- 1Department of Anesthesiology, Division of Critical Care, Duke University School of Medicine, Durham, NC, United States
- 2Human Pharmacology and Physiology Laboratory (HPPL), Duke University School of Medicine, Durham, NC, United States
- 3Department of Medicine, Division of Cardiology, Duke University School of Medicine, Durham, NC, United States
- 4Department of Intensive Care Adults, Erasmus MC University Medical Center Rotterdam, Rotterdam, Netherlands
- 5Centre for Perioperative Medicine, Department of Targeted Intervention, University College London, London, United Kingdom
- 6Departments of Radiology and Surgery, Duke University Medical Center, Duke Cancer Institute Center for Prostate & Urologic Cancers, Durham, NC, United States
- 7Department of Surgery, Division of Trauma, Acute and Critical Care, Duke University School of Medicine, Durham, NC, United States
- 8Pulmonary, Allergy and Critical Care Medicine, Duke University School of Medicine, Department of Medicine, Durham, NC, United States
- 9Department of Pulmonology and Critical Care, NYU School of Medicine, New York, NY, United States
- 10Deparment of Pulmonology and Critical Care, Columbia University Vagelos College of Physicians and Surgeons, New York, NY, United States
- 11Pontificia Universidad Católica de Chile, Department of Intensive Care, Santiago, Chile
Background: Muscle wasting is a common finding in critically ill patients associated with increased days of mechanical ventilation in the ICU. Muscle wasting and associated morphological changes are hallmarks of ICU-acquired weakness. Muscle wasting can be diagnosed and quantified by muscle biopsy, but biopsies can cause multiple adverse effects. MuscleSound® has developed a non-invasive, real-time novel MusculoSkeletal (MSK) ultrasound approach to measure the ratio between percentage intramuscular adipose tissue (%IMAT) with muscle cross-section area (MCSA) (termed IMAT-Index). The present study aimed to assess the IMAT-Index in ICU patients longitudinally and compare it to age-matched healthy controls.
Methods: Transverse (short-axis) ultrasound images of the rectus femoris muscle were obtained upon admission and discharge in 35 ICU patients and compared to age-matched healthy controls (n = 975). The echo intensity of the image taken from the muscle is used to automatically calculate the ratio between intramuscular adipose tissue by cm2 (IMAT-Index), the cross-sectional area of the muscle (MCSA), and muscle thickness (MT).
Results: IMAT-Index was successfully measured in all subjects. The mean IMAT-index (%IMAT/cm2) upon admission was significantly higher in critically ill patients compared to healthy controls (7.4 ± 4.3 vs. 3.1 ± 0.9, p < 0.001). At ICU discharge, the IMAT-index increased in the ICU group compared to admission (8.1 ± 3.5 vs. 7.4 ± 4.3, p < 0.05).
Conclusion: Real-time noninvasive MSK ultrasound IMAT-Index was higher in ICU patients than in healthy controls and increased during the ICU stay, both in male and female patients.
Introduction
ICU-acquired weakness (ICU-AW) is a significant complication in critically ill patients, characterized by muscle wasting and neuromuscular disorders that develop due to admission to the intensive care unit (ICU). The pathophysiology of ICU-AW is complex and includes an inflammatory response, bioenergetic dysfunction, altered protein balance, neuronal axon degeneration, changes in muscle histology, and muscle wasting (1–4). Impaired muscle protein homeostasis eventually reduces muscle mass and strength, independent survival predictors (2, 5–10). Eighty to a hundred percent of survivors of critical illness still exhibit exercise limitation, decreased physical quality of life, and increased costs and use of health care services after twelve months to even five years (11–13).
A growing body of evidence suggests that the decline in muscle function and physical fitness before, during, and after critical illness results from reduced skeletal muscle mass and muscle quality, including muscle composition and morphology (14–17). Skeletal muscle quality is recognized as a marker of function in healthy individuals and critically ill patients; it is also an emerging descriptor of prognosis characterized by the accumulation of intra- and intermyocellular lipids, defined as myosteatosis. All are associated with altered muscle function, insulin resistance, type 2 diabetes, obesity, and survival in critical illness (7, 15, 18–24).
Body composition alterations with a loss of muscle density and surface, measured by computed tomography (CT) in mechanically ventilated patients, are independently associated with higher 6-month mortality in different populations (10, 16, 25–27). Intramuscular adipose tissue (IMAT) can also be derived from CT and MRI images and correlate well with functional outcomes, physical fitness, and mortality in the elderly and patients undergoing major abdominal surgery (17, 22, 28–30). Muscle protein breakdown, as determined by reduced rectus femoris muscle cross-sectional area (MSCA) and skeletal muscle bioenergetic status, is observed during the first week of critical illness and is more severe in patients with multiorgan failure (7, 8). Muscle quality can also be assessed by quantification of lipid components (IMAT) in the muscle tissue using high-resolution NMR spectroscopy and MRI (21, 29, 31). However, these techniques have limitations because they are minimally invasive, expensive, and mostly unavailable. Thus, there is a need to develop new, fast, noninvasive approaches for muscle analyses. MuscleSound (Denver, CO, USA) developed a real-time, noninvasive novel AI MusculoSkeletal (MSK) ultrasound approach to determine IMAT-Index, quickly triage patients in ICU, assess their risk, and set new standards in patient personalized care.
MSK ultrasound has become an important tool for assessing muscle composition in both health and disease (32, 33). It is cost-effective, non-invasive, and sensitive to changes over time compared to other assessments such as muscle biopsy, CT, or NMR/MRI (25, 34–42). Ultrasound imaging is based on the fundamental principle that ultrasound waves are reflected by tissue in the path of the ultrasound beam. Differences in acoustic impedance between tissues determine the amount of sound reflected. The amount of sound reflected is determined by the product of tissue density and acoustic velocity (43, 44). Healthy muscle is predominantly hypoechoic, with only scattered small hyperechoic regions, resulting in a “starry night” appearance (40, 45, 46). Muscle wasting increases intramuscular adipose tissue (IMAT), fibrosis, myonecrosis, and effusion surrounding the fascicles (7, 8, 47). The reflection of ultrasonic waves at the tissue interface level results in diffuse backscattering which can be quantified as the number of intramuscular tissue interactions within each muscle of interest (45, 46, 48–50). Muscles with high intramuscular fat infiltration (IMAT) have predominantly hyperechoic appearances, with only minor hypoechoic regions (51–53).
Equations intended to quantify and translate muscle echo Intensity into percent intramuscular adipose tissue (%IMAT) on the rectus femoris muscle were introduced by Young et al. through a comparison of intramuscular adipose tissue (IMAT) determined from T1-weighted MRI image with ultrasound images of the rectus femoris muscle (54). Historically, these determinations of echo intensity were made manually using imaging software such as Image-J (https://imagej.nih.gov/ij/). More recently, these equations have been incorporated into an automated dicom (image) analysis algorithm as part of MuscleSound® (Denver, CO, USA) Technology. MuscleSound® (Denver, CO, USA) dicom (image) analysis uses proprietary software to identify muscle and fat boundaries and analyze muscle composition using the principles, calibration equations, and algorithms outlined above (51). The technology has been extensively used in healthy individuals, including athletes, where temporal changes in muscle composition, including intramuscular glycogen content, have been described and validated by muscle biopsies (55, 56). Research has shown inconsistent results regarding muscle wasting and composition with functional outcomes during ICU stay (7). Therefore, there is a need for valid and reliable measures of skeletal muscle quality (57). This study aimed to use ultrasound in critically ill patients to assess IMAT and cross-sectional muscle size (MSCA) longitudinally in the rectus femoris muscle.
Methods
This longitudinal observational feasibility study was conducted at the ICU department of the Erasmus Medical Centre (MC) in Rotterdam, the Netherlands. The Erasmus MC's Medical Ethical Committee approved the study. The patients’ legal representatives gave written informed consent when the patient was unable to give consent (October 2,016—July 2017).
All adult patients admitted to the ICU between the period of October 2016 and July 2017 (8 months) were eligible for inclusion, and no exclusion criteria were set. The chosen sample size of n = 35 for this observational longitudinal study is based on the recommendations of Kiesser and Wassmer (58). Patients’ data were compared with healthy controls. The authors analyzed a secondary de-identified dataset obtained via secure electronic means from MuscleSound® (Denver, CO, USA). The dataset contained anthropometric and MSK-ultrasound data of a reference population comprising healthy adult volunteers of multiple age groups. Each subject signed an authorization for disclosure of Protected Health Information (PHI) to MuscleSound® and granted MuscleSound® authorization to access PHI as necessary for research purposes. The reference population was recruited from facilities such as, but not limited to, healthcare clinics, university labs, academic research centers, fitness training centers, and physical therapy centers. Only subjects who were self-declared to be in their baseline state of health and not admitted to the hospital at the time of assessment were included in the dataset. Assessors trained and certified by MuscleSound obtained MSK-ultrasound parameters of the RFM. US examinations were performed in the morning and before training.
The imaging protocol used a linear array transducer (Philips Lumify L12-4, frequency range 4–12 MHz) in the factory musculoskeletal (MSK) preset mode, with standard gain at 50% and depth set at 3.5 cm. Subjects were placed supine with knees at 10–15o and in neutral rotation if possible. The image was taken at the midpoint of the estimated femoral craniocaudal diameter (in inches), calculated by the revised Steele method (with height in inches) (59):
The obtained distance was measured from the upper border of the patella, indicating the precise point of insonation. The calibration equations and algorithms were optimized and corrected for the use of the Philips Lumify ultrasound device. A minimum of two short-axis scan images were taken bilaterally. The images were assessed for inconsistency in the fat/muscle area and fat/muscle depth. The corresponding image was not used in the analyses if the variance was high due to inconsistent scanning position and pressure. Short-axis high-resolution B-mode DICOM images were recorded and uploaded to the MuscleSound® analytic portal for online analysis by its proprietary algorithms (see Figure 1).
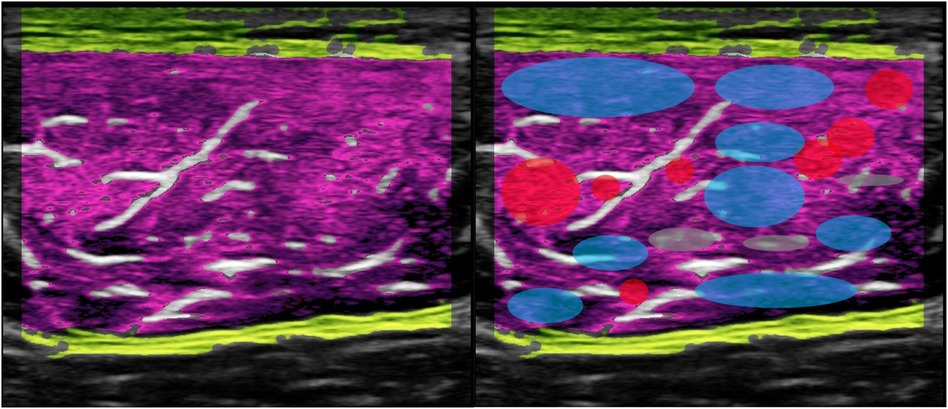
Figure 1. Echo-Intensity (EI) heatmap MuscleSound® IMAT-Index, healthy control (male); red dots (neon pink) = high EI, blue dots (dark purple) =low IE, white: connective tissue/aponeurosis, yellow = fascia. M. rectus femoris.
All measurements were done by one experienced researcher (JM). Images were taken at baseline within 48 h after ICU admission and 12 h before discharge. In the controls, all images were taken simultaneously every day for three consecutive days using the same scanning protocol used in the ICU patients.
All images from both legs were analyzed by MuscleSound® (Denver, CO, USA) algorithm, an FDA-registered automated MSK ultrasound AI analytics platform, for inconsistency using a proprietary algorithm from Young et al. (51).
The MuscleSound® algorithm determined if the variance was too high due to inconsistent scanning position or pressure; the corresponding image was not used in the analyses. The analyses did not use an average of 16% of all images due to high variance. The MuscleSound® algorithm identifies and crops the muscle (Figure 1) of interest automatically and calculates the muscle thickness (MT), muscle cross-sectional area (MCSA), and%IMAT. Combining%IMAT with MCSA produces the IMAT-Index (%IMAT/cm2). IMAT-Index will give insight into the combined changes of%IMAT and MCSA. A compromised metabolic muscle phenotype will show a higher%IMAT with a reduced MCSA, which results in an elevated IMAT-Index.
Assessing IMAT-Index, muscle thickness, and area have been reported to have high reliability (intraclass correlation coefficients [1.1] = 0.857–0.959 (60).
Descriptive statistics of baseline characteristics and ultrasound measurements of muscle were performed, and the data were assessed for normality using the Shapiro-Wilk test (W = 0.87, p > 0.05). Analyses were conducted using the student's t-test and the Pearson correlation coefficient (r-value). A p-value < 0.05 was considered statistically significant. Results are given for all patients and controls and men and women, as there is a known difference in IMAT-index between sexes (51). Statistical analyses were performed in PRISM 10.0.
Results
Thirty-five patients and a dataset of healthy controls were included in this study. Baseline characteristics of the patients are shown in Table 1. The control group consisted of 975 adults (369 women (38%) and 606 men (62%), mean age 34 (SD ± 14.0) years, Body Mass Index (BMI) 24.9 (SD ± 10.7)). The patient group comprised 13 women (37%) and 22 men (63%). All patient's mean age was 41.9 (SD ± 18.8) years, and they had a BMI of 24.7 (SD ± 4.1); age was statistically significantly (p < 0.001) higher in the patients group.
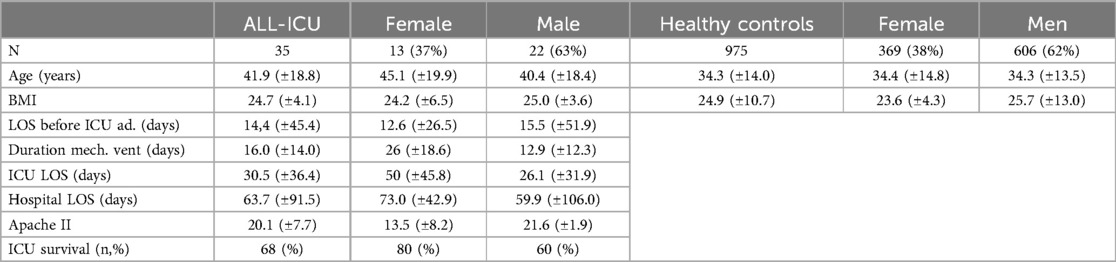
Table 1. Baseline characteristics patients: all-ICU female and male/healthy controls female and male. Data are given as standard deviations (SD).
Reasons for ICU admission were acute respiratory distress syndrome (ARDS) (40%), sepsis (29%), neurotrauma (17%), heart- and lung transplant (8%), and left ventricular assisted device (LVAD)(6%) insertion. Co-morbidities were seen in 20% of all patients: 3 patients with hypertension, 3 patients with diabetes type II, 2 patients with morbid obesity, 2 patients with COPD, and 1 patient with ischemic heart disease. Renal replacement therapy was done in 10 patients (29%). Hydrocortisone was given to 6 patients (17%) upon admission. All patients were lung- and heart-transplant and received physiotherapy (early mobilization) as the standard of care. ICU mortality was 32%.
All patients have significantly (p < 0.001) lower MCSA and MT both at admission and discharge than healthy controls (Table 2). IMAT-Index was significantly higher (p < 0.001) in patients at admission and discharge compared to aged-matched healthy controls. During ICU stay, there was a significant (p < 0.05) increase in IMAT-Index comparing ICU All at admission to discharge. Tables 3 and 4. show the results dependent on sex, as MCSA and MT were significantly higher in males than in females’ controls (p < 0.001) and patients at admission (p < 0.05). Similar to males, female patients also have a significantly lower%IMAT and IMAT-Index than female controls (Table 3). During the ICU stay, there was a slight decrease in MCSA and MT and an increase in IMAT-Index, not reaching the level of statistical significance in all groups. Between male patients at admission and healthy controls, numerous significant differences were found MT (p < 0.001), MCSA (p < 0.001), and IMAT-Index (p < 0.001) (Table 4). IMAT-Index assessed upon admission correlated low (r = 0.51) though significantly (p < 0.05) with ICU length of stay. The change of IMAT-Index over time is reflected by the finding that IMAT-Index at discharge correlated (r = 0.58) significantly (p < 0.05) with total length of stay in the hospital, and there was a high correlation between IMAT-Index at discharge and duration of mechanical ventilation (r = 0.81) (p < 0.05). In the ICU group, a high correlation (r = 0.84) (P < 0.05) was seen between IMAT-Index upon admission and APACHE II scores.
Discussion
This study demonstrates that ultrasound in critically ill patients can measure muscle quality in the ICU. Muscle quality was defined as the ratio between%IMAT and MCSA, resulting in IMAT-Index. Differences in muscle quality were observed between critically ill patients and controls in both male and female groups. The method employed also facilitated the description of temporal changes in IMAT-index among critically ill patients upon admission and discharge.
IMAT-Index was significantly higher in patients upon admission than controls and further increased during ICU stay. There was no significant difference in BMI between the male controls and the male ICU group, nor was there any significant difference between the female groups. A possible explanation of the difference found in IMAT-Index between the control group and the ICU group upon admission lies in the older average age of the ICU patients and the presence of comorbidities, which could have affected the muscle metabolic profiles. Also, the length of hospital stay prior to admission to the ICU could potentially have resulted in higher values of IMAT-Index and lower values of MCSA and MT (61, 62).
We did find a weak but significant correlation between IMAT-Index measured upon admission and ICU length of stay, suggesting that there are differences in patients in which patients with a low IMAT-Index at admission are less vulnerable for extended ICU stay than those with a high IMAT-Index, but this was not an endpoint of this study and warrants further research.
The significant increased IMAT-Index has been described previously during critical care admission (7, 8). Puthucheary et al. found that intramuscular lipid accumulation, assessed by muscle biopsies, results in dysregulated lipid oxidation and reduced ATP bioavailability, contributing to a compromised skeletal muscle bioenergetic status (7). Thus, the accumulation of intramuscular lipids, and therefore increased IMAT-Index during ICU stay combined with the decrease of MCSA, indicates a compromised skeletal muscle metabolic status, which is synonymous with acute mitochondrial dysfunction and even perturbed regeneration (7, 8, 63). The hypoxic or inflammatory stimuli associated with critical illness impair muscle protein synthesis and replacement of the functional muscle tissue with IMAT due to reduced mitochondrial biogenesis (63–65). This might also explain our finding of a significant correlation between IMAT-Index at discharge and length of mechanical ventilation (r = .58) (p < 0.05) and hospital stay (r = .81) (p < 0.05). The systemic inflammatory response gives rise to skeletal muscle wasting within 24 h upon admission to the ICU. Acute skeletal muscle wasting occurs early and is rapidly defined as the reduction in mitochondrial beta-oxidation, mitochondrial biogenesis markers, and intramuscular ATP content (7). This could be explanatory of why the IMAT-index upon admission correlates highly (r = .84) (p < 0.05) with the APACHE II score. A high IMAT-index is also correlated with decreased functional performances like muscle strength, speed, agility, and cardiorespiratory fitness in adults and children (17, 66–68).
One limitation of the work carried out by Young et al., which forms the basis for IMAT estimations in the MuscleSound® (Denver, CO, USA) algorithm, is that IMAT-Index validations were not taken in a clinical population with known skeletal muscle abnormalities such as COPD, CHF, and neuromuscular diseases (51). Further, all ultrasound images were only taken from the rectus femoris muscle. This muscle group may respond differently during critical illness regarding MT, MCSA, and IMAT-Index than other muscle groups. We also need to consider the heterogeneity, and therefore the potential confounding effect, of the population. The muscle metabolic phenotype of ARDS, sepsis, and neurotrauma can differ based on the etiology of the disease state prior to admission.
The limiting factor of the IMAT-Index algorithm is not being able to distinguish between muscular lipids stored either in interstitial adipose tissue (extramyocellular lipid) or in lipid droplets within muscle cells (intramyocellular lipid). The high content of intramyocellular lipids is primarily associated with obesity, type 2 diabetes, and the development of insulin resistance (54, 69–71). Further studies are necessary to confirm the temporal changes described in this study in a more extensive and better-defined population and their further role in recovering ICU-acquired muscle weakness. Validation of%IMAT and IMAT-Index in the clinical population is needed. Comparative analysis of ultrasound images with CT images will be the next step.
Conclusion
Demonstrating feasibility, MSK ultrasound was employed to quantify muscle quality, specifically IMAT-Index, sequentially in critically ill patients. Significant differences in IMAT-Index were observed between healthy controls and patients, as well as between patients at admission and discharge. Muscle wasting in critical illness serves as a marker of systemic metabolic health and prognosis. Prioritizing the ongoing development of clinically relevant techniques to assess muscle phenotype in intensive care is crucial for understanding the critical illness phenotype and monitoring disease and therapeutic trajectories.
Data availability statement
The raw data supporting the conclusions of this article will be made available by the authors, without undue reservation.
Ethics statement
The studies involving humans were approved by Erasmus MC Medical Ethics Review Committee (MERC). The studies were conducted in accordance with the local legislation and institutional requirements. The participants provided their written informed consent to participate in this study.
Author contributions
JM: Writing – original draft, Writing – review & editing. JW: Conceptualization, Formal Analysis, Supervision, Writing – review & editing. HE: Formal Analysis, Methodology, Supervision, Writing – review & editing. DM: Writing – review & editing. RG: Writing – review & editing. MF: Writing – review & editing. KH: Writing – review & editing. CB: Writing – review & editing. ZH: Writing – review & editing. PW: Supervision, Writing – review & editing. JB: Supervision, Writing – review & editing.
Funding
The author(s) declare financial support was received for the research, authorship, and/or publication of this article. This study was PI-initiated and internally funded in the Erasmus Medical Center Rotterdam. MuscleSound did not participate in the design and conduct of the study; collection, management, analysis, and interpretation of the data; preparation, review, or approval of the manuscript; and decision to submit the manuscript for publication. Only the authors and investigators at the Erasmus Medical Center Rotterdam and Duke University participated in design and conduct of the study; collection, management, analysis, and interpretation of the data; preparation, review, or approval of the manuscript; and decision to submit the manuscript for publication.
Conflict of interest
The authors declare that the research was conducted in the absence of any commercial or financial relationships that could be construed as a potential conflict of interest.
Publisher's note
All claims expressed in this article are solely those of the authors and do not necessarily represent those of their affiliated organizations, or those of the publisher, the editors and the reviewers. Any product that may be evaluated in this article, or claim that may be made by its manufacturer, is not guaranteed or endorsed by the publisher.
Abbreviations
IMAT, intramuscular adipose tissue; %IMAT, percentage Intramuscular adipose tissue; MT, muscle thickness; MCSA, muscle cross-sectional area; IMAT-Index, ratio%IMAT and MCSA; CT, computed tomography, NMR, nuclear magnetic resonance; MRI, magnetic resonance imaging; LVAD, left ventricular assisted device; ARDS, acute respiratory distress syndrome; MSK, musculoskeletal.
References
1. Fazzini B, Markl T, Costas C, Blobner M, Schaller SJ, Prowle J, et al. The rate and assessment of muscle wasting during critical illness: a systematic review and meta-analysis. Crit Care. (2023) 27(1):2. doi: 10.1186/s13054-022-04253-0
2. Herridge MS, Cheung AM, Tansey CM, Matte-Martyn A, Diaz-Granados N, Al-Saidi F, et al. One-year outcomes in survivors of the acute respiratory distress syndrome. N Engl J Med. (2003) 348(8):683–93. doi: 10.1056/NEJMoa022450
3. Wollersheim T, Grunow JJ, Carbon NM, Haas K, Malleike J, Ramme SF, et al. Muscle wasting and function after muscle activation and early protocol-based physiotherapy: an explorative trial. J Cachexia Sarcopenia Muscle. (2019) 10(4):734–47. doi: 10.1002/jcsm.12428
4. Batt J, Herridge M, Dos Santos C. Mechanism of ICU-acquired weakness: skeletal muscle loss in critical illness. Intensive Care Med. (2017) 43(12):1844–6. doi: 10.1007/s00134-017-4758-4
5. Puthucheary ZA, Phadke R, Rawal J, McPhail MJ, Sidhu PS, Rowlerson A, et al. Qualitative ultrasound in acute critical illness muscle wasting. Crit Care Med. (2015) 43(8):1603–11. doi: 10.1097/CCM.0000000000001016
6. Puthucheary Z, Montgomery H, Moxham J, Harridge S, Hart N. Structure to function: muscle failure in critically ill patients. J Physiol (Lond). (2010) 588(23):4641–8. doi: 10.1113/jphysiol.2010.197632
7. Puthucheary ZA, Astin R, McPhail MJW, Saeed S, Pasha Y, Bear DE, et al. Metabolic phenotype of skeletal muscle in early critical illness. Thorax. (2018) 73(10):926–35. doi: 10.1136/thoraxjnl-2017-211073
8. Puthucheary ZA, Rawal J, McPhail M, Connolly B, Ratnayake G, Chan P, et al. Acute skeletal muscle wasting in critical illness. JAMA. (2013) 310(15):1591–600. doi: 10.1001/jama.2013.278481
9. Nakanishi N, Oto J, Tsutsumi R, Iuchi M, Onodera M, Nishimura M. Upper and lower limb muscle atrophy in critically ill patients: an observational ultrasonography study. Intensive Care Med. (2018) 44(2):263–4. doi: 10.1007/s00134-017-4975-x
10. Weijs P, Looijaard WGPM, Dekker IM, Stapel SN, Girbes AR, Oudemans-van Straaten HM, et al. Low skeletal muscle area is a risk factor for mortality in mechanically ventilated critically ill patients-annotated. Crit Care. (2014) 18:R12. doi: 10.1186/cc13189
11. McNelly AS, Rawal J, Shrikrishna D, Hopkinson NS, Moxham J, Harridge SD, et al. An exploratory study of long-term outcome measures in critical illness survivors: construct validity of physical activity, frailty, and health-related quality of life measures. Crit Care Med. (2016) 44(6):e362–369. doi: 10.1097/CCM.0000000000001645
12. Silveira L, Silva JMD, Tanaka C, Fu C. Decline in functional status after intensive care unit discharge is associated with ICU readmission: a prospective cohort study. Physiotherapy. (2019) 105(3):321–7. doi: 10.1016/j.physio.2018.07.010
13. Herridge MS, Tansey CM, Matté A, Tomlinson G, Diaz-Granados N, Cooper A, et al. Functional disability 5 years after acute respiratory distress syndrome. N Engl J Med. (2011) 364(14):1293–304. doi: 10.1056/NEJMoa1011802
14. Correa-de-Araujo R, Harris-Love MO, Miljkovic I, Fragala MS, Anthony BW, Manini TM. The need for standardized assessment of muscle quality in skeletal muscle function deficit and other aging-related muscle dysfunctions: a symposium report. Front Physiol. (2017) 8:87. doi: 10.3389/fphys.2017.00087
15. Fragala MS, Kenny AM, Kuchel GA. Muscle quality in aging: a multi-dimensional approach to muscle functioning with applications for treatment. Sports Med. (2015) 45(5):641–58. doi: 10.1007/s40279-015-0305-z
16. Looijaard WG, Dekker IM, Stapel SN, Girbes AR, Twisk JW, Oudemans-van Straaten HM, et al. Skeletal muscle quality as assessed by CT-derived skeletal muscle density is associated with 6-month mortality in mechanically ventilated critically ill patients. Crit Care. (2016) 20(1):386. doi: 10.1186/s13054-016-1563-3
17. West MA, van Dijk DPJ, Gleadowe F, Reeves T, Primrose JN, Abu Hilal M, et al. Myosteatosis is associated with poor physical fitness in patients undergoing hepatopancreatobiliary surgery. J Cachexia Sarcopenia Muscle. (2019) 10(4):860–71. doi: 10.1002/jcsm.12433
18. Watanabe Y, Yamada Y, Fukumoto Y, Yokoyama K, Yoshida T, Miyake M, et al. Echo intensity obtained from ultrasonography images reflecting muscle strength in elderly men. Clin Interv Aging. (2013) 8:993–8. doi: 10.2147/CIA.S47263
19. Sieber CC. Frailty—from concept to clinical practice. Exp Gerontol. (2017) 87(Pt B):160–7. doi: 10.1016/j.exger.2016.05.004
20. Kelley GA, Kelley KS. Is sarcopenia associated with an increased risk of all-cause mortality and functional disability? Exp Gerontol. (2017) 96:100–3. doi: 10.1016/j.exger.2017.06.008
21. Hu HH, Chen J, Shen W. Segmentation and quantification of adipose tissue by magnetic resonance imaging. Magnt Reson Mater Phys Biol Med. (2015) 29(2):259–76. doi: 10.1007/s10334-015-0498-z
22. Czigany Z, Kramp W, Bednarsch J, van der Kroft G, Boecker J, Strnad P, et al. Myosteatosis to predict inferior perioperative outcome in patients undergoing orthotopic liver transplantation. Am J Transplant. (2020) 20(2):493–503. doi: 10.1111/ajt.15577
23. Miljkovic I, Kuipers AL, Cauley JA, Prasad T, Lee CG, Ensrud KE, et al. Greater skeletal muscle fat infiltration is associated with higher all-cause and cardiovascular mortality in older men. J Gerontol A Biol Sci Med Sci. (2015) 70(9):1133–40. doi: 10.1093/gerona/glv027
24. Berger J, Bunout D, Barrera G, de la Maza MP, Henriquez S, Leiva L, et al. Rectus femoris (RF) ultrasound for the assessment of muscle mass in older people. Arch Gerontol Geriatr. (2015) 61(1):33–8. doi: 10.1016/j.archger.2015.03.006
25. Looijaard W, Molinger J, Weijs PJM. Measuring and monitoring lean body mass in critical illness. Curr Opin Crit Care. (2018) 24(4):241–7. doi: 10.1097/MCC.0000000000000511
26. Dusseaux MM, Antoun S, Grigioni S, Beduneau G, Carpentier D, Girault C, et al. Skeletal muscle mass and adipose tissue alteration in critically ill patients. PLoS One. (2019) 14(6):e0216991. doi: 10.1371/journal.pone.0216991
27. Ng CC, Lee ZY, Chan WY, Jamaluddin MF, Tan LJ, Sitaram PN, et al. Low muscularity as assessed by abdominal computed tomography on intensive care unit admission is associated with mortality in a critically ill Asian population. JPEN J Parenter Enteral Nutr. (2020) 44(3):425–33. doi: 10.1002/jpen.1666
28. Hahn MH, Won YY. Bone mineral density and fatty degeneration of thigh muscles measured by computed tomography in hip fracture patients. J Bone Metab. (2016) 23(4):215–21. doi: 10.11005/jbm.2016.23.4.215
29. Shenvi SD, Taber DJ, Hardie AD, Botstein JO, McGillicuddy JW. Assessment of magnetic resonance imaging derived fat fraction as a sensitive and reliable predictor of myosteatosis in liver transplant recipients. HPB (Oxford). (2020) 22(1):102–8. doi: 10.1016/j.hpb.2019.06.006
30. Zopfs D, Theurich S, Grosse Hokamp N, Knuever J, Gerecht L, Borggrefe J, et al. Single-slice CT measurements allow for accurate assessment of sarcopenia and body composition. Eur Radiol. (2020) 30(3):1701–8. doi: 10.1007/s00330-019-06526-9
31. Srivastava NK, Yadav R, Mukherjee S, Pal L, Sinha N. Abnormal lipid metabolism in skeletal muscle tissue of patients with muscular dystrophy: in vitro, high-resolution NMR spectroscopy based observation in early phase of the disease. Magn Reson Imaging. (2017) 38:163–73. doi: 10.1016/j.mri.2017.01.001
32. Mourtzakis M, Wischmeyer P. Bedside ultrasound measurement of skeletal muscle. Curr Opin Clin Nutr Metab Care. (2014) 17(5):389–95. doi: 10.1097/MCO.0000000000000088
33. Milan S, Hill JC, Wischmeyer P. 35th International symposium on intensive care and emergency medicine. Crit Care. (2015) 19 Suppl 1(Suppl 1):P1–P578.25860163
34. Joskova V, Patkova A, Havel E, Najpaverova S, Uramova D, Kovarik M, et al. Critical evaluation of muscle mass loss as a prognostic marker of morbidity in critically ill patients and methods for its determination. J Rehabil Med. (2018) 50(8):696–704. doi: 10.2340/16501977-2368
35. Rustani K, Kundisova L, Capecchi PL, Nante N, Bicchi M. Ultrasound measurement of rectus femoris muscle thickness as a quick screening test for sarcopenia assessment. Arch Gerontol Geriatr. (2019) 83:151–4. doi: 10.1016/j.archger.2019.03.021
36. Parry SM, Burtin C, Denehy L, Puthucheary ZA, Bear D. Ultrasound evaluation of quadriceps muscle dysfunction in respiratory disease. Cardiopulm Phys Ther J. (2019) 30(1):15–23. doi: 10.1097/CPT.0000000000000102
37. Hobson-Webb LD, Simmons Z. Ultrasound in the diagnosis and monitoring of amyotrophic lateral sclerosis: a review. Muscle Nerve. (2019) 60(2):114–23. doi: 10.1002/mus.26487
38. Ramírez-Fuentes C, Mínguez-Blasco P, Ostiz F, Sánchez-Rodríguez D, Messaggi-Sartor M, Macías R, et al. Ultrasound assessment of rectus femoris muscle in rehabilitation patients with chronic obstructive pulmonary disease screened for sarcopenia: correlation of muscle size with quadriceps strength and fat-free mass. Eur Geriatr Med. (2018) 10(1):89–97. doi: 10.1007/s41999-018-0130-7
39. Williams DGA, Molinger J, Wischmeyer PE. The malnourished surgery patient: a silent epidemic in perioperative outcomes? Curr Opin Anaesthesiol. (2019) 32(3):405–11. doi: 10.1097/ACO.0000000000000722
40. Formenti P, Umbrello M, Coppola S, Froio S, Chiumello D. Clinical review: peripheral muscular ultrasound in the ICU. Ann Intensive Care. (2019) 9(1):57. doi: 10.1186/s13613-019-0531-x
41. Bear DE, Hart N, Puthucheary Z. Continuous or intermittent feeding: pros and cons. Curr Opin Crit Care. (2018) 24(4):256–61. doi: 10.1097/MCC.0000000000000513
42. Nijholt W, Beek LT, Hobbelen JSM, van der Vaart H, Wempe JB, van der Schans CP, et al. The added value of ultrasound muscle measurements in patients with COPD: an exploratory study. Clin Nutr ESPEN. (2019) 30:152–8. doi: 10.1016/j.clnesp.2019.01.001
43. Wagner DR. Ultrasound as a tool to assess body fat. J Obes. (2013) 2013:280713. doi: 10.1155/2013/280713
44. Pigula-Tresansky AJ, Wu JS, Kapur K, Darras BT, Rutkove SB, Anthony BW. Muscle compression improves reliability of ultrasound echo intensity. Muscle Nerve. (2018) 57(3):423–9.28833292
45. Simon NG, Noto Y-I, Zaidman CM. Skeletal muscle imaging in neuromuscular disease. J Clin Neurosci. (2016) 33:1–10. doi: 10.1016/j.jocn.2016.01.041
46. Fay B. Ultrasonic backscattering: fundamentals and applications. In: Leroy O, Breazeale MA, editors. Physical Acoustics: Fundamentals and Applications. Boston, MA: Springer US (1991). p. 41–53.
47. Phadke R, Puthucheary Z, Rawal J, McPhail M, Sidhu P, Rowlerson A, et al. Fasciitis frequently accompanies myopathy in acute critical illness muscle wasting: evidence from qualitative ultrasound and muscle biopsy analysis. Neuromuscul Disord. (2015) 25(2):S250. doi: 10.1016/j.nmd.2015.06.237
48. Pillen S, Tak RO, Zwarts MJ, Lammens MM, Verrijp KN, Arts IM, et al. Skeletal muscle ultrasound: correlation between fibrous tissue and echo intensity. Ultrasound Med Biol. (2009) 35(3):443–6. doi: 10.1016/j.ultrasmedbio.2008.09.016
49. Cheeke D, Finch RD. Fundamentals and applications of ultrasonic waves. J Acoust Soc Am. (2003) 113(1):14–5. doi: 10.1121/1.1527963
50. Zaidman CM, Holland MR, Hughes MS. Quantitative ultrasound of skeletal muscle: reliable measurements of calibrated muscle backscatter from different ultrasound systems. Ultrasound Med Biol. (2012) 38(9):1618–25. doi: 10.1016/j.ultrasmedbio.2012.04.020
51. Young HJ, Jenkins NT, Zhao Q, McCully KK. Measurement of intramuscular fat by muscle echo intensity. Muscle Nerve. (2015) 52(6):963–71. doi: 10.1002/mus.24656
52. Khoury V, Cardinal E, Brassard P. Atrophy and fatty infiltration of the supraspinatus muscle: sonography versus MRI. AJR Am J Roentgenol. (2008) 190(4):1105–11. doi: 10.2214/AJR.07.2835
53. Soliman SB, Rosen KA, Williams PC, Spicer PJ, Williams LK, Rao SD, et al. The hyperechoic appearance of the deltoid muscle on shoulder ultrasound imaging as a predictor of diabetes and prediabetes. J Ultrasound Med. (2020) 39(2):323–9. doi: 10.1002/jum.15110
54. Hasegawa N, Kurihara T, Sato K, Homma T, Fujie S, Fujita S, et al. Intramyocellular and extramyocellular lipids are associated with arterial stiffness. Am J Hypertens. (2015) 28(12):1473–9. doi: 10.1093/ajh/hpv041
55. Hill JC, Millan IS. Validation of musculoskeletal ultrasound to assess and quantify muscle glycogen content. A novel approach. Phys Sportsmed. (2014) 42(3):45–52. doi: 10.3810/psm.2014.09.2075
56. Nieman DC, Shanely RA, Zwetsloot KA, Meaney MP, Farris GE. Ultrasonic assessment of exercise-induced change in skeletal muscle glycogen content. BMC Sports Sci Med Rehabil. (2015) 7:9. doi: 10.1186/s13102-015-0003-z
57. Mourtzakis M, Parry S, Connolly B, Puthucheary Z. Skeletal muscle ultrasound in critical care: a tool in need of translation. Ann Am Thorac Soc. (2017) 14(10):1495–503. doi: 10.1513/AnnalsATS.201612-967PS
58. Whitehead AL, Julious SA, Cooper CL, Campbell MJ. Estimating the sample size for a pilot randomised trial to minimise the overall trial sample size for the external pilot and main trial for a continuous outcome variable. Stat Methods Med Res. (2016) 25(3):1057–73. doi: 10.1177/0962280215588241
59. Simmons T, Jantz RL, Bass WM. Stature estimation from fragmentary femora: a revision of the steele method. J Forensic Sci. (1990) 35(3):628–36. doi: 10.1520/JFS12868J
60. Akazawa N, Harada K, Okawa N, Tamura K, Hayase A, Moriyama H. Relationships between muscle mass, intramuscular adipose and fibrous tissues of the quadriceps, and gait independence in chronic stroke survivors: a cross-sectional study. Physiotherapy. (2018) 104(4):438–45. doi: 10.1016/j.physio.2017.08.009
61. Pagano AF, Brioche T, Arc-Chagnaud C, Demangel R, Chopard A, Py G. Short-term disuse promotes fatty acid infiltration into skeletal muscle. J Cachexia Sarcopenia Muscle. (2018) 9(2):335–47. doi: 10.1002/jcsm.12259
62. Parry SM, Puthucheary ZA. The impact of extended bed rest on the musculoskeletal system in the critical care environment. Extrem Physiol Med. (2015) 4:16. doi: 10.1186/s13728-015-0036-7
63. Sciorati C, Clementi E, Manfredi AA, Rovere-Querini P. Fat deposition and accumulation in the damaged and inflamed skeletal muscle: cellular and molecular players. Cell Mol Life Sci. (2015) 72(11):2135–56. doi: 10.1007/s00018-015-1857-7
64. Vettor R, Milan G, Franzin C, Sanna M, De Coppi P, Rizzuto R, et al. The origin of intermuscular adipose tissue and its pathophysiological implications. Am J Physiol Endocrinol Metab. (2009) 297(5):E987–998. doi: 10.1152/ajpendo.00229.2009
65. Yang W, Hu P. Skeletal muscle regeneration is modulated by inflammation. J Orthop Translat. (2018) 13:25–32. doi: 10.1016/j.jot.2018.01.002
66. Verwaaijen EJ, van Hulst AM, Molinger J, Hartman A, Pieters R, Grootenhuis MA, et al. The utility of a portable muscle ultrasound in the assessment of muscle alterations in children with acute lymphoblastic leukaemia. J Cachexia Sarcopenia Muscle. (2023) 14(5):2216–25. doi: 10.1002/jcsm.13305
67. García-Alonso Y, García-Hermoso A, Alonso-Martínez AM, Legarra-Gorgoñon G, Izquierdo M, Ramírez-Vélez R. Associations between physical fitness components with muscle ultrasound parameters in prepuberal children. Int J Obes (Lond). (2022) 46(5):960–8. doi: 10.1038/s41366-022-01066-7
68. Wallen MP, Woodward AJ, Hall A, Skinner TL, Coombes JS, Macdonald GA. Poor cardiorespiratory fitness is a risk factor for sepsis in patients awaiting liver transplantation. Transplantation. (2018) 103(3):529–35. doi: 10.1097/TP.0000000000002360
69. Tamura Y, Kakehi S, Takeno K. Intracellular lipid accumulation and insulin sensitivity in muscle and liver: fighting against “intracellular obesity”. J Phys Fit and Sports Med. (2014) 3(5):501–5. doi: 10.7600/jpfsm.3.501
70. Badin PM, Langin D, Moro C. Dynamics of skeletal muscle lipid pools. Trends Endocrinol Metab. (2013) 24(12):607–15. doi: 10.1016/j.tem.2013.08.001
Keywords: IMAT intermuscular adipose tissue, muscle ultrasound, ICU acquired muscle weakness, muscle wasting, muscle weakneess, critical illness
Citation: Molinger J, Whittle J, Endeman H, MacLeod D, Gupta R, Fudim M, Haines KL, Barkauskas C, Healy Z, Wischmeyer P and Bakker J (2024) A novel ultrasound approach in assessing IMAT in critically ill patients. Front. Anesthesiol. 3:1458633. doi: 10.3389/fanes.2024.1458633
Received: 2 July 2024; Accepted: 21 August 2024;
Published: 11 September 2024.
Edited by:
James Bailey, Northeast Georgia Medical Center, United StatesReviewed by:
Francesco Forfori, University of Pisa, ItalyGiuliano Bolondi, Maurizio Bufalini Hospital, Italy
Copyright: © 2024 Molinger, Whittle, Endeman, MacLeod, Gupta, Fudim, Haines, Barkauskas, Healy, Wischmeyer and Bakker. This is an open-access article distributed under the terms of the Creative Commons Attribution License (CC BY). The use, distribution or reproduction in other forums is permitted, provided the original author(s) and the copyright owner(s) are credited and that the original publication in this journal is cited, in accordance with accepted academic practice. No use, distribution or reproduction is permitted which does not comply with these terms.
*Correspondence: Jeroen Molinger, amVyb2VuLm1vbGluZ2VyQGR1a2UuZWR1