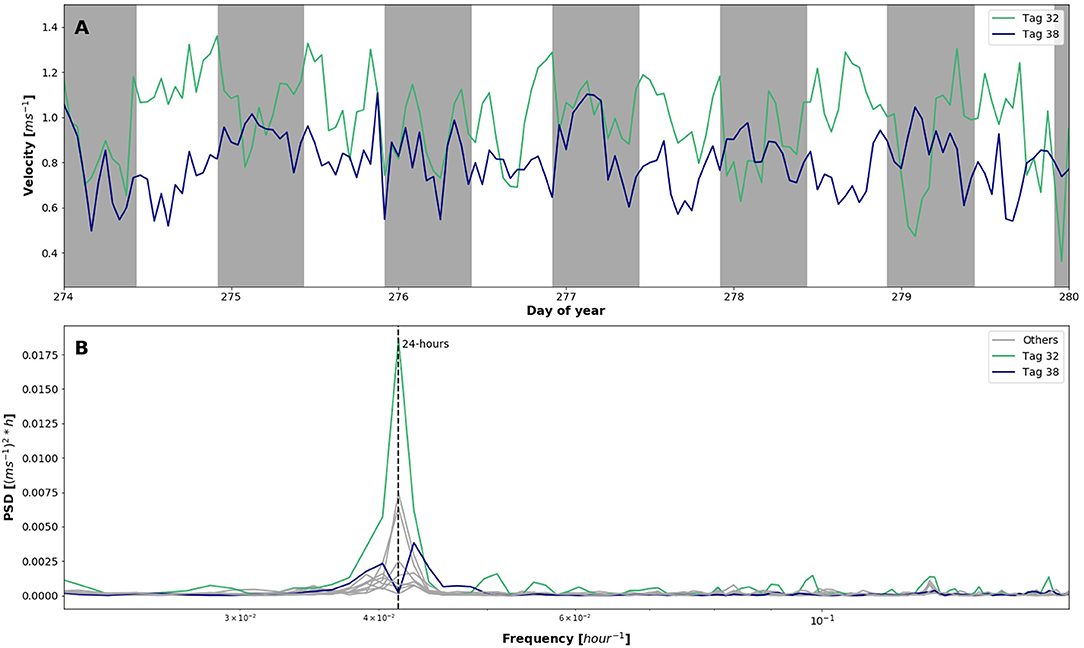
Figure 5. Spectral analysis of tagged fish velocity [ms−1]. (A) One-week one hour rolling mean time series of two individuals: Fish 5 (green) and Fish 9 (blue) with night highlighted in grey. (B) Power spectral density plot using 1 h rolling mean of all individuals highlighting fish 5 and 9 where frequency is in hours.
Fish Movement in Relation to External Variables
The rapid change in temperature and DO associated with the fall overturn and breakdown of the seasonal thermocline allowed division of the dataset into two well-defined periods with distinct and relatively stable conditions in terms of temperature and DO. Days 274–289 are defined as “Before” when temperatures ranged from 12 to 17°C and DO from 5.0 to 7.2 mgL−1, and days 290–304 are defined as “After”, with temperature ranging from 6.5 to 10°C and DO from 6.8 to 8.3 mgL−1 (Figure 6). Comparison of the four fish positional variables between these two periods showed that for distance from centre [m], 80% of tagged individuals moved slightly towards the inner part of the net pen after the fall overturn, with a relative change between 0.8 and 15% of their previous position (Figure 7A). In contrast, Fish 1 and 10 moved towards the edge of the net pen by a relative change of 0.33 and 4.55%, respectively. As a population, the fish moved towards the centre with a significant decrease from a mean of 9.4 ± 1.4 m (Before) to 8.7 ± 1.3 m (After; p < 0.05, Table 1). Examination of depth distribution before and after the overturn revealed greater inter-individual variability than distance from centre (Figure 7B), which resulted in non-significant differences in depth (p = 0.284, Table 1). Swimming velocity showed a pattern similar to horizontal distance, with 90% of individuals decreasing velocity by 8.3–33.8% after the fall overturn (Figure 7C). Before the change in DO and temperature, the mean velocity of the tagged population was 0.69 ± 0.15 ms−1, which decreased significantly to 0.57 ± 0.11 ms−1 after the fall overturn (p < 0.01, Table 1). Fish 8 was the only individual in which a 7% increase in velocity occurred after the overturn. High inter-individual variability in turning angle (Figure 7D) resulted in a non-significant difference at the tagged population level between periods (p = 0.092, Table 1).
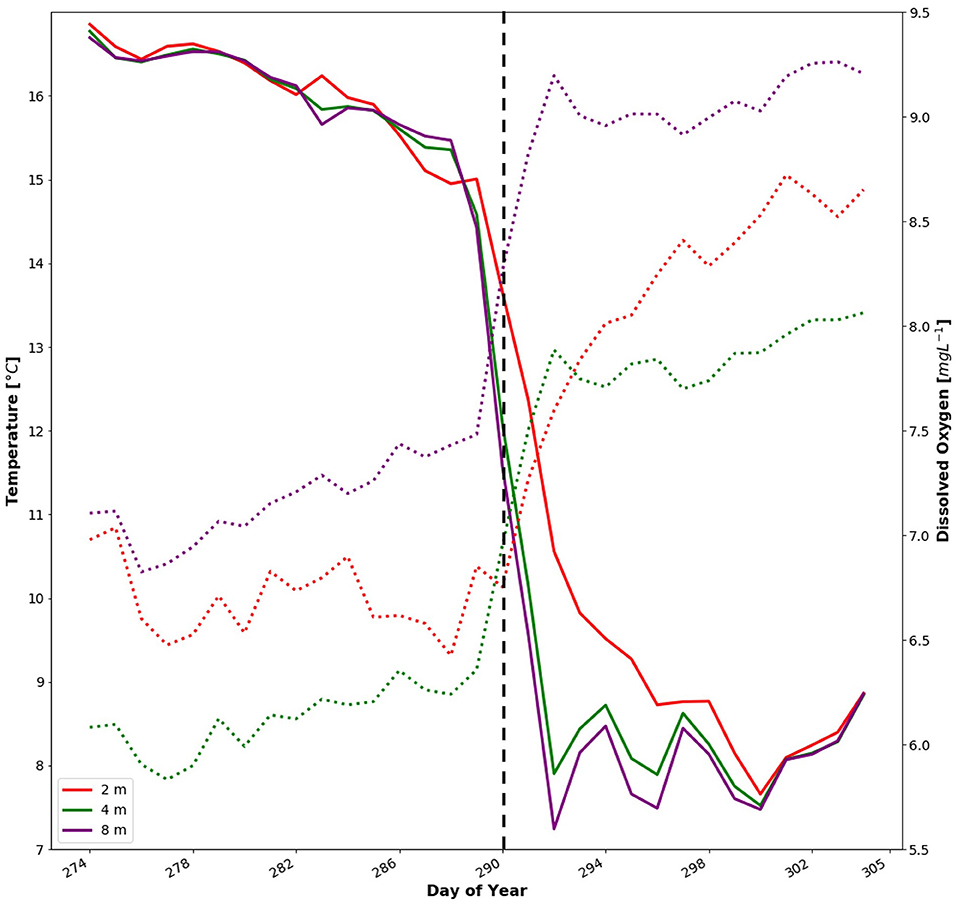
Figure 6. Daily average of temperature [°C] (solid lines) and dissolved oxygen [mgL−1] (dotted lines) measured in study cage at 2 m (red), 4 m (green), and 8 m (purple) depth (adapted from Burke et al., 2021). Vertical black line denotes split between before and after the fall overturn.
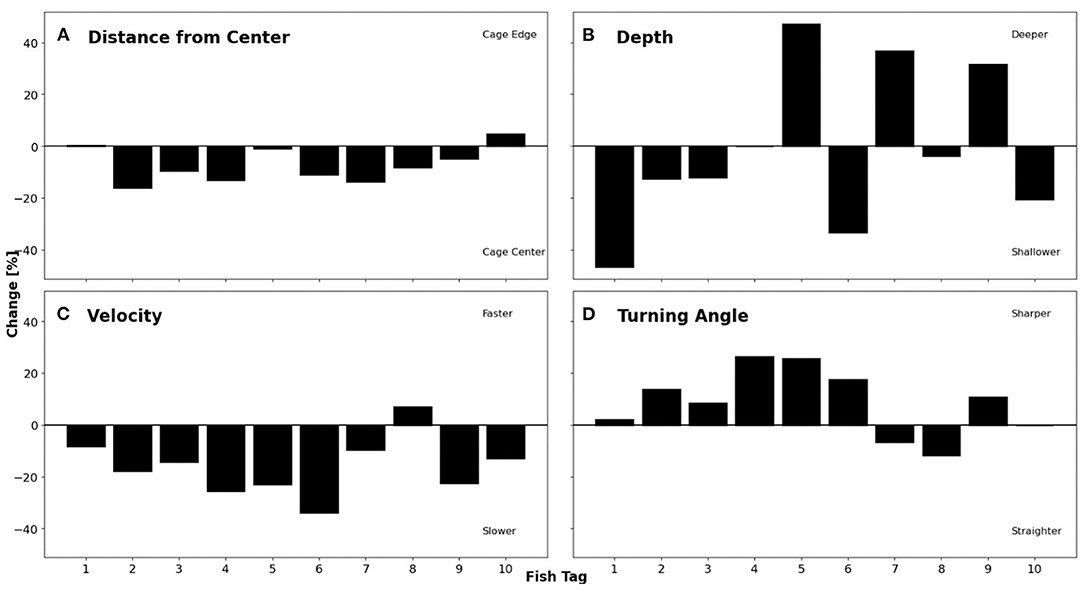
Figure 7. Percent change of individual fish comparing before the fall overturn (1–16 October) temperature and DO change to after (19–31 October). (A) Change in distance from centre where a positive change represents a shift towards cage edge. (B) Change in depth where a positive change signifies movement towards the bottom. (C) Change in velocity where an increase in velocity is represented by a positive change. (D) Change in turning angle with a positive change representing a sharper turning angle.
The same four fish variables were used to compare the effect of feeding (Figure 8). Feeding was defined as any time period that feed was distributed within the net pen, while before feeding is any period in which fish were not being fed. No statistical differences were observed in terms of distance from the centre (Figure 8A) before and during feeding periods (p = 0.240, Table 1). Depth increased for 80% of the individuals by 1.7–40.4% during feeding (Figure 8B). In contrast, Fish 4 and 10 moved towards the surface by 1.8 and 8.7%, respectively. Depth for the tagged population averaged 2.9 ± 1.2 m from the surface for non-feeding and 3.4 ± 1.1 m for feeding periods, leading to a significant difference (p < 0.05, Table 1). All individuals increased in velocity between 1.2 and 19.7% during feeding (Figure 8C). As a population, the mean velocity before feeding periods was 0.64 ± 0.13 ms−1, which increased significantly up to 0.72 ± 0.18 ms−1 during feeding (p < 0.01, Table 1). All individuals showed smaller turning angles, between 2.7 and 36.1% less, while being fed (Figure 8D). Population turning angle while feeding averaged 41.0 ± 5.6°, significantly different from non-feeding, which averaged 46.8 ± 5.0° (p < 0.01, Table 1).
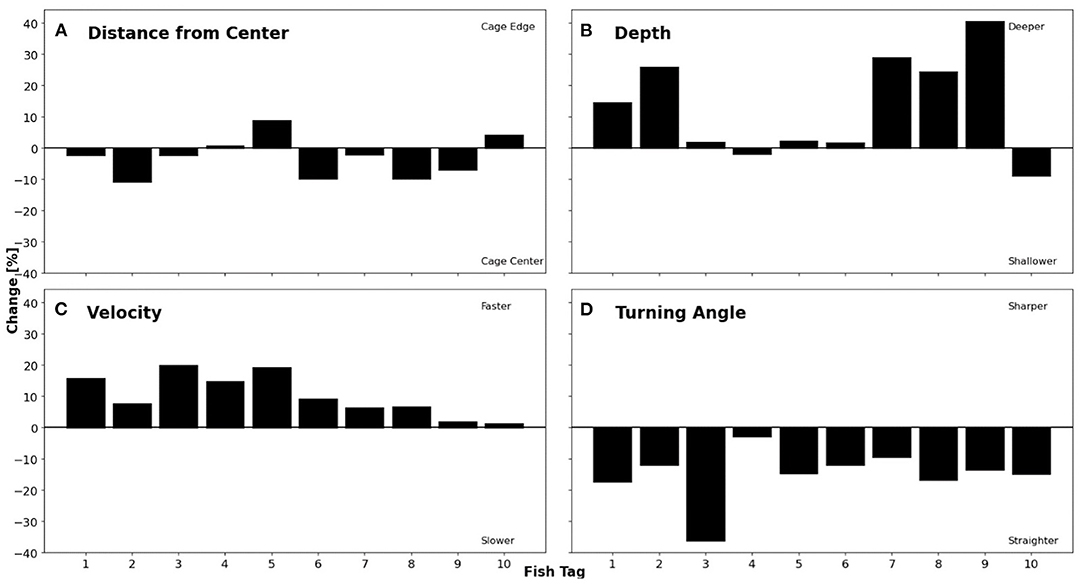
Figure 8. Percent change of individual fish comparing feeding times to non-feeding times. (A) Change in distance from centre where a positive change represents a shift towards cage edge. (B) Change in depth where a positive change signifies movement towards the bottom of the net pen. (C) Change in velocity where an increase in velocity is represented by a positive change. (D) Change in turning angle with a negative change characterising a straighter turning angle.
A storm event characterised by 30 ± 8 kmh−1 winds was observed after the fall overturn during days 298 and 299, which allowed for a comparison before, during, and after the storm. Before the storm event, temperature ranged from 7.5 to 8.7°C and DO from 7.7 to 9.0 mgL−1; while after the storm, temperature ranged from 7.4 to 8.0°C and DO from 7.8 to 9.2 mgL−1. No significant differences were observed for any fish variable when comparing before, during, and after the storm (Table 1). Averaging across these three periods, distance from centre was 8.9 ± 1.3 m, depth 2.9 ± 1.2 m, velocity 0.55 ± 0.12 ms−1, and turning angle 53.1 ± 9.2°.
Discussion
The combination of receiver array and acoustic tags has offered a 3-dimensional view of Atlantic salmon swimming behaviour and positioning in a commercial net pen. This detailed information has provided further understanding of fish behavioural response to different environmental stimuli. Previous studies have described vertical movement (Fernö et al., 1995; Juell and Fosseidengen, 2004; Johansson et al., 2006, 2009; Føre et al., 2017) and behaviour (Martins et al., 2011; Oppedal et al., 2011; Castanheira et al., 2017) of cultured salmon, however measuring movement in 3-dimensions is less common (Cubitt et al., 2003).
Swimming Movement
There was a distinguishable circular, counterclockwise swimming pattern within the group of Atlantic salmon. The circular swimming pattern is affected by water currents, but directionality may change among net pens or farms (Juell, 1995; Johansson et al., 2014). A shoal, or a group of fish remaining together, adopts a polarised swimming pattern to minimise the possibility of collisions, and deviations from this pattern by individuals can affect the group reaction (Martins et al., 2011). Within a shoal, there are “traffic rules” used by all individuals, and consequently, the analysis of a few individuals can provide insight into group behaviour. The counterclockwise swimming pattern observed in this study is an example of schooling behaviour as in the above definition, suggesting that group behaviour can be inferred from individual tags.
There was a distinct diel rhythm in depth with swimming nearer the surface at night, compared to the day. Diel rhythms in depth in salmon farming have been linked to light intensity, feed anticipation, and the apparent threat of predation (Oppedal et al., 2001, 2007; Juell and Fosseidengen, 2004; Johansson et al., 2006, 2009). The diel rhythm observed in this study ranged ~0–6 m, corresponding to the upper half of the net pen. This observation matches previous studies where populations remain in the upper half of net pens, regardless of site depth (Fernö et al., 1995; Juell and Fosseidengen, 2004; Johansson et al., 2006, 2009; Føre et al., 2017). Net pens in Norway are up to 50 m deep and located in fjords, where in Atlantic Canada net pens are on average about 6–15 m deep. The avoidance of net pen bottom observed could characterise anti-predator evasion since large piscivorous fish have been detected under net pens (Dempster et al., 2009), or a natural biological response (Tanaka et al., 2005).
A diel rhythm in swimming speed, with higher velocities during the day was also observed in this study. Previous studies have found swimming speeds to range between 0 and 2 ms−1, similar to the values observed in this study, with the highest speeds during the day (Juell and Westerberg, 1993; Andrew et al., 2002; Føre et al., 2011, 2018b). Swimming speeds are customarily faster during the day than at night due to light availability (Oppedal et al., 2001) because Atlantic salmon rely heavily on vision while swimming (Oppedal et al., 2011). The tagged salmon in this study thus followed the expected pattern previously described in the literature with greater depths and higher velocity during daylight hours and lower velocity and lesser depths during nighttime (Fernö et al., 1995; Andrew et al., 2002; Johansson et al., 2006; Føre et al., 2011, 2018b; Oppedal et al., 2011).
Fish Movement in Relation to External Variables
Following the rapid temperature and DO change halfway through the study period due to the fall turnover, individuals showed a shift towards the cage centre with a decrease in velocity but no significant change in depth distribution. Changes in proximity towards the centre, although significant, were small and on the order of one fish length. It is difficult to assign causality to this minor shift, but associated results for swimming speed are consistent with an expected decrease caused by a drop in temperature (Hvas et al., 2017).
During feeding, there was an increase in depth while individuals increased speed and decreased turning angle. During daylight hours, fish tend to swim deeper in net pens but ascend towards the surface when motivated by feed (Fernö et al., 1995; Oppedal et al., 2001), contrary to this study where the opposite occurred. It is likely more crowded at the surface during feeding, an observation apparent even to a casual observer. An increase of speed during feeding is indicative of foraging behaviour to capture food pellets nearby (Martins et al., 2011). A decrease in turning angle is suggestive of straighter paths towards food pellets. Although turning angle was calculated from adjacent swimming vectors, turning is a continuous process in part dictated by cage diameter and thus the tightness of circular swimming.
During a storm with wind speeds between 35 and 45 kmh−1, there were no significant movements horizontally or vertically in the tagged group, suggesting that fish behaviour was not affected by the storm. This does not rule out effects on fish at higher wind speeds. The movement of net pens during storms has become a concern for farmers due to the possibility of increased net movement causing damage to fish as well as generally leading to increased stress. Although no responses on these four variables were significant from this storm, it does not neglect other stressors, e.g., fin and scale damage, that could cause physical damage not seen with movement data.
Conclusions
This study used detailed positioning data to catalogue behavioural responses using fish position to calculate swimming speed and vertical/horizontal location. There are a variety of external variables available to evaluate as determinants of behaviour including oxygen, temperature, and wind. In addition, husbandry variables including feeding provide further basis for comparison. Nonetheless, the net pen environment is confined and the scope for behavioural responses is limited. Some of the changes in defined behaviours were subtle and difficult to assess with respect to causality. Previous studies have confirmed the use of acoustic tags in field experiments at commercial fish farms and have found common swimming patterns (e.g., circular swimming pattern and diel vertical movement) (e.g., Føre et al., 2017; Muñoz et al., 2020; Macaulay et al., 2021). This study is one of the few employing tags to resolve 3D positioning, but also able to incorporate high frequency data on both horizontal and vertical movement. As fish farming continues to embrace precision aquaculture (Føre et al., 2018a; O'Donncha and Grant, 2019), further delineation of both fish behaviour and its environmental forcing will be possible, as well as input into husbandry management.
High frequency acoustic tags provide a detailed temporal view of fish positioning within aquaculture net pens; however, some data loss is possible due to the signal interference caused by the high density of fish in the cages (Al-Dharrab et al., 2013). Although only 3 receivers are needed to triangulate fish positioning, a total of 8 receivers were placed in this study to minimise data loss. One of the assumptions to triangulate positioning, and minimise error, is to have stationary receivers. In this study, the receivers were attached to the gear that keeps the cage in place, which was assumed to be the most stationary option. Although this gear is not completely stationary, the short-term drift of the receivers should be negligible compared to fish movement during the 2 or 3 consecutive datapoints (~6 sec) used for the mathematical calculation of positioning, velocity, and turning angle. The use of a reference tag on the ocean bottom could be used to quantify this potential error in future studies.
The study intended to use position-based behaviours to examine the response to apparent stress by conditions such as storms. However, stress is manifest by morphological and physiological responses assessed by operational welfare indicators (OWI), including fin and scale health as well as other metrics of condition (Martins et al., 2011; Oppedal et al., 2011; Noble et al., 2018). OWIs would provide complementary information that if compiled appropriately could be used to understand the welfare of farmed Atlantic salmon (Stien et al., 2013). Positioning data provides the function-based information needed to understand how changes in conditions affect changes in behaviour and ultimately welfare. Although this study examined changes in behaviour with respect to documented events such as storms and the fall overturn, these events are not necessarily judged as extreme. In comparison, OWIs which depend on mostly negative morphological changes are reflective of rather severe impacts on fish condition. This study has provided a highly resolved data set on farmed salmon behaviour that creates a baseline for responses to the ambient environment. The study posited that these data would provide a sensitive response to external conditions, however more extreme events such as severe storms might yield a more obvious behavioural response. This approach results in a quantitative set of metrics with which to approach fish behaviour. As oceanic conditions such as storminess and hypoxia become more common in a changing climate, it is anticipated a wider range of response in fish swimming and positioning behaviour.
Climate change is leading to increased sea surface temperatures, decrease oxygen, and increased frequency and intensity of storms (Handisyde et al., 2006; Keeling et al., 2009; Johannesen et al., 2020). An understanding of fish behavioural response to these variables provides further management options including cage and mooring design, feeding regimens, oxygen supplementation, and site selection. Research progress in an increasing sensor and data-rich farm environment will inevitably lead to better fish welfare and more sustainable aquaculture.
Data Availability Statement
The datasets presented in this article are not readily available because the raw data are proprietary information and cannot be released. Requests to access the datasets should be directed to Caitlin L. Stockwell, cstockwell@dal.ca.
Ethics Statement
The animal study was reviewed and approved by Dalhousie University Animal Care Council.
Author Contributions
CS designed and executed field experiment, performed data analysis, and wrote first draft of manuscript. RF and JG secured funding for research and provided guidance for data analysis. All authors edited, read, and approved manuscript.
Funding
This research was funded by the Ocean Frontier Institute (Module K: Novel sensors for fish health and welfare), and NSERC Collaborative Research and Development Grant (NSERC-Cooke Aquaculture CRD 511841-17) and is also a contribution to the EU Horizon 2020 GAIN (G.A. 773330) project.
Conflict of Interest
The authors declare that the research was conducted in the absence of any commercial or financial relationships that could be construed as a potential conflict of interest.
Acknowledgments
The authors are thankful to Cooke Aquaculture, especially Eoin MacInnis for performing tag implantation and the Shelburne site crew for helping deploy and retrieve the system. We would also like to thank InnovaSea Systems, Inc., especially Dale Webber, for their guidance on experimental design and data interpretation.
References
Al-Dharrab, S., Uysal, M., and Duman, T. M. (2013). Cooperative underwater acoustic communications. IEEE Commun. Mag. 51, 146–153. doi: 10.1109/MCOM.2013.6553691
Andrew, J. E., Noble, C., Kadri, S., Jewell, H., and Huntingford, F. A. (2002). The effect of demand feeding on swimming speed and feeding responses in Atlantic salmon Salmo salar L., gilthead sea bream Sparus aurata L. and European sea bass Dicentrarchus labrax L. in sea cages. Aquaculture Res. 33, 501–507. doi: 10.1046/j.1365-2109.2002.00740.x
Broell, F., Noda, T., Wright, S., Domenici, P., Steffensen, J. F., Auclair, J. P., et al. (2013). Accelerometer tags: detecting and identifying activities in fish and the effect of sampling frequency. J. Exp. Biol. 216, 1255–1264. doi: 10.1242/jeb.088336
Burke, M., Grant, J., Filgueira, R., and Stone, T. (2021). Oceanographic processes control dissolved oxygen variability at a commercial Atlantic salmon farm: application of a real-time sensor network. Aquaculture 533:736143. doi: 10.1016/j.aquaculture.2020.736143
Burt, K., Hamoutene, D., Mabrouk, G., Lang, C., Puestow, T., Drover, D., et al. (2012). Environmental conditions and occurrence of hypoxia within production cages of Atlantic salmon on the south coast of Newfoundland. Aquaculture Res. 43, 607–620. doi: 10.1111/j.1365-2109.2011.02867.x
Castanheira, M. F., Conceição, L. E., Millot, S., Rey, S., Bégout, M. L., Damsgård, B., et al. (2017). Coping styles in farmed fish: consequences for aquaculture. Rev. Aquaculture. 9, 23–41. doi: 10.1111/raq.12100
Childs, A. R., Cowley, P. D., Næsje, T. F., Booth, A. J., Potts, W. M., Thorstad, E. B., et al. (2008). Do environmental factors influence the movement of estuarine fish? A case study using acoustic telemetry. Estuar. Coast. Shelf Sci. 78, 227–236. doi: 10.1016/j.ecss.2007.12.003
Clark, T. D., Sandblom, E., Hinch, S. G., Patterson, D. A., Frappell, P. B., and Farrell, A. P. (2010). Simultaneous biologging of heart rate and acceleration, and their relationships with energy expenditure in free-swimming sockeye salmon (Oncorhynchus nerka). J. Comp. Physiol. B. 180, 673–684. doi: 10.1007/s00360-009-0442-5
Cooke, S. J., Niezgoda, G. H., Hanson, K. C., Suski, C. D., Phelan, F. J., Tinline, R., et al. (2005). Use of CDMA acoustic telemetry to document 3-D positions of fish: relevance to the design and monitoring of aquatic protected areas. Mar. Tech. Soc. J. 39, 31–41. doi: 10.4031/002533205787521659
Coutant, C. C. (1977). Compilation of temperature preference data. J. Fish. Board Can. 34, 739–745. doi: 10.1139/f77-115
Crossin, G. T., Heupel, M. R., Holbrook, C. M., Hussey, N. E., Lowerre-Barbieri, S. K., Nguyen, V. M., et al. (2017). Acoustic telemetry and fisheries management. Ecol. Appl. 27, 1031–1049. doi: 10.1002/eap.1533
Cubitt, K. F., Churchill, S., Rowsell, D., Scruton, D. A., and McKinley, R. S. (2003). “3-dimensional positioning of salmon in commercial sea cages: assessment of a tool for monitoring behaviour,” in Aquatic telemetry. Advances and applications. Proceedings of the Fifth Conference on Fish Telemetry held in Europe, Ustica, Italy, 25–33.
Dempster, T., Uglem, I., Sanchez-Jerez, P., Fernandez-Jover, D., Bayle-Sempere, J., Nilsen, R., et al. (2009). Coastal salmon farms attract large and persistent aggregations of wild fish: an ecosystem effect. Marine Ecol. Prog. Ser. 385, 1–14. doi: 10.3354/meps08050
Dempster, T., Wright, D., and Oppedal, F. (2016). Identifying the Nature, Extent and Duration of Critical Production Periods for Atlantic salmon in Macquarie Harbour, Tasmania, During Summer. Fisheries Research and Development Corporation Report no. 2016–229.
Elliott, J. M., and Elliott, J. A. (2010). Temperature requirements of Atlantic salmon, brown trout and Arctic charr: predicting the impacts of climate change. J. Fish Biol. 77, 1793–1817 doi: 10.1111/j.1095-8649.2010.02762.x
Fernö, A., Huse, I., Juell, J. E., and Bjordal, Å. (1995). Vertical distribution of Atlantic salmon (Salmo solar L.) in net pens: trade-off between surface light avoidance and food attraction. Aquaculture 132, 285–296. doi: 10.1016/0044-8486(94)00384-Z
Føre, M., Alfredsen, J. A., and Gronningsater, A. (2011). Development of two telemetry-based systems for monitoring the feeding behaviour of Atlantic salmon (Salmo salar L.) in aquaculture sea-cages. Comput. Electron. Agric. 76, 240–251. doi: 10.1016/j.compag.2011.02.003
Føre, M., Frank, K., Dempster, T., Alfredsen, J. A., and Høy, E. (2017). Biomonitoring using tagged sentinel fish and acoustic telemetry in commercial salmon aquaculture: a feasibility study. Aquacultural Eng. 78, 163–172 doi: 10.1016/j.aquaeng.2017.07.004
Føre, M., Frank, K., Norton, T., Svendsen, E., Alfredsen, J. A., Dempster, T., et al. (2018a). Precision fish farming: a new framework to improve production in aquaculture. Biosyst. Eng. 173, 176–193. doi: 10.1016/j.biosystemseng.2017.10.014
Føre, M., Svendsen, E., Alfredsen, J. A., Uglem, I., Bloecher, N., Sveier, H., et al. (2018b). Using acoustic telemetry to monitor the effects of crowding and delousing procedures on farmed Atlantic salmon (Salmo salar). Aquaculture 495, 757–765. doi: 10.1016/j.aquaculture.2018.06.060
Gamperl, A. K., Ajiboye, O. O., Zanuzzo, F. S., Sandrelli, R. M., Ellen de Fátima, C. P., and Beemelmanns, A. (2020). The impacts of increasing temperature and moderate hypoxia on the production characteristics, cardiac morphology and haematology of Atlantic Salmon (Salmo salar). Aquaculture 519, 734874. doi: 10.1016/j.aquaculture.2019.734874
Handisyde, N. T., Ross, L. G., Badjeck, M. C., and Allison, E. H. (2006). The Effects of Climate Change on World Aquaculture: A Global Perspective. Aquaculture and Fish Genetics Research Programme, Stirling Institute of Aquaculture. Final Technical Report. (Stirling: DFID) 151.
Hargrave, B. T., Silvert, W., and Keizer, P. D. (2005). “Assessing and managing environmental risks associated with marine finfish aquaculture,” in Environmental Effects of Marine Finfish Aquaculture (Berlin; Heidelberg: Springer), 433–461.
Haulsee, D. E., Breece, M. W., Brown, L. M., Wetherbee, B. M., Fox, D. A., and Oliver, M. J. (2018). Spatial ecology of Carcharias taurus in the northwestern Mid-Atlantic coastal ocean. Mar. Ecol. Prog. Ser. 597, 191–206. doi: 10.3354/meps12592
Hvas, M., Folkedal, O., Imsland, A., and Oppedal, F. (2017). The effect of thermal acclimation on aerobic scope and critical swimming speed in Atlantic salmon, Salmo salar. J. Exp. Bio. 220, 2757–2764. doi: 10.1242/jeb.154021
IPCC (2018). Global Warming of 1.5 C. An IPCC Special Report on the Impacts of Global Warming of 1.5°C Above Pre-industrial Levels and Related Global Greenhouse Gas Emission Pathways, in the Context of Strengthening the Global Response to the Threat of Climate Change, Sustainable Development, and Efforts to Eradicate Poverty, Vol. 1, eds V. Masson-Delmotte, P. Zhai, H.-O. Pörtner, D. Roberts, J. Skea, P. R. Shukla, A.Pirani, W. Moufouma-Okia, C. Péan, R. Pidcock, S. Connors, J. B. R. Matthews, Y. Chen, X. Zhou, M.I. Gomis, E. Lonnoy, T. Maycock, M. Tignor, and T. Waterfield (IPCC), 1–9.
Javaid, M. Y., and Anderson, J. M. (1967). Thermal acclimation and temperature selection in Atlantic salmon, Salmo salar, and rainbow trout, S. gairdneri. J. Fish. Board Can. 24, 1507–1513. doi: 10.1139/f67-124
Jobling, M. (1981). Temperature tolerance and the final preferendum—rapid methods for the assessment of optimum growth temperatures. J. Fish Bio. 19, 439–455. doi: 10.1111/j.1095-8649.1981.tb05847.x
Johannesen, Á., Patursson, Ø., Kristmundsson, J., Dam, S. P., and Klebert, P. (2020). How caged salmon respond to waves depends on time of day and currents. PeerJ. 8:e9313. doi: 10.7717/peerj.9313
Johansson, D., Laursen, F., Fern,ö, A., Fosseidengen, J. E., Klebert, P., Stien, L. H., et al. (2014). The interaction between water currents and salmon swimming behaviour in sea cages. PloS ONE 9:e97635. doi: 10.1371/journal.pone.0097635
Johansson, D., Ruohonen, K., Juell, J. E., and Oppedal, F. (2009). Swimming depth and thermal history of individual Atlantic salmon (Salmo salar L.) in production cages under different ambient temperature conditions. Aquaculture 290, 296–303. doi: 10.1016/j.aquaculture.2009.02.022
Johansson, D., Ruohonen, K., Kiessling, A., Oppedal, F., Stiansen, J. E., Kelly, M., et al. (2006). Effect of environmental factors on swimming depth preferences of Atlantic salmon (Salmo salar L.) and temporal and spatial variations in oxygen levels in sea cages at a fjord site. Aquaculture 254, 594–605. doi: 10.1016/j.aquaculture.2005.10.029
Juell, J. E. (1995). The behaviour of Atlantic salmon in relation to efficient cage-rearing. Rev. Fish Bio. Fish. 5, 320–335. doi: 10.1007/BF00043005
Juell, J. E., and Fosseidengen, J. E. (2004). Use of artificial light to control swimming depth and fish density of Atlantic salmon (Salmo salar) in production cages. Aquaculture 233, 269–282. doi: 10.1016/j.aquaculture.2003.10.026
Juell, J. E., and Westerberg, H. (1993). An ultrasonic telemetric system for automatic positioning of individual fish used to track Atlantic salmon (Salmo salar L.) in a sea cage. Aquacultural Eng. 12, 1–18. doi: 10.1016/0144-8609(93)90023-5
Kraus, R. T., Holbrook, C. M., Vandergoot, C. S., Stewart, T. R., Faust, M. D., Watkinson, D. A., et al. (2018). Evaluation of acoustic telemetry grids for determining aquatic animal movement and survival. Methods Ecol. Evol. 9, 1489–1502. doi: 10.1111/2041-210X.12996
Kupilik, M. J., and Petersen, T. (2014). Acoustic tracking of migrating salmon. J. Acoust. Soc. Am. 136, 1736–1743. doi: 10.1121/1.4894796
Macaulay, G., Warren-Myers, F., Barrett, L. T., Oppedal, F., Føre, M., and Dempster, T. (2021). Tag use to monitor fish behaviour in aquaculture: a review of benefits, problems and solutions. Rev. Aquaculture. doi: 10.1111/raq.12534
Martins, C. I. M., Galhardo, L., Noble, C., Damsgård, B., Spedicato, M. T., Zupa, W., et al. (2011). Behavioural indicators of welfare in farmed fish. Fish Physiol. Biochem. 38, 17–41. doi: 10.1007/s10695-011-9518-8
Moore, A., Russell, I. C., and Potter, E. C. E. (1990). The effects of intraperitoneally implanted dummy acoustic transmitters on the behaviour and physiology of juvenile Atlantic salmon, Salmo salar L. J. Fish Biol. 37, 713–721. doi: 10.1111/j.1095-8649.1990.tb02535.x
Muñoz, L., Aspillaga, E., Palmer, M., Saraiva, J. L., and Arechavala-Lopez, P. (2020). Acoustic telemetry: a tool to monitor fish swimming Behavior in sea-cage aquaculture. Front. Mar. Sci. 7:645. doi: 10.3389/fmars.2020.00645
Noble, C., Gismervik, K., Iversen, M. H., Kolarevic, J., Nilsson, J., Stien, L. H., et al. (2018). Welfare Indicators for Farmed Atlantic salmon: Tools for Assessing Fish Welfare. 351.
O'Donncha, F., and Grant, J. (2019). Precision Aquaculture. IEEE Internet Things Mag. 2, 26–30. doi: 10.1109/IOTM.0001.1900033
Ogilvie, D. M., and Anderson, J. M. (1965). Effect of DDT on temperature selection by young Atlantic salmon, Salmo salar. J. Fish. Board Can. 22, 503–512. doi: 10.1139/f65-046
Oldham, T., Oppedal, F., and Dempster, T. (2018). Cage size affects dissolved oxygen distribution in salmon aquaculture. Aquaculture Environ. Interact. 10, 149–156. doi: 10.3354/aei00263
Oliver, E. C., Donat, M. G., Burrows, M. T., Moore, P. J., Smale, D. A., Alexander, L. V., et al. (2018). Longer and more frequent marine heatwaves over the past century. Nat. Commun. 9, 1–12. doi: 10.1038/s41467-018-03732-9
Oppedal, F., Dempster, T., and Stien, L. H. (2011). Environmental drivers of Atlantic salmon behaviour in sea-cages: a review. Aquaculture 311, 1–18. doi: 10.1016/j.aquaculture.2010.11.020
Oppedal, F., Juell, J. E., and Johansson, D. (2007). Thermo-and photoregulatory swimming behaviour of caged Atlantic salmon: implications for photoperiod management and fish welfare. Aquaculture 265, 70–81. doi: 10.1016/j.aquaculture.2007.01.050
Oppedal, F., Juell, J. E., Tarranger, G. L., and Hansen, T. (2001). Artificial light and season affects vertical distribution and swimming behaviour of post-smolt Atlantic salmon in sea cages. J. Fish Biol. 58, 1570–1584. doi: 10.1111/j.1095-8649.2001.tb02313.x
Palstra, A. P., Arechavala-Lopez, P., Xue, Y., and Roque, A. (2021). Accelerometry of Seabream in a Sea-Cage: Is Acceleration a Good Proxy for Activity?. Front. Marine Sci. 8:144. doi: 10.3389/fmars.2021.639608
Pinkiewicz, T. H., Purser, G. J., and Williams, R. N. (2011). A computer vision system to analyse the swimming behaviour of farmed fish in commercial aquaculture facilities: A case study using cage-held Atlantic salmon. Aquacultural Eng. 45, 20–27. doi: 10.1016/j.aquaeng.2011.05.002
Remen, M., Oppedal, F., Torgersen, T., Imsland, A. K., and Olsen, R. E. (2012). Effects of cyclic environmental hypoxia on physiology and feed intake of post-smolt Atlantic salmon: initial responses and acclimation. Aquaculture 326, 148–155. doi: 10.1016/j.aquaculture.2011.11.036
Remen, M., Sievers, M., Torgersen, T., and Oppedal, F. (2016). The oxygen threshold for maximal feed intake of Atlantic salmon post-smolts is highly temperature-dependent. Aquaculture 464, 582–592. doi: 10.1016/j.aquaculture.2016.07.037
Rillahan, C., Chambers, M. D., Howell, W. H., and Watson, I. I. I. W. H. (2011). The behavior of cod (Gadus morhua) in an offshore aquaculture net pen. Aquaculture 310, 361–368. doi: 10.1016/j.aquaculture.2010.10.038
Rothermel, E. R., Balazik, M. T., Best, J. E., Breece, M. W., Fox, D. A., Gahagan, B. I., et al. (2020). Comparative migration ecology of striped bass and Atlantic sturgeon in the US Southern mid-Atlantic bight flyway. PloS ONE 15:e0234442. doi: 10.1371/journal.pone.0234442
Sindermann, C. J. (1984). Disease in marine aquaculture. Helgoländer Meeresuntersuchungen 37, 505–530. doi: 10.1007/BF01989327
Stehfest, K. M., Carter, C. G., McAllister, J. D., Ross, J. D., and Semmens, J. M. (2017). Response of Atlantic salmon Salmo salar to temperature and dissolved oxygen extremes established using animal-borne environmental sensors. Sci. Rep. 7:4545. doi: 10.1038/s41598-017-04806-2
Stien, L. H., Bracke, M. B., Folkedal, O., Nilsson, J., Oppedal, F., Torgersen, T., et al. (2013). Salmon Welfare Index Model (SWIM 1.0): a semantic model for overall welfare assessment of caged Atlantic salmon: review of the selected welfare indicators and model presentation. Rev. Aquaculture 5, 33–57. doi: 10.1111/j.1753-5131.2012.01083.x
Tanaka, H., Naito, Y., Davis, N. D., Urawa, S., Ueda, H., and Fukuwaka, M. A. (2005). First record of the at-sea swimming speed of a Pacific salmon during its oceanic migration. Marine Ecol. Prog. Ser. 291, 307–312. doi: 10.3354/meps291307
Thorstad, E. B., Rikardsen, A. H., Alp, A., and Økland, F. (2013). The use of electronic tags in fish research–an overview of fish telemetry methods. Turk. J. Fish. Aquat. Sci. 13, 881–896. doi: 10.4194/1303-2712-v13_5_13
Ward, D., Føre, M., Howell, W. H., and Watson, W. (2012). The influence of stocking density on the swimming behavior of adult Atlantic cod, Gadus morhua, in a near shore net pen. J. World Aquaculture Soc. 43, 621–634. doi: 10.1111/j.1749-7345.2012.00593.x
Keywords: acoustic telemetry, Atlantic salmon, temperature, oxygen, feeding, behaviour
Citation: Stockwell CL, Filgueira R and Grant J (2021) Determining the Effects of Environmental Events on Cultured Atlantic Salmon Behaviour Using 3-Dimensional Acoustic Telemetry. Front. Anim. Sci. 2:701813. doi: 10.3389/fanim.2021.701813
Received: 28 April 2021; Accepted: 17 June 2021;
Published: 13 July 2021.
Edited by:
Martin Føre, Norwegian University of Science and Technology, NorwayReviewed by:
Pablo Arechavala-Lopez, University of Algarve, PortugalFletcher Warren-Myers, The University of Melbourne, Australia
Copyright © 2021 Stockwell, Filgueira and Grant. This is an open-access article distributed under the terms of the Creative Commons Attribution License (CC BY). The use, distribution or reproduction in other forums is permitted, provided the original author(s) and the copyright owner(s) are credited and that the original publication in this journal is cited, in accordance with accepted academic practice. No use, distribution or reproduction is permitted which does not comply with these terms.
*Correspondence: Caitlin L. Stockwell, cstockwell@dal.ca