- 1Department of Chemistry, Pepperdine University, Malibu, CA, United States
- 2Department of Ecology and Evolutionary Biology, University of California, Los Angeles, Los Angeles, CA, United States
- 3Department of Biology, Pepperdine University, Malibu, CA, United States
Sexually dimorphic phenotypes are consequential to animal survival, and this is especially apparent with defense phenotypes. Amphibians have poison glands, and several lineages maintain a neurotoxin, tetrodotoxin (TTX), which is largely considered a derived chemical defense trait. However, production pathways are unclear, and, as such, whether males and females differentially produce or appropriate toxin concentrations is not known. We evaluated the relationship between TTX concentrations, sex, and morphology by collecting trait data from more than 850 wild newts of the genus Taricha and tested for sex-based differences, potential sex-based changes to the phenotype between breeding seasons, and factors influencing toxicity. Females, regardless of reproductive status, exhibited greater concentrations than males, and temporal patterns indicate male and female trait values tend to fluctuate concordantly at short time scales, with females showing greater change over time. Tree regression results underscored the importance of size and sex to the phenotype. Sexual dimorphism and plasticity of toxins broaden the ecological roles of animal poisons beyond defense only and should recast how we model the evolution of tetrodotoxin.
1 Introduction
Sexually dimorphic traits are recognized as crucial phenotypes for survival, including size, ornamentation, color, hormones, and behaviors (Williams and Carroll, 2009; Mori et al., 2017) that can be driven by female-choice, founder events, and geographic differences, in addition to internal sex-limited gene expression (Emlen et al., 2007; Williams and Carroll, 2009). Anisogamous animals exhibit dissimilar phenotypes associated with dimorphic gamete production (Scharer et al., 2012) including amphibians, which overwhelmingly exhibit female-biased sexual size dimorphism (Shine, 1979; Lindenfors et al., 2007; Mori et al., 2017; Pogoda and Kupfer, 2018). A defining trait of amphibians is the presence of poison glands, but few studies have evaluated whether or not toxin phenotypes are sexually dimorphic (Mebs and Pogoda, 2005; Daly et al., 2008; Saporito et al., 2010; Stokes et al., 2011; Jeckel et al., 2015; Preißler et al., 2019; De Meester et al., 2021). Previous evaluations have focused on poison gland morphology and arrangement (Aoto, 1950; Staub and Julie, 1997; Chen et al., 2017; Zamora-Camacho, 2022) without addressing sex-based differences (Mailho-Fontana et al., 2019) and the few studies that have addressed poison composition and quantities found that anurans (family Dendrobatidae) exhibit sex-based differences due to diet (Saporito et al., 2006; Daly et al., 2008; Saporito et al., 2010; Jeckel et al., 2015). In addition, urodeles (genus Salamandra) possess, overall, a similar alkaloid composition (Preißler et al., 2019), but show distinct quantities of component toxin between sexes (Mebs and Pogoda, 2005; De Meester et al., 2021).
One of the more enigmatic toxins that amphibians possess is tetrodotoxin (TTX), a non-proteinaceous, low-molecular-weight neurotoxin found in both marine and terrestrial classes of animals (Bucciarelli et al., 2021). The species that possess TTX comprise 13 phyla of eukaryotes, the majority of which harbor TTX-producing bacteria (Bucciarelli et al., 2021). However, the mechanisms underlying TTX production are unclear. For all eukaryotes it is unclear whether or not TTX is a heritable phenotype, and, therefore, if the phenotype can respond to selection. Moreover, it is debated whether TTX is driven by symbionts and, if so, how hosts facilitate, maintain, or enforce bacterial production (Vaelli et al., 2020).
Newts (genus Taricha) possess TTX, harbor bacteria that produce TTX (Vaelli et al., 2020), display individual- and population-level fluctuations to TTX concentrations, and can be induced to increase their toxin concentrations (Hanifin et al., 1999; Hanifin et al., 2008; Bucciarelli et al., 2016; Bucciarelli et al., 2017). Previous research has suggested that the trait is a chemical defense phenotype that responds to selection under evolutionary, not ecological, timescales within a model of antagonistic “arms race” coevolution (Hanifin et al., 1999; Hanifin et al., 2008). In this study, we quantitated the total TTX concentrations from wild gravid or non-gravid females and males from 38 breeding populations across California to test if TTX is a sexually dimorphic trait. We also tested whether the toxin concentrations of both sexes fluctuate concordantly across sites over periods of months to years, and evaluated which factors influence total individual toxin concentrations. Collectively, the results of this study broaden our understanding of sexually dimorphic poison traits.
2 Materials and methods
2.1 Animal sampling
All animal-related research was approved by the Pepperdine Institutional Animal Care and Use Committees (IACUC) and conducted in accordance with the relevant guidelines, regulations and permits from the California Department of Fish and Wildlife (SC-12430).
The genus Taricha contains four species, three of which are endemic to California (T. torosa, T. sierrae, and T. rivularis) and a fourth that ranges from the central coast of California to Alaska (T. granulosa) (Figure 1A). The four species of Taricha exhibit sexually dimorphic secondary sexual characteristics, including size, weight, skin texture (coarse or smooth), and tail morphology, which were noted in the field prior to sampling and used to distinguish males from females during field data collection. Using the methods from Bucciarelli et al. (2014) we non-lethally collected a 2-mm radius of dorsolateral tissue from 858 sexually mature wild adults sampled at 38 sites during the annual breeding seasons from 2012 to 2020 (Table S1). Tissue was collected using a skin biopsy tool that was sterilized between individuals and replaced between sites. The newts were sampled only if they were in breeding condition in pools; no terrestrial newts were included. A combination of capture–mark–recapture methods were used to ensure that no adult was resampled within a breeding season. Each tissue sample was placed in 300 µL of 0.1 M aqueous acetic acid and held on dry ice before being transported to the laboratory, where they were stored at −80°C until extractions were completed. All the sampling tools were cleaned and sterilized between use and individuals.
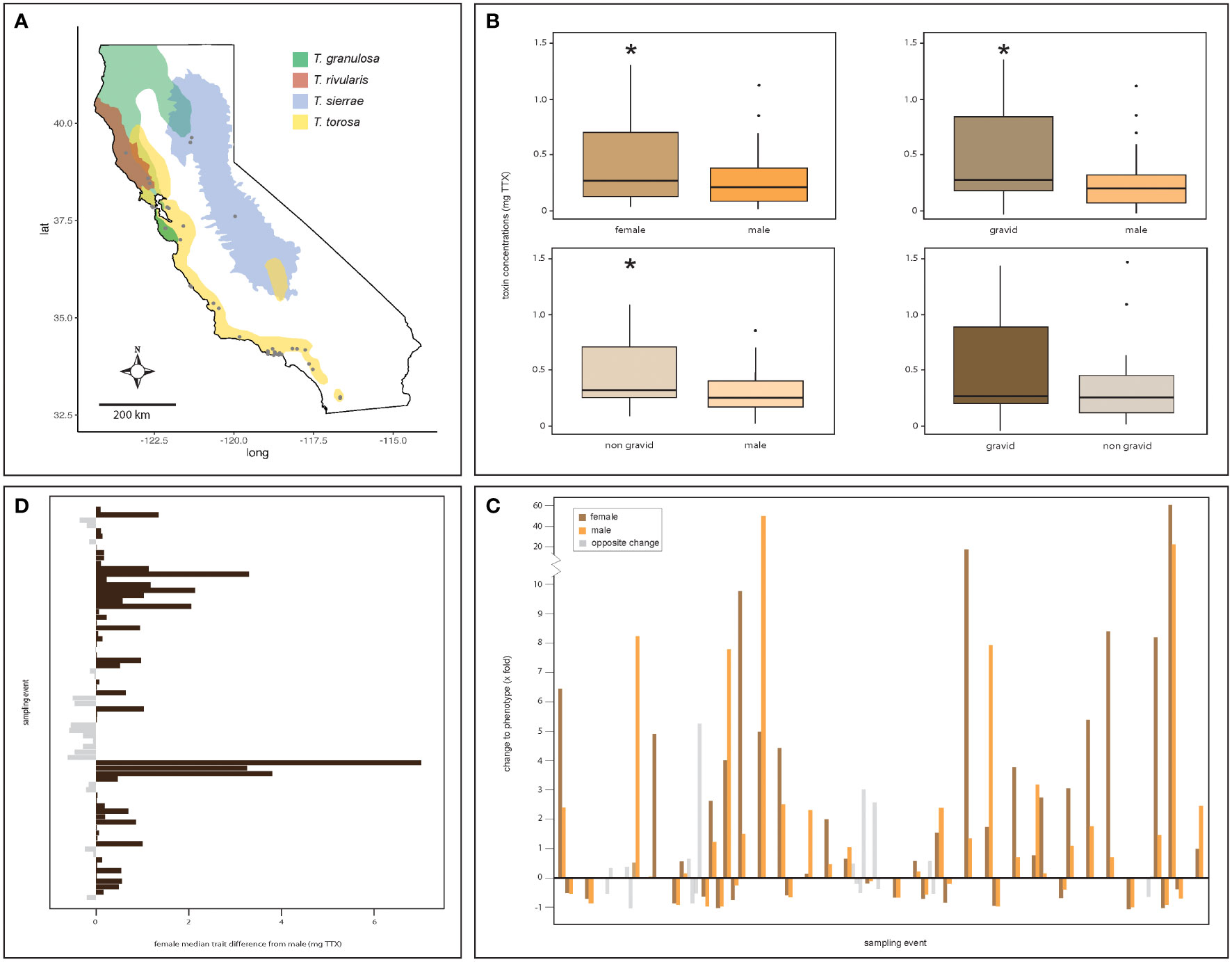
Figure 1 Strong evidence for female-biased sexual dimorphism in poisonous newts. Clockwise from upper left: (A) males and females of all four Taricha newt species sampled at sites (gray dots) distributed across California differ in their TTX concentrations with (B) females (n = 242) possessing greater TTX concentrations than males (n = 616) (Wilcoxon paired test, p < 0.001), regardless of reproductive status [Wilcoxon paired tests between males and females, gravid (n = 118, p = 0.002) or non-gravid (n = 124, p = 0.03)]. However, gravid and non-gravid females tend to have similar TTX concentrations (p = 0.75). (C) Evaluated over time, male and female toxin concentrations fluctuate and generally undergo concordant directional change within a site (n = 23 sites; each breeding site is demarcated by breaks in the data on the x-axis). (D) Females at breeding sites typically possess greater trait values relative to males, or, if they do not, are relatively close in value, underscoring the trait bias toward females. The asterisks denote statistical significance.
2.2 Tetrodotoxin extraction and quantification
The 2-mm tissue samples were weighed, macerated in their original 0.1 M acetic acid, boiled for 5 min, and then cooled for 5 min. The suspensions were centrifuged for 20 min in centrifugal filters [10,000 molecular weight cutoff (MWCO); Amicon® Ultra], rinsed with 100 µL of 0.1 M acetic acid, and centrifuged a second time for 20 min. The filtrate was collected and diluted to 1 mL with 0.1 M acetic acid (Bucciarelli et al., 2014). The extracts were immediately submitted for analysis by high-performance liquid chromatography with fluorescence detection (HPLC-FLD) or stored at −80°C for later analysis.
The HPLC-FLD consisted of an analytical pump (Shimadzu 20AD) equipped with an autosampler (SIL-20AC). The analytical column (SphereClone ODS 5 μm dp, 4.6 × 150 mm; Phenomenex®, Torrance, CA, USA) was protected with a SecurityGuard™ C18 guard cartridge (Phenomenex). Ion-pair chromatography was carried out using a mobile phase of 2 mM heptanesulfonic acid in 4 mM phosphate buffer, pH 6.00, at a flow rate of 0.35 mL min−1. A second HPLC pump, identical to the analytical pump, supplied 4 M NaOH at 0.35 mL min−1 to facilitate post-column derivatization. The eluent from the analytical column was mixed with 4 M NaOH at a PEEK mixing tee and passed through the post-column reactor (Analytical Scientific Instruments; model 310 equipped with a 500-mL reactor cartridge) thermostatted at 125°C. The derivatized components were determined by fluorescence detection (Waters model 474, Ex. 381 nm, Em. 505 nm). All HPLC solvents were continuously vacuum-degassed during the analysis. The TTX was quantitated by comparison of the TTX peak area to a rectilinear calibration curve. The individual TTX concentrations (mg/individual) were estimated using previously established methods (Hanifin et al., 2004).
2.3 Statistical methods
Possible sexually dimorphic traits of mass, tail height, and size [snout–vent length (SVL)] were assessed using Mann–Whitney tests on the full dataset (n = 858). Non-parametric tests were used due to non-normal data patterns driven by morphological differences between sexes, sites, and species. To test the associations between body condition and toxin concentrations, we regressed the ratio of mass and SVL [body mass index (BMI) or body condition] against total toxin data using linear mixed-effects models executed in R (The R Foundation for Statistical Computing, Vienna, Austria) using nlme (Pinheiro et al., 2017). The models were populated with an interaction term for sex and a random effect for the site. The toxin values were log-transformed to account for skewness.
To further assess potential differences in toxin concentrations between males and females, we calculated median TTX values at each breeding site for males (n = 616), all females (n = 242), gravid females only (n = 118), and non-gravid females only (n = 124). We used four individual Wilcoxon signed-rank tests to compare the paired median values between males and all females, males and gravid females, males and non-gravid females, and gravid and non-gravid females at a breeding site (Table S2). In each analysis, sites without a paired data point were omitted from the analysis.
For the sites where males and females of the population were sampled more than once (number of sites, n = 23), we evaluated if TTX concentrations of males and females tended to change in the same direction between sampling events. We counted the instances when both sexes showed the same direction of change in TTX concentrations from previous sampling events (increase or decrease) and used a chi-squared test to determine if these values were significantly different from random. To test for potential sex-biased trait patterns, we evaluated the difference between median female and male total TTX concentrations (mg TTX/individual) for each sampling event. We then enumerated the instances where female concentrations exceeded those of males and used a chi-squared test to determine if females possessed greater amounts of TTX.
The potential changes to toxin concentrations at sites over time by sex were evaluated using a general additive model (GAM). We calculated the number of elapsed days between the sampling events and used a smooth term for elapsed days between sampling events by sex coupled with a parametric term for sex. A random effect for breeding site was incorporated as a smooth term to account for recognized fine-scale geographic variation to the phenotype (Bucciarelli et al., 2016). The TTX data were fitted with a logistic function to account for skewness and a parameter estimation was performed with restricted maximum likelihood. The GAM was implemented in R using mgcv (Wood and Wood, 2015) then fitted, and the results were evaluated using the function gam.check. To evaluate phenotypic patterns further, we used a conditional inference tree to assess the importance and directionality of variables influencing TTX concentrations. Like the GAM, we fit a conditional inference tree using the function ctree in party (Hothorn et al., 2010), with terms for sex and continuous predictors for mass and SVL. Because two of the four species were sparsely sampled (Table S2), and because we sought to explore potential sex-based differences across the genus, we did not include a term for species in the model. Tree models implement recursive partitioning to identify predictors with the strongest association to the response variable, leading to binary splits of predictors that we used to evaluate the threshold values on the TTX phenotype.
3 Results
Overall, we found strong support to indicate there is sexual dimorphism of the toxin phenotype in newts. The sex-based differences were apparent for mass (Mann–Whitney, W = 49,136, p < 0.001), SVL (Mann–Whitney, W = 53,537, p < 0.001), and tail height (Mann–Whitney, W = 18,274, p < 0.001), and these factors were significant predictors of how mass, SVL, and sex interact to explain TTX concentrations (sex M × BMI: β = 2.02, t = 2.65, p = 0.008; sex M: β = –0.69, t = –4.28, p = 0.000; BMI: β = 0.64, t = 0.64, p = 0.52).
In general, we found that females within a population possessed greater concentrations of TTX than males. We observed significant differences between female and male total toxin concentrations in a breeding population (Wilcoxon signed-rank tests, p = 0.001, V = 154; Figure 1B, upper left panel), with median estimates of individual total TTX concentrations being greater in females. Regardless of breeding condition, the females tended to possess significantly different and typically greater concentrations of TTX (Wilcoxon signed-rank tests, gravid female : males, p = 0.002, V = 95; non-gravid females : males, p = 0.03, V = 120; Figure 1B, upper right and bottom left panels). However, female toxin concentrations did not significantly differ between gravid and non-gravid females given reproductive status (Wilcoxon signed-rank tests, gravid female : non-gravid females, p = 0.75, V = 137; Figure 1B, bottom right panel).
In the 23 sites where we sampled both sexes more than once, we evaluated total TTX concentrations over time by sex to determine if changes to the phenotype occurred in the same direction between sampling events for males and females. We found non-random phenotypic change between the sexes within sites [chi-squared test, χ2 = 20.48, p < 0.001, degrees of freedom (df) = 1; Figure 1C]. Specifically, females and males showed similar longitudinal patterns of change to total TTX concentrations; both sexes showed concordant changes to TTX concentrations within a site between sampling events in 41 out of 50 instances. A general comparison of median total TTX concentrations by sex for each sampling event showed that female TTX concentrations generally exceeded those of males, with females possessing greater concentrations of TTX in 50 out of 73 sampling events (chi-squared test, χ2 = 9.98, p = 0.001, df = 1; Figure 1D).
The GAM results confirmed that wild male and female TTX concentrations fluctuated between sampling events across the 23 sites. Evaluated by sex, the model showed significant variations in total TTX concentrations over time for both males [GAM, elapsed days : sex (M): effective degrees of freedom (edf) = 6.4, ref. df = 7.24, F = 7.02, p < 0.001; Figure 2A] and females (GAM, elapsed days : sex (F): edf = 3.89, ref. df = 4.37, F = 5.29, p < 0.001; Figure 2B). The magnitude of change to the phenotype was greater in females and significantly differed between sites (GAM: edf = 21.25, ref. df = 22.00, F = 30.69, p < 0.001). The conditional inference trees further explain the ways in which the BMI and sex covariates explain the patterns of toxin concentrations, with mass positively associated with greater TTX concentrations, regardless of sex. Specifically, body mass and TTX concentrations were positively correlated for individuals with a body mass > 28 g, whereas the trend was negative with smaller (< 57 mm) adults with less weight (< 20 g). These individuals had the lowest median toxin concentrations. For males and females > 57 mm in length and weighing < 20 g, larger males (SVL > 69 mm) had greater toxin concentrations, but female median TTX concentrations were greater than males’, regardless of size or mass (Figure 2C).
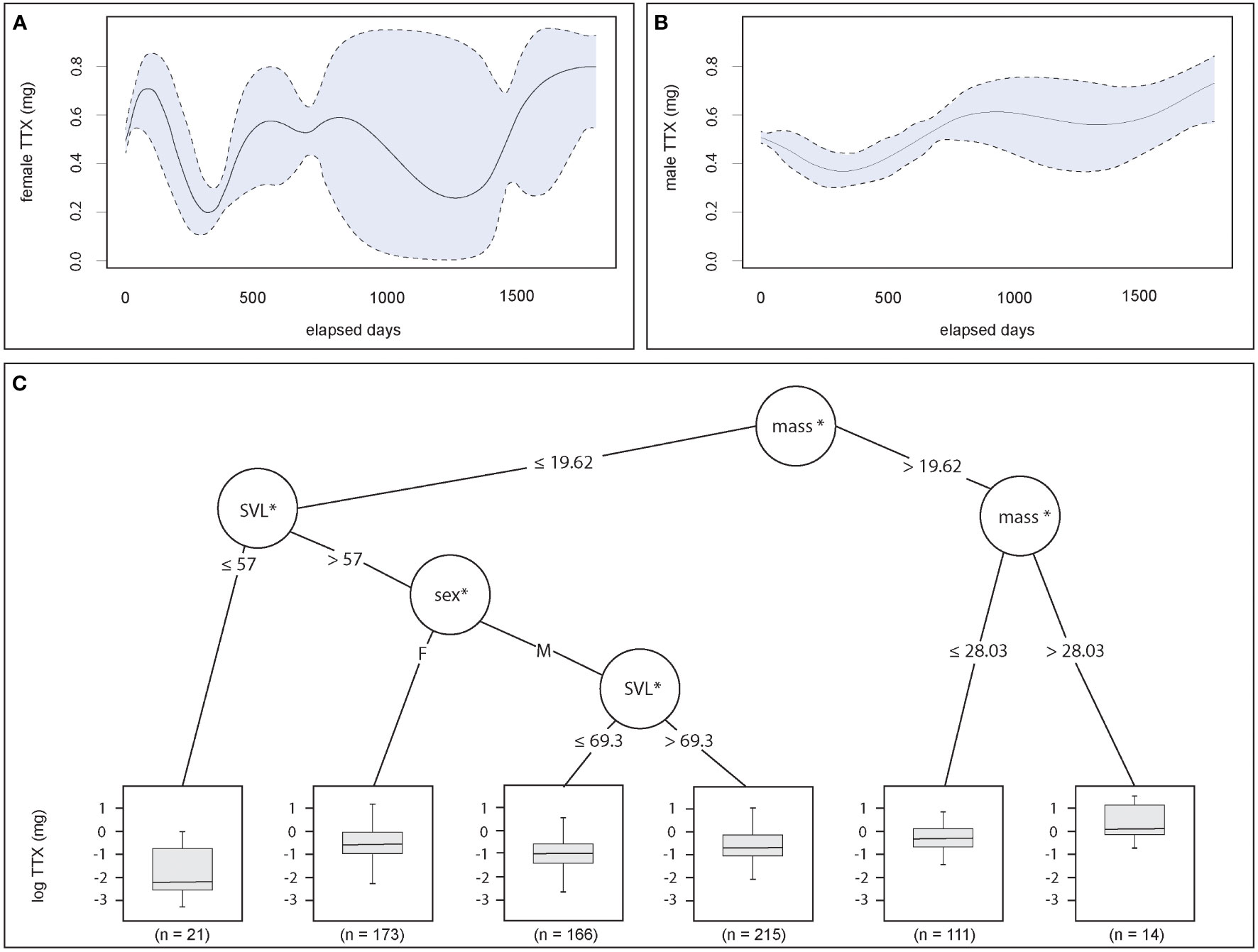
Figure 2 Size and sex influence the TTX phenotype. (A, B) Change to toxin concentrations (mg TTX per individual) occurs in female and male adults over relatively short temporal scales across breeding sites, and generally shifts more dramatically in females (GAM, smooth terms for elapsed time by sex; female: p < 0.001; male: p < 0.001). (C) Tree regression analysis split data at the first node by mass, indicating that no matter the sex, the largest individuals (mass > 19.62 g) had the highest toxin concentrations (log-transformed mg TTX per individual), and the smallest individuals generally had the lowest (mass < 19.62 g, SVL < 57 mm). Between these extremes, sex mattered. Females tended to have greater TTX concentrations independent of size, whereas males were split into size classes, with larger males (SVL > 69.3 mm) tending to exhibit greater toxin concentrations. The asterisks denote statistical significance.
4 Discussion
We aimed to determine (i) if TTX concentrations differed by sex and within females by reproductive status; (ii) if concentrations fluctuated and if they did so concordantly for both sexes over time; and (iii) whether or not morphological factors explain potential sexual dimorphism. The results of our analyses between gravid and non-gravid females and males from our 38 focal wild populations show that TTX is a sexually dimorphic trait. The toxin was generally more concentrated in females, regardless of reproductive status, and showed greater temporal change in concentrations. Females are highly vulnerable during protracted courtship and ovipositing behaviors, and greater investment in toxin production would be favored by selection to reduce predation risk, a strategy that has evolved in other poisonous animals (Start and De Lisle, 2018; Yamate et al., 2021). That females within a population generally possessed greater concentrations of TTX than males and that those concentrations tended to be similar between females regardless of reproductive status suggests that mechanisms related to toxin production may differ between sexes. One possibility is that females differentially invest resources critical to the TTX phenotype, a strategy that has been observed in poison frogs, moths, beetles, and octopi (Gonzalez et al., 1999; Stynoski et al., 2014; Winters et al., 2014; Start and De Lisle, 2018). Given that sex-biased mortality rates driven by predation pressure can result in sexually dimorphic traits (Christe et al., 2006), then females with increased TTX concentrations during the breeding season arguably have a greater chance of survival from predators, as would the offspring that are provided greater amounts of toxins by exceedingly TTX-laden females (Hanifin et al., 2003; Gall et al., 2012). If females confer TTX to eggs and there is an associated cost, fecundity selection could reinforce this process.
Alternatively, if newts do not produce TTX de novo, females may not shunt TTX to eggs but instead vertically transfer crucial TTX-producing bacteria to offspring. This may explain the similarity of toxin concentrations observed between gravid and non-gravid females, challenging the possibility that females supply sizeable amounts of TTX to eggs. For species that do not provide offspring care, there are limited opportunities for the vertical transfer of the parental microbiome (Bunker et al., 2021). Among Taricha newts, neither sex exhibits parental care after oviposition. Females stand to be a likely source of vertical transmission (Marshall et al., 1990), although it is possible that adults transfer symbionts in breeding pools to larvae (Mebs and Yotsu-Yamashita, 2021). The transmission of the microbiome, associated with parent–offspring phenotypes in other systems, may also explain the extreme difference between male and female values, especially because different bacterial groups are likely to contribute asymmetrically to the phenotype (Hughey et al., 2017; Brunetti et al., 2019). Although we have focused on maternal vertical transmission of the microbiome, it is also possible that components of male spermatophores contribute to the TTX phenotype, although this is less likely.
In addition, greater female toxin concentrations may be linked to skin traits. For example, females may possess a higher number of toxin glands per surface area of skin, glands could have a higher capacity for holding TTX, or females may produce greater amounts of TTX-binding proteins that facilitate gland accumulation of TTX products (Saporito et al., 2010; Chen et al., 2017; Mailho-Fontana et al., 2019). Amphibian skin contains four distinct gland types, of which granular glands are responsible for containing cutaneous substances that can be distasteful, noxious, or poisonous (Hanifin et al., 2002; Zamora-Camacho, 2022). A recent comparison of two populations found that one population generally exhibited high TTX concentrations and contained a greater number of type I cells in the granular glands that hold TTX in flocculate granular form. But, potential sexual dimorphism of type I cells was unaddressed in the eight adults examined in the study (Mailho-Fontana et al., 2019). In previous assessments of toxin concentrations in wild populations, measured sex-based differences were not apparent, but this is likely because sexual dimorphism within and between populations was not explicitly tested for or analyses were restricted to a single population (Hanifin et al., 2008; Stokes et al., 2011). Previous research also documented that TTX persists in captive newts and that captive females typically possess greater TTX concentrations than captive males (Hanifin et al., 2002). However, the mechanisms that drive sexual dimorphism remain largely undiscovered.
Remarkably, the toxin concentrations of both sexes tend to fluctuate concordantly across sites over periods of months to years. There are innumerable sexually dimorphic traits, but none that are poisonous, plastic, or temporally dynamic for both sexes. The few instances of unmatched directionality that we observed (Figure 2A) may be explained by delayed responsiveness of the phenotype and the time required to mount a response (Bucciarelli et al., 2017). Sites may also lack the resources essential for TTX production, whether the result of a de novo synthesis, symbionts, or processes associated with both (Bucciarelli et al., 2021). Numerous aspects of the TTX phenotype remain unresolved, including its origin, mechanisms of production, and whether it is heritable. The costs, if any, that are paid to bear TTX remain undetermined. However, our linear and tree model results show newt toxin concentrations interact with size, mass, and sex. This implies that there is a physiological price to pay for toxicity, which may be exacted by bacterial symbionts (Mone et al., 2014; Schwartzman et al., 2015) as opposed to costly internal processes. Previous work shows that experimentally induced elevated shifts to adult TTX concentrations led to reduced adult body mass, suggesting there are tradeoffs between the growth and maintenance of toxins (Bucciarelli et al., 2017).
We observed significantly greater and more drastically fluctuating TTX concentrations in females, which could facilitate mate attraction at breeding sites and possibly induce males to increase toxicity (Bucciarelli et al., 2016). Chemical communication is critical across amphibian life stages, especially reproduction (Secondi et al., 2005; Soong and Venkatesh, 2006), and females assess mates based on body size, tail height, and likely toxicity (Bucciarelli et al., 2016; Brunetti et al., 2019). Males also appear to rely on TTX as a chemical cue to select breeding sites and abandon pools that they have shown fidelity to in the past if their relative toxin concentration is inferior to other males (Bucciarelli et al., 2016). In sex-biased populations where males outnumber females, a mechanism of limited cost should exist that both assists males with finding mates quickly and engages females (Grayson et al., 2012; Woodley and Staub, 2021). Relative long-range communication is often costly, but readily available molecules can serve a secondary role in mate detection, thereby reducing costs (Rohr et al., 2004; Woodley, 2015). That TTX may serve an ecological role to facilitate reproductive processes and moderate competition between males would not be unusual, as TTX has been found to affect larval newt behavior (Elliott et al., 1993; Zimmer et al., 2006), snail feeding (Hwang et al., 2004), pufferfish mating (Matsumura, 1995; Noguchi et al., 2022), and benthic invertebrate foraging behavior and community dynamics (Bucciarelli and Kats, 2015). The evolution and ecology of TTX has largely focused on its role in chemical defense (Elliott et al., 1993; Gonzalez et al., 1999; Zimmer et al., 2006; Start and De Lisle, 2018), but its complex ecological roles are emerging (Vaelli et al., 2020; Bucciarelli et al., 2021).
The eco–evo underpinnings of toxins in newts have largely been cast within a model of antagonistic coevolution between TTX-bearing newts and resistant snake predators, leading to an arms race (Hanifin et al., 2008; Feldman et al., 2012). Absent from this model has been a consideration of plasticity, and, now, ways in which toxin sexual dimorphism may affect evolutionary dynamics (Bucciarelli et al., 2021). The genes associated with snake resistance appear to have evolved at a pace that outcompetes newt toxicity, leading to a mismatch between populations that always shows snakes ahead in the arms race (Hanifin et al., 2008). But our results argue that the current characterization of mismatch lacks a full consideration of female toxicity. Incorporating female toxicity, inducibility, and temporal variation may require a new evolutionary model to account for the TTX phenotype, specifically one that includes roles for bacterial symbionts and sensing of environmental conditions by hosts and microbes in the system (Hanifin et al., 2003). This model, which we refer to as “information coevolution” (Bucciarelli et al., 2022) provides a framework for the host and symbiont to interact with one another, as well as abiotic and biotic conditions of the environment. Sexual dimorphism of TTX concentrations occurs in newts and such sex-biased phenotypic variation may have critical relevance to ecological and evolutionary processes. There is much to be uncovered, particularly the implications for mating behaviors and sexual selection broadly. Collectively, our results indicate that sex matters in this group of toxin-bearing amphibians.
Data availability statement
The original contributions presented in the study are included in the article/Supplementary Material. Further inquiries can be directed to the corresponding author.
Ethics statement
The animal study was approved by the Pepperdine Institutional Animal Care and Use Committee. The study was conducted in accordance with the local legislation and institutional requirements.
Author contributions
AF: Conceptualization, Formal analysis, Methodology, Visualization, Writing – original draft, Writing – review & editing. GB: Conceptualization, Formal analysis, Methodology, Supervision, Visualization, Writing – original draft, Writing – review & editing. DH: Formal analysis, Methodology, Writing – review & editing. LK: Conceptualization, Funding acquisition, Methodology, Project administration, Writing – review & editing. DG: Conceptualization, Funding acquisition, Methodology, Resources, Supervision, Writing – review & editing.
Funding
The author(s) declare financial support was received for the research, authorship, and/or publication of this article. AF was supported by the Stauffer Fellowship through Pepperdine from the Natural Science Division. Funding was provided by the Office of the Provost to support field data collection.
Acknowledgments
The authors are grateful to D. Green and M. Kruel for their technical support. We thank the National Park Services and California State Parks for allowing us access to their land. We also thank Andy Lin for his statistical advice and support.
Conflict of interest
The authors declare that the research was conducted in the absence of any commercial or financial relationships that could be construed as a potential conflict of interest.
The author(s) GB and LK declare that they were editorial board members of Frontiers, at the time of submission. This had no impact on the peer-review process and the final decision.
Publisher’s note
All claims expressed in this article are solely those of the authors and do not necessarily represent those of their affiliated organizations, or those of the publisher, the editors and the reviewers. Any product that may be evaluated in this article, or claim that may be made by its manufacturer, is not guaranteed or endorsed by the publisher.
Supplementary material
The Supplementary Material for this article can be found online at: https://www.frontiersin.org/articles/10.3389/famrs.2023.1279848/full#supplementary-material
References
Aoto T. (1950). A remarkable swelling of male skin of a salamander (Hynotius retardatus dunn) in the breeding season. J. Faculty Science Hokkaido Univ. 10, 45–53.
Brunetti A. E., Lyra M. L., Melo W. G. P., Andrade L. E., Palacios-Rodriguez P., Prado B. M., et al. (2019). Symbiotic skin bacteria as a source for sex-specific scents in frogs. Proc. Natl. Acad. Sci. U.S.A. 116, 2124–2129. doi: 10.1073/pnas.1806834116
Bucciarelli G. M., Alsalek F., Kats L. B., Green D. B., Shaffer H. B. (2022). Toxic relationships and arms-race coevolution revisited. Annu. Rev. Anim. Biosci. 10, 63–80. doi: 10.1146/annurev-animal-013120-024716
Bucciarelli G. M., Green D. B., Shaffer H. B., Kats L. B. (2016). Individual fluctuations in toxin levels affect breeding site fidelity in a chemically defended amphibian. Proc. Biol. Sci. 283, 283–291. doi: 10.1098/rspb.2016.0468
Bucciarelli G. M., Kats L. B. (2015). Effects of newt chemical cues on the distribution and foraging behavior of stream macroinvertebrates. Hydrobiologia 749, 69–81. doi: 10.1007/s10750-014-2146-4
Bucciarelli G. M., Lechner M., Fontes A., Kats L. B., Eisthen H. L., Shaffer H. B. (2021). From poison to promise: the evolution of tetrodotoxin and its potential as a therapeutic. Toxins 13, 517. doi: 10.3390/toxins13080517
Bucciarelli G. M., Li A., Kats L. B., Green D. B. (2014). Quantifying tetrodotoxin levels in the California newt using a non-destructive sampling method. Toxicon 80, 87–93. doi: 10.1016/j.toxicon.2014.01.009
Bucciarelli G. M., Shaffer H. B., Green D. B., Kats L. B. (2017). An amphibian chemical defense phenotype is inducible across life history stages. Sci. Rep. 7, 8185. doi: 10.1038/s41598-017-08154-z
Bunker M. E., Elliott G., Heyer-Gray H., Martin M. O., Arnold A. E., Weiss S. L. (2021). Vertically transmitted microbiome protects eggs from fungal infection and egg failure. Anim. Microbiome 3, 43. doi: 10.1186/s42523-021-00104-5
Chen W., Hudson C. M., DeVore J. L., Shine R. (2017). Sex and weaponry: The distribution of toxin-storage glands on the bodies of male and female cane toads (Rhinella marina). Ecol. Evol. 7, 8950–8957. doi: 10.1002/ece3.2914
Christe P., Keller L., Roulin A. (2006). The predation cost of being a male: implications for sex-specific rates of ageing. Oikos 114, 381–384. doi: 10.1111/j.2006.0030-1299.15130.x
Daly J. W., Garraffo H. M., Spande T. F., Giddings L.-A., Saporito R. A., Vieites D. R., et al. (2008). Individual and geographic variation of skin alkaloids in three species of madagascan poison frogs (Mantella). J. Chem. Ecol. 34, 252–279. doi: 10.1007/s10886-007-9396-9
De Meester G., Sunje E., Prinsen E., Verburggen E., Van Damme R. (2021). Toxin variation among salamander populations: discussing potential causes and future directions. Integr. Zoology 16, 336–353. doi: 10.1111/1749-4877.12492
Elliott S. A., Kats L. B., Breeding J. A. (1993). The use of conspecific chemical cues for cannibal avoidance in california newts (Taricha torosa). Ethology 95, 186–192. doi: 10.1111/j.1439-0310.1993.tb00469.x
Emlen D. J., Marangelo J., Ball B., Cunningham C. W. (2007). Diversity in the weapons of sexual selection: horn evolution in the beetle genus Onthophagus (Coleoptera: Scarabaeidae). Evolution 59, 1060–1084. doi: 10.1111/j.0014-3820.2005.tb01044.x
Feldman C. R., Brodie E. D. Jr., Brodie E. D. 3rd, Pfrender M. E. (2012). Constraint shapes convergence in tetrodotoxin-resistant sodium channels of snakes. Proc. Natl. Acad. Sci. U.S.A. 109, 4556–4561. doi: 10.1073/pnas.1113468109
Gall B. G., Stokes A. N., French S. S., Brodie E. D. 3rd, Brodie E. D. Jr. (2012). Female newts (Taricha granulosa) produce tetrodotoxin laden eggs after long term captivity. Toxicon 60, 1057–1062. doi: 10.1016/j.toxicon.2012.07.017
Gonzalez A., Rossini C., Eisner M., Eisner T. (1999). Sexually transmitted chemical defense in a moth (Utetheisa ornatrix). Proc. Natl. Acad. Sci. U.S.A. 96, 5570–5574. doi: 10.1073/pnas.96.10.5570
Grayson K. L., De Lisle S. P., Jackson J. E., Black S. J., Crespi E. J. (2012). Behavioral and physiological female responses to male sex ratio bias in a pond-breeding amphibian. Front. Zool 9, 24. doi: 10.1186/1742-9994-9-24
Hanifin C. T., Brodie E. D. 3rd, Brodie E. D. Jr. (2002). Tetrodotoxin levels of the rough-skin newt, Taricha granulosa, increase in long-term captivity. Toxicon 40, 1149–1153. doi: 10.1016/s0041-0101(02)00115-0
Hanifin C. T., Brodie E. D. 3rd, Brodie E. D. Jr. (2003). Tetrodotoxin levels in eggs of the rough-skin newt, Taricha granulosa, are correlated with female toxicity. J. Chem. Ecol. 29, 1729–1739. doi: 10.1023/a:1024885824823
Hanifin C. T., Brodie E. D. 3rd, Brodie E. D. Jr. (2004). A predictive model to estimate total skin tetrodotoxin in the newt Taricha granulosa. Toxicon 43, 243–249. doi: 10.1016/j.toxicon.2003.11.025
Hanifin C. T., Brodie E. D., Brodie E. D. (2008). Phenotypic mismatches reveal escape from arms-race coevolution. PloS Biol. 6, e60. doi: 10.1371/journal.pbio.0060060
Hanifin C. T., Yotsu-Yamashita M., Yasumoto T., Brodie E. D., Brodie E. D. (1999). Toxicity of dangerous prey: variation of tetrodotoxin levels within and among populations of the newt Taricha granulosa. J. Chem. Ecol. 25, 2161–2175. doi: 10.1023/A:1021049125805
Hothorn T., Hornik K., Strobl C., Zeileis A. (2010). Party: A laboratory for recursive partytioning.
Hughey M. C., Delia J., Belden L. K. (2017). Diversity and stability of egg-bacterial assemblages: The role of paternal care in the glassfrog Hyalinobatrachium colymbiphyllum. Biotropica 49, 792–802. doi: 10.1111/btp.12461
Hwang P. A. I., Noguchi T., Hwang D.-F. (2004). Neurotoxin tetrodotoxin as attractant for toxic snails. Fisheries Sci. 70, 1106–1112. doi: 10.1111/j.1444-2906.2004.00911.x
Jeckel A. M., Saporito R. A., Grant T. (2015). The relationship between poison frog chemical defenses and age, body size, and sex. Front. Zool 12, 27. doi: 10.1186/s12983-015-0120-2
Lindenfors P., Gittleman J., Jones K. (2007). Sexual size dimorphism in mammals. In: Evolutionary Studies of Sexual Size Dimorphism. 16–26. doi: 10.1093/acprof:oso/9780199208784.003.0003
Mailho-Fontana P. L., Jared C., Antoniazzi M. M., Sciani J. M., Pimenta D. C., Stokes A. N., et al. (2019). Variations in tetrodotoxin levels in populations of Taricha granulosa are expressed in the morphology of their cutaneous glands. Sci. Rep. 9, 18490. doi: 10.1038/s41598-019-54765-z
Marshall C. J., Doyle L. S., Kaplan R. H. (1990). Intraspecific and Sex-Specific Oophagy in a Salamander and a Frog: Reproductive Convergence of Taricha torosa and bombina orientalis. Herpetologica 46, 395–399.
Matsumura K. (1995). Reexamination of tetrodotoxin production by bacteria. Appl. Environ. Microbiol. 61, 3468–3470. doi: 10.1128/aem.61.9.3468-3470.1995
Mebs D., Pogoda W. (2005). Variability of alkaloids in the skin secretion of the European fire salamander (Salamandra salamadra terrestris). Toxicon 45, 603–606. doi: 10.1016/j.toxicon.2005.01.001
Mebs D., Yotsu-Yamashita M. (2021). Acquiring toxicity of a newt, Cynops orientalis. Toxicon 198, 32–35. doi: 10.1016/j.toxicon.2021.04.025
Mone Y., Monnin D., Kremer N. (2014). The oxidative environment: a mediator of interspecies communication that drives symbiosis evolution. Proc. Biol. Sci. 281, 20133112. doi: 10.1098/rspb.2013.3112
Mori E., Mazza G., Lovari S. (2017). Sexual dimorphism. In: Encyclopedia of Animal Cognition and Behavior. 1–7. doi: 10.1007/978-3-319-47829-6_433-1
Noguchi Y., Suzuki T., Matsutani K., Sakakibara R., Nakahigashi R., Adachi M., et al. (2022). An almost nontoxic tetrodotoxin analog, 5,6,11-trideoxytetrodotoxin, as an odorant for the grass puffer. Sci. Rep. 12, 15087. doi: 10.1038/s41598-022-19355-6
Pinheiro J., Bates D., DebRoy S., Sarkar D., Heisterkamp S., Van Willigen B., et al. (2017). Package ‘nlme’. Linear and nonlinear mixed effects models, version 3, 1, 274.
Pogoda P., Kupfer A. (2018). Flesh and bone: An integrative approach towards sexual size dimorphism of a terrestrial salamander (genus Salamandrina). J. Morphol 279, 1468–1479. doi: 10.1002/jmor.20883
Preißler K., Gippner S., Lüddecke T., Krause E. T., Schulz S., Vences M., et al. (2019). More yellow more toxic? Sex rather than alkaloid content is correlated with yellow coloration in the fire salamander. J. Zoology 308, 293–300. doi: 10.1111/jzo.12676
Rohr J. R., Park D., Sullivan A. M., McKenna M., Propper C. R., Madison D. M. (2004). Operational sex ratio in newts: field responses and characterization of a constituent chemical cue. Behav. Ecol. 16, 286–293. doi: 10.1093/beheco/arh164
Saporito R. A., Donnelly M. A., Garraffo H. M., Spande T. F., Daly J. W. (2006). Geographic and seasonal variation in alkaloid-based chemical defenses of Dendrobates pumilio from Bocas del Toro, Panama. J. Chem. Ecol. 32, 795–814. doi: 10.1007/s10886-006-9034-y
Saporito R. A., Donnelly M. A., Madden A. A., Garraffo H. M., Spande T. F. (2010). Sex-related differences in alkaloid chemical defenses of the dendrobatid frog Oophaga pumilio from Cayo Nancy, Bocas del Toro, Panama. J. Nat. Prod 73, 317–321. doi: 10.1021/np900702d
Scharer L., Rowe L., Arnqvist G. (2012). Anisogamy, chance and the evolution of sex roles. Trends Ecol. Evol. 27, 260–264. doi: 10.1016/j.tree.2011.12.006
Schwartzman J. A., Koch E., Heath-Heckman E. A., Zhou L., Kremer N., McFall-Ngai M. J., et al. (2015). The chemistry of negotiation: rhythmic, glycan-driven acidification in a symbiotic conversation. Proc. Natl. Acad. Sci. U.S.A. 112, 566–571. doi: 10.1073/pnas.1418580112
Secondi J., Haerty W., Lodé T. (2005). Female attraction to conspecific chemical cues in the palmate newt Triturus helveticus. Ethology 111, 726–735. doi: 10.1111/j.1439-0310.2005.01096.x
Shine R. (1979). Sexual selection and sexual dimorphism in the amphibia. Copeia 1979, 297–306. doi: 10.2307/1443418
Soong T. W., Venkatesh B. (2006). Adaptive evolution of tetrodotoxin resistance in animals. Trends Genet. 22, 621–626. doi: 10.1016/j.tig.2006.08.010
Start D., De Lisle S. (2018). Sexual dimorphism in a top predator (Notophthalmus viridescens) drives aquatic prey community assembly. Proc. Biol. Sci. 285, 285–291. doi: 10.1098/rspb.2018.1717
Staub N. L., Julie P. (1997). The presence of modified granular glands in male and female aneides lugubris (Amphibia: plethodontidae). Herpetologica 53, 339–344.
Stokes A. N., Cook D. G., Hanifin C. T., Brodie E. D. (2011). Sex-biased predation on newts of the genus taricha by a novel predator and its relationship with tetrodotoxin toxicity. Am. Midland Nat. 165, 389–399. doi: 10.1674/0003-0031-165.2.389
Stynoski J. L., Torres-Mendoza Y., Sasa-Marin M., Saporito R. A. (2014). Evidence of maternal provisioning of alkaloid-based chemical defenses in the strawberry poison frog Oophaga pumilio. Ecology 95, 587–593. doi: 10.1890/13-0927.1
Vaelli P. M., Theis K. R., Williams J. E., O’Connell L. A., Foster J. A., Eisthen H. L. (2020). The skin microbiome facilitates adaptive tetrodotoxin production in poisonous newts. eLife 9, e53898. doi: 10.7554/eLife.53898
Williams T. M., Carroll S. B. (2009). Genetic and molecular insights into the development and evolution of sexual dimorphism. Nat. Rev. Genet. 10, 797–804. doi: 10.1038/nrg2687
Winters A. E., Stevens M., Mitchell C., Blomberg S. P., Blount J. D. (2014). Maternal effects and warning signal honesty in eggs and offspring of an aposematic ladybird beetle. Funct. Ecol. 28, 1187–1196. doi: 10.1111/1365-2435.12266
Woodley S. (2015). Chemosignals, hormones, and amphibian reproduction. Horm. Behav. 68, 3–13. doi: 10.1016/j.yhbeh.2014.06.008
Woodley S. K., Staub N. L. (2021). Pheromonal communication in urodelan amphibians. Cell Tissue Res. 383, 327–345. doi: 10.1007/s00441-020-03408-1
Yamate Y., Takatani T., Takegaki T. (2021). Levels and distribution of tetrodotoxin in the blue-lined octopus Hapalochlaena fasciata in Japan, with special reference to within-body allocation. J. Molluscan Stud. 87, 279–90. doi: 10.1093/mollus/eyaa042
Zamora-Camacho F. J. (2022). Sex and habitat differences in size and coloration of an amphibian’s poison glands match differential predator pressures. Integr. Zool 17, 764–776. doi: 10.1111/1749-4877.12597
Keywords: tetrodotoxin, Taricha torosa, sexual dimorphism, phenotypic variation, plasticity
Citation: Frey AR, Bucciarelli GM, Hu DD, Kats LB and Green DB (2023) An amphibian toxin phenotype is sexually dimorphic and shows seasonal concordant change between sexes. Front. Amphib. Reptile Sci. 1:1279848. doi: 10.3389/famrs.2023.1279848
Received: 18 August 2023; Accepted: 26 October 2023;
Published: 28 November 2023.
Edited by:
Anssi Laurila, Uppsala University, SwedenReviewed by:
János Ujszegi, Hungarian Academy of Sciences, HungaryHenrik Lauridsen, Aarhus University, Denmark
Copyright © 2023 Frey, Bucciarelli, Hu, Kats and Green. This is an open-access article distributed under the terms of the Creative Commons Attribution License (CC BY). The use, distribution or reproduction in other forums is permitted, provided the original author(s) and the copyright owner(s) are credited and that the original publication in this journal is cited, in accordance with accepted academic practice. No use, distribution or reproduction is permitted which does not comply with these terms.
*Correspondence: Gary M. Bucciarelli, Z2FyeWJAdWNkYXZpcy5lZHU=
†Present address: Gary M. Bucciarelli, Department of Wildlife, Fish, and Conservation Biology, UC Davis, University of California, Davis, CA, United States
‡These authors have contributed equally to this work