- 1Center for Wildlife Health, University of Tennessee Institute of Agriculture, Knoxville, TN, United States
- 2Biological Sciences Department, Salisbury University, Salisbury, MD, United States
- 3Department of Biomedical and Diagnostic Sciences, University of Tennessee, College of Veterinary Medicine, Knoxville, TN, United States
Introduction: Batrachochytrium salamandrivorans (Bsal) poses a major threat to global amphibian biodiversity. It is essential we understand Bsal transmission to develop better-informed management strategies. Infected carcasses are an important source of transmission for several human and wildlife disease systems; however, they have not been examined as sources for Bsal exposure. Here, we evaluated whether infected newt carcasses could contribute to Bsal transmission dynamics.
Methods: We cohoused infected carcasses with susceptible newts in two cohousing chamber types (partitioned or non-partitioned) at three timepoints post-mortem ([0,24[, [24,48, [48,72] hrs). The partitioned chamber prevented newt-to-newt contact hence only allowed indirect, waterborne transmission of zoospores. We measured shedding rates of infected carcasses at each post-mortem timepoint and monitored infection status and mortality of susceptible newts which were exposed during cohousing events.
Results: Our results indicate carcasses are capable of transmitting Bsal to susceptible newts up to at least 72 hrs post-mortem, even without live newts directly contacting carcasses. All susceptible newts in each chamber type and post-mortem period became infected and >90% experienced disease-induced mortality. Bsal genomic copies/uL in skin swabs taken from infected carcasses were high, averaging 7.4x105, 8.6x105, and 2.0x106 at 24, 48, and 72 hrs post-mortem, respectively. Water samples collected from cohousing chambers averaged 2743 Bsal genomic copies/uL (approximately 1357 zoospores) and did not decline over 72 hrs.
Discussion: Our results indicate Bsal infection can occur rapidly between infected carcasses and susceptible aquatic salamanders via indirect and direct transmission pathways, and carcasses may prolong outbreaks by increasing the duration that infected individuals remain infectious. Carcass removal may be a strategy to reduce Bsal transmission and the impacts of outbreaks.
1 Introduction
The application of disease management strategies is context dependent and typically targets the host, environment, pathogen, or combinations thereof. A suite of disease management strategies focuses on reducing pathogen spread within a population (Woodhams et al., 2018). These strategies often attempt to reduce environmental persistence of pathogens or contact rates between hosts (Garner et al., 2016; Woodhams et al., 2018; Thomas et al., 2019). Identifying key transmission pathways is a fundamental step to understanding which management strategies are likely to be most effective at reducing infection and disease in populations (Islam et al., 2021).
Amphibian diseases are one of the leading causes for observed amphibian population declines around the globe (Canessa et al., 2018; Scheele et al., 2019). Given the pronounced effect diseases have on amphibians, successful management of amphibian populations hinges on the effective management of disease. Currently, there are three notifiable amphibian pathogens recognized by the World Organization for Animal Health (OIE), which have been designated as notifiable due to the known threat each poses to amphibians. These notifiable pathogens include viruses in the genus Ranavirus and two chytrid fungus (Batrachochytrium) species. Ranaviruses and Batrachochytrium dendrobatidis (Bd) have caused declines in amphibian populations across the globe for several decades. Recently, a new chytrid fungus (B. salamandrivorans, Bsal) was identified associated with enigmatic declines of salamanders in Europe (Brunner et al., 2015; Olson et al., 2021).
In 2013, Bsal was first described after declines of European Fire Salamanders (Salamandra salamandra) were observed in the Bunderbos region of Netherlands (Annemarieke Spitzen-van der et al., 2013; Martel et al., 2013). Bsal kills a host by creating necrotic ulcerations through the epidermis, thereby allowing bacterial infiltration and interrupting important physiological processes, such as osmoregulation (Bletz et al., 2018; Sheley et al., 2023). Mortality due to Bsal chytridiomycosis can occur less than two weeks post-exposure to the pathogen in highly susceptible hosts (Carter et al., 2021), suggesting that Bsal transmission and epidemics can occur rapidly (Islam et al., 2021).
A key to understanding Bsal epidemiology and devising effective disease management is identifying transmission pathways (Peace et al., 2019; Islam et al., 2021). Bsal can be transmitted directly between infected and susceptible individuals via as little as one second of contact (Malagon et al., 2020), and exposure to motile Bsal zoospores in water can result in transmission and mortality (Carter et al., 2021). For highly susceptible hosts in aquatic ecosystems, such as eastern newts (Notophthalmus viridescens), the functional form of Bsal transmission among all possible pathways is density independent (Tompros et al., 2022). One contributor to density independent transmission is an environmental reservoir for the pathogen. Environmental reservoirs identified for chytrid fungi include alternative hosts or growth substrates, such as invertebrates or decaying organic matter (McMahon et al., 2013; Stegen et al., 2017; Canessa et al., 2019). Recent evidence suggests that Bsal can be saprophytic (Kelly et al., 2021). Thus, it is possible that infected individuals that experience disease-induced mortality could continue to be a source of transmission as a carcass decays or until it is removed from the environment by scavengers (Le Sage et al., 2019). If carcasses are a source of Bsal transmission, it also suggests they could be removed as part of a disease management plan to reduce the effects of an outbreak.
Carcasses have been evaluated for their importance in other disease systems, yet they have not been researched as a source of transmission for either Bd or Bsal. Throughout human history, infected corpses have been suspected sources of disease transmission dating back to bubonic plague outbreaks, although the route of transmission was often incorrectly attributed to miasma released by their decay (Smith, 2013). A more contemporary account of disease spread from corpses has been noted with Ebola outbreaks (Prescott et al., 2015). In non-amphibian wildlife disease systems, carcasses have been recognized as a source of transmission for African swine-fever and chronic wasting disease (Jennelle et al., 2009; Pepin et al., 2020). Transmission of ranaviruses by carcasses can be an important pathway contributing to infection and disease (Brunner et al., 2007; Le Sage et al., 2019; Peace et al., 2019). Given post-mortem transmission of pathogens is known for other disease systems, we designed an experiment to evaluate whether and for how long Bsal could be spread from infected carcasses to uninfected, susceptible individuals through either direct contact or via indirect transmission from the shedding of infectious zoospores. We also estimated zoospore shedding rates from infected carcasses, which do not exist for Bd or Bsal (DiRenzo et al., 2014; Maguire et al., 2016; Islam et al., 2021). We hypothesized that infected carcasses would remain infectious for a short duration (<48 hrs) and lose their ability to shed zoospores, hence contributing minimally to post-mortem Bsal epidemiology.
2 Materials and methods
2.1 Animal housing and sample collection
2.1.1 Ethics statement
Each animal procedure was approved by the University of Tennessee Institutional Animal Care and Use Committee under protocol #2623. All newts were euthanized at humane endpoints, when necessary, via water bath exposure to benzocaine hydrochloride followed by cardiac puncture, unless specified otherwise. Individuals exposed to Bsal were humanely euthanized if they displayed focal lesions on their bodies and exhibited at least one additional sign of clinical disease. These signs include hemorrhage, unresponsiveness, loss of righting ability, or anorexia. Biosafety level-2 procedures were used to ensure pathogen containment within the biosecure facility where experiments were performed.
2.1.2 Model organism
We used eastern newts (Notophthalmus viridescens) as our model organism. This species was selected because it has a broad range across eastern North America, is known to be susceptible to Bsal, and has been used in previous studies as a model organism for Bsal disease dynamics (Martel et al., 2014; Longo et al., 2019; Kumar et al., 2020; Carter et al., 2021). All newts used for each experiment were collected from the wild in wetlands near Crab Orchard, Tennessee, USA under Tennessee Scientific Collection permit #1504.
2.1.3 Bsal transmission from infected carcasses
After collection, newts were transported to the lab where they were heat-treated at 30°C for 10 days to eliminate any preexisting Bd infections (Chatfield and Richards-Zawacki, 2011; Bletz, 2013). Newts were tested following the heat treatment for Bd genomic DNA using a standardized swabbing method for sample collection and quantitative polymerase chain reaction (qPCR) (Blooi et al., 2013), and verified to be negative. Bd coinfections with Bsal could impact survival (Longo et al., 2019); however, prior exposure to Bd does not appear to elicit a secondary immune response to Bsal (Longo et al., 2019). We did not exclude individuals from the study based on other pathogen or parasite infections; however, none were obviously present. Individually housed newts were then randomly assigned to either an infected animal group or one of six susceptible groups. Treatment groups were randomly assigned by generating a series of random numbers and sorting treatment groups by the ascending random number order. The randomly sorted treatment groups were then assigned to each newt based on the newt identification number.
We exposed 65 newts to 1 mL of 5x106 Bsal zoospores/mL for 24 hrs in a 100-mL container holding 9 mL of autoclaved dechlorinated water. The Bsal isolate (AMFP13/1) used was obtained from a morbid fire salamander (Salamandra salamandra) provided by An Martel and Frank Pasman (University of Ghent, Ghent Belgium), and transferred to the University of Tennessee under a material transfer agreement. The culture used was cryo-archived and passed no more than five times prior to the experiment. The culture was maintained at the University of Tennessee in tryptone gelatin hydrolysate broth (TGhL). We grew Bsal for six days on TGhL plates and harvested Bsal zoospores by flooding each plate with 7 mL of autoclaved dechlorinated water. We filtered zoospores and zoosporangia through a 20-uM filter so that only the zoospore life-stage was present in the resulting filtrate. We enumerated zoospores using a hemocytometer and diluted the zoospore solution to the 5x106/mL zoospore dose used for the exposure, which is a dose known to cause Bsal chytridiomycosis in eastern newts (Carter et al., 2021). Following Bsal exposure, the infected newts were placed in individual 2000-cm3 containers holding 300 mL of dechlorinated water and a semicircular PVC cover object. All animals (exposed and susceptible) were housed at 14°C for the entire experiment duration. We waited for Bsal-induced mortality to occur in five of the infected newts, as evidence that clinical infection was achieved. Following the five disease-induced mortalities, we euthanized the remaining 60 infected newts by applying ~200 uL of 1% benzocaine hypochlorite gel to the dorsal aspect of each animal’s cephalic region. Fifteen minutes after the benzocaine application and following cessation of movement, each newt was exsanguinated via cardiac puncture to ensure humane euthanasia was achieved. The euthanized newt was rinsed with distilled water to remove any residual benzocaine prior to cohousing with susceptible newts.
Twenty susceptible newts were individually cohoused with one Bsal-infected carcass at different post-mortem timepoints for 24 hrs within two cohousing container types. Ten newts were assigned to each poster-mortem timepoint and cohousing chamber type combination as previous Bsal experiments have demonstrated this sample size is sufficient to determine survival and infection load differences between groups. The exposures between susceptible newts and infected carcasses occurred for 24 hrs between post-mortem timepoints: 0 – 24 (20 newts), 24 – 48 (20 newts), and 48 – 72 hrs (20 newts). Hereafter each post-mortem timepoint will be referenced as either 0 – 24, 24 – 48, or 48 – 72 hrs. Each timepoint represents an independent 24hr cohousing event such that in mathematical notation our post-mortem timepoints are [0,24[, [24,48, [48,72]. The cohousing chambers were 1500-cm3 rectangular containers holding 1 L of water. At the start of each post-mortem timepoint, 10 of the susceptible newts were assigned to a cohousing chamber with or without a partition (3 post-mortem timepoints x 2 co-housing scenarios = 6 treatments). The inclusion of partitioned chambers allowed us to evaluate differences between Bsal transmission from zoospores shed by infected carcasses only and transmission from carcasses that susceptible newts could contact while also being exposed to shed zoospores (Le Sage et al., 2019). Each partition was constructed using plastic mesh material with 0.5-mm2 openings. The mesh partitions were affixed to the bottom and walls of each container using silicon adhesive. The mesh openings were small enough to prevent direct contact between susceptible newts and the infected carcass but large enough to allow zoospores to freely pass from one side of the cohousing container to the other (note: Bsal zoospores measure 4 – 5.5 μm in diameter and are flagellated allowing them to maneuver through the water column (Martel et al., 2013). Each cohousing container was placed in environmental chambers set to 14°C for the duration of the cohousing event, which is a temperature known to be suitable for Bsal transmission (Carter et al., 2021; Tompros et al., 2022). Containers were not moved during cohousing to prevent the unnatural movement of zoospores. At the start of each post-mortem timepoint, the infected newt carcasses were swabbed following the previously described standardized protocol to determine initial Bsal load using qPCR (Boyle et al., 2004). Following cohousing, the infected carcass was swabbed again to determine whether the Bsal load changed during the 24-hr period. Also, after each post-mortem cohousing period, 200 mL of water from each chamber was collected for eDNA estimation of Bsal zoospores shed by each infected carcass over the 24-hr period. We assumed that shed zoospores detected during the 24 hrs were viable and were only released from the infected carcasses, as it would take multiple days for a newly infected animal to begin shedding the pathogen.
After each cohousing event, susceptible newts were placed in individual 2000-cm3 containers holding 300 mL of water and a semicircular PVC cover object. Newts were fed 2% of their body weight with frozen bloodworms (Chironomus plumosus) approximately 24 hrs prior to each water change. Water changes were conducted every three days and consisted of changing each newt’s housing container, water, and PVC cover object. Following the cohousing events, all susceptible newts were swabbed every six days to monitor for infection and provide evidence of successful transmission by host contact (Malagon et al., 2020). We continued to monitor susceptible newts for 66 days following the cohousing event, which is sufficient duration to document Bsal transmission and observe Bsal-induced mortality (Malagon et al., 2020; Carter et al., 2021; Tompros et al., 2022).
2.2 Batrachochytrium salamandrivorans genomic DNA testing procedures
Swab samples and water samples collected for eDNA testing were processed using similar extraction methods. Genomic DNA was extracted from swab samples using Qiagen DNeasy extraction kits (Qiagen, Hilden, Germany) following a modified version of the blood and tissue extraction method.
Water samples collected from each cohousing container for eDNA testing were processed using the same Qiagen DNeasy kit following a few post-digestion modifications (Laramie et al., 2015; Brunner, 2020). Specifically, water samples were filtered using a 0.45-μm Sterlitech filter funnel (Sterlitech, Washington, United States). Each filter membrane was divided in half using a disposable scalpel blade and the halves were placed in microcentrifuge tubes for either DNA extraction or long-term storage in a -80°C freezer. Prior to performing the DNeasy extraction protocol, each filter sample was spun through a Qiashredder spin column following the initial digestion step of the DNeasy protocol.
We quantified Bsal genomic DNA by performing qPCR amplification of extracted DNA recovered from swab and water samples in each experiment. Our qPCR protocol follows the methods described in Blooi et al. (2013). Individual samples were considered positive if duplicate sample wells amplified within 45 PCR cycles. Genomic Bsal DNA copies/uL were determined based on comparison with a standard curve derived from a synthetic DNA fragment corresponding to the amplification sequence targeted by the primers and probes described in Blooi et al. (2013). For filtered water samples, we converted Bsal genomic copies/uL detected in our eDNA samples to total Bsal zoospores shed over 24 hrs by assuming an average 10.9 genomic copies/zoospore (estimated using qPCR standard curve for known quantities of zoospores) and multiplying the resulting zoospore number by the total volume of water in each newt housing container. We assumed that detected Bsal DNA was viable, which is reasonable within 24 hours given zoospores are known to have a life cycle of 4 – 6 days (Martel et al., 2013; Robinson et al., 2020).
2.3 Bsal transmission from infected carcasses statistical analyses
2.3.1 Survival analysis on carcass transmission experiment newts
We created Cox proportional hazard models to determine whether the mortality rate of susceptible individuals was impacted by cohousing type (partitioned or not) and post-mortem timepoint. We compared candidate Cox proportional models using AIC and plotted hazard ratios from the best fit Cox proportional model using the ggforest function from the “survminer” package (Kassambara et al., 2021). Based on our AIC model comparisons we determined the best model included cohousing type (Partitioned=No contact or Not Partitioned=Contact) and post-mortem timepoints (0 – 24, 24 – 48, 48 – 72 hrs) without an interaction term. We evaluated the Cox proportional hazard model assumptions for our best fit model by plotting Cox-Snell residuals. We plotted Kaplan-Meier survival curves for each post-mortem timepoint and cohousing type using the ggsurvplot function. In order to see if the infection load on a carcass had an impact on the survival of susceptible newts, we created a new Cox proportional hazard model that included post-mortem timepoints and cohousing types as strata and the log transformed loads of each infected carcass as the only factor. After finding this model was significant, we created an additional Cox proportional model that included post-mortem timepoints and cohousing types as strata and the carcass infection load subtracted from the median carcass infection load as a new factor. The model was then used to simulate relative hazard estimates for susceptible individuals paired with infected carcasses of varying infection loads representing the range of infection loads measured on carcasses in our study. The model simulations was preformed using the coxsimlinear function from the “simPH” package (Gandrud, 2015).
2.3.2 Carcass experiment exposed animal Bsal load and shedding rates
To determine how post-mortem timepoint impacted Bsal shedding rates from infected carcasses, we performed analyses evaluating skin swabs and eDNA shedding rates using the Bsal genomic copies/uL determined during our qPCR assays on each sample type. We evaluated whether Bsal genomic copies/uL from carcass skin swabs differed among post-mortem timepoints (0 – 24, 24 – 48, 48 – 72 hours) by averaging pre- and post-cohousing swabs of the twenty carcasses used at each timepoint and performing an analysis-of-variance (ANOVA). If the ANOVA was significant (α = 0.05), we performed Tukey’s honest significance test to determine which pairwise post-mortem timepoints differed in Bsal genomic copies/uL detected. To determine whether Bsal loads on individual carcasses changed over the 24-hr cohousing period, we tested whether loads differed between pre- and post-cohousing swabs by performing paired Wilcoxon signed-rank tests (Conover, 1999). We also used ANOVA to test whether base 10 logarithm transformed Bsal genomic copies/uL detected from water samples (eDNA) differed among post-mortem timepoints. To determine whether eDNA detected in cohousing containers was correlated with swabs recovered from carcasses, we created a linear model in which the average Bsal genomic copies/uL detected from carcass swabs was used to predict eDNA detected in the cohousing water samples.
3 Results
3.1 Survival analysis
There was 100% infection of susceptible newts cohoused with carcasses across all post-mortem timepoints and cohousing types. Both the post-mortem timepoint and cohousing type influenced the survival of susceptible newts (Figure 1A, Table 1). Susceptible newts indirectly exposed to Bsal were 66% (HR = 0.34; 95% CI = [0.19, 0.60]; P< 0.001) less likely to die each day compared to those that were allowed to contact the carcasses (Figure 1B). Across cohousing types, susceptible newts cohoused in the 48 – 72-hr post-mortem timepoint were >2.5X [HR = 2.69; 95% CI = (1.36, 5.31)] more likely to die each day respectively, compared to the 0 – 24-hr group (Figure 1B). There were no differences between survival of susceptible newts exposed during the 0 – 24 and 24 – 48-hr post-mortem timepoints [HR = 1.13; 95% CI = (0.58, 2.2)]. Overall susceptible animal mortality across 0 – 24 and 24 – 48 hrs post-mortem timepoints was 85% and 90%, respectively. We further evaluated whether survival time was affected by the Bsal load on the infected carcass by creating a Cox proportional model stratified by post-mortem period and cohousing type. We found Bsal genomic copies/uL of the carcass was a significant predictor of susceptible animal survival. With each tenfold increase in Bsal genomic copies/uL on swabs from infected carcasses, there was a 70% [HR = 1.70; 95% CI = (1.27, 2.28)] increase in the likelihood of death each day (Figure 2).
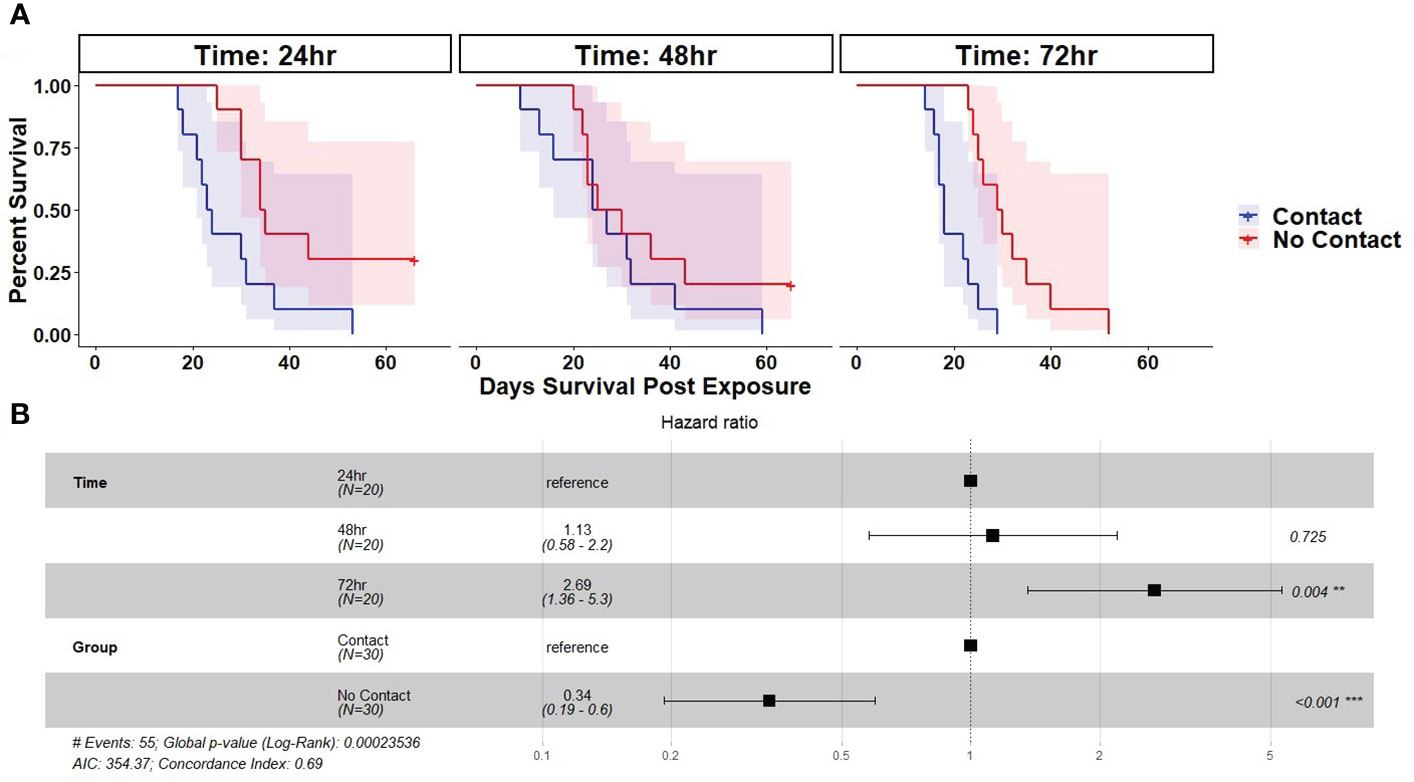
Figure 1 (A) Kaplan-Meier survival curves showing probability of mortality and associated 95% confidence interval by day for susceptible Notophthalmus viridescens exposed to Batrachochytrium salamandrivorans via cohousing with an infected N. viridescens carcass. Individual colored lines and shaded areas represent different cohousing types (Partitioned=No contact or Not Partitioned=Contact). Plots are faceted by post-mortem timepoints (0 – 24, 24 – 48, 48 – 72 hrs). (B) Results of best fit Cox-proportional hazard model fit to the survival of susceptible newts cohoused with Bsal infected carcasses. Plot shows hazard ratio estimate (black square), 95% CI of the hazard ratio (whiskers), and corresponding P-value.
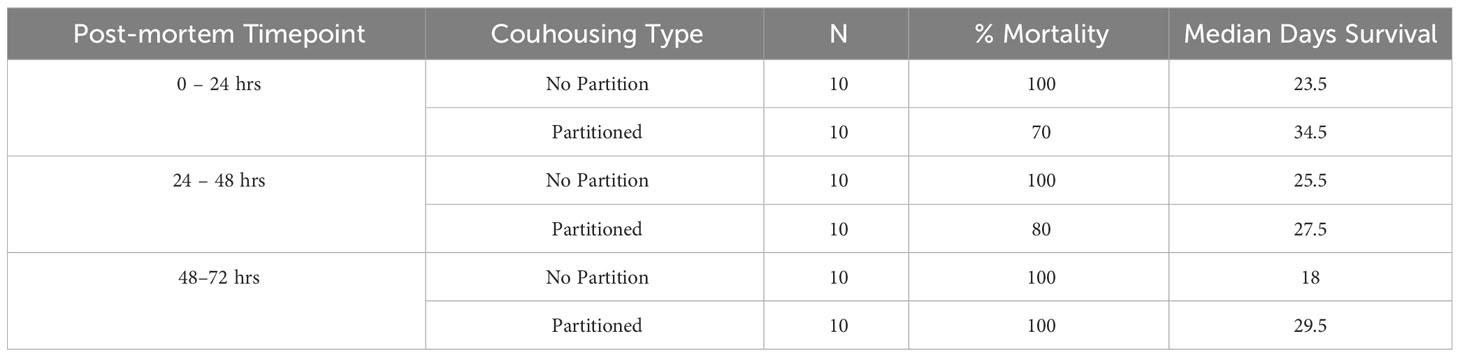
Table 1 Treatment size, percentage group mortality, and median days survival for susceptible Notophthalmus viridescens cohoused with Batrachochytrium salamandrivorans (Bsal) infected N. viridescens carcasses at different post-mortem timepoints (0 – 24, 24 – 48, 48 – 72hrs) in either partitioned or not partitioned cohousing chambers.
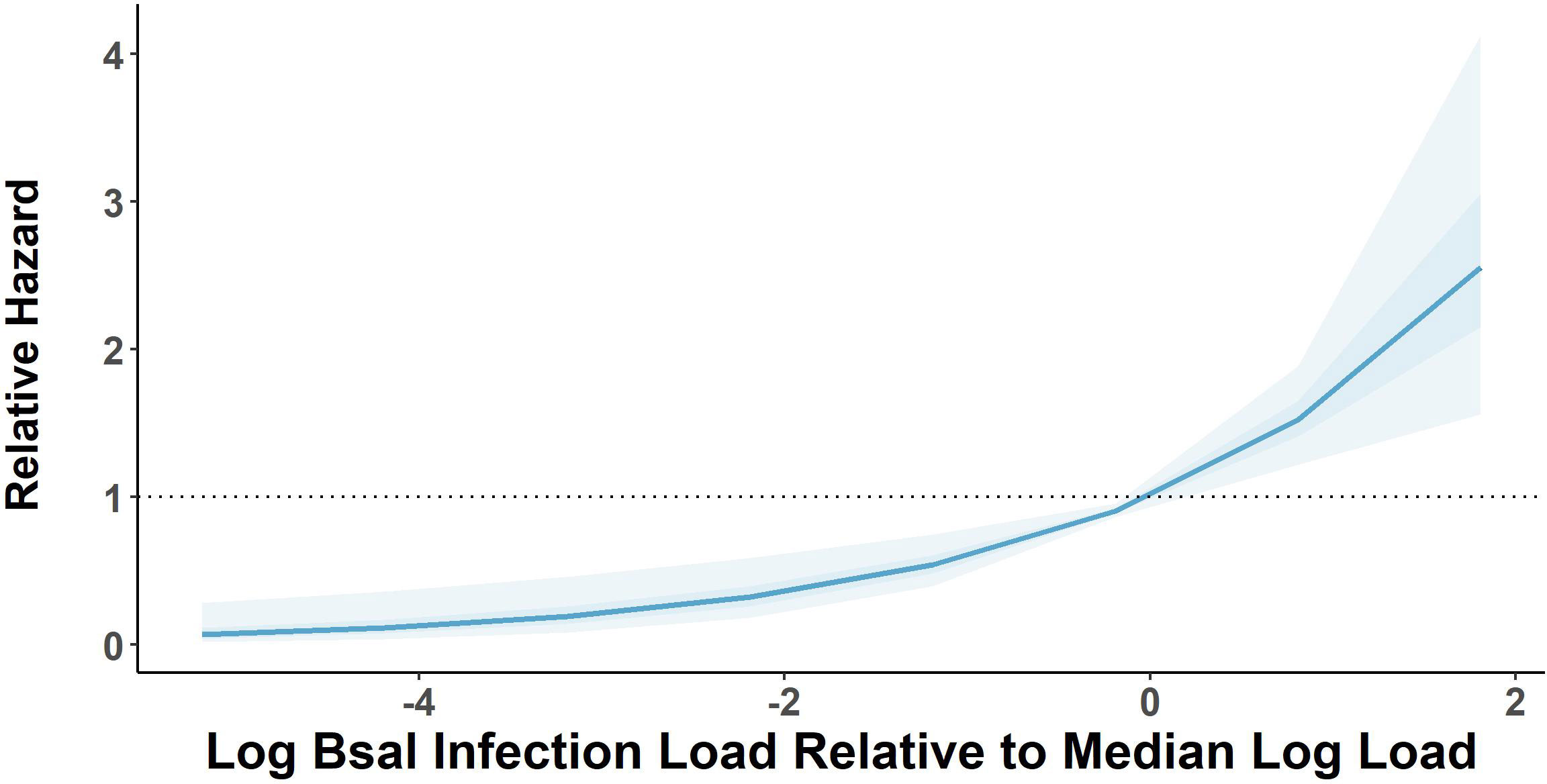
Figure 2 Relative mortality hazard estimates of susceptible Notophthalmus viridescens based on 1000 model simulations performed on a Cox proportional hazard model estimating relative hazard of log base 10-transformed Batrachochytrium salamandrivorans (Bsal) load estimates measured on Bsal-infected carcasses. Dark and light shaded areas show bounds of model simulations for 50 and 95% of simulated models, respectively. Relative hazard measurements greater than black dotted line indicates increased risk of mortality [zero-centered] median log load.
3.2 Bsal infection load analysis
Bsal genomic copies/uL measured from swab samples taken from each carcass were positively correlated with the amount of Bsal eDNA measured in water samples (R = 0.48; β = 0.62 ± 0.22; P = 0.007; Figure 3). For carcasses used in the partitioned cohousing treatments, log Bsal genomic copies/uL measured from the water did not differ among post-mortem timepoints (F2,27 = 0.38; P = 0.68). Across post-mortem timepoints an average of 2743 Bsal genomic copies/uL was detected in the partitioned cohousing chambers was (Supplementary Table 1). Based on the Bsal genomic copies detected in water chambers, we estimated that carcasses shed on average 1850, 999, and 1224 zoospores/day at 0 – 24, 24 – 48, and 48 – 72 hrs post-mortem, respectively. However, average carcass Bsal genomic copies/uL measured from swab samples were over 400X greater than what we detected in water samples (X̅ = 1,216,975; SE = 197,271). We found that Bsal genomic copies/uL detected on swabs of carcasses differed among post-mortem timepoints (F2,57 = 4.39; P=0.017). Our post-hoc analysis revealed that carcasses in the 0 – 24-hr post-mortem timepoint (X̅ = 744,984; SE = 196,139) had 2.7X fewer Bsal genomic copies/uL than carcasses 48 – 72 hrs post-mortem (X̅ = 2,043,173; SE = 457,478; P = 0.02). No differences were detected between carcasses in the 24 – 48-hr post-mortem timepoint and the other post-mortem timepoints (Figure 4). For all post-mortem timepoints combined, we found only a marginal difference in Bsal genomic copies/uL between swabs taken from carcasses prior to and following each post-mortem period (P=0.056, effect size = 0.247; Supplementary Table 2).
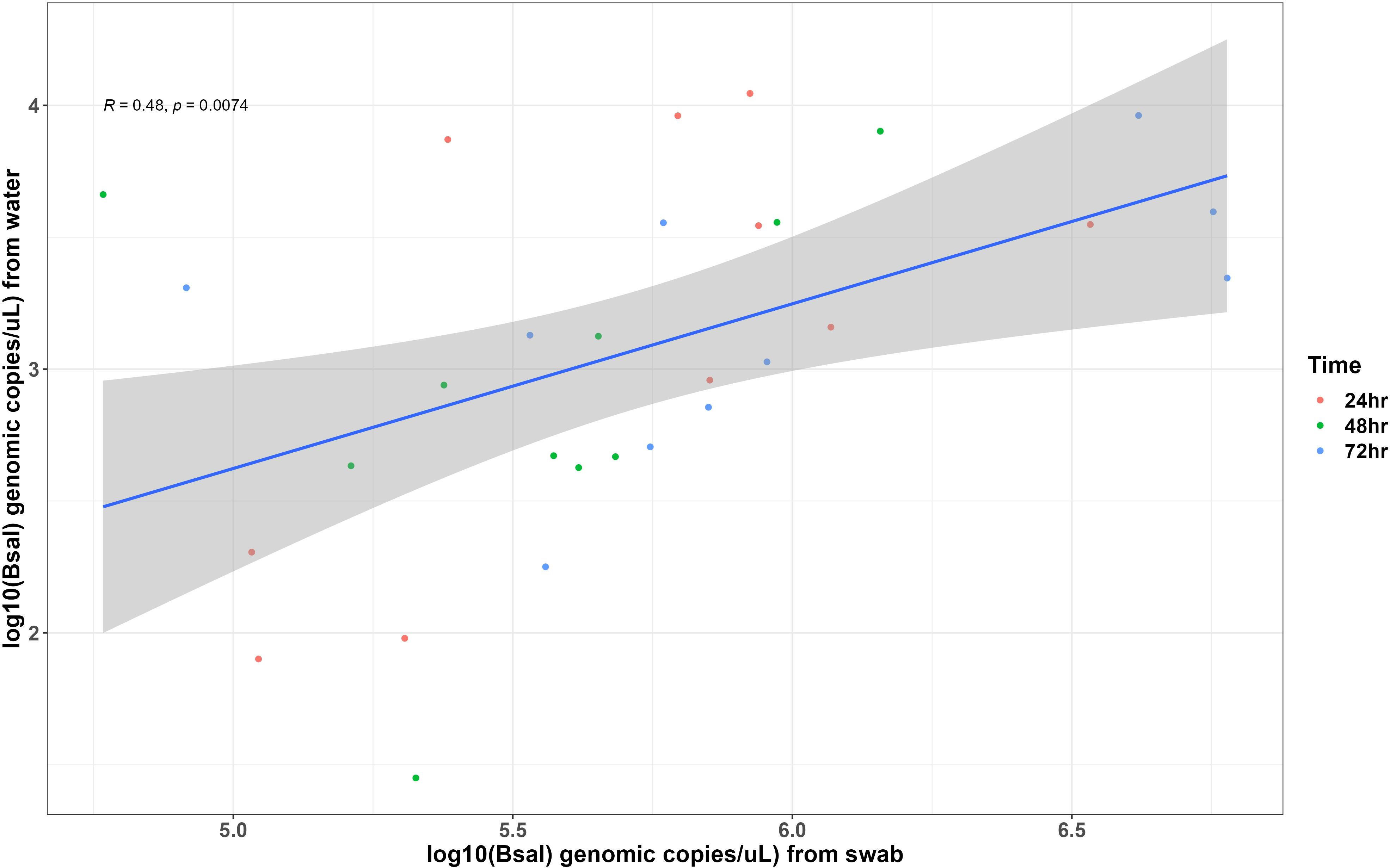
Figure 3 Each point represents measurements of Batrachochytrium salamandrivorans (Bsal) genomic copies/uL detected on skin swabs and in water samples collected from Bsal infected Notophthalmus viridescens carcasses following 24 hrs of being cohoused with susceptible individuals. Points are colored by post-mortem timepoints. The trendline and shaded area shows the line of best fit and 95% confidence interval of the relationship between Bsal infection load and zoospore shedding by infected carcasses.
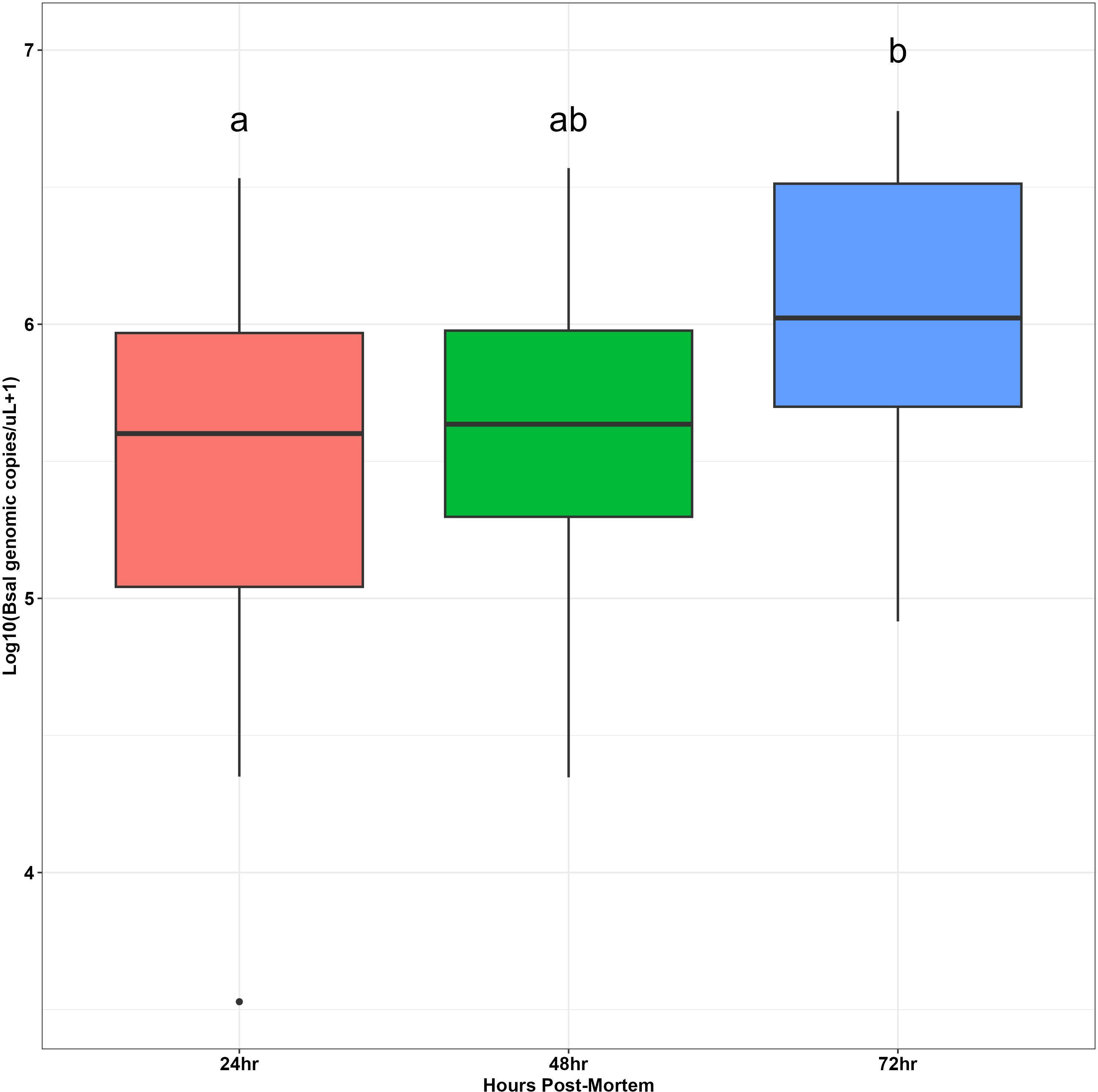
Figure 4 Boxplots showing log base 10-transformed Batrachochytrium salamandrivorans (Bsal) genomic copies/uL load measurements from skin swabs of carcasses at different timepoints post-mortem (24, 48 or 72 hrs). Boxplots show medians (black middle line) for each group. The upper and lower portions of each box represent the 75 and 25% quartile range, respectively, and whiskers extend to minimum and maximum values for each group. Dots represent outliers. Dissimilar groups based on significant Tukey’s Honest significant difference tests (P < 0.05) are indicated with different lowercase letters.
4 Discussion
Our carcass transmission experiment demonstrates the potential importance of infected carcasses to Bsal epidemiology. All susceptible newts paired with carcasses became infected during the cohousing timepoints, even when only indirect, waterborne transmission was allowed. We found newt carcasses can be infectious for at least three days post-mortem, hence extending the infectious duration of a host, which likely would influence the overall reproductive number of Bsal. Furthermore, carcasses likely contribute to frequency-dependent transmission, as they extend the infectious period of infected animals (Tompros et al., 2022). The median duration of survival for susceptible newts paired with carcasses was 26.5 days (95% CI = 24, 31). Based on the survival time of susceptible newts from this experiment, carcasses could extend the duration of infectiousness and zoospore shedding by at least 10% (26.5 days + 3 additional days as an infectious carcass). While we did perform a heat treatment method to clear Bd infections in all individuals used in our experiments, we did not clear them of all possible pathogens or parasites which could influenced their susceptibility. That said, we did not observe any gross signs of other infections, and other than Bd, there have been no co-infection experiments performed with Bsal, so the possible directional effects are unknown. Bsal loads on the infected carcasses and the number of shed zoospores released by carcasses did not decline over the 72-hr experiment duration. Further, greater Bsal loads were detected on infected carcasses 72 hrs post-mortem compared to those sampled 24 hrs after death. The greater pathogen loads measured 72 hrs post-mortem may be the result of the carcass epidermis deteriorating making swabbing efforts more successful. Alternatively, any adaptive or innate immune defenses against Bsal would have ceased function following death, facilitating unregulated pathogen growth. We also observed greater rates of mortality in susceptible newts paired with carcasses at 24 – 48 and 48 – 72 hrs post-mortem than for hosts paired with carcasses immediately after death. These results collectively suggest that the contribution of carcasses to Bsal epidemiology may extend beyond 72 hrs and warrants additional investigation. The rate of carcass decay is likely an important factor which would impact the overall contribution of carcasses to secondary infections. If carcasses persist in the environment for longer durations, they would likely continue to increase the overall of Bsal (assuming Bsal continues to grow on the carcasses). Collectively, this study indicates that carcasses likely play an important role in Bsal transmission dynamics for aquatic salamander hosts, and Bsal should be considered “necronotic” (nekro = corpse, nosos = disease) given the fact carcasses may contribute substantially to the overall basic reproductive number within a Bsal disease system.
Our results demonstrate that Bsal epidemiology models should include a carcass compartment in addition to the traditional susceptible, exposed, and infected classes (Fischer et al., 2013). In ranavirus systems, carcasses can transmit virions by contact and through the water (similar to Bsal), but also via necrophagy (Pearman et al., 2004; Harp and Petranka, 2006; Le Sage et al., 2019; Peace et al., 2019). Unlike scavenging anuran tadpoles, necrophagy may not be an important transmission pathway for Bsal in caudates, because larval and adult salamanders are insectivores (Wells, 2007). However, necrophagy may play a role in transmission of Bsal to anurans, considering some frog species are susceptible to Bsal, and larval amphibians can become infected by the pathogen (Nguyen et al., 2017; Nguyen et al., 2017; Gray et al., 2023). Bd infections in anuran tadpoles are often observed in the keratinized mouthparts prior to metamorphosis (McMahon and Rohr, 2015). Given the rasping nature of tadpole feeding, it is possible their mouthparts could become infected while feeding on Bsal-infected carcasses. Following introduction to a tank, carcasses shed approximately 1850 zoospores in the first 24 hrs, 999 zoospores in the next 24 hrs, and 1224 spores in the third 24-hr period. These shedding rates resulted in highly efficient indirect transmission (100%) and 83% mortality of susceptible newts that became infected in the partitioned cohousing chambers. These estimates represent the first quantification of zoospore shedding rates for Bsal-infected newts. Ranavirus virion and Bd zoospore shedding rates have been estimated previously in live animals (DiRenzo et al., 2014; Peace et al., 2019). DiRenzo et al. (DiRenzo et al., 2014) estimated Bd zoospore shedding rates by Atelopus zeteki immediately before euthanasia were equivalent to 23.55 zoospores genomic equivalents per minute which is nearly 500X the shedding rate measured in our study for recently dead carcasses. The greater shedding rate measured by DiRenzo et al. (DiRenzo et al., 2014) may be related to the high pathogen loads on their frogs, which were >4x106 Bd zoospore equivalents. It is unclear from our study how long carcasses would continue contributing to the zoospore pool during a Bsal outbreak. However, we observed no decline in Bsal shedding over the 72-hr period indicating that carcasses may shed for longer durations. With carcasses lacking any immunological control of pathogen growth, competitive growth of other microbes or deterioration of growth substrate (i.e., skin for Bsal) are likely processes that would eventually decrease pathogen shedding from carcasses.
Our results indicate that Bsal response plans should consider strategies that reduce carcass density, such as physical removal of infected individuals or carcasses. Introduction of or implementation of habitat management strategies that enhance invertebrate scavenger populations might facilitate carcass removal (Le Sage et al., 2019) and reduce pathogen spread. However, it also is possible that scavenging could release zoospores as carcasses are consumed and shredding of infected tissues releases fragmented epidermal cells containing zoosporangia. Some scavenging species also could become infected with Bsal or mechanically transmit it to susceptible hosts. For example, crayfish are known to become infected with Bd (McMahon et al., 2013) and as scavengers may readily ingest amphibian carcasses. Habitat manipulations, such as dewatering a wetland, could be used to accelerate carcass decomposition and reduce indirect transmission rates from carcasses. However, this strategy would likely have negative effects on other resident aquatic species. We recommend all Bsal response strategies be conducted in consultation with experts and appropriate regulatory authorities (Grant et al., 2017; Hopkins et al., 2018).
If Bsal becomes established in naïve regions (such as the United States), eradication will be challenging due to the pathogen’s ability to infect multiple host species (Martel et al., 2014; Carter et al., 2019; Friday et al., 2020), environmentally stable life-stages (Stegen et al., 2017), and recent evidence suggesting that at least some Bsal isolates may have saprophytic abilities (Kelly et al., 2021). Unless the pathogen is introduced into a heavily monitored amphibian population, initial detections could occur years following introduction, making containment difficult. If Bsal eradication is difficult to achieve, as has been the case in Europe (Stegen et al., 2017; Canessa et al., 2018; Martel et al., 2020), we must be willing to use disease management strategies to prevent potentially catastrophic losses to amphibian diversity and preserve the valuable ecosystem services these species provide. Evaluating the contribution of all Bsal transmission routes is a critical first step to identifying effective strategies. Successful management of Bsal will likely require alteration of transmission routes and implementation of strategies that increase host resistance or tolerance to the pathogen (Wilber et al., 2017; Canessa et al., 2018; Islam et al., 2021).
Data availability statement
The raw data supporting the conclusions of this article will be made available by the authors, without undue reservation.
Ethics statement
The animal study was approved by The University of Tennessee Institutional Animal Care and Use Committee under protocol #2623. The study was conducted in accordance with the local legislation and institutional requirements.
Author contributions
EC: Conceptualization, Data curation, Formal analysis, Investigation, Methodology, Resources, Visualization, Writing – original draft, Writing – review & editing. JD: Conceptualization, Data curation, Formal analysis, Methodology, Resources, Writing – original draft, Writing – review & editing. MW: Conceptualization, Formal analysis, Investigation, Supervision, Writing – original draft, Writing – review & editing. DM: Conceptualization, Funding acquisition, Investigation, Supervision, Writing – original draft, Writing – review & editing. MG: Conceptualization, Formal analysis, Funding acquisition, Investigation, Methodology, Project administration, Resources, Supervision, Writing – original draft, Writing – review & editing.
Funding
The author(s) declare financial support was received for the research, authorship, and/or publication of this article. This research was conducted using funds from the National Science Foundation Division of Environmental Biology (Ecology and Evolution of Infectious Diseases Program, https://www.nsf.gov/funding/pgmsumm.jsp?pimsid=5269) Grant #1814520 and the USDA National Institute of Food and Agriculture Hatch Project #7001607.
Conflict of interest
The authors declare that the research was conducted in the absence of any commercial or financial relationships that could be construed as a potential conflict of interest.
Publisher’s note
All claims expressed in this article are solely those of the authors and do not necessarily represent those of their affiliated organizations, or those of the publisher, the editors and the reviewers. Any product that may be evaluated in this article, or claim that may be made by its manufacturer, is not guaranteed or endorsed by the publisher.
Supplementary material
The Supplementary Material for this article can be found online at: https://www.frontiersin.org/articles/10.3389/famrs.2024.1284608/full#supplementary-material
References
Annemarieke Spitzen-van der S., Frank S., Wilbert B., Marnix de Z., Tom van der M., Edo G., et al. (2013). Rapid enigmatic decline drives the fire salamander (Salamandra salamandra) to the edge of extinction in the Netherlands. Amphibia-Reptilia 34 (2), 233–239. doi: 10.1163/15685381-00002891
Bletz M. (2013). Probiotic Bioaugmentation of an Anti-Bd Bacteria, Janthinobacterium Lividum, on the Amphibian, Notophthalmus Viridescens: Transmission Efficacy and Persistence of the Probiotic on the Host and Non-target Effects of Probiotic Addition on Ecosystem Components (Harrisonburg, VA: James Madison University).
Bletz M. C., Kelly M., Sabino-Pinto J., Bales E., Van Praet S., Bert W., et al. (2018). Disruption of skin microbiota contributes to salamander disease. Proc. R. Soc. B: Biol. Sci. 285 (1885), 20180758. doi: 10.1098/rspb.2018.0758
Blooi M., Pasmans F., Longcore J. E., Spitzen-van der Sluijs A., Vercammen F., Martel A. (2013). Duplex real-time PCR for rapid simultaneous detection of Batrachochytrium dendrobatidis and Batrachochytrium salamandrivorans in Amphibian samples. J. Clin. Microbiol. 51 (12), 4173–4177. doi: 10.1128/JCM.02313-13
Boyle D. G., Boyle D. B., Olsen V., Morgan J. A. T., Hyatt A. D. (2004). Rapid quantitative detection of chytridiomycosis (Batrachochytrium dendrobatidis) in amphibian samples using real-time Taqman PCR assay. Dis. Aquat Org. 60, 141–148. doi: 10.3354/dao060141
Brunner J. L. (2020). Pooled samples and eDNA-based detection can facilitate the “clean trade” of aquatic animals. Sci. Rep. 10 (1), 10280. doi: 10.1038/s41598-020-66280-7
Brunner J., Schock D., Collins J. (2007). Transmission dynamics of the amphibian ranavirus Ambystoma tigrinum virus. Dis. Aquat. Organisms. 77 (2), 87–95. doi: 10.3354/dao01845
Brunner J. L., Storfer A., Gray M. J., Hoverman J. T. (2015). “Ranavirus ecology and evolution: from epidemiology to extinction,” in Ranaviruses: Lethal Pathogens of Ectothermic Vertebrates, vol. p . Eds. Gray M. J., Chinchar V. G. (Cham: Springer International Publishing), 71–104.
Canessa S., Bozzuto C., Grant E., Cruickshank S., Fisher M., Koella J., et al. (2018). Decision-making for mitigating wildlife diseases: From theory to practice for an emerging fungal pathogen of amphibians. J. Appl. Ecol. 55, 1987–1996. doi: 10.1111/1365-2664.13089
Canessa S., Bozzuto C., Pasmans F., Martel A. (2019). Quantifying the burden of managing wildlife diseases in multiple host species. Conserv. Biol. 33 (5), 1131–1140. doi: 10.1111/cobi.13313
Carter E. D., Bletz M. C., Le Sage M., LaBumbard B., Rollins-Smith L. A., Woodhams D. C., et al. (2021). Winter is coming–Temperature affects immune defenses and susceptibility to Batrachochytrium salamandrivorans. PloS Pathogens. 17 (2), e1009234. doi: 10.1371/journal.ppat.1009234
Carter E. D., Miller D. L., Peterson A. C., Sutton W. B., Cusaac J. P. W., Spatz J. A., et al. (2019). Conservation risk of Batrachochytrium salamandrivorans to endemic lungless salamanders. Conserv. Letters. 0 (0), e12675. doi: 10.1111/conl.12675
Chatfield M. W., Richards-Zawacki C. L. (2011). Elevated temperature as a treatment for Batrachochytrium dendrobatidis infection in captive frogs. Dis. Aquat Organ. 94 (3), 235–238. doi: 10.3354/dao02337
DiRenzo G. V., Langhammer P. F., Zamudio K. R., Lips K. R. (2014). Fungal infection intensity and zoospore output of Atelopus zeteki, a potential acute Chytrid supershedder. PLoS One 9 (3), 1–6. doi: 10.1371/journal.pone.0093356
Fischer J. W., Phillips G. E., Nichols T. A., VerCauteren K. C. (2013). Could avian scavengers translocate infectious prions to disease-free areas initiating new foci of chronic wasting disease? Prion 7 (4), 263–266. doi: 10.4161/pri.25621
Friday B., Holzheuser C., Lips K. R., Longo A. V. (2020). Preparing for invasion: Assessing risk of infection by chytrid fungi in southeastern plethodontid salamanders. J. Exp. Zoology Part A: Ecol. Integr. Physiol. 333 (10), 829–840. doi: 10.1002/jez.2427
Gandrud C. (2015). simPH: an R package for illustrating estimates from cox proportional hazard models including for interactive and nonlinear effects. J. Stat. Software 65 (3), 1–20. doi: 10.18637/jss.v065.i03
Garner T. W. J., Schmidt B. R., Martel A., Pasmans F., Muths E., Cunningham A. A., et al. (2016). Mitigating amphibian chytridiomycoses in nature. Philos. Trans. R Soc. Lond B Biol. Sci. 371 (1709), 20160207. doi: 10.1098/rstb.2016.0207
Grant E. H. C., Muths E., Katz R. A., Canessa S., Adams M. J., Ballard J. R., et al. (2017). Using decision analysis to support proactive management of emerging infectious wildlife diseases. Front. Ecol. Environment. 15 (4), 214–221. doi: 10.1002/fee.1481
Gray M. J., Carter E. D., Piovia-Scott J., Cusaac J. P. W., Peterson A. C., Whetstone R. D., et al. (2023). Broad host susceptibility of North American amphibian species to Batrachochytrium salamandrivorans suggests high invasion potential and biodiversity risk. Nat. Commun. 14 (1), 3270. doi: 10.1038/s41467-023-38979-4
Harp E. M., Petranka J. W. (2006). Ranavirus in wood frogs (Rana sylvatica): Potential sources of transmission within and between ponds. J. Wildlife Diseases. 42 (2), 307–318. doi: 10.7589/0090-3558-42.2.307
Hopkins M. C., Adams M. J., Super P. E., Olson D. H., Hickman C. R., English P., et al. (2018). “Batrachochytrium salamandriovrans (Bsal) in Appalachia—Using scenario building to proactively prepare for a wildlife disease outbreak caused by an invasive amphibian chytrid fungus,” in Report. Ed. Reston V. A. U.S. Geological Survey, Sunrise Valley Drive, Suite 300 Reston, VA, 2018–1150.
Islam M. R., Gray M. J., Peace A. (2021). “Identifying the dominant transmission pathway in a multi-stage infection model of the emerging fungal pathogen Batrachochytrium salamandrivorans on the eastern Newt,” in Infectious Diseases and Our Planet. Eds. Teboh-Ewungkem M. I., Ngwa G. A. (Cham: Springer International Publishing), 193–216.
Jennelle C. S., Samuel M. D., Nolden C. A., Berkley E. A. (2009). Deer carcass decomposition and potential scavenger exposure to chronic wasting disease. J. Wildlife Management. 73 (5), 655–662. doi: 10.2193/2008-282
Kassambara A., Kosinski M., Beicek P. (2021). survminer: Drawing Survival Curves using 'ggplot2'. R package version 0.4.9. Available at: https://CRAN.R-project.org/package=survminer.
Kelly M., Pasmans F., Muñoz J. F., Shea T. P., Carranza S., Cuomo C. A., et al. (2021). Diversity, multifaceted evolution, and facultative saprotrophism in the European Batrachochytrium salamandrivorans epidemic. Nat. Commun. 12 (1), 6688. doi: 10.1038/s41467-021-27005-0
Kumar R., Malagon D. A., Carter E. D., Miller D. L., Bohanon M. L., Cusaac J. P. W., et al. (2020). Experimental methodologies can affect pathogenicity of Batrachochytrium salamandrivorans infections. bioRxiv 2020, 154328. doi: 10.1371/journal.pone.0235370
Laramie M. B., Pilliod D. S., Goldberg C. S. (2015). Characterizing the distribution of an endangered salmonid using environmental DNA analysis. Biol. Conserv. 183, 29–37. doi: 10.1016/j.biocon.2014.11.025
Le Sage M. J., Towey B. D., Brunner J. L. (2019). Do scavengers prevent or promote disease transmission? The effect of invertebrate scavenging on Ranavirus transmission. Funct. Ecology. 33 (7), 1342–1350. doi: 10.1111/1365-2435.13335
Longo A., Fleischer R. C., Lips K. (2019). Double trouble: co-infections of chytrid fungi will severely impact widely distributed newts. Biol. Invasions. 21, 2233–2245. doi: 10.1007/s10530-019-01973-3
Maguire C., DiRenzo G. V., Tunstall T. S., Muletz C. R., Zamudio K. R., Lips K. R. (2016). Dead or alive? Viability of chytrid zoospores shed from live amphibian hosts. Dis. Aquat. Organisms. 119 (3), 179–187. doi: 10.3354/dao02991
Malagon D. A., Melara L. A., Prosper O. F., Lenhart S., Carter E. D., Fordyce J. A., et al. (2020). Host density and habitat structure influence host contact rates and Batrachochytrium salamandrivorans transmission. Sci. Rep. 10 (1), 5584. doi: 10.1038/s41598-020-62351-x
Martel A., Blooi M., Adriaensen C., Van Rooij P., Beukema W., Fisher M. C., et al. (2014). Recent introduction of a chytrid fungus endangers Western Palearctic salamanders. Science 346 (6209), 630–631. doi: 10.1126/science.125826
Martel A., Spitzen-van der Sluijs A., Blooi M., Bert W., Ducatelle R., Fisher M. C., et al. (2013). Batrachochytrium salamandrivorans sp nov causes lethal chytridiomycosis in amphibians. Proc. Natl. Acad. Sci. U S A. 110 (38), 15325–15329. doi: 10.1073/pnas.1307356110
Martel A., Vila-Escale M., Fernández-Giberteau D., Martinez-Silvestre A., Canessa S., Van Praet S., et al. (2020). Integral chain management of wildlife diseases. Conserv. Lett. 13, e12707. doi: 10.1111/conl.12707
McMahon T. A., Brannelly L. A., Chatfield M. W. H., Johnson P. T. J., Joseph M. B., McKenzie V. J., et al. (2013). Chytrid fungus Batrachochytrium dendrobatidis has nonamphibian hosts and releases chemicals that cause pathology in the absence of infection. Proc. Natl. Acad. Sci. 110 (1), 210–215. doi: 10.1073/pnas.1200592110
McMahon T. A., Rohr J. R. (2015). Transition of chytrid fungus infection from mouthparts to hind limbs during amphibian metamorphosis. Ecohealth 12 (1), 188–193. doi: 10.1007/s10393-014-0989-9
Nguyen T. T., Nguyen T., Ziegler T., Pasmans F., Martel A. (2017). Trade in wild anurans vectors the urodelan pathogen Batrachochytrium salamandrivorans into Europe. Amphibia-Reptilia 38 (4), 554–556. doi: 10.1163/15685381-00003125
Olson D. H., Ronnenberg K. L., Glidden C. K., Christiansen K. R., Blaustein A. R. (2021). Global patterns of the fungal pathogen Batrachochytrium dendrobatidis support conservation urgency. Front. Veterinary Sci. 8. doi: 10.3389/fvets.2021.685877
Peace A., O’Regan S. M., Spatz J. A., Reilly P. N., Hill R. D., Carter E. D., et al. (2019). A highly invasive chimeric ranavirus can decimate tadpole populations rapidly through multiple transmission pathways. Ecol. Modelling. 410, 108777. doi: 10.1016/j.ecolmodel.2019.108777
Pearman P. B., Garner T. W., Straub M., Greber U. F. (2004). Response of the Italian agile frog (Rana latastei) to a Ranavirus, frog virus 3: a model for viral emergence in naïve populations. J. Wildl Dis. 40 (4), 660–669. doi: 10.7589/0090-3558-40.4.660
Pepin K. M., Golnar A. J., Abdo Z., Podgórski T. (2020). Ecological drivers of African swine fever virus persistence in wild boar populations: Insight for control. Ecol. Evolution. 10 (6), 2846–2859. doi: 10.1002/ece3.6100
Prescott J., Bushmaker T., Fischer R., Miazgowicz K., Judson S., Munster V. J. (2015). Postmortem stability of Ebola virus. Emerg. Infect. Dis. 21 (5), 856–859. doi: 10.3201/eid2105.150041
Robinson K. A., Pereira K. E., Bletz M. C., Carter E. D., Gray M. J., Piovia-Scott J., et al. (2020). Isolation and maintenance of Batrachochytrium salamandrivorans cultures. Dis. Aquat. Organisms. 140, 1–11. doi: 10.3354/dao03488
Scheele B. C., Pasmans F., Skerratt L. F., Berger L., Martel A., Beukema W., et al. (2019). Amphibian fungal panzootic causes catastrophic and ongoing loss of biodiversity. Science 363 (6434), 1459. doi: 10.1126/science.aav0379
Sheley W. C., Gray M. J., Wilber M. Q., Cray C., Carter E. D., Miller D. L. (2023). Electrolyte imbalances and dehydration play a key role in Batrachochytrium salamandrivorans chytridiomycosis. Front. Veterinary Scienc 9. doi: 10.3389/fvets.2022.1055153
Smith K. M. B. (2013). Conceptions of Disease Contagion in Ancient Literature [M.A.] (Canada – Ontario, CA: Queen's University (Canada).
Stegen G., Pasmans F., Schmidt B. R., Rouffaer L. O., Van Praet S., Schaub M., et al. (2017). Drivers of salamander extirpation mediated by Batrachochytrium salamandrivorans. Nature 544 (7650), 353. doi: 10.1038/nature22059
Thomas V., Yu W., Pascale Van R., Elin V., Vojtech B., Jaime B., et al. (2019). Mitigating Batrachochytrium salamandrivorans in Europe. Amphibia-Reptilia 40 (3), 265–290. doi: 10.1163/15685381-20191157
Tompros A., Dean A. D., Fenton A., Wilber M. Q., Carter E. D., Gray M. J. (2022). Frequency-dependent transmission of Batrachochytrium salamandrivorans in eastern newts. Transboundary Emerging Dis. 69, 731–741. doi: 10.1111/tbed.14043
Wells D. K. (2007). “The ecology and behavior of amphibians,” in World Scientific. Chicago, Illinois: The University of Chicago Press.
Wilber M. Q., Knapp R. A., Toothman M., Briggs C. J. (2017). Resistance, tolerance and environmental transmission dynamics determine host extinction risk in a load-dependent amphibian disease. Ecol. letters. 20 (9), 1169–1181. doi: 10.1111/ele.12814
Keywords: amphibian, chytridiomycosis, chytrid, transmission, disease ecology
Citation: Carter ED, DeMarchi JA, Wilber MQ, Miller DL and Gray MJ (2024) Batrachochytrium salamandrivorans is necronotic: carcasses could play a role in Bsal transmission. Front. Amphib. Reptile Sci. 2:1284608. doi: 10.3389/famrs.2024.1284608
Received: 28 August 2023; Accepted: 23 January 2024;
Published: 21 February 2024.
Edited by:
Joana Sabino Pinto, University of Groningen, NetherlandsReviewed by:
Laura Adamovicz, University of Illinois at Urbana-Champaign, United StatesJacob Kerby, University of South Dakota, United States
Copyright © 2024 Carter, DeMarchi, Wilber, Miller and Gray. This is an open-access article distributed under the terms of the Creative Commons Attribution License (CC BY). The use, distribution or reproduction in other forums is permitted, provided the original author(s) and the copyright owner(s) are credited and that the original publication in this journal is cited, in accordance with accepted academic practice. No use, distribution or reproduction is permitted which does not comply with these terms.
*Correspondence: E. Davis Carter, ZWRjYXJ0ZXJAc2FsaXNidXJ5LmVkdQ==