- Department of Animal and Rangeland Sciences, Oregon State University, Corvallis, OR, United States
Nuclear factor erythroid 2-related factor 2 (NRF2) plays a key role in the response to oxidative stress. Diets containing known NRF2 modulators could be used to minimize oxidative stress in dairy cows. Currently, studies evaluating the activity of NRF2 in bovine have used the classical in vitro approach using synthetic media, which is very different than in vivo conditions. Furthermore, studies carried out in vivo cannot capture the short-term and dynamic response of NRF2. Thus, there is a need to develop new approaches to study NRF2 modulation. The aim of the present study was to establish an in vitro–in vivo hybrid system to investigate activation of NRF2 in bovine cells that can serve as an intermediate model with results closer to what is expected in vivo. To accomplish the aim, we used a combination of a gene reporter assay in immortalized bovine mammary cells, synthetic NRF2 modulators, and blood serum from periparturient cows. Synthetic agonist tert-butylhydroquinone and sulforaphane confirmed to be effective activators of bovine NRF2 with acute and large effect at 30 and 5 μM, respectively, with null response after the above doses due to cytotoxicity. When the agonists were added to blood serum the response was more linear with maximum activation of NRF2 at 100 and 30 μM, respectively, and the cytotoxicity was prevented. High concentration of albumin in blood serum plays an important role in such an effect. Brusatol (100 nM) was observed to be an effective NRF2 inhibitor while also displaying general protein synthesis inhibition and cytotoxicity when added to synthetic media. A consistent inhibition of NRF2 was observed when brusatol was added to the blood serum but the cytotoxicity was reduced. The synthetic inhibitor ML385 had no effect on modulation of bovine NRF2. Hydrogen peroxide activates NRF2 in bovine mammary cells starting from 100 μM; however, strong cytotoxicity was detected starting at 250 μM when cells were cultivated in the synthetic media, while blood serum prevented cytotoxicity. Overall, our data indicated that the use of synthetic media can be misleading in the study of NRF2 in bovine and the use of blood serum appears necessary.
Introduction
Oxidative stress is a physiological condition that negatively affects living organisms (Sies, 1997; Glasauer and Chandel, 2013). For high producing dairy cows, the most common incidences of oxidative stress occur as these animals transition from pregnancy to lactation. The peripartum is typically accompanied by a large mobilization of non-esterified fatty acids which are metabolized for energy via β-oxidation in a process that can leak electrons resulting in increased production of reactive oxygen species (ROS), one of the main culprits of oxidative stress (Bernabucci et al., 2005; Sordillo and Aitken, 2009; Marinho et al., 2014). The consequences of oxidative stress in dairy cows can also directly compromise milk production due to apoptosis and impaired function of mammary cells (Capuco et al., 2001).
Many of the endogenous antioxidant systems utilized by animals are regulated by a redox-sensitive transcription factor known as nuclear factor erythroid 2-related factor 2 (NRF2). NRF2 can be activated by a variety of conditions associated with oxidative stress. Once activated, NRF2 is released from KEAP1 and it is translocated into the nucleus where it functions to promote the transcription of a battery of genes associated with antioxidant defense and cell survival (Taguchi et al., 2011; Cuadrado et al., 2018).
While information regarding the role of NRF2 in ruminants is limited, prior research has indicated that it may be a target for reducing and preventing oxidative stress. In vitro models have been used to show that treatment of bovine cells with NRF2 activators, like sulforaphane and resveratrol, increases activity of NRF2 with concomitant antioxidant response limiting the damage caused by oxidative stress (Jin et al., 2016a; Sohel et al., 2018). In vivo studies have also shown that supplementing the feed of dairy cattle with chestnut tannins (Liu et al., 2013) or rumen-protected methionine (Han et al., 2018) improves the antioxidant status of the animals via upregulation of putative canonical NRF2 target genes associated with antioxidant defense and cell survival. While these studies have advanced our understanding of the role of NRF2 in ruminants, they are limited in the conclusions they can make regarding NRF2 modulation and activity in vivo. Studies conducted in vitro using synthetic media do not account for the environment that bovine cells are typically exposed to. Compounds present in circulating serum, including metabolites and hormones, can cause changes in activity of transcription factors and, thus, transcription of genes. However, the difficulty of measuring actual NRF2 activation in vivo is a clear limitation associated with these studies. Analyzing the regulation of NRF2 by assessing phosphorylation of NRF2 and changes in expression of NRF2 target genes in tissues obtained via biopsy, as these studies have done, provides only an indirect measure of NRF2 activation. Furthermore, the target genes of NRF2, although well-established in monogastric animals, have not been fully confirmed in bovine. Finally, NRF2 is a short-term dynamic response (Bischoff et al., 2019), that is difficult to capture with the limited number of samples that is possible to collect via biopsy. In order to overcome these limitations and advance the study of NRF2 in bovine, especially considering the potential for nutrigenomic approaches to alleviate oxidative stress via activation of this nuclear receptor (Houghton et al., 2016), new techniques need to be developed.
The objective of this study was to evaluate the accuracy and feasibility of a hybrid in vitro–in vivo system for evaluating NRF2 modulation in bovine mammary cells. To achieve this, serum collected from cows around parturition was used in place of synthetic media and in combination with an NRF2 gene reporter.
Materials and Methods
Common Materials and Methods
Blood Serum
Blood serum was collected from 5 Jersey and 5 Holstein cows in the control group of a prior study (Jaaf et al., 2020). Pool of blood serum samples were created using equal amount of serum from the five cows. Two prepartum serum pools [(–)Serum] were made, one pool using serum collected from Jersey cows (−29 ± 2 days relative to calving) and a second pool from Holstein cows (−21 ± 4 days relative to calving). Two post-partum serum pools [(+)Serum] were made, one pool using serum collected from Jersey cows (7 ± 0 days relative to calving) and a second pool from Holstein cows (10 ± 4 days relative to calving). Undiluted blood serum (i.e., 100%) was used with the cells in our study. The use of serum instead of plasma was due to preliminary observation of a certain degree of cytotoxicity when using plasma as a substrate for cells (data not shown) likely due to the presence of fibrinogen and other clotting factors in plasma, which are known to have cytotoxic effects and inhibit proliferation in vitro (Muraglia et al., 2017).
Cell Culture
Bovine mammary alveolar (MACT, Cellosaurus accession CVCL_U226) cells and human mammary carcinoma cells (MCF7, Cellosaurus accession CVCL_0031; generously provided by Dr. Brian Dolan, Carlson College of Veterinary Medicine, Oregon State University) were cultured in high-glucose Dulbecco's Modified Eagle's Medium (DMEM) with sodium pyruvate [Cat #25–500, Genesee Scientific, San Diego, CA] and 10% fetal bovine serum [Cat #F4135, Genesee Scientific] and grown and sub-cultured as previously described (Osorio and Bionaz, 2017). For the experiment described in Figure 4, 1% penicillin/streptomycin [Cat #P4333, Sigma-Aldrich, Milwaukee, WI] and 0.3% Amphotericin B [Cat #A2942, Sigma-Aldrich] were added to the media to assess the effect that antimitotic and antibiotic may have on NRF2 modulation. For this experiment, DMEM without antimitotics or antibiotics was denoted as DMEM(–/–), while the DMEM containing these supplements was denoted as DMEM(+/+).
Reagents
Tert-butylhydroquinone (tBHQ) [Cat #112941, Sigma-Aldrich], sulforaphane (SFN) [Cat #S4441, Sigma-Aldrich], ML385 [Cat #SML1833, Sigma-Aldrich], and brusatol (BRU) [Cat #SML1868, Sigma-Aldrich] were used to treat cells using a HP D300e Digital Dispenser [HP Inc., Palo Alto, CA]. All treatments were normalized for the amount of DMSO. Stock solutions were prepared by diluting each of the compounds in DMSO [Cat #21660, Research Organics, Cleveland, OH] to 50 mM for tBHQ, SFN, and ML385 and 8 mM for BRU. Bovine serum albumin, endotoxin free [Cat #AK8917-0100, Akron Biotech, Boca Raton, FL] was diluted in DMEM to concentrations of 1.2% and 3.2%. Hydrogen peroxide (H2O2) [Cat #HX0640-5, EMD Millipore, Billerica, MA] was diluted in DMEM to a working concentration of 500 μM.
Dual Luciferase Reporter Assay
MACT cells were cotransfected with the pgl4.37 [luc2P/ARE/Hygro] [Cat #E3641, Promega Corporation, Madison, WI] and pRL-TK [Cat #E2241, Promega Corporation] plasmids at a 40:1 ratio in OptiMEM [Cat #11058021, Genesee Scientific] using Lipofectamine 3000 [Cat #L3000001, Thermo Fisher Scientific, Carlsbad, CA] in accordance with a previously validated method (Osorio and Bionaz, 2017). Twenty-four hours after transfection, the media was removed and replaced with the appropriate treatment and cells were returned to the incubator. All treatments presented in this manuscript were performed for 24 h before the luciferase assay. The dual luciferase reporter assay was performed using the Dual-Glo Luciferase Assay System kit [Cat #E2920, Promega Corporation] following the manufacturer's instructions using a Synergy HTX Multi-Mode Reader [BioTek Inc., Winooski, VT] to read luminescence, at 1 mm height, 5 s integration time, and 200 gain.
Cell Imaging
Cell viability was assessed via staining with propidium iodide [PI, Cat #R37108, Thermo Fisher Scientific] and Hoescht 33342 Blue Dye [Cat #62249, Thermo Fisher Scientific] and viewed using DAPI and TXRed filters on a Leica DMI6000B Microscope [Leica Microsystems, Buffalo Grove, IL]. Images were analyzed for cell count and viability using Cell Profiler 3.0 (Mcquin et al., 2018) following a prior published pipeline (Osorio and Bionaz, 2017).
Experiments
Test of Synthetic NRF2 Modulators
While there are many compounds that activate NRF2, the natural isothiocyanate SFN and the synthetic phenol tBHQ were selected due to their utilization in other bovine cell studies (Jin et al., 2016b; Sohel et al., 2018) where 10 μM was found to be an effective dose for both compounds. Two compounds tested for their NRF2 inhibitory capacity were used in this study. BRU is a natural quassinoid and has been utilized in many studies in mammals as an effective inhibition of NRF2 at a dose of ≥100 nM (Olayanju et al., 2015). The other NRF2 inhibitor used in this study was the synthetic small molecule ML385. This molecule inhibited NRF2 activity in human lung carcinoma cells A549 at a dose of 5 μM (Singh et al., 2016).
In order to evaluate NRF2 modulation in the different growth media, we tested the above compounds using six increasing doses (from 0 to 100 μM for all compounds, except for SFN where the larger dose was 50 μM) in DMEM, (–)Serum or (+)Serum collected from Jersey cows for 24 h in MACT cells transfected with the NRF2 gene reporter. The renilla luminescence was used as an indicator of both cell activity/viability (Paguio et al., 2010) and protein synthesis/translation. Acute toxicity was assessed after 24 h of treatment by staining cells with Hoescht 33342 and propidium iodide. The # of cells/well served as a count of the total number of nucleated cells per frame scaled to the total well area. Acute toxicity was calculated as % of the number of red cells (i.e., dead cells) over the number of blue cells (all cells).
A second experiment was performed to study the finer modulation of NRF2 by SFN and tBHQ (10 doses from 0 to 30 for SFN and 0 to 50 μM for tBHQ) and to assess larger doses for ML385 (seven doses from 0 to 500 μM). In the same experiment we also withdrew the use of antibiotics and used the serum collected from Holstein cows. The change was justified by the fact that antibiotics in cell culture can induce oxidative stress (Kalghatgi et al., 2013) and cattle breeds can have a different response to oxidative stress-modulating treatments (Xu et al., 2017; Jaaf et al., 2020). Thus, we assessed also if the response to NRF2 activators is consistent when MACT cells are cultivated in absence of penicillin/streptomycin and amphotericin B and if there is any breed effect on the NRF2 modulation by the various compounds by using (–)Serum or (+)Serum collected from Holstein cows. The modulation of NRF2 and cytotoxicity were assessed as described above.
Role of Albumin and Antibiotics in Modulation of NRF2 by SFN and tBHQ
Blood contains a large amount of albumin. In bovine it is normally found in the 30 g/L range (Bionaz et al., 2007). It has been demonstrated that albumin can bind both SFN (Abassi et al., 2013) and tBHQ (Fathi et al., 2016). To assess if albumin play a role in the modulation of NRF2 by SFN and tBHQ when used in blood serum, an experiment was performed where MACT cells were cultivated in DMEM without antibiotics/antimitotic containing 0, 1.2, or 3.2% albumin or in blood serum and treated with 10 μM SFN or 30 μM tBHQ. To better understand the effect of antibiotics on SFN and tBHQ modulation, MACT cells were also cultivated in DMEM containing antibiotics and antimitotic and treated with SFN and tBHQ as above. Modulation of NRF2 as well cytotoxicity were assessed as described for section Test of Synthetic NRF2 Modulators.
Comparison of NRF2 Modulation by SFN and ML385 Between Bovine and Human Mammary Cells
ML385 is an effective inhibitor of NRF2 in human cells (Singh et al., 2016). To assess if ML385 inhibition of NRF2 is species-specific, we performed a side by side comparison between the response of NRF2 in human (MCF7) and bovine (MACT) mammary cells. Both cells were cultivated in the DMEM media treated with 10 μM SFN, 50 μM ML385, and a combination of the two. In addition, to better visualize the effect of blood serum on the various treatment, MACT cells were also cultivated in blood serum obtained by pooling samples collected from both pre- and post-partum Jersey cows and treated at the same above doses of SFN, ML385, and their combination. Modulation of NRF2 as well cytotoxicity were assessed as described for section Test of Synthetic NRF2 Modulators.
Modulation of NRF2 by H2O2 in DMEM and Blood Serum
The modulation of NRF2 by H2O2 was assessed in MACT cells cultivated in DMEM without antibiotics and antimitotic or in blood serum obtained by pooling samples collected from both pre- and post-partum Jersey cows. The MACT cells were transfected with the plasmids described in section Dual Luciferase Reporter Assay, and cells were treated for 24 h with varying doses of H2O2. Modulation of NRF2 as well cytotoxicity were assessed as described for section Test of Synthetic NRF2 Modulators.
Statistical Analysis
Statistical analysis of data was performed using SAS, Version 9.4 (SAS Institute, Cary, NC, USA). The PROC REG procedure in SAS was used to identify and remove outliers that had studentized t ≥ 3.0. The PROC GLIMMIX procedure was used with ARH (1) covariance structure to evaluate the treatment effect of all parameters measured and the differences between growth media types. Fixed effects in the model were dose, growth media type, or their interactions. Random effects were replicates (n = 4). Significance was declared at p ≤ 0.05. The significance of the linear and quadratic effects of dose were also assessed using GLM and denoted when significant.
Results
SFN and tBHQ Differentially Activate Bovine NRF2 in DMEM and Blood Serum
Treatment with SFN activated NRF2, although with a different dose-response pattern between cells cultured in DMEM and cells cultivated in blood serum. In cells cultivated in DMEM, peak NRF2 activation occurred at 5 μM, with a sharp decrease with higher doses (Figure 1A). In cells cultivated in blood serum SFN had a linear increase in activation of NRF2 up to 30 μM with no differences between the two blood sera collected from cows at different stages of lactation (Figure 1A); however, the activation of NRF2 decreased at higher doses. When renilla activity was utilized as a proxy for cell viability (Paguio et al., 2010) and number of cells and acute toxicity were assessed (Figures 1B–D), it became apparent that cell viability was compromised by SFN in a dose-dependent manner when added to DMEM. These results reflect what was found in another study where a dose of 5 μM was detected to be cytotoxic in human K562 lymphoblast cells 24 h after treatment (Pinz et al., 2014). Blood serum had a cytoprotective effect: while rates of translation were generally lower in the two blood serum pools vs. DMEM (Figure 1B), cytotoxicity was greater in DMEM vs. serum at doses between 5 and 30 μM (Figures 1C,D), helping to explain the perceived sharp decrease in NRF2 activation observed in DMEM after the 5 μM dose (Figure 1A).
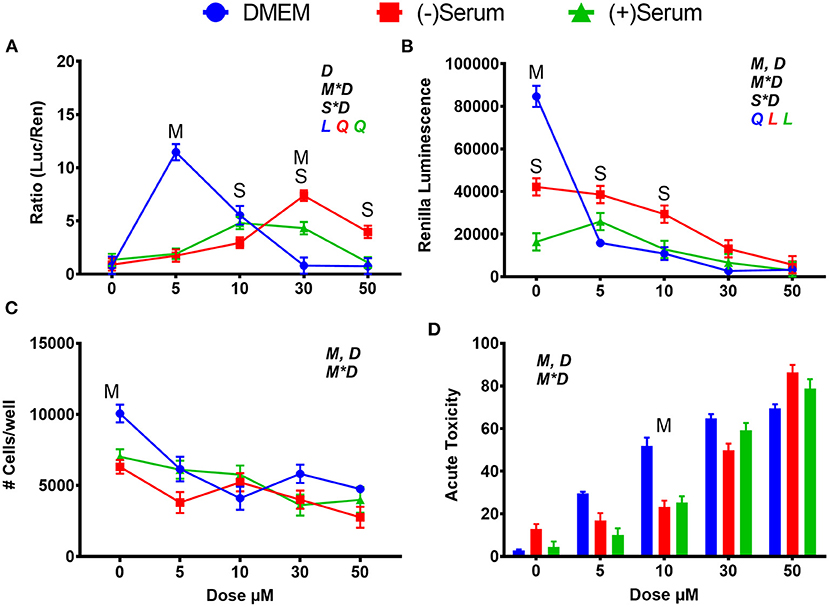
Figure 1. Modulation of NRF2 and cytotoxicity in MACT cells by sulforaphane after 24 h of treatment with increased doses in normal media containing 10% FBS (DMEM), blood serum collected from Jersey cows ~1-month prior parturition [(–) Serum] or collected at 7 days post-partum [(+) Serum]. (A) NRF2 activation was assessed via luc2P/ARE/Hygro and normalized by TK-renilla. (B) Renilla luminescence was used as an indicator of both cell activity/viability and protein synthesis/translation. (C) The # of cells/well served as a count of the total number of nucleated cells per frame scaled to the total well area. (D) Acute toxicity was assessed by staining cells with Hoescht 33,342 and propidium iodide. Acute toxicity was calculated as % of the number of red cells (i.e., dead cells) over the number of blue cells (all cells). Overall statistical effects of dose (D), media type (M), serum type (S), media type*dose (M*D), and serum type*dose (S*D) are indicated in the graph when significant (P ≤ 0.05). Indicated by L and Q symbols in the graph with the specific color for each treatment are significant (P ≤ 0.05) linear (L) and/or quadratic (Q) effects of the dose, respectively. When overall S*D and/or M*D are significant, the difference in response with P ≤ 0.05 at the same dose between the two blood serums or between serum and DMEM are indicated by the symbol S and M, respectively.
Similarly, to SFN, tBHQ activated NRF2, but at different doses in DMEM when compared to serum. In DMEM, the only level of tBHQ that activated NRF2 was 30 μM, with null effect at dose of ≥50 μM (Figure 2A). The rapid decrease in NRF2 activation after 30 μM in DMEM can be partly explained by the visual acidification of the DMEM, as observed by media transitioning to an orange color. It is known that a 1% solution of tBHQ in water has a pH of 4.56 (Pubchem, n.d.). The putative acidification is likely the cause of the cytotoxic effect observed supported by the tremendous decrease in renilla luminescence and cell viability (Figures 2B–D). Given these data, the low level of NRF2 activation seen at 50 and 100 μM of tBHQ in DMEM can be attributed to a reduction in cell survival. When tBHQ was added to cells cultivated in serum collected from pre- or post-partum cows, the dose-effect was linear, with the highest activation occurring at a dose of 100 μM in both sera (Figure 2A). In post-partum serum a significant activation of NRF2 was observed already at 10 μM. Different from DMEM, tBHQ in serum did not appear to be cytotoxic (Figures 2B–D). Even more so than what can be seen with SFN, serum media is serving a cytoprotective role, preventing the same levels of cell death seen in DMEM from occurring at doses of 10 μM and above.
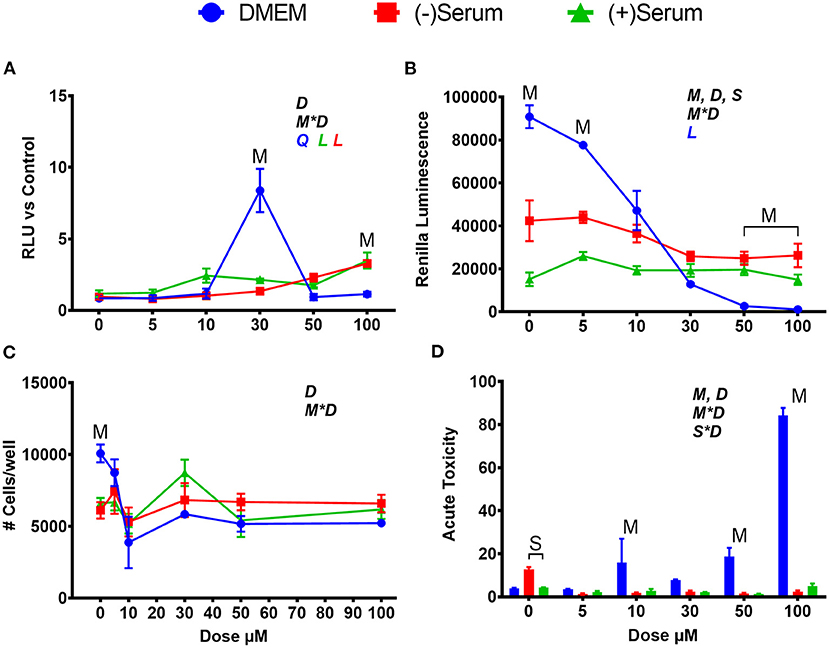
Figure 2. Modulation of NRF2 and cytotoxicity in MACT cells by tert-butylhydroquinone after 24 h of treatment with increased doses in normal media containing 10% FBS (DMEM), blood serum collected from Jersey cows ~1-month prior parturition [(–) Serum] or collected at 7 days post-partum [(+) Serum]. (A) NRF2 activation was assessed via luc2P/ARE/Hygro and normalized by TK-renilla. (B) Renilla luminescence was used as an indicator of both cell activity/viability and protein synthesis/translation. (C) The # of cells/well served as a count of the total number of nucleated cells per frame scaled to the total well area. (D) Acute toxicity was assessed by staining cells with Hoescht 33342 and propidium iodide. Acute toxicity was calculated as % of the number of red cells (i.e., dead cells) over the number of blue cells (all cells). Overall statistical effects of dose (D), media type (M), serum type (S), media type*dose (M*D), and serum type*dose (S*D) are indicated in the graph when significant (P ≤ 0.05). Indicated by L and Q symbols in the graph with the specific color for each treatment are significant (P ≤ 0.05) linear (L) and/or quadratic (Q) effects of the dose, respectively. When overall S*D and/or M*D are significant, the difference in response with P ≤ 0.05 at the same dose between the two blood serums or between serum and DMEM are indicated by the symbol S and M, respectively.
Within this experiment, many trends regarding the effects of DMEM and blood serum were observed. First, rates of translation, as measured by renilla luminescence, were significantly lower in (–)Serum and (+)Serum compared to DMEM, with (+)Serum being the lowest. It can also be seen that (–)Serum was more cytotoxic to cells when no treatment was applied (i.e., no NRF2 modulator). Prior studies have noted that cell proliferation and growth are significantly inhibited in mouse fibroblasts (L929 cells) when >40% blood serum is present in the medium (Liu et al., 2011). In our experiment, cells were exposed to 100% blood serum. A potential explanation for these observations may be the drastic differences in glucose concentrations between the DMEM and blood serum. The high-glucose formulation of DMEM contains ~25 mM of glucose whereas serum obtained from cows before and after parturition contains between 3.5 and 4.7 mM of glucose (Bionaz et al., 2007). It is also of interest the lower overall cell viability in serum collected from post-partum vs. pre-partum cows, suggesting that the former may contain compounds that negatively affect cell viability. Among those compounds, free fatty acids are known to be cytotoxic (Busato and Bionaz, 2020) and are elevated during the early post-partum (Bionaz et al., 2007; Gonçalves-De-Albuquerque et al., 2019). However, blood serum is also known to be cytotoxic by the release of reactive oxygen species when exposed to cell culture environment, as recently reviewed (Boehm and Bourke, 2019).
Activation of NRF2 by tBHQ and SFN Requires Specific Doses, and It Is Affected by Media Composition and Breed of Cows
The acute peaks in NRF2 activation seen in DMEM when cells were treated with SFN and tBHQ appear peculiar and further investigation was needed to assess the dose-response with a tighter range of doses.
In the previous experiment, peak activation of NRF2 in DMEM by SFN was observed at 5 μM, with a steep decline to 30 μM and beyond (Figure 1A); thus, in the current experiment, a closer range of doses (10 doses from 0 to 30 μM) were assessed. As shown in Figure 3A, activation of NRF2 increased from 5 to 7.5 to 10 μM SFN, with a sharp decline observed at 15 μM. Similar to what was found in the previous experiment (Figure 1A), activation of NRF2 increased in blood serum as the dose increased from 10 to 30 μM (Figure 3A). The sharp decline in NRF2 activation seen in DMEM at doses above 10 μM are likely due to losses in cell viability, as indicated by the significant decrease in renilla luminescence (Figure 3B).
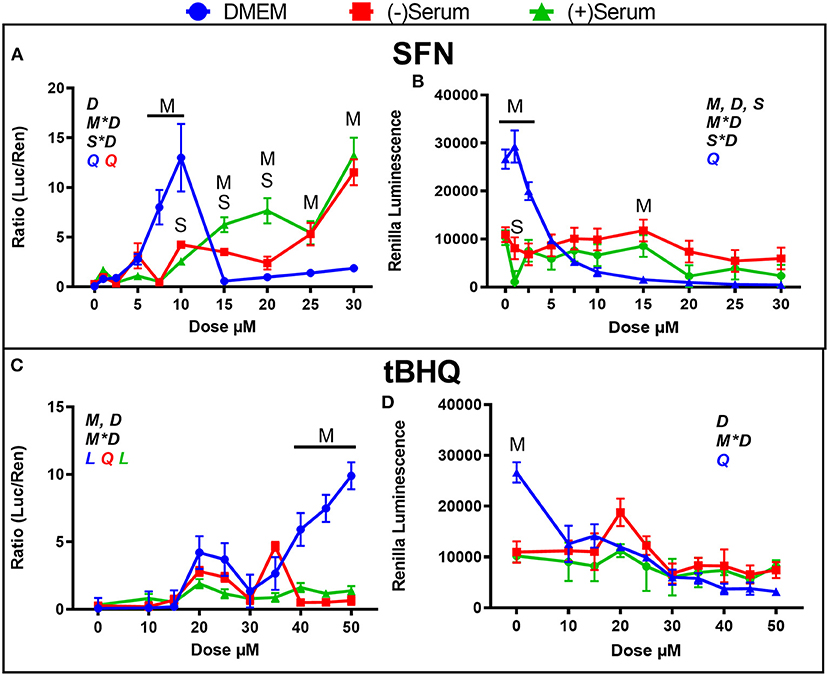
Figure 3. Modulation of NRF2 and cytotoxicity by a close range of doses of the putative activators (A,B) sulforaphane (SFN) and (C,D) tert-butylhydroquinone (tBHQ) in MACT cells. NRF2 activation was measured using luciferase luminescence normalized by renilla. The luminescence of renilla was used as proxy for cytotoxicity. Cells were treated for 24 h in normal media containing 10% FBS (DMEM). Blood serum was collected from Holstein cows ~1 month prior to parturition [(–)Serum] or ~10 days post-partum [(+)Serum]. Overall statistical effects of dose (D), media type (M), serum type (S), media type*dose (M*D), and serum type*dose (S*D) are indicated in the graph when significant (P ≤ 0.05). Indicated by L and Q symbols in the graph with the specific color for each treatment are significant (P ≤ 0.05) linear (L) and/or quadratic (Q) effects of the dose, respectively. When overall S*D and/or M*D are significant, the difference in response with P ≤ 0.05 at the same dose between the two blood serums or between serum and DMEM are indicated by the symbol S and M, respectively.
The range between 10 and 50 μM tBHQ, with peak activation at 30 μM that was observed in Figure 2A was also further investigated by using a closer range of doses, as shown in Figure 3C. Activation of NRF2 was significant with doses of 20 and 25 μM tBHQ in DMEM, but not at 30 μM as was observed in the previous experiment (Figure 2A). Different than the experiment performed without antibiotics where a decrease activation of NRF2 was observed with tBHQ >30 μM (Figure 2A), when tBHQ was added to media with antibiotics and antimitotic we observed an increase activation of NRF2 from 30 μM up to 50 μM (Figure 3C). As for the experiment in Figures 2B–D, the tBHQ was cytotoxic at dose ≥10 μM when MACT cells were cultivated in DMEM. In the previous experiment tBHQ activation of NRF2 had a linear increase starting at 10 μM dose in (+)Serum with significant activation in both blood serums collected from Jersey cows at 50 μM dose (Figure 2A). In the present experiment, where blood serum pools from Holstein cows were used minimal activation of NRF2 with tBHQ was observed for all doses, including 50 μM (Figure 3C). The only exception was an increased activation of NRF2 with 35 μM in pre-partum serum obtained from Holstein cows. The reason for the difference in the response of NRF2 modulation to tBHQ in MACT cells when cultivated in the blood serum of the two breeds of cows is unknown. However, as for the experiment performed in blood serum from Jersey cows, the toxicity of tBHQ was prevented when MACT cells were cultivated in blood serum collected from Holstein cows (Figure 3D).
Albumin in Media Affects the Modulation of NRF2 by Agonists and Prevents Cell Toxicity
The difference in NRF2 modulation by various synthetic agonists/antagonists when MACT cells are cultivated in blood serum or DMEM may be explained by the higher concentration of albumin in blood serum compared to DMEM. The DMEM used in this experiment contained ~0.2 g/L of albumin (from the 10% FBS supplemented) whereas the albumin level in blood serum is >30 g/L (Bionaz et al., 2007). It has been demonstrated that albumin can bind both SFN (Abassi et al., 2013) and tBHQ (Fathi et al., 2016); thus, it seems possible that the high concentrations of albumin in pre- and post-partum blood serum reduced the amount of free SFN and tBHQ available to modulate NRF2, as well as their cytotoxicity. This would mean that a higher concentration of those NRF2 activators would be needed in order to achieve the same result when cells are cultivated in blood serum as in DMEM. Thus, we performed an experiment where we tested increased levels of albumin in DMEM to test the hypothesis that the difference in activation of NRF2 by SFN and tBHQ observed between DMEM and serum is due to the higher level of albumin in blood serum. Increased concentration of albumin in media both decreased cytotoxicity and NRF2 activation when treated with 10 μM SFN (Figure 4A) or 30 μM tBHQ (Figure 4B) with media containing 3.2% BSA having the greater effect. Taken together, these results support our hypothesis of an important role of albumin in the observed difference in the NRF2 activation when cells are cultivated in blood serum or DMEM.
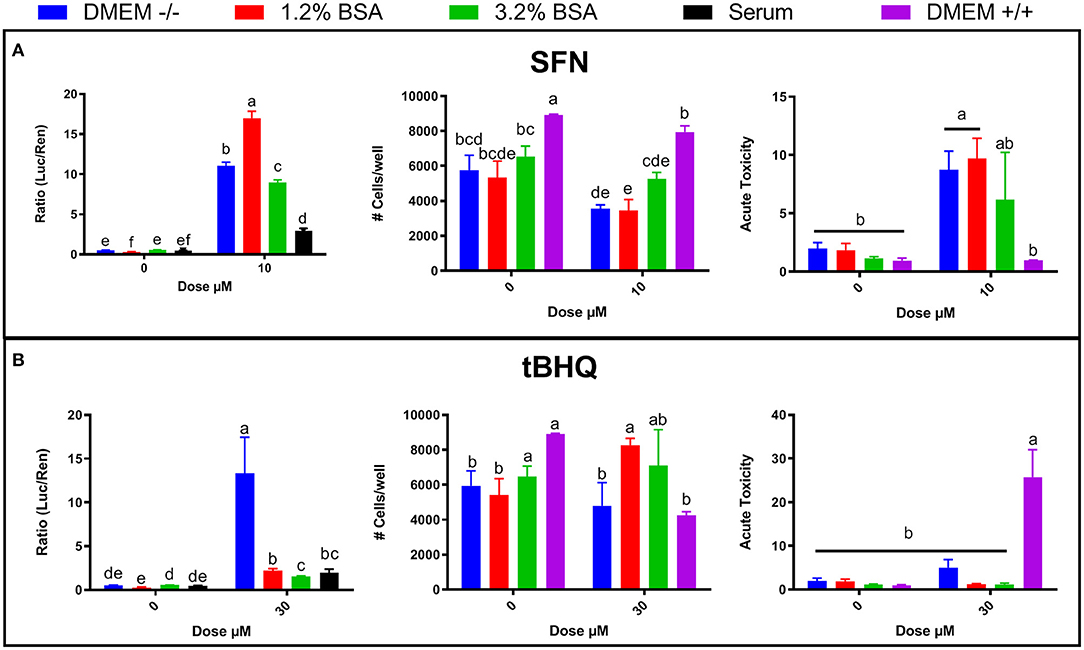
Figure 4. Effects of bovine serum albumin (BSA) concentration in DMEM (0.2, 1.2, and 3.2%), blood serum from periparturient Jersey cows, and media supplemented with FBS + penicillin/streptomycin + amphotericin B (DMEM +/+) on NRF2 modulation and cell viability by NRF2 activators sulforaphane (SFN) (A) and tert-butylhydroquinone (tBHQ) (B). NRF2 modulation was assessed by luciferase luminescence normalized by renilla. The number of cells/well served as a count of the total number of nucleated cells per frame scaled to the total well area. Acute toxicity was calculated as % of propidium iodide-stained cells (red cells) over all cells (blue). Significant (P ≤ 0.05) differences between treatments are denoted with dissimilar letters (i.e., a, b, c). Treatment*dose was significant (P ≤ 0.05) for all graphs.
DMEM With Antibiotic and Antifungal Supplements Affects Cell Viability and Toxicity
Given the discrepancy between the findings presented in Figures 1A, 2A, 3A on NRF2 activation and cell viability in response to SFN or tBHQ treatments, cell viability, and acute toxicity in MACT cells were also compared when cultivated in DMEM supplemented with antibiotics and antimitotic (DMEM+/+) or without them (DMEM–/–). In MACT cells not treated with NRF2 agonists, cell number as well as acute toxicity were improved in DMEM+/+ compared to DMEM–/–. Interestingly, treatment with 10 μM SFN in DMEM+/+ resulted in significantly lower acute toxicity than the same treatment performed in DMEM–/–. In contrast, treatment with 30 μM tBHQ in DMEM+/+ resulted in significantly greater acute toxicity than the same treatment performed in DMEM–/– (Figure 4).
Currently, there is no work indicating an interaction between SFN or tBHQ with any of the antifungal or antibiotic agents used in this study. Further work identifying any of these interactions of explanations for the differences in observations made in this study are warranted.
Brusatol Is an Effective Inhibitor of Bovine NRF2
When BRU was added to DMEM (Figure 5A), the inhibition of NRF2 activity was detected starting at 50 nM with the maximum inhibition observed with 75–100 nM. NRF2 was also inhibited at 100 nM of BRU when added to blood serum collected from pre- or post-partum cows; however, ≥50% inhibition was observed with 25 nM in both blood sera, with significant levels of inhibition at all doses in postpartum blood serum but not in prepartum serum (Figure 5A).
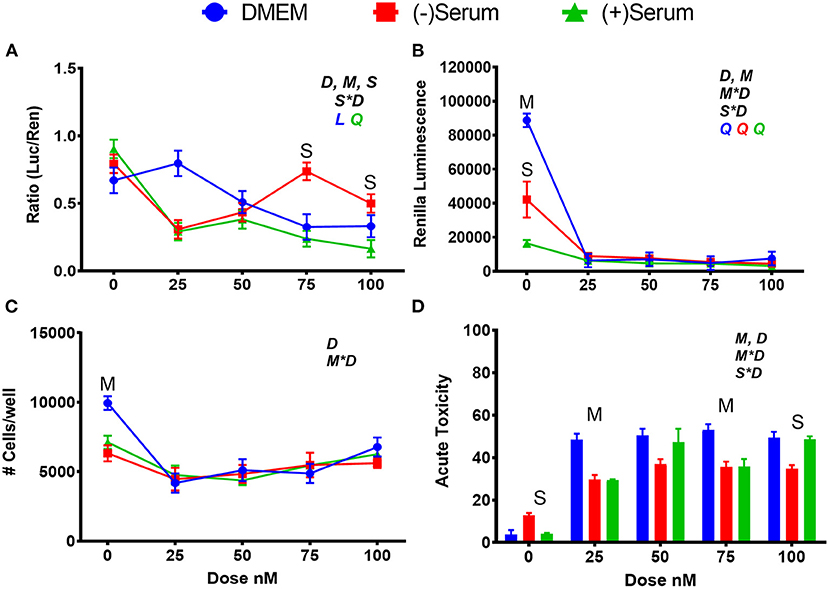
Figure 5. Modulation of NRF2 and cytotoxicity in MACT cells by brusatol after 24 h of treatment with increased doses in normal media containing 10% FBS (DMEM), blood serum collected from Jersey cows ~1-month prior parturition [(–) Serum] or collected at 7 days post-partum [(+) Serum]. (A) NRF2 activation was assessed via luc2P/ARE/Hygro and normalized by TK-renilla. (B) Renilla luminescence was used as an indicator of both cell activity/viability and protein synthesis/translation. (C) The # of cells/well served as a count of the total number of nucleated cells per frame scaled to the total well area. (D) Acute toxicity was assessed by staining cells with Hoescht 33342 and propidium iodide. Acute toxicity was calculated as % of the number of red cells (i.e., dead cells) over the number of blue cells (all cells). Overall statistical effects of dose (D), media type (M), serum type (S), media type*dose (M*D), and serum type*dose (S*D) are indicated in the graph when significant (P ≤ 0.05). Indicated by L and Q symbols in the graph with the specific color for each treatment are significant (P ≤ 0.05) linear (L) and/or quadratic (Q) effects of the dose, respectively. When overall S*D and/or M*D are significant, the difference in response with P ≤ 0.05 at the same dose between the two blood serums or between serum and DMEM are indicated by the symbol S and M, respectively.
Brusatol is an effective inhibitor of bovine NRF2, but it has also a known inhibitory effect on global protein synthesis (Vartanian et al., 2016). The function of BRU as a global protein synthesis inhibitor and cytotoxic agent was confirmed in MACT cells by analysis of constitutively expressed renilla, and by our cell viability assays (Figures 5B–D). A steep decline in renilla luminescence was observed at all doses and media utilized in this study, and mimic the patterns seen in the two measurements of cell viability. Both an ~50% decrease in cell viability and ~50% increase in acute toxicity were observed in DMEM at all doses above 0 μM whereas renilla luminescence decreased by ~95%. Taking these data together, it can be estimated that ~45% of the decrease in renilla luminescence caused by BRU can be attributed to its translation inhibitory capacity.
ML385 Fails to Inhibit Basic NRF2 Activity in Bovine Cells but Partly Prevents NRF2 Activation by SFN
ML385 is an effective inhibitor of NRF2 in human cells (Singh et al., 2016), but no such effect was observed in MACT cells (Figure 6A) with a minimal effect on cell viability (Figures 6B–D). Interestingly, 10 μM ML385 had a significant level of cytotoxicity in (+)Serum as measured both by the number of cells per well as well as the acute toxicity (Figures 6B–D). However, this result was not reflected at doses above 10 μM.
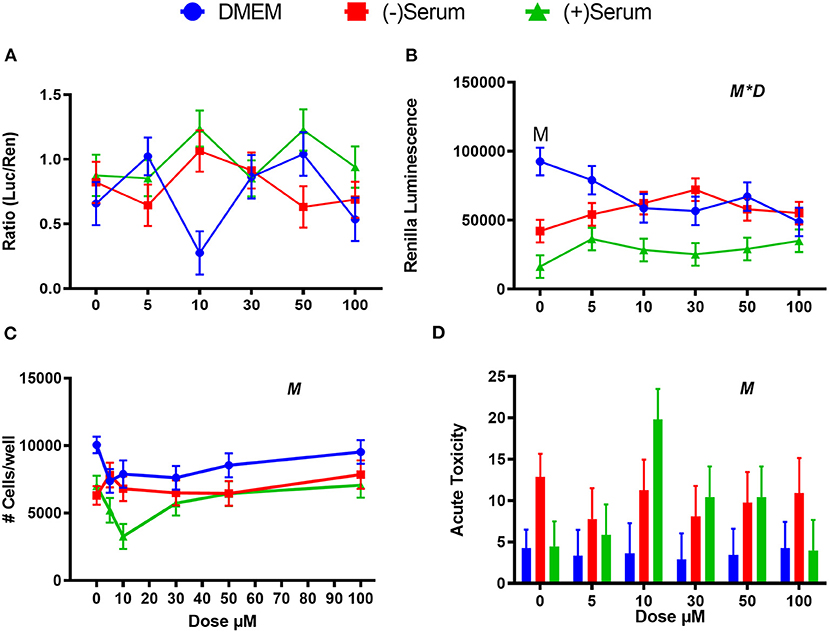
Figure 6. Modulation of NRF2 and cytotoxicity in MACT cells by ML385 after 24 h of treatment with increased doses in normal media containing 10% FBS (DMEM), blood serum collected from Jersey cows ~1-month prior parturition [(–) Serum] or collected at 7 days post-partum [(+) Serum]. (A) NRF2 activation was assessed via luc2P/ARE/Hygro and normalized by TK-renilla. (B) Renilla luminescence was used as an indicator of both cell activity/viability and protein synthesis/translation. (C) The # of cells/well served as a count of the total number of nucleated cells per frame scaled to the total well area. (D) Acute toxicity was assessed by staining cells with Hoescht 33342 and propidium iodide. Acute toxicity was calculated as % of the number of red cells (i.e., dead cells) over the number of blue cells (all cells). Overall statistical effects of dose (D), media type (M), and media type*dose (M*D) are indicated in the graph when significant (P ≤ 0.05). When overall M*D is significant, the difference in response with P ≤ 0.05 at the same dose between t serum and DMEM is indicated by the symbol M.
We used doses of ML385 that are known to inhibit NRF2 in human (Singh et al., 2016), but it is possible that higher doses are needed for ML385 to inhibit NRF2 in bovine. For this reason, we tested doses beyond 100 μM in MACT cells. As shown in Figure 7A, ML385 failed to inhibit NRF2 at doses above 100 μM. The observed activation at doses of 300 and 500 μM can likely be attributed to higher concentrations of DMSO required for that treatment. Due to the stock solution being at a concentration of 50 mM, DMSO at a level of 2.5% was present only in these treatments, while a 1% DMSO was used for all the other treatments. DMSO activate NRF2 at levels as low as 0.8% (Liang et al., 2011). DMSO is also known to be toxic for cells with doses >1%, as reported for a rat retinal ganglion cell line (Galvao et al., 2014). The sharp decrease in viability with doses of DMSO >1% (i.e., ML385 ≥ 300 μM) appears to confirm those observations (Figure 7B).
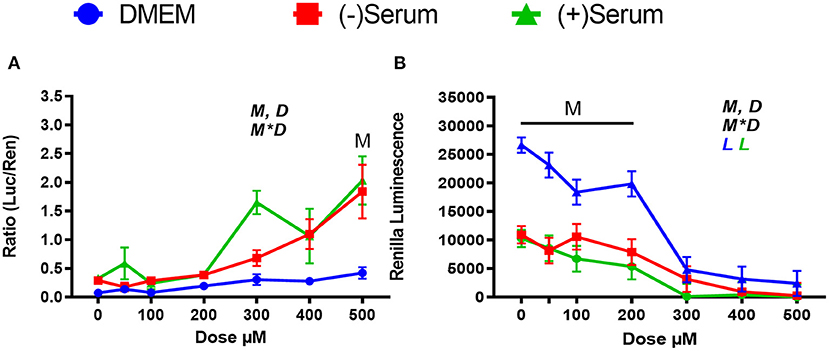
Figure 7. Modulation of NRF2 and cytotoxicity by a larger ranges of doses of the putative inhibitor ML385 in MACT cells. (A) NRF2 activation was measured using luciferase luminescence normalized by renilla. (B) The luminescence of renilla was used as proxy for cytotoxicity. Cells were treated for 24 h in normal media containing 10% FBS (DMEM). Blood serum was collected from Holstein cows ~1 month prior to parturition [(–)Serum] or ~10 days post-partum [(+)Serum]. Overall statistical effects of dose (D), media type (M), and media type*dose (M*D) are indicated in the graph when significant (P ≤ 0.05). Significant (P ≤ 0.05) linear effect of the dose is indicated by the L symbol in the graph with the specific color for each treatment. When overall M*D is significant, the difference in response with P ≤ 0.05 at the same dose between serum and DMEM is indicated by the symbol M.
In a follow-up experiment, we confirmed the lack of effect of ML385 on NRF2 modulation in MACT cells and an effective inhibitory effect of the compound on NRF2 in human mammary cells MCF7 (Figures 8A,B). However, despite the inability of ML385 to inhibit the basal activation of NRF2 in MACT cells, it was able to significantly reduce NRF2 activation by SFN in both bovine and human mammary cells (Figures 8A,B).
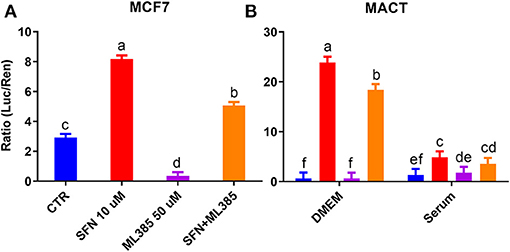
Figure 8. Modulation of NRF2 by SFN (10 μM), ML385 (50 μM), and their combination in human mammary cells (MCF7) cultivated in DMEM (A) or bovine mammary cells (MACT) cultivated in DMEM or a pool of blood serum samples from peripartum Jersey cows (B). NRF2 modulation was assessed by luciferase luminescence normalized by renilla. Significant (P ≤ 0.05) differences between treatments are denoted with dissimilar letters (i.e., a, b, and c). In (B) the NRF2 activation was significantly higher in DMEM compared to blood serum (P ≤ 0.05).
One possible explanation for the lack of inhibition of NRF2 by ML385 in bovine cells but effective inhibition in human cells, is the difference in protein sequence between bovine and human NRF2. The effect of ML385 on NRF2 was demonstrated to be on the functional domain Nrf2-ECH homology (Neh1) section of human NRF2, which codes for the basic leucine zipper (BRLZ), the domain responsible for DNA binding activity of the protein, located at positions 434–561 of the protein (Singh et al., 2016). We performed a domain-specific alignment of the whole NRF2 sequence in human, bovine, and mouse (Supplementary Figure 1). Our results revealed almost complete identity, with the exception of a point mutation in the basic zipper domain from a lysine to an arginine at position 547, 49 amino acids from the beginning of the domain. ML385 is an effective NRF2 inhibitor also in mouse (Tan et al., 2020); however, our analyses revealed that mouse NRF2 is more similar to human NRF2 than to its bovine counterpart, and that the point mutation observed between human and bovine NRF2 is not present in mouse NRF2 (Supplementary Figure 1).
H2O2 Activates NRF2 at Supraphysiological Doses, but It Is Cytotoxic in DMEM
There is considerable evidence supporting the role of H2O2 as an NRF2 activator, but its mechanism in promoting NRF2 activation is not fully understood (Fourquet et al., 2010). Low concentrations (<200 μM) of H2O2 promote rapid de novo synthesis of NRF2, while NRF2 translocation into the nucleus is a more delayed response (Covas et al., 2013). Studies on the effects of doses ≥200 μM supraphysiological doses in ruminant models is limited, but it has been shown that H2O2 up to 500 μM can affect NRF2 activity, albeit with negative consequences on cell viability (Jin et al., 2016a). In order to assess the effect of increasing concentrations of H2O2 on NRF2 activity and cell viability, NRF2 modulation using a luciferase assay and a cell toxicity assay were performed following 24 h of treatment.
As shown in Figure 9A, 250 μM H2O2 activates NRF2 in both blood serum and DMEM and 500 μM H2O2 causing significant activation only in blood serum, where the dose-response activation was linear. The dramatic decline in NRF2 activation detected with 500 μM H2O2 in DMEM can be explained by the cytotoxicity. At both 250 and 500 μM H2O2, cytotoxicity was observed in DMEM, but not or minor cytotoxicity was detected when the same treatments were performed in blood serum (Figure 9B). Taken together, the low activation of NRF2 with 500 μM H2O2 in DMEM can be attributed to cell death.
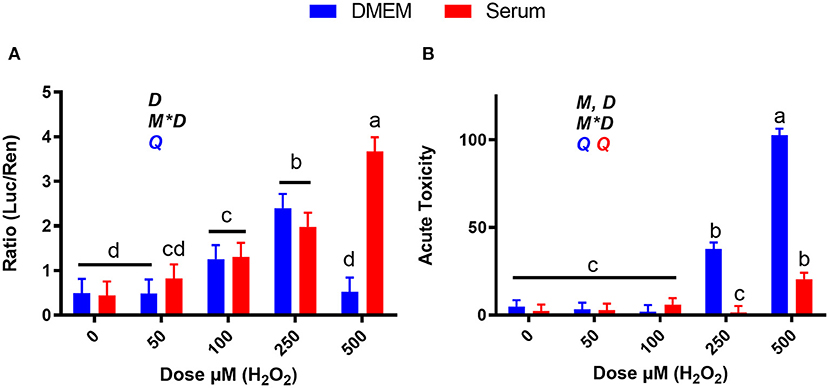
Figure 9. Dose-response effects of H2O2 on NRF2 modulation and acute toxicity in DMEM or a pool of blood serum samples from peripartum Jersey cows. (A) NRF2 modulation was assessed by luciferase luminescence normalized by renilla. (B) The number of cells/well served as a count of the total number of nucleated cells per frame scaled to the total well area. Acute toxicity was calculated as % of propidium iodide-stained cells (red cells) over all cells (blue). Significant (P ≤ 0.05) differences between doses are denoted with dissimilar letters (i.e., a, b, and c). Overall statistical effects of dose (D), media type (M), and media type*dose (M*D) are indicated in the graph when significant (P < 0.05). The symbol Q in the graph denotes a significant (P ≤ 0.05) quadratic effect of the dose with the color corresponding to the media type.
Discussion
A hybrid in vivo–in vitro approach was assessed to investigate the modulation of the transcription factor NRF2 in bovine mammary cells with the purpose to determine best conditions to identify in future studies target genes of NRF2. Of the four synthetic modulators tested, only ML385 failed to modulate NRF2 in bovine cells.
Synthetic NRF2 Activators Are Effective in Bovine Cells With Important Difference When Cells Are Cultivated in Synthetic Media or Blood Serum
The findings presented in this study confirmed that SFN and tBHQ are effective NRF2 activators, further validating findings in other bovine models (Jin et al., 2016b; Sohel et al., 2018). It should be noted that in these studies both 10 μM SFN and 10 μM tBHQ were effective in preventing H2O2-induced (Sohel et al., 2018) and heat-induced (Jin et al., 2016a) oxidative damage.
Despite being effective NRF2 activators the compounds present cytotoxicity. This must be considered in selecting an appropriate dose of them to study NRF2 activation in vitro especially if synthetic media is used. In DMEM, both SFN and tBHQ caused significant losses in cell viability. The use of blood serum significantly reduced cytotoxicity. This effect of media types also influenced the dose-response to these treatments. The cytotoxic effects of SFN and tBHQ are well-understood in in vitro models (Eskandani et al., 2014; Sestili and Fimognari, 2015); however, the influence of blood serum on these treatments has not been previously explored. The difference in NRF2 activation and prevention of cytotoxicity observed between DMEM and blood serum can partly be explained by the level of albumin, which is known to bind both SFN and tBHQ (Abassi et al., 2013; Fathi et al., 2016).
SFN, abundant in cruciferous vegetables such as broccoli, is considered to be one of the most effective phytochemicals with nutraceutical and nutrigenomic properties due to its agonistic effect on NRF2 and the high bioavailability (Houghton et al., 2016; Houghton, 2019). Interestingly, there are few brassica plants potentially containing SFN that can be used as forages for ruminants including turnips, swedes, rape, kale, and hybrids (Havilah, 2011). Thus, our data indicating a strong activation of NRF2 by SFN with little cytotoxicity when the cells are cultivated in blood serum, support the possibility of increasing NRF2 activation in vivo by the use of forages containing high amount of SFN. Our data however indicated that the activation of NRF2 with SFN can be achieved by a specific dose, which prompt for in vivo studies to identify the best dose of the forages containing SFN to obtain effective NRF2 activation. Based upon this, it is plausible to suggest that inclusion of forages containing SFN in the diet of dairy cows experiencing oxidative stress may be an effective way to improve animal health and performance.
tBHQ is commonly used in human food as a preservative, especially for its chemical antioxidant properties (García-García and Searle, 2016). tBHQ is also a strong antioxidant compound at the cellular level by acting on different pathways, with NRF2 playing a central role (Zhao et al., 2020). Different than SFN, tBHQ is a synthetic compound that is not present in plants. Thus, the use of this compound can be effective in studying NRF2 in bovine cells in vitro, but the use of this compound in vivo appears to be limited. Similar to what was observed for SFN, our data clearly indicated the limitations of using synthetic media to study NRF2 activation using tBHQ and the use of blood serum appears a better model, with less toxicity and a likely more realistic depiction of what could happen in vivo.
Our data reveal a strong effect of the various conditions of the media, such as the presence of antibiotics and antimitotic, and/or albumin, on the effect of NRF2 modulators. Taken together, our data emphasize the necessity for a hybrid approach like the one utilized in this study that can better account for in vivo conditions and allow for more biologically relevant conclusions to be made.
Synthetic NRF2 Inhibitors Have Limited Use in Bovine Cells
Brusatol, a natural quassinoid derived from the Brucea javanica plant, has been investigated recently for its NRF2-inhibiting qualities especially in regard to cancer, where constitutive activation of NRF2 can impart a degree of chemoresistance to tumors (Olayanju et al., 2015). In our study, the role of brusatol as an effective NRF2 inhibitor in bovine cells was confirmed but we also observed the classical inhibition of protein translation. In both serum and DMEM, brusatol was able to inhibit NRF2 activation albeit at different doses. The differences in dose response between treatments performed in DMEM compared to those performed in blood serum was an unexpected finding and can partially be explained by the increased concentration of albumin present in serum. The negative effect on protein translation can however be a confounding factor when using this modulator to study NRF2 activation. The overall decrease in translation can affect many more pathways than only NRF2; thus, limiting the possibility of using this compound to perform transcriptome analysis with the purpose of identifying NRF2 target genes.
Our data clearly indicate that ML385, a synthetic inhibitor of NRF2 in human cells, cannot be used to inhibit NRF2 in bovine cells. The analysis of the protein sequence indicated a high degree of conservation of the bovine NRF2 with the human NRF2, including the BRLZ domain. While these results might suggest identical magnitude of function of the two proteins, BRLZ domains are highly conserved across several species (Malek and Haft, 2001) and specific mutations in the protein sequence can cause functional changes (Bloom et al., 2002; Hayes and Mcmahon, 2009). Furthermore, point mutations in the BRLZ cause drastic change in protein behavior: such is the case of the Neural Retina Leucine Zipper (NRL), a similar leucine zipper domain to the one of NRF2, in which an individual with a serine to arginine substitution caused reduced DNA-binding of NRL, and a decrease in its transcriptional activity (Collin et al., 2011). As such, it is entirely plausible that a single substitution in the BRLZ domain might lead bovine NRF2 to lose sensitivity to ML385. The above dissimilarities can be enough to determine a difference in response, as previously inferred by a high conservation between bovine and monogastric Peroxisome Proliferator-activated Receptors but a difference in response to fatty acids (Bionaz et al., 2012).
It is however of interest the observation that ML385 interferes with the activation of NRF2 by SFN in both human and bovine cells. It is unclear the reason for such observation. One potential explanation could be differences in how NRF2 is bound to KEAP1, with species-specific differences perhaps allowing for ML385 to function before NRF2 is released in mouse and human cells but preventing ML385 from interacting with NRF2 until it has already been activated in bovine cells. However, multispecies investigations comparing the NRF2-KEAP1 interaction are limited with previous studies focusing on differences in NRF2 target genes (Hayes and Mcmahon, 2009). Future experiments investigating the NRF2-KEAP-ML385 interaction could be performed to further understand this phenomenon.
H2O2 Is a NRF2 Activator but Only at Supraphysiological Doses
Our results confirmed H2O2 to be a NRF2 activator, but at doses that are likely above physiological concentrations typically found in cows. Measurement of H2O2 is very difficult due to the high reactivity of H2O2 which rapidly disappears. However, in literature the concentration of H2O2 in bovine plasma/serum has been previously reported. Concentration of H2O2 in plasma was reported for multiparous Holstein cows during the transition period (Gong and Xiao, 2018) or in blood serum during an unspecified stage of lactation (Nedić et al., 2019). However, in the study performed during the transition period, plasma concentrations of H2O2 appear excessive (>40 mM) and ~9-fold higher than the maximum dose we tested in our in vitro study. In the latter study the concentration of H2O2 in blood serum was in the 40–80 μM range that we tested in vitro. Those doses had however a minimal-to-not effect on NRF2 activation in our in vitro study. If the data presented in the latter study are what could be considered physiological, our data may indicate a minor role of circulating H2O2 in activating NRF2 in vivo. However, H2O2 is produced intracellularly and it is released in high concentration locally by immune cells especially as consequence of oxidative burst (Wittmann et al., 2012). H2O2 is known to be cytotoxic, however the extracellular concentration of H2O2 must be very high to damage the cells considering that the extracellular H2O2 is taken up by cells in a minor fashion. It has been measured a 390-fold difference between external and internal concentration of H2O2 (Lyublinskaya and Antunes, 2019). In our model, the high concentrations of H2O2 that were added into the media might be not biologically relevant.
As expected (Clément and Pervaiz, 2001), in DMEM H2O2 is cytotoxic at doses ≥250 μM. As seen with SFN and tBHQ, using blood serum in place of DMEM as growth media seemingly prevented the cytotoxicity of H2O2 at the least up to 500 μM, a phenomena that can be in part attributed to elevated levels of albumin in the blood serum. Considerable work has been done providing evidence for albumin interacting with H2O2 and hydroxyl radicals (Carballal et al., 2003; Roche et al., 2008), and preventing its interaction with other biochemical components.
While somewhat unexpected, there is evidence indicating that albumin can interact with H2O2 and free hydroxyl radicals (Carballal et al., 2003; Roche et al., 2008) thereby preventing their interaction with other biomolecules. Thus, it is possible that the presence of high levels of albumin in blood serum weakened the effects of H2O2 at higher doses and prevented the cytotoxic effects observed at the same doses in DMEM.
Conclusion
The use of a hybrid model for studying modulation of NRF2 in immortalized bovine cells resulted in important findings regarding this transcription factor and considerations for future in vitro studies, especially if the purpose is to determine the target genes of bovine NRF2. Putative NRF2 agonists tBHQ (30 μM) and SFN (10 μM) were confirmed to be effective NRF2 activators. In addition, H2O2 is a NRF2 activator but likely at supraphysiological doses.
For the inhibitors, although the putative antagonist brusatol (100 nM) inhibits basal activity of NRF2, the negative effect on overall protein synthesis is a confounding factor that limits the use of such compounds to determine, for instance, bovine NRF2 target genes. The previously tested small-molecule ML385 did not result in any NRF2 inhibition in MACT cells, with the exception of a negative interaction with SFN-induction of NRF2. Thus, an effective NRF2 inhibitor in bovine still need to be found.
Our experiments clearly demonstrated a significant difference in results for NRF2 activation as well as cytotoxicity when MACT cells were cultivated in synthetic media or blood serum and treated with NRF2 modulators. Both NRF2 activation and observed cytotoxicity at higher doses were reduced when MACT cells were cultivated in blood serum when compared to synthetic media. Our analysis reveal an important role of albumin in the observed effects.
Overall, our study provided the best doses to be used for modulating NRF2 using synthetic compounds in MACT cells. The performed optimization can be used to study with higher precision bovine NRF2, including the determination of target genes. Most importantly, our data reveal large differences in the response of MACT cells to NRF2 modulators when cultivated in synthetic media or blood serum. Considering that in vivo cells are surrounded by blood serum, our data indicate that in vitro studies should be performed using blood serum to increase biological relevance.
Limitations of the Study
Conclusions from our study should be limited to the model used (i.e., MACT cells). NRF2 activation can have a wide range of effects in different tissue types and so the results seen in this investigation may be different when the same set of tests are performed in other cells. Additionally, the blood serum used in this experiment was only from peripartum cows. While this allowed for a glimpse of the effect on NRF2 modulation by blood serum circulating in cows during the transition period, further testing using blood serum samples from dairy cows in additional stages of lactation are necessary to develop a more complete understanding of NRF2 modulation. Additionally, our data indicate a differential effect of various NRF2 modulators when in the presence of blood serum from Jersey or Holstein cows. The reason for such differential response is unclear; however, the data underline the limit of inferring data obtained by using blood serum from a specific breed to other breeds of cows.
Data Availability Statement
The raw data supporting the conclusions of this article will be made available by the authors, without undue reservation.
Ethics Statement
This study involved the use of animal serum collected in a previous study which was approved by the Institutional Animal Care and Use Committees of Oregon State University (protocol# 4894).
Author Contributions
HF conceived the idea, designed the experiments, performed the experiments, analyzed the data, created the graphs, interpreted the data, and wrote the manuscript. SB performed the alignment of NRF2 between species and wrote the related section, helped with carrying out some of the experiments, and revised the manuscript. MB conceived the idea, helped designing the experiments, helped interpreting the data, helped writing the manuscript, and revised the final manuscript. All authors contributed to the article and approved the submitted version.
Funding
This study was funded by the Agriculture Research Foundation.
Conflict of Interest
The authors declare that the research was conducted in the absence of any commercial or financial relationships that could be construed as a potential conflict of interest.
Supplementary Material
The Supplementary Material for this article can be found online at: https://www.frontiersin.org/articles/10.3389/fanim.2021.674355/full#supplementary-material
Supplementary Figure 1. Genomic alignment of human, bovine, and mouse NRF2.
References
Abassi, P., Abassi, F., Yari, F., Hashemi, M., and Nafisi, S. (2013). Study on the interaction of sulforaphane with human and bovine serum albumins. J. Photochem. Photobiol. B 122, 61–67. doi: 10.1016/j.jphotobiol.2013.02.001
Bernabucci, U., Ronchi, B., Lacetera, N., and Nardone, A. (2005). Influence of body condition score on relationships between metabolic status and oxidative stress in periparturient dairy cows. J. Dairy Sci. 88, 2017–2026. doi: 10.3168/jds.S0022-0302(05)72878-2
Bionaz, M., Thering, B. J., and Loor, J. J. (2012). Fine metabolic regulation in ruminants via nutrient-gene interactions: saturated long-chain fatty acids increase expression of genes involved in lipid metabolism and immune response partly through PPAR-α activation. Brit. J. Nutr. 107, 179–191.
Bionaz, M., Trevisi, E., Calamari, L., Librandi, F., Ferrari, A., and Bertoni, G. (2007). Plasma paraoxonase, health, inflammatory conditions, and liver function in transition dairy cows. J. Dairy Sci. 90, 1740–1750. doi: 10.3168/jds.2006-445
Bischoff, L. J. M., Kuijper, I. A., Schimming, J. P., Wolters, L., Braak, B. T., Langenberg, J. P., et al. (2019). A systematic analysis of Nrf2 pathway activation dynamics during repeated xenobiotic exposure. Arch. Toxicol. 93, 435–451. doi: 10.1007/s00204-018-2353-2
Bloom, D., Dhakshinamoorthy, S., and Jaiswal, A. K. (2002). Site-directed mutagenesis of cysteine to serine in the DNA binding region of Nrf2 decreases its capacity to upregulate antioxidant response element-mediated expression and antioxidant induction of NAD(P)H:quinone oxidoreductase1 gene. Oncogene 21, 2191–2200. doi: 10.1038/sj.onc.1205288
Boehm, D., and Bourke, P. (2019). Safety implications of plasma-induced effects in living cells - a review of in vitro and in vivo findings. Biol. Chem. 400, 3–17. doi: 10.1515/hsz-2018-0222
Busato, S., and Bionaz, M. (2020). The interplay between non-esterified fatty acids and bovine peroxisome proliferator-activated receptors: results of an in vitro hybrid approach. J. Anim. Sci. Biotechnol. 11:91. doi: 10.1186/s40104-020-00481-y
Capuco, A. V., Wood, D. L., Baldwin, R., Mcleod, K., and Paape, M. J. (2001). Mammary cell number, proliferation, and apoptosis during a bovine lactation: relation to milk production and effect of bST. J. Dairy Sci. 84, 2177–2187. doi: 10.3168/jds.S0022-0302(01)74664-4
Carballal, S., Radi, R., Kirk, M. C., Barnes, S., Freeman, B. A., and Alvarez, B. (2003). Sulfenic acid formation in human serum albumin by hydrogen peroxide and peroxynitrite. Biochemistry 42, 9906–9914. doi: 10.1021/bi027434m
Clément, M. V., and Pervaiz, S. (2001). Intracellular superoxide and hydrogen peroxide concentrations: a critical balance that determines survival or death. Redox Rep. 6, 211–214. doi: 10.1179/135100001101536346
Collin, R. W. J., Born, L. I., van den Klevering, B. J., Castro-Miró, M., de Littink, K. W., Arimadyo, K., et al. (2011). High-resolution homozygosity mapping is a powerful tool to detect novel mutations causative of autosomal recessive RP in the dutch population. Invest. Ophthalmol. Vis. Sci. 52, 2227–2239.
Covas, G., Marinho, H. S., Cyrne, L., and Antunes, F. (2013). Activation of Nrf2 by H2O2: de novo synthesis versus nuclear translocation. Methods Enzymol. 528, 157–171. doi: 10.1016/B978-0-12-405881-1.00009-4
Cuadrado, A., Manda, G., Hassan, A., Alcaraz, M. J., Barbas, C., Daiber, A., et al. (2018). Transcription factor NRF2 as a therapeutic target for chronic diseases: a systems medicine approach. Pharmacol. Rev. 70, 348–383. doi: 10.1124/pr.117.014753
Eskandani, M., Hamishehkar, H., and Ezzati Nazhad Dolatabadi, J. (2014). Cytotoxicity and DNA damage properties of tert-butylhydroquinone (TBHQ) food additive. Food Chem. 153, 315–320. doi: 10.1016/j.foodchem.2013.12.087
Fathi, F., Ezzati Nazhad Dolatanbadi, J., Rashidi, M. R., and Omidi, Y. (2016). Kinetic studies of bovine serum albumin interaction with PG and TBHQ using surface plasmon resonance. Int. J. Biol. Macromol. 91, 1045–1050. doi: 10.1016/j.ijbiomac.2016.06.054
Fourquet, S., Guerois, R., Biard, D., and Toledano, M. B. (2010). Activation of NRF2 by nitrosative agents and H2O2 involves KEAP1 disulfide formation. J. Biol. Chem. 285, 8463–8471. doi: 10.1074/jbc.M109.051714
Galvao, J., Davis, B., Tilley, M., Normando, E., Duchen, M. R., and Cordeiro, M. F. (2014). Unexpected low-dose toxicity of the universal solvent DMSO. FASEB J. 28, 1317–1330. doi: 10.1096/fj.13-235440
García-García, R., and Searle, S. S. (2016). “Preservatives: food use,” in Encyclopedia of Food and Health, eds. B. Caballero, P. M. Finglas, and F. Toldrá (Oxford: Academic Press), 505–509.
Glasauer, A., and Chandel, N. S. (2013). ROS. Curr. Biol. 23, R100–102. doi: 10.1016/j.cub.2012.12.011
Gonçalves-De-Albuquerque, C. F., Barnese, M. R. C., Soares, M. A., Castro Faria, M. V., Silva, A. R., Castro Faria Neto, H. C., et al. (2019). Serum albumin-fatty acid saturation test. MethodsX 6, 1871–1875. doi: 10.1016/j.mex.2019.08.004
Gong, J., and Xiao, M. (2018). Effect of organic selenium supplementation on selenium status, oxidative stress, and antioxidant status in selenium-adequate dairy cows during the periparturient period. Biol. Trace Elem. Res. 186, 430–440. doi: 10.1007/s12011-018-1323-0
Han, L., Batistel, F., Ma, Y., Alharthi, A. S. M., Parys, C., and Loor, J. J. (2018). Methionine supply alters mammary gland antioxidant gene networks via phosphorylation of nuclear factor erythroid 2-like 2 (NFE2L2) protein in dairy cows during the periparturient period. J. Dairy Sci. 101, 8505–8512. doi: 10.3168/jds.2017-14206
Havilah, E. J. (2011). “Forages and Pastures | Annual Forage and Pasture Crops - Establishment and Management,” in Encyclopedia of Dairy Sciences, 2nd Edn, ed. J.W. Fuquay (San Diego, CA: Academic Press), 563–575.
Hayes, J. D., and Mcmahon, M. (2009). NRF2 and KEAP1 mutations: permanent activation of an adaptive response in cancer. Trends Biochem. Sci. 34, 176–188. doi: 10.1016/j.tibs.2008.12.008
Houghton, C. A. (2019). Sulforaphane: its “Coming of Age” as a clinically relevant nutraceutical in the prevention and treatment of chronic disease. Oxid. Med. Cell. Longev. 2019, 2716870–2716870. doi: 10.1155/2019/2716870
Houghton, C. A., Fassett, R. G., and Coombes, J. S. (2016). Sulforaphane and other nutrigenomic Nrf2 activators: can the clinician's expectation be matched by the reality? Oxid. Med. Cell. Longev. 2016:7857186. doi: 10.1155/2016/7857186
Jaaf, S., Batty, B., Krueger, A., Estill, C. T., and Bionaz, M. (2020). Selenium biofortified alfalfa hay fed in low quantities improves selenium status and glutathione peroxidase activity in transition dairy cows and their calves. J. Dairy Res. 87, 184–190. doi: 10.1017/S002202992000028X
Jin, X., Wang, K., Liu, H., Hu, F., Zhao, F., and Liu, J. (2016a). Protection of bovine mammary epithelial cells from hydrogen peroxide-induced oxidative cell damage by resveratrol. Oxid. Med. Cell. Longev. 2016:2572175. doi: 10.1155/2016/2572175
Jin, X. L., Wang, K., Liu, L., Liu, H. Y., Zhao, F. Q., and Liu, J. X. (2016b). Nuclear factor-like factor 2-antioxidant response element signaling activation by tert-butylhydroquinone attenuates acute heat stress in bovine mammary epithelial cells. J. Dairy Sci. 99, 9094–9103. doi: 10.3168/jds.2016-11031
Kalghatgi, S., Spina, C. S., Costello, J. C., Liesa, M., Morones-Ramirez, J. R., Slomovic, S., et al. (2013). Bactericidal antibiotics induce mitochondrial dysfunction and oxidative damage in Mammalian cells. Sci. Transl. Med. 5:192ra185. doi: 10.1126/scitranslmed.3006055
Liang, C., Xue, Z., Cang, J., Wang, H., and Li, P. (2011). Dimethyl sulfoxide induces heme oxygenase-1 expression via JNKs and Nrf2 pathways in human umbilical vein endothelial cells. Mol. Cell Biochem. 355, 109–115. doi: 10.1007/s11010-011-0844-z
Liu, H. W., Zhou, D. W., and Li, K. (2013). Effects of chestnut tannins on performance and antioxidative status of transition dairy cows. J. Dairy Sci. 96, 5901–5907. doi: 10.3168/jds.2013-6904
Liu, M., Hu, P. A., and Ding, K. X. (2011). [The effect of serum concentration on the growth, proliferation and collagen secretion in mouse L929 fibroblasts]. Xi Bao Yu Fen Zi Mian Yi Xue Za Zhi 27, 736–739.
Lyublinskaya, O., and Antunes, F. (2019). Measuring intracellular concentration of hydrogen peroxide with the use of genetically encoded H2O2 biosensor HyPer. Redox Biol. 24:101200. doi: 10.1016/j.redox.2019.101200
Malek, J. A., and Haft, D. H. (2001). Conserved protein domains are maintained in an average ratio to proteome size. Genome Biol. 2. doi: 10.1186/gb-2001-2-5-preprint0004
Marinho, H. S., Real, C., Cyrne, L., Soares, H., and Antunes, F. (2014). Hydrogen peroxide sensing, signaling and regulation of transcription factors. Redox Biol. 2, 535–562. doi: 10.1016/j.redox.2014.02.006
Mcquin, C., Goodman, A., Chernyshev, V., Kamentsky, L., Cimini, B. A., Karhohs, K. W., et al. (2018). CellProfiler 3.0: next-generation image processing for biology. PLoS Biol. 16:e2005970. doi: 10.1371/journal.pbio.2005970
Muraglia, A., Nguyen, V. T., Nardini, M., Mogni, M., Coviello, D., Dozin, B., et al. (2017). Culture medium supplements derived from human platelet and plasma: cell commitment and proliferation support. Front. Bioeng. Biotechnol. 5, 66–66. doi: 10.3389/fbioe.2017.00066
Nedić, S., Vakanjac, S., SamardŽija, M., and Borozan, S. (2019). Paraoxonase 1 in bovine milk and blood as marker of subclinical mastitis caused by Staphylococcus aureus. Res. Vet. Sci. 125, 323–332. doi: 10.1016/j.rvsc.2019.07.016
Olayanju, A., Copple, I. M., Bryan, H. K., Edge, G. T., Sison, R. L., Wong, M. W., et al. (2015). Brusatol provokes a rapid and transient inhibition of Nrf2 signaling and sensitizes mammalian cells to chemical toxicity-implications for therapeutic targeting of Nrf2. Free Radic. Biol. Med. 78, 202–212. doi: 10.1016/j.freeradbiomed.2014.11.003
Osorio, J. S., and Bionaz, M. (2017). Plasmid transfection in bovine cells: optimization using a realtime monitoring of green fluorescent protein and effect on gene reporter assay. Gene 626, 200–208. doi: 10.1016/j.gene.2017.05.025
Paguio, A., Stecha, P., Wood, K. V., and Fan, F. (2010). Improved dual-luciferase reporter assays for nuclear receptors. Curr. Chem. Genomics 4, 43–49. doi: 10.2174/1875397301004010043
Pinz, S., Unser, S., and Rascle, A. (2014). The natural chemopreventive agent sulforaphane inhibits STAT5 activity. PLoS One 9:e99391. doi: 10.1371/journal.pone.0099391
Pubchem. Pubchem Tert-Butylhydroquinone. Available online at: https://pubchem.ncbi.nlm.nih.gov/compound/tert-butyl-hydroquinone (accessed February 2, 2021).
Roche, M., Rondeau, P., Singh, N. R., Tarnus, E., and Bourdon, E. (2008). The antioxidant properties of serum albumin. FEBS Lett. 582, 1783–1787. doi: 10.1016/j.febslet.2008.04.057
Sestili, P., and Fimognari, C. (2015). Cytotoxic and antitumor activity of sulforaphane: the role of reactive oxygen species. Biomed. Res. Int. 2015:402386. doi: 10.1155/2015/402386
Sies, H. (1997). Oxidative stress: oxidants and antioxidants. Exp. Physiol. 82, 291–295. doi: 10.1113/expphysiol.1997.sp004024
Singh, A., Venkannagari, S., Oh, K. H., Zhang, Y. Q., Rohde, J. M., Liu, L., et al. (2016). Small molecule inhibitor of NRF2 selectively intervenes therapeutic resistance in KEAP1-deficient NSCLC tumors. ACS Chem. Biol. 11, 3214–3225. doi: 10.1021/acschembio.6b00651
Sohel, M. M. H., Amin, A., Prastowo, S., Linares-Otoya, L., Hoelker, M., Schellander, K., et al. (2018). Sulforaphane protects granulosa cells against oxidative stress via activation of NRF2-ARE pathway. Cell Tissue Res. 374, 629–641. doi: 10.1007/s00441-018-2877-z
Sordillo, L. M., and Aitken, S. L. (2009). Impact of oxidative stress on the health and immune function of dairy cattle. Vet. Immunol. Immunopathol. 128, 104–109. doi: 10.1016/j.vetimm.2008.10.305
Taguchi, K., Motohashi, H., and Yamamoto, M. (2011). Molecular mechanisms of the Keap1-Nrf2 pathway in stress response and cancer evolution. Genes Cells 16, 123–140. doi: 10.1111/j.1365-2443.2010.01473.x
Tan, Y., Wan, H., Sun, M., Zhang, W., Dong, M., Ge, W., et al. (2020). Cardamonin protects against lipopolysaccharide-induced myocardial contractile dysfunction in mice through Nrf2-regulated mechanism. Acta Pharmacol. Sinica 1–10.
Vartanian, S., Ma, T. P., Lee, J., Haverty, P. M., Kirkpatrick, D. S., Yu, K., et al. (2016). Application of mass spectrometry profiling to establish brusatol as an inhibitor of global protein synthesis. Mol. Cell Proteomics 15, 1220–1231. doi: 10.1074/mcp.M115.055509
Wittmann, C., Chockley, P., Singh, S. K., Pase, L., Lieschke, G. J., and Grabher, C. (2012). Hydrogen peroxide in inflammation: messenger, guide, and assassin. Adv. Hematol. 2012:541471. doi: 10.1155/2012/541471
Xu, T., Cardoso, F. C., Pineda, A., Trevisi, E., Shen, X., Rosa, F., et al. (2017). Grain challenge affects systemic and hepatic molecular biomarkers of inflammation, stress, and metabolic responses to a greater extent in Holstein than Jersey cows. J. Dairy Sci. 100, 9153–9162. doi: 10.3168/jds.2017-13321
Keywords: Nrf2, bovine, blood serum, mammary cells, oxidative stress, luciferase, albumin
Citation: Ford HR, Busato S and Bionaz M (2021) In vitro–In vivo Hybrid Approach for Studying Modulation of NRF2 in Immortalized Bovine Mammary Cells. Front. Anim. Sci. 2:674355. doi: 10.3389/fanim.2021.674355
Received: 20 March 2021; Accepted: 27 May 2021;
Published: 21 June 2021.
Edited by:
Pasquale De Palo, University of Bari Aldo Moro, ItalyReviewed by:
Loredana Basiricò, University of Tuscia, ItalyMariangela Caroprese, University of Foggia, Italy
Copyright © 2021 Ford, Busato and Bionaz. This is an open-access article distributed under the terms of the Creative Commons Attribution License (CC BY). The use, distribution or reproduction in other forums is permitted, provided the original author(s) and the copyright owner(s) are credited and that the original publication in this journal is cited, in accordance with accepted academic practice. No use, distribution or reproduction is permitted which does not comply with these terms.
*Correspondence: Massimo Bionaz, bWFzc2ltby5iaW9uYXpAb3JlZ29uc3RhdGUuZWR1