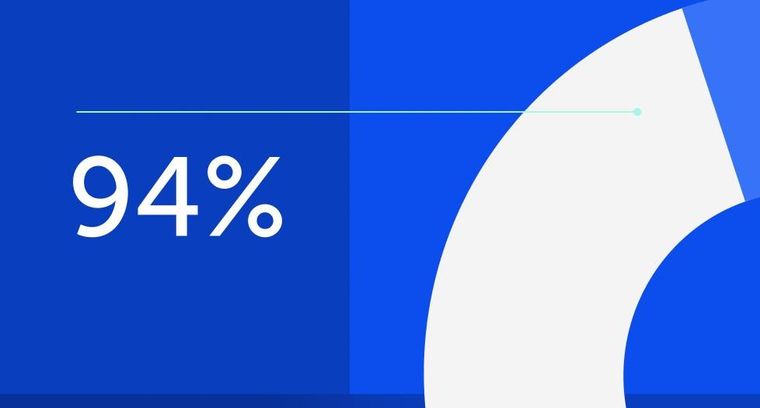
94% of researchers rate our articles as excellent or good
Learn more about the work of our research integrity team to safeguard the quality of each article we publish.
Find out more
ORIGINAL RESEARCH article
Front. Amphib. Reptile Sci., 29 March 2023
Sec. Behavior and Ecology
Volume 1 - 2023 | https://doi.org/10.3389/famrs.2023.1129253
This article is part of the Research TopicEditor's Showcase: Behavior, Evolution and EcologyView all 3 articles
The Colorado checkered whiptail (Aspidoscelis neotesselatus) is a parthenogenetic lizard that is listed as a “species of special concern” in the state of Colorado. A. neotesselatus occupies a small range that includes the US Army Fort Carson Military Base in Colorado Springs, Colorado. The species is exposed to a variety of military disturbances, including aircraft flyover noise. We sampled 82 females during the 2021 reproductive season to assess whether scheduled flyovers would impact the behavior, stress, and metabolism of A. neotesselatus, while controlling for size and reproductive stage differences. We measured corticosterone (CORT) as a marker of anthropogenically induced stress during flyovers compared to a control. We further tested for the downstream effects of flyovers on plasma glucose (free energy available to tissues), elevated metabolism with oxidative stress (ROMs), and ketone bodies (alternative cerebral energy substrates to glucose). When disturbed by flyovers, these lizards spent less time moving but more time eating. Aircraft noise also increased CORT when controlling for clutch size, indicating a stress response driven by flyovers, as well as an independent effect of reproductive investment on CORT. CORT did not affect plasma glucose. Flyovers led to a marginally decrease in circulating ROMs, with gravid females experiencing lower plasma ROMs than non-gravid females, but that later effect was independent of flyovers. Flyovers significantly increased ketone bodies, with smaller animals experiencing higher ketone concentrations than larger individuals, yet the effect of size on ketone bodies was independent of the flyover treatment. Although A. neotesselatus seem to adjust their behavior and eat more to buffer the potentially negative effect of flyovers on energetic pathways, they still appear to suffer a metabolic cost driven by the stress response via ketone accumulation, as well as a reproductive cost driven by clutch size investment that is independent of flyover disturbance. We suggest that military aircraft operators attempt to avoid dense populations of A. neotesselatus during the reproductive season or fly at altitudes that lead to decibel reads that fall below 50 dB at ground level, as a cautious management step that ensures the resilience and local abundance of A. neotesselatus at Fort Carson.
Reptiles are on the decline worldwide and vulnerable to many anthropogenic threats (Gibbons et al., 2000). Habitat loss and degradation, introduced invasive species, environmental pollution, disease and parasitism, unsustainable land use, and global climate change all have profound, detrimental impacts on reptile populations (Gibbons et al., 2000). Human factors have greatly increased the need for long-term monitoring and conservation efforts aimed at improving the trajectories of native reptile populations (Todd et al., 2010; Tan et al., 2023). Most of these threats are obvious in their impacts on reptile population viability; habitat loss, including fragmentation and land use conversion, is thought to be the leading cause of decline for reptile populations (Amburgey et al., 2021; Sala et al., 2000). Habitat loss can impact reptiles directly through mortality events, or indirectly by limiting their ability to meet survival and reproductive ends (e.g. Todd and Andrews, 2008). Shifts in niche space driven by climate change are predicted to be a further detriment to habitat suitability by shifting physiology, life history traits, and population distribution. This may ultimately lead to extinctions directly related to increasing temperature and decreasing precipitation (e.g. Dunham and Overall, 1994).
There are additional threats that have received less attention, likely due to how overlooked anthropogenic disturbance may be to human perception. However, “minor” disturbances may have dramatic impacts on wildlife. Light and noise pollution are amongst the fastest growing, but least understood, anthropogenic stressors on the planet (Dominoni et al., 2020; Buxton et al., 2017; Kyba et al., 2017), altering sensory environments at a global scale. While anthropogenic light sources affect visual perception, noise reduces the ability to receive other acoustic signals and may cause distress. These sensory disturbances can, in turn, impact communication (e.g. Francis & Barber, 2013), movement (e.g. Cabrera-Cruz et al., 2018), foraging (e.g. Bennie et al., 2015), energetic budgets (e.g. Touzot et al., 2019), predation risk (Francis & Barber, 2013), and stress (e.g. Ouyang et al., 2018) within wild animal populations. Anthropogenic changes such as these are often telling of the pathways by which an individual organism can be negatively influenced. As sound and light pollution continues to expand over space and time, there is an urgent need to understand how such sensory pollutants affect physiology, life history traits, and fitness (Kernbach et al., 2021).
Anthropogenic noise is often louder and more frequent than natural sounds (Patricelli & Blickley, 2006; Popper & Hastings, 2009). Sound or noise, especially those that are intense and unpredictable, can negatively affect animals (e.g. Warren et al., 2006; Slabbekoorn and Ripmeester, 2008; Popper and Hastings, 2009; Barber et al., 2010; Slabbekoorn et al., 2010; Shannon et al., 2016), and lead to a stress response (Jakob-Hoff et al., 2019). The release of adrenal corticosteroids in response to a stressor is well-documented across vertebrates. Stimulation of the hypothalamus during a stressful event leads to the release of CORT from the adrenal gland. While the primary function of CORT is to mobilize energy for the central nervous system, it has also been found to be an accurate indicator of a systemic stress response. Anthropogenic noise can initiate a hormonal stress response by elevating circulating glucocorticoids, “GCs”, in birds (Hayward et al., 2011; Blickley et al., 2012), mammals (Creel et al., 2002), amphibians (Tennessen et al., 2014; Kaiser et al., 2015) and fishes (Wysocki et al., 2006; Nichols et al., 2015; Mills et al., 2020), but not systematically (Crino et al., 2013; Angelier et al., 2016).
Anthropogenic noise may also lead to shifts in behaviors that trigger a stress response or prove maladaptive in other ways. In a study of the eastern blue-tongued lizard, individuals spent more time in a “freezing” behavior when exposed to high frequency mining machinery noise, a sign of chronic stress (Mancera et al., 2017). With more unconventional types of noise pollution, green, flatback and hawksbill turtles showed disturbance behaviors in response to drones flying at low elevations (20-30m) (Bevan et al., 2018). Traffic noise increased levels of a stress-relevant glucocorticoid in female wood frogs (Lithobates sylvaticus) and impaired female travel towards a male breeding chorus in the field (Tennessen et al., 2014). Behavioral responses to noise pollution often translate into stress responses, as loud noise increase cortisol levels in several species (Hall 2010, for a review see Kight & Swaddle, 2011).
Noise-related stress can shift behaviors and activity budgets by increasing vigilance (i.e. more time spent retreating or hiding), which can ultimately result in less time spent foraging. Reduction in foraging activity and the potential for associated weight loss are predicted to increase metabolic processing and mobilization of cholesterol to fuel the production of stress hormones; when stressed, individuals rapidly use stored resources to regulate neuroendocrine responses to said stress (Kight and Swaddle, 2011). Anthropogenic noise disturbance increased serum glucose, cortisol, and lactate in European sea bass (Santulli et al., 1999) and oxygen consumption in shore crabs (Wale et al., 2013), suggesting that anthropogenic noise my increase metabolic rate by increasing both physical activity and energy consumption.
Noise-induced stress also appears to affect females disproportionately, most likely because of sex differences in size, hormone expression, and reproductive investment (Kight and Swaddle, 2011). In this study, we propose to quantify the impact of flyover noise pollution on the behavior, stress physiology and metabolism of the Colorado checkered whiptail, Aspidoscelis neotesselatus, a parthenogenic, all-female, whiptail species whose range mostly overlaps the Fort Carson military installation near Colorado Springs, Colorado, USA. The reduced genetic variation of this parthenogenetic species, made up entirely of female congeners, could increase susceptibility to environmental disturbances (Aubry et al., 2019; Aubry et al., 2020; Caracalas et al., 2021). To-date, the species has had multiple conservation listings, likely due to its small natural range. It is designated as near threatened by the International Union for Conservation of Nature (IUCN 2007), deemed a species of special concern by Colorado Parks and Wildlife, and listed by the U.S. Army as a species at risk.
Fort Carson Military Base houses both military and air force units and their aircraft. This includes but is not limited to Apache, Chinook, and Blackhawk helicopters, transport aircraft, and F-16 fighter jets. A. neotesselatus on Fort Carson are exposed to regular aircraft flyovers within 20,000 feet above ground with a reported max decibel capability of 161dB produced during operation. This decibel range has been reported as harmful and/or agitating to humans, even at a substantial distance from the source (Nykaza et al., 2009). Mexican Spotted owls have also shown to initiate a significant response to noise disturbance as low as 68 decibels (Pater et al., 2009). We measured the behavior, stress, and metabolic response of A. neotesselatus by comparing a control versus flyover treatment, each a week in length, during a flyover experiment. We specifically measured i) activity budgets to document behavioral responses to flyovers; ii) the stress response in activating corticosterone release (‘CORT’); iii) the downstream effects of stress on glucose; iv) oxidative reactive oxygen metabolites (‘ROMs’) as an index of oxidative stress level (oxidant capacity); and v) ketone bodies, which can increase when the brain demands extra glucose from the body in times of stress. We investigated the effect of flyovers on (ii-v) while controlling for differences in size, weight, and reproductive state (Conceptual Figure 1). We hypothesized that anthropogenic disturbance in the form of military aircraft flyover events would have significant behavioral and physiological impacts on the Colorado checkered whiptail population via behavioral responses and shifts in energetic expenditures related to the sensory stress driven by noise pollution.
Figure 1 Conceptual energetic budget allocation of A. neotesselatus following noise disturbance (i.e. flyover treatment) when resources are partitioned into multiple competing processes involving a behavioral response, energy mobilizing hormones, and shifts in metabolic allocation. Internal resources are represented in the right column across a gradient with high internal resource indicated in green and low internal resources indicated in red. Resources are expected to diminish with the initiation of multiple physiological processes in the setting of noise induced physiological and behavioral changes.
Data were collected from A. neotesselatus lizards (Figure 2) living on the Fort Carson (FC) U.S. Army Installation located in unincorporated El Paso County, Colorado, near the city of Colorado Springs, USA. FC covers 55,000 hectares and extends into Pueblo and Fremont Counties. A. neotesselatus was sampled and surveyed in the northern edge of its range in FC. Lizards were surveyed and sampled in 2021 for the purpose of this specific study, which is part of a longer-term study that was initiated in 2018. The species is known to occur in several different training areas (TAs) (Figure 2), but we specifically surveyed TA 55 in coordination with military activities where our liaison biologist at FC scheduled aircraft flyovers on specific days over the study period, so that we could time our surveys with respect to flyovers.
Figure 2 Surveys of A. neotesselatus conducted in 1991-2007 (yellow dots), 2007-2013 (green dots), and 2014 (red dots) at Fort Carson, CO. Surveys used in this study (2018-2019) are represented by blue dots, with intensive sampling focused on TA45, TA48, and TA55.
In TA 55, the majority of A. neotesselatus were found in and along the banks of a dry creek bed. The dominating vegetation type was One-seeded Juniper (Juniperus monosperma) and mixed grassland, located around the edges of the sample area. The shrubs found included Shadscale (Atriplex confertifolia), Four-wing Saltbush (Atriplex canescens), James’ Seaheath (Frankenia jamesii) and Rubber Rabbitbrush (Chrysothamnus nauseosus).
Military aircraft flown over the field site included UH-60 Black Hawks, AH-64 Apaches, CH-47 Chinooks, and F-16 fighter jets. Given that helicopter range from the ground during flight is highly variable (Up to 20,000 ft maximum), readings were taken on the ground using a data logging sound reader (ExTech, EN300 5-in-1 Environmental Meter) at the time that each lizard was captured. Ground noise was measured in decibels; the decibel scale is logarithmic with value increases representing significant increases in the perceived noise intensity. Sampling dates on which no flyovers occurred were 6/14/2021-6/20/2021. Sampling dates on which flyovers occurred were 6/23/2021- 6/25/2021). On the non-flyover dates, ground decibel readings ranged from 30.1 to 55.8 decibels. During the flyover period, ground decibel readings ranged from 33.9 to 112.2 decibels. Because the experiment took place on a military base where each training area is access-restricted, there were no anthropogenic disturbances at the field location else than the presence of 3 to 4 field workers during the control, and the presence of field workers in addition to aircraft noise during the treatment.
Note that in order to minimize the influence of external environmental factors on our flyover experiment i) all observations were taken from the same location (Figure 2), which limits potential differences in habitat, predation rates, and resource availability; ii) the treatment was applied right after the control, which limits any potential life history differences since the study took place over a two-week period; iii) clutch size (i.e. ranges from 0 to 4) and reproductive stage (i.e. gravid versus not) differences were present across individuals, which is why we controlled for these effects in our models; iv) since the same animal was never resampled either within or across treatments, all observations were independent from one another (i.e. no pseudoreplication). Microclimatic conditions averaged over sampling days were similar between the control (mean = 30.4 °C, sd = 5.3 °C) and the treatment (mean = 30.5°C, sd = 5.1 °C). We were limited in our ability to replicate our experiment, with only one control and one treatment.
Behavior was recorded by observing individuals for three minutes prior to initiating capture (Table 1). The observers were quiet across the sampling period and remained at minimum two meters away from the individual to ensure the behaviors recorded were naturally occurring, and not displayed in response to human presence. We ended the session if the individual was alarmed by the observers’ presence at any time before or during the recording. We recorded audio descriptions of the ongoing behaviors on the “Voice Memos” app of an Apple iPhone 12 to instantly save the completed memo to a local database. We categorized In-situ behaviors into one of nine behaviors: basking, searching, eating, basking while eating, eating while searching, moving, moving while searching, null, and out of sight (reference Table 2 for an explicit description of each behavior). We summarized behavior by playing back video recordings and noting the proportion of time each lizard spent in a specific behavior category. The out of sight behavior was not used in final analyses due to the lizard being out of the observer’s sightline, therefore a behavior was not observed.
Table 1 a. Sample size for behavioral data (Null; Eat; Basking; Basking while eating; Move; Searching; Eating while searching; Moving while searching; Out of sight) collected on A. neotesselatus, with respect to treatment (control versus flyovers).
b. Sample size for data collected on A. neotesselatus, with respect to treatment control versus flyovers and the dependent variables of interest i.e. CORT, Glucose, ROMs, and Ketones.
Table 2 Description of behaviors recorded during the three-minute observation period for A. neotesselatus. Code represents the nomenclature later used in analyses.
We conducted transect surveys during the morning and early afternoon hours (0900-1300) to seek out and capture individuals. After collecting behavioral data, lizards were caught using a snare pole and kept in mesh fabric bags in the shade to later be processed. Coordinates were recorded for the exact location of each individual capture. Toeclip ID has been used at all FC sites and across all study years to track long term data for individual animals. For recaptures, the unique preexisting toeclip ID was recorded. Newly caught individuals were given a unique toeclip ID. Individuals from the 2021 season were marked with a unique number using a non-toxic paint pen; these highly visible, temporary cohort markings allowed us to avoid recapturing individuals and exposing them to repeated capture stress over the course of the study. Weight, tail length, tail diameter, and Snout vent length (SVL) were measured on the day of capture. Ultrasounds were performed to assess gravidity, clutch size, and follicular/egg size of adult females. Follicle is henceforth used as an inclusive term to span the range of reproductive development from the smallest measurable follicle, to the largest clearly defined egg, which ranged from 0.17 to 1.05 cm. Follicle size measurement, i.e. the length along the longest axis, was conducted with a Sonosite Turbo ultrasound unit with an external linear probe (Sonosite Turbo ultrasound, FUJIFILM SonoSite Inc., Bothell, Washington, USA), and each follicle in a clutch was our sampling unit. Once processed, lizards were released in the exact coordinate location of capture.
We collected blood from the retroorbital sinus of each captured lizard using a heparinized microhematocrit capillary tube (Fisherbrand, # 22-362566). Blood samples were collected within the first 3 minutes of being pursued for capture, a validated window of time to measure baseline physiological activity in lizards (Romero and Reed, 2005). If capture attempts exceeded 3 minutes in duration, we did not collect blood samples from that individual lizard to limit the effect of a stress response elicited by the actual pursuit of the animal. After collection, blood samples were immediately stored on ice after centrifugation at 6000 RPM for 10 minutes to isolate plasma. We separated plasma from red blood cells by drawing it from the top of the sample with a Hamilton syringe (SigmaAldrich, #20888). Samples were frozen, transported to the laboratory on dry ice, and stored at −20 °C for assays. Samples were only taken during a standardized timeframe to avoid daily circadian differences in CORT released by stimuli (Dallman et al., 1987; Jones and Gillham, 1988; Romero and Wingfield, 2001). We recorded site temperature at each capture, which averaged 30.48°C with a standard deviation of 4.05, thus quite consistently hot across the sampling period. The sampling period was between 0700-1200 hours and was found to have no relationship between circulating CORT and time of day (Hudson et al., 2020). Body temperature at the time of capture does not impact baseline plasma CORT levels either in field caught lizards (Racic et al., 2020)”. Plasma samples were analyzed to measure baseline levels of physiological activity as described below (Romero and Reed, 2005; Sheriff et al., 2011).
To determine CORT concentrations, enzyme-linked immunosorbent assay (ELISA) kits were validated and used with blood plasma (10 uL), following Hudson et al. (2020). This colorimetric ELISA is based on competitive binding between sheep polyclonal antibodies and plasma hormone that takes place on a donkey anti-sheep immunoglobulin (IgG) microtiter plate. We assayed each sample in duplicate across one 96-well plates according to manufacturer guidelines (Product # ADI-901-097; Enzo Life Sciences, Farmingdale, NY). Due to sample volume constraints, some samples were not run in duplicate.The intra-assay coefficient of variation (CV) was 9.2%.
Blood samples were collected within 3 min of capture, and blood glucose was immediately measured from the whole blood using a handheld glucometer (AccuChek Aviva Plus glucose monitoring system; Roche 182 Diagnostics, Indiana, USA). If there was an initial error in the glucose reading, then we re-tested a blood subsample to obtain a valid measure.
Circulating ROMs were quantified using an assay kit (MC435, Diacron International, Italy), which detects levels of hydroperoxides that oxidize an alkyl-substituted aromatic amine (A-NH2). Plasma was diluted in the assay buffer solution (5 μl: 100 μl). Samples were run in duplicate in a 96-well microplate following the manufacturer’s instructions for “endpoint” mode with modifications for use (French et al., 2017). This reaction resulted in a color change that was measured with a spectrophotometer at 505 nm (xMark; Bio-Rad). Values were calculated as absorbance change relative to the standard. The inter-assay coefficient of variation (CV) was 5.38% and the intra-assay CV was 6.88%.
We tested whole blood concentrations of β-ketones by pipetting blood samples (1 uL) into test strips designed for a STAT-Site dual analyte measurement system (#7000-001, EKF Diagnostics, Boerne, Texas, USA). Results were automatically acquired within 10 seconds of use if concentrations ranged 0.1 to 8.0 mmol/L. If the meter yielded a calculation error, samples were re-pipetted into a new strip and tested for a valid measure unless out of range.
All analyses and visual representation of data were performed using default packages in RStudio (version 1.2.5001, R Core Team, 2019) and additional packages: ‘dplyr’ (Wickham, 2020) and ‘ggplot2’ (Wickham 2016).
We quantified the impact of flyover treatment on three behavioral categorizations: moving vs. not moving (i.e. “moving”, “moving while searching” vs. all other behaviors), eating vs. not eating (i.e. “eating”, “eating and searching”, “eating and basking” vs. all other behaviors), and active vs. inactive (i.e. “searching”, “eating while searching”, “moving”, “moving while searching” vs. all other behaviors). We then calculated the proportion of time each individual spent in any given behavior and averaged these proportions across individuals to test for significant differences in the proportion of time spent moving vs. not moving. We used a two-way ANOVA model to test for these differences using the ‘aov’ function in program R (Chambers et al., 1992).
We first tested for preliminary effects of flyovers treatments on each measure of interest (i.e. CORT, glucose, ROMs, and ketones) using t-tests. We then used generalized linear models with a gaussian distribution and an identity link to model the relationship between flyover treatments (control vs. flyover) on dependent variables of interest (Bolker et al., 2009) while controlling for the effect of size ‘svl’, body mass, reproductive state (gravid versus not), and clutch size (which varied from 0 to 4 follicles), which are known predictors of physiology in this species (Aubry et al., 2019; Aubry et al., 2020; Hudson et al., 2020; Caracalas et al., 2021). A set of a priori defined models competed within a model selection framework (Anderson and Burnham, 2002) where models were compared based on their respective AIC values (Akaike, 1973). The top-performing model within each set is further discussed in the result section.
For all models, we tested for normality of model residuals using Shapiro’s test (Royston, 1982) and for homogeneity of variance using Levene’s test (i.e., homoscedasticity, Hines and Hines, 2000). Note that ROMs were log-transformed, and ketone concentrations were square-rooted, to un-skew their distribution and normalize residuals. Model assumptions of normality of residuals and homoscedasticity were tested using appropriate statistics and are reported in the result section below. We adopted a significance level alpha of 0.05 for statistical significance in all analyses.
Although Figure 3 indicated that animals, on average, spent more time moving when there were no flyovers, ANOVA test results (Table 3) indicated that there was no significant difference between the average time spent moving vs. remaining stationary (Table 3). The flyover treatment did not have a significant effect on the time spent in either group of behaviors (Table 3). Further, the interaction between behavior and treatment did not significantly impact the average proportion of time spent in either moving or stationary behaviors (Table 3).
Figure 3 Average proportion of time (%) spent by A. neotesselatus moving (‘M’ moving, ‘MS’ moving while searching) vs. all other behaviors (‘B’ basking, ‘BE’ basking while eating, ‘E’ eating, ‘ES’ eating while searching, ‘N’ null, ‘S’ searching/scanning/foraging) between the treatment (flyovers) and the control (no flyovers).
Table 3 ANOVA test results investigating the impact of a) movement, flyover treatment, and their interaction; b) eating, flyover treatment, and their interaction; and c) active, flyover treatment, and their interaction, on the proportion of time spent in the behavior.
Figure 4 indicated that animals spent more time basking (B), moving (M) or searching (S) for food than actively eating (E) when undisturbed. However, the pattern was reversed when flyovers occurred. While animals spent less time basking, moving or searching when flyovers occurred, focusing more on eating and eating while searching instead (Figure 4). There was a significant difference between the average time spent eating vs. all other behaviors (Table 3). Yet, the interaction between behavior and treatment did not significantly impact the average proportion of time spent eating vs. all other behaviors (Table 3).
Figure 4 Average proportion of time (%) spent by A. neotesselatus eating (‘BE’ basking while eating, ‘E’ eating, ‘ES’ eating while searching) vs. all other behaviors (‘B’ basking, ‘M’ moving, ‘MS’ moving while searching, ‘N’ null, ‘S’ searching/scanning/foraging) between the treatment (flyovers) and the control (no flyovers).
Animals disturbed by flyovers spent less time moving or searching (Figure 5). They spent significantly less time in assessing vs. passive behaviors such as basking and eating (Table 3). The flyover treatment had a marginally significant effect on the time spent in either group of behaviors, with animals spending significantly less time assessing surroundings when flyovers occurred (Table 3). Yet, the interaction between behavior and treatment did not significantly impact the average proportion of time spent assessing vs. all other behaviors (Table 3).
Figure 5 Average proportion of time (%) spent assessing the environment (i.e. Stationary: ‘ES’ eating while searching, ‘M’ moving, ‘MS’ moving while searching, ‘S’ searching/scanning/foraging) vs. any other behavior (‘B’ basking, ‘BE’ basking while eating, ‘E’ eating, ‘N’ null) by A. neotesselatus in key behaviors between the treatment (flyovers) and the control (no flyovers).
There are no significant relationship between noise disturbance and circulating CORT (t-value = -1.3476, df = 36.238, p-value = 0.1861). Mean concentrations of plasma CORT averaged 0.3452 ng/mL in the control, and 0.4479 ng/mL within the flyover treatment (Figure 6). Model selection results (Table 4) indicated that the best fitting model supported an effect of the flyover treatment on plasma CORT while correcting for clutch size. The effect of flyover treatment had significant and positive effect on CORT (βtreatment = 0.3644, std. error = 0.1655, p-value = 0.0312), and so did clutch size, whereby an increase in clutch size was associated with increased CORT as well (βclutchsize = 0.1060, std. error = 0.0418, p-value = 0.0136), whilst the interaction between clutch size and treatment did no significantly affect CORT (βclutchsize*treatment = -0.0897, std. error = 0.0597, p-value = 0.1375).
Figure 6 Impact of flyover treatments (Noise ‘N’ = control; Noise ‘Y’ = flyover) on circulating plasma corticosterone (ng/mL).
Table 4 Model selection results: comparison of generalized linear models testing for the effect of flyover treatments (control vs. flyover) on circulating Cort (in ng/mL), Glucose (mg/dL), ROMs (mg/H202/dL), and Ketones, while controlling for the effect of size (svl in mm), mass (in gr), reproductive state (gravid versus not), and clutch size (0-4 follicles). K: the number of parameters.
There was no significant relationship between plasma glucose and noise disturbance during flyovers (Welch Two Sample t-test: t-value = -0.2664, df = 25.259, p-value = 0.7921) where mean concentrations of plasma glucose averaged 325.30770 mg/dL for the control and 328.1579 mg/dL for the flyover treatment (Figure 7). Further, model selection results (Table 4) indicated that the best fitting model did not support a relationship between flyovers and plasma glucose while correcting for size, mass, or reproductive investment (βtreatment = 2.850, std. error = 9.228, p-value = 0.758).
Figure 7 Impact of flyover treatments (Noise ‘N’ = control; Noise ‘Y’ = flyover) on plasma glucose (mg/dL).
Noise disturbance marginally impacted circulating ROMs (Welch Two Sample t-test: t-value = 1.8963, df = 45.975, p-value = 0.06422) where mean concentrations of plasma ROMs averaged 16.9616 mg/H2O2/dL within the control, and 11.6889 mg/H2O2/dL within the flyover treatment (Figure 8). Model selection results (Table 4) indicated that the best fitting model supported an effect of the flyover treatment on plasma ROMs while correcting for reproductive stage. The effects of flyover treatment had a significant effect on ROMs (βtreatment = 2.8305, std. error = 0.4328, p-value < 0.001), while gravidity had a negative effect on ROMs when compared to non-gravid females (βrep.stage = -1.0622, std. error = 0.4007, p-value = 0.0098), but the interaction between reproductive stage and treatment was not significant in affecting ROMs (βrep.stagel*treatment = -0.4648, std. error = 0.6692, p-value = 0.4895).
Figure 8 Impact of flyover treatments (Noise ‘N’ = control; Noise ‘Y’ = flyover) on plasma ROMs (mg/H202/dL).
Noise disturbance did correspond significantly with plasma ketones (Welch Two Sample t-test: t-value = -2.9504, df = 29.367, p-value = 0.0062), where mean concentrations of ketones averaged 4.6895 within the control, and 10.13043 within the flyover treatment (Figure 9). Model selection results (Table 4) indicated that the best fitting model supported a positive effect of the flyover treatment on ketones (βtreatment = 6.9416, std. error = 2.5692, p-value = 0.0085) while correcting for size mattered as well, with smaller animals experiencing higher ketone concentrations (βslv = -0.0645, std. error = 0.0256, p-value = 0.0138); yet, the interaction between size and treatment was not significant (βsvl*treatment = 0.0142, std. error = 0.0411, p-value = 0.7313).
Our results suggest that A. neotesselatus spent less time assessing their environment and more time eating when disturbed by flyovers. This was determined when lizards were observed feeding in a stationary position or traveling while carrying prey items. Specifically, our results suggest that animals, on average, spent significantly more time in passive behaviors such as basking (B), stationary eating (E), and eating while searching (ES) when disturbed. Aircraft noise increased CORT when clutch size was controlled for; but did not impact plasma glucose during the flyover treatment. Contrary to our predictions, noise disturbance marginally decreased circulating ROMs and was negatively correlated with CORT (t=-3.007, df=67, p-value=0.0037, Pearson’s product-moment correlation cor=-0.344). Reproductive stage was important in explaining these differences as well, with gravid females experiencing lower plasma ROMs than non-gravid females; there were no significant interactions between noise and reproductive stage in affecting ROMs. Noise disturbance did significantly increase ketone production, and smaller animals experienced higher ketone concentrations, but the latter was independent of noise exposure despite model selection indicating that the interaction between size and noise was important in explaining variability in ketones.
Behavioral activity shifted in response to noise pollution, which has been observed in a variety of taxa, including reptiles (for review, see for e.g., Warren et al., 2006; Slabbekoorn and Ripmeester, 2008; Popper and Hastings, 2009; Barber et al., 2010; Slabbekoorn et al., 2010). The shift towards more elusive behavior and a lack of movement corroborates other studies which observed “freezing” behavior when exposed to noise disturbance (e.g., Warwick et al., 2013; Mancera et al., 2017). However, we did find animals did spend significantly more time eating while flyovers took place, suggesting an adjustment in their behavior that could potentially mitigate the negative impact of flyovers on their metabolism.
Both flyover disturbance and clutch size influenced plasma CORT, though their effects were independent of one another. Positive correlations between reproductive mass and glucocorticoid levels have been reported in common side-blotched lizards (Wilson and Wingfield, 1992), yet some studies of reptiles do not show elevated CORT in the presence of chronic anthropogenic stress (Injaian et al., 2020; Malisch et al., 2020). Given that most of the studied anthropogenic sources of stress are fixed (e.g., roadways, light and noise pollution from cities), our results may be indicative of an acute stress response whereby the transient and sporadic nature of flyovers may act as an acute rather than chronic stressor.
We hypothesized that glucose mobilization by CORT may reduce plasma glucose levels in the presence of acute flyover stress, leading to the production or utilization of the alternate energy sources (Romero and Beattie, 2022). Specifically, we expected that lower plasma glucose during flyover noise disturbance would reflect a rapid uptake of energy to cope with an acute stressor. However, we found plasma glucose was unaffected by the flyover treatment, which refutes the idea of energetic reallocation because of sensory disturbance, at least in the context of glucose levels. Most birds, amphibians, and reptiles secrete CORT to maximize short-term fitness through the modulation of lipid metabolism, but there is considerable variation in these responses (Gangloff et al., 2017; Neuman-Lee et al., 2020; Kelley et al., 2021). Butler and Barrientos (2020) compared free-living house sparrow (Passer domesticus) nestlings exposed to a one-hour stressor to control individuals, and quantified circulating CORT, triglyceride, and glycerol levels. Nestlings exposed to a stressor had reduced circulating triglycerides consistent with an increased rate of glucogenesis during an acute stressor. Although the timeframe of our sampling may not have detected stress-induced glucose changes (e.g. transient or slow responding), our results do not support links between CORT and aspects of glucogenesis in response to an acute stressor, a finding supported by other reptile studies (Neuman-Lee et al., 2020). The molecular pathways connecting these metrics still need to be elucidated via controlled experiments in the lab.
Our results suggest behavioral variation in A. neotesselatus during flyover and non-flyover conditions. A. neotesselatus spent a longer duration of time eating during the flyovers. This behavioral change may serve to buffer the potentially negative effect of flyovers on energetic pathways. This result is quite novel in that most studies report on the negative impacts of noise pollution on body mass and condition via shifts in glucogenesis driven by decreased feeding time when exposed to noise (reviewed in Kight and Swaddle, 2011). It has been hypothesized that noise-driven declines in foraging activity, and potential weight loss, may lead to increased metabolic processing and mobilization of cholesterol to fuel the production of stress hormones, because when stressed, individuals rapidly use stored resources to regulate neuroendocrine responses to stress (Kight and Swaddle, 2011). Although we did detect a stress response (increased CORT) during flyovers, we did not detect the expected decline in plasma glucose levels, most likely because of the behavioral adjustment A. neotesselatus made in eating more and moving less, which may have fueled plasma glucose levels. This result would need to be confirmed by additional controlled noise pollution experiments in the field.
Reproductive investment did play a role in modulating some of these responses, as suggested by the interaction between clutch size and noise in elevating CORT, which was retained by the model selection process. In rock iguanas, free glycerol levels are highest during the beginning of the breeding season and decrease with gravidity (Webb et al., 2019). Likely due to fat catabolism for vitellogenesis at the beginning of the season and uptake during gravidity. In the context of our study, sampling occurred one month into the breeding season and reproductive stages ranged from mid to post reproduction as determined by ultrasound. Although our findings are consistent with the seasonal timeframe in which glycerol trends were observed by Webb et al. (2019), we did not observe a relationship between reproductive stage or investment, glucogenesis and noise exposure. The behavioral adjustment which we qualify here as “compensatory feeding” in A. neotesselatus is a plausible explanation for the lack of impact of noise exposure on glucose metabolism. We did, however, observe an increase in CORT with reproductive investment, which we had observed before in the same population (Aubry et al., 2020; Caracalas et al., 2021).
ROMs values were marginally impacted by noise, but not in the expected direction, in that they were higher under control conditions and reduced during the flyover treatment. The ROMs test measures blood levels of hydroperoxides, which are produced when biomolecules (fatty acids, nucleic acids, proteins, etc.) are oxidized (Costantini, 2016). ROMs may exceed the capacity of antioxidant defense and repair mechanisms (i.e., oxidative stress), leading to oxidative damage of biomolecules (Metcalfe and Alonso-Alvarez, 2010). Lower ROMs during flyovers may be indicative of a lack of oxidative damage. During flyover events, lizards were observed eating and eating while searching their environment for a significantly higher proportion of time than was spent in other behaviors (Table 3; Figure 4), and were most likely not in a digestive state which sometimes carried an oxidative cost. Results suggest that compensatory eating in A. neotesselatus may keep metabolic processing at bay, which would explain the lower ROMs values observed during flyovers.
Although A. neotesselatus seem to adjust their behavior during flyovers by increasing the time they spend eating to buffer the potentially negative effect of flyovers on energetic pathways, they still suffered a metabolic cost driven by the stress response via ketone accumulation, especially when considering smaller-sized animals. Indeed, plasma ketones were significantly higher during flyover noise disturbance when we accounted for body size. An increase in ketones in response to acute and prolonged stress has been documented before (Ricart-Jané et al., 2002; Neuman-Lee et al., 2015). When blood glucose levels are significantly altered due to stress or physical activity, fatty acid metabolism may be initiated (Rojas-Morales et al., 2020). Ketones are products of fat metabolism that can be utilized as alternative sources of energy when glucose is low, but plasma glucose was unaffected by the flyover treatment in our study, suggesting individuals may preferentially mobilize ketones, even when glucose may be maintained via observed “compensatory eating” behavior we observed in the field.
Colorado checkered whiptails at Fort Carson responded to acute anthropogenic stress in a variety of ways that included: a compensatory behavioral response (less time spent moving, but more time spent eating), a stress response (increased CORT) and partial energy mobilization (increase in ketones). In an oviparous lizard actively allocating yolk, we found that results must be analyzed in the context of reproductive timing and maternal investment by controlling for reproductive status and clutch size, as conducted here. Anthropogenic disturbance may have a higher impact on parthenogenetic lizards during the breeding season when maternal sources of energy must be invested into developing offspring. However, A. neotesselatus seem to adjust their behavior to minimize the negative impacts of noise disturbance on some energetic pathways and may maintain energy levels through compensatory eating during flyovers. In summary, although anthropogenic disturbance in the form of military aircraft flyover events have significant physiological impacts on this Colorado checkered whiptail population, behavioral adjustments and compensatory feeding appear to limit energetic expenditures to some extent in relation to noise pollution. Although behavioral plasticity may help mitigate some of the potentially negative impacts of noise exposure on A. neotesselatus metabolism, the impacts may also be mitigated if aircraft operators maintain an elevation that is sufficiently high enough to lead to decibel reads on the ground that fall below 50 dB.
The raw data supporting the conclusions of this article and the R code are available upon request, granted authorization from the Division of Public Works at Fort Carson, CO; please contact senior author LMA for access.
The animal study was reviewed and approved by Colorado State University IACUC 18–7772A.
LMA & SSF conceived the study; MEK, LOS, & SBH collected the data; MEK, LOS, & SHS conducted the lab work; LOS analyzed the behavior audio recordings; LMA analyzed the data; AJJL facilitated field activities; LMA wrote the first draft of the manuscript; MEK, LJJS, SBH, AOL & SSF participated in the writing of subsequent versions of the manuscript. All authors contributed to the article and approved the submitted version.
This research predominantly funded by Fort Carson by way of a US Fish and Wildlife Cooperative Agreement F17AC00326. This research was also supported in part by the US Department of Agriculture, Animal and Plant Health Inspection Service, Wildlife Services, National Wildlife Research Center.
We would like to thank Roger Payton and FC’s Directorate of Public Works, Conservation Branch, for logistical and administrative assistance in facilitating our research. We are extremely grateful to the military personal at range tower for helping us coordinate flyovers at Fort Carson, and for making room for data collection and biological conservation on their active military base.
The authors declare that the research was conducted in the absence of any commercial or financial relationships that could be construed as a potential conflict of interest.
The findings and conclusions in this publication have not been formally disseminated by the US Department of Agriculture and should not be construed to represent any agency determination or policy.
All claims expressed in this article are solely those of the authors and do not necessarily represent those of their affiliated organizations, or those of the publisher, the editors and the reviewers. Any product that may be evaluated in this article, or claim that may be made by its manufacturer, is not guaranteed or endorsed by the publisher.
Amburgey S. M., Miller D. A. W., Rochester C. J., Delaney K. S., Riley S. P. D., Brehme C. S., et al. (2021). The influence of species life history and distribution characteristics on species responses to habitat fragmentation in an urban landscape. J. Anim. Ecol. 90 (3), 685–697. doi: 10.1111/1365-2656.13403
Angelier F., Meillère A., Grace J. K., Trouvé C., Brischoux F. (2016). No evidence for an effect of traffic noise on the development of the corticosterone stress response in an urban exploiter. Gen. Comp. Endocrinol. 232, 43–50. doi: 10.1016/j.ygcen.2015.12.007
Akaike H. (1973). Maximum likelihood identification of gaussian autoregressive moving average models. Biometrika 60 (2), 255–265.
Anderson D. R., Burnham K. P. (2002). Avoiding pitfalls when using information-theoretic methods. J. Wildl. Manag. 912–918.
Aubry L. M., Eifler D., Utsumi K., French S. S. (2019). Demographic assessment of the triploid parthenogenetic lizard Aspidoscelis neotesselatus at the northern edge of its range. Herpetol. Conserv. Biol. 14 (2), 411.
Aubry L. M., Hudson S. B., Kluever B. M., Webb A. C., French S. S. (2020). Competing reproductive and physiological investments in an all-female lizard, the Colorado checkered whiptail. Evolutionary Ecol. 34 (6), 999–1016. doi: 10.1007/s10682-020-10081-x
Barber J. R., Crooks K. R., Fristrup K. M. (2010). The costs of chronic noise exposure for terrestrial organisms. Trends Ecol. Evol. 25, 180–189. doi: 10.1016/j.tree.2009.08.002
Bennie J., Davies T. W., Cruse D., Inger R., Gaston K. J. (2015). Cascading effects of artificial light at night: resource-mediated control of herbivores in a grassland ecosystem. Philos. Trans. R. Soc. London Biol. Sci. 3701667, 20140131. doi: 10.1098/rstb.2014.0131
Bevan E., Whiting S., Tucker T., Guinea M., Raith A., Douglas R. (2018). Measuring behavioral responses of sea turtles, saltwater crocodiles, and crested terns to drone disturbance to define ethical operating thresholds. PloS One 13 (3), e0194460. doi: 10.1371/journal.pone.0194460
Blickley J. L., Word K. R., Krakauer A. H., Phillips J. L., Sells S. N., Taff C. C., et al. (2012). Experimental chronic noise is related to elevated fecal corticosteroid metabolites in lekking male greater sage-grouse (Centrocercus urophasianus). PloS One 7 (11), 50462. doi: 10.1371/journal.pone.0050462
Bolker B. M., Brooks M. E., Clark C. J. (2009). Generalized linear mixed models: a practical guide for ecology and evolution. Trends Ecol. Evol. 24 (3), 127–135. doi: 10.1016/j.tree.2008.10.008
Butler M. J., Barrientos R. M. (2020). The impact of nutrition on COVID-19 susceptibility and long-term consequences. Brain Behav. Immun. 87, 53–54.
Buxton R. T., McKenna M. F., Mennitt D., Fristrup K., Crooks K., Angeloni L., et al. (2017). Noise pollution is pervasive in US protected areas. Science 356 (6337), 531–533.
Cabrera-Cruz S. A., Smolinsky J. A., Buler J. J. (2018). Light pollution is greatest 1 within migration passage areas for nocturnally-migrating birds around the world. Sci. Rep. 8, 1–8.
Caracalas H. E., French S. S., Hudson S. B., Kluever B. M., Webb A. C., Eifler D., et al. (2021). Reproductive trade-offs in the colorado checkered whiptail lizard (Aspidoscelis neotesselatus): an examination of the relationship between clutch and follicle size. Evolutionary Ecol. 35 (5), 779–794. doi: 10.1007/s10682-021-10131-y
Chambers J. M., Freeny A., Heiberger R. M. (1992). “Analysis of variance; designed experiments. chapter 5 of statistical models,” in S. Eds. Chambers J. M., Hastie T. J. (Milton Park, Abingdon, Oxfordshire, United Kingdon: Wadsworth & Brooks/Cole).
Costantini D. (2016). Oxidative stress ecology and the d-ROMs test: facts, misfacts and an appraisal of a decade’s work. Behav. Ecol. Sociobiol. 70, 809–820. doi: 10.1007/s00265-016-2091-5
Creel S., Fox J. E., Hardy A., Sands J., Garrott B. O. B., Peterson R. O. (2002). Snowmobile activity and glucocorticoid stress responses in wolves and elk. Conserv. Biol. 16 (3), 809–814. doi: 10.1046/j.1523-1739.2002.00554.x
Crino O. L., Johnson E. E., Blickley J. L., Patricelli G. L., Breuner C. W. (2013). Effects of experimentally elevated traffic noise on nestling white-crowned sparrow stress physiology, immune function and life history. J. Exp. Biol. 216 (11), 2055–2062. doi: 10.1242/jeb.081109
Dallman M. F., Akana S. F., Jacobson L., Levin N., Cascio C. S., Shinsako J. (1987). Characterization of corticosterone feedback regulation of ACTH secretion. Ann. New York Acad. Sci. 512, 402–414.
Dominoni D. M., Halfwerk W., Baird E., Buxton R. T., Fernández-Juricic E., Fristrup K. M., et al. (2020). Why conservation biology can benefit from sensory ecology. Nat. Ecol. Evol. 4 (4), 502–511.
Dunham A. E., Overall K. L. (1994). Population responses to environmental change: life history variation, individual-based models, and the population dynamics of short-lived organisms. Am. Zool. 34 (3), 382–396. doi: 10.1093/icb/34.3.382
Francis C. D., Barber J. R. (2013). A framework for understanding noise impacts on wildlife: an urgent conservation priority. Front. Ecol. Environ. 11, 305–313. doi: 10.1890/120183
French S. S., Neuman-Lee L. A., Terletzky P. A., Kiriazis N. M., Taylor E. N., DeNardo D. F. (2017). Too much of a good thing? human disturbance linked to ecotourism has a “dose-dependent“ impact on innate immunity and oxidative stress in marine iguanas, amblyrhynchus cristatus. Biol. Conserv. 210, 37–47.
Gangloff E. J., Sparkman A. M., Holden K. G., Corwin C. J., Topf M., Bronikowski A. M. (2017). Geographic variation and within-individual correlations of physiological stress markers in a widespread reptile, the common garter snake (Thamnophis sirtalis). Comp. Biochem. Physiol. Part A: Mol. Integr. Physiol. 205, 68–76. doi: 10.1016/j.cbpa.2016.12.019
Gibbons J. W., Scott D. E., Ryan T. J., Buhlmann K. A., Tuberville T. D., Metts B. S., et al. (2000). The global decline of reptiles, déjà vu amphibians: Reptile species are declining on a global scale. six significant threats to reptile populations are habitat loss and degradation, introduced invasive species, environmental pollution, disease, unsustainable use, and global climate change. BioScience 50 (8), 653–666. doi: 10.1641/0006-3568(2000)050[0653:TGDORD]2.0.CO;2
Hayward L. S., Bowles A. E., Ha J. C., Wasser S. K. (2011). Impacts of acute and long-term vehicle exposure on physiology and reproductive success of the northern spotted owl. Ecosphere 2 (6), 1–20. doi: 10.1890/ES10-00199.1
Hines W. G. S., Hines R. O. H. (2000). Increased power with modified forms of the levene (Med) test for heterogeneity of variance. Biometrics 56 (2), 451–454. doi: 10.1111/j.0006-341X.2000.00451.x
Hudson S. B., Kluever B. M., Webb A. C., French S. S. (2020). Steroid hormones, energetic state, and immunocompetence vary across reproductive contexts in a parthenogenetic lizard. Gen. Comp. Endocrinol. 288, 113372. doi: 10.1016/j.ygcen.2019.113372
Injaian A. S., Francis C. D., Ouyang J. Q., Dominoni D. M., Donald J. W., Fuxjager M. J., et al. (2020). Baseline and stress-induced corticosterone levels across birds and reptiles do not reflect urbanization levels. Conserv. Physiol. 8 (1), coz110.
Jakob-Hoff R., Kingan M., Fenemore C., Schmid G., Cockrem J. F., Crackle A., et al. (2019). Potential impact of construction noise on selected zoo animals. Animals 9 (8), 504. doi: 10.3390/ani9080504
Jones M. T., Gillham B. (1988). Factors involved in the regulation of adrenocorticotropic hormone/beta-lipotropic hormone. Physiol. Rev. 68 (3), 743–818.
Kaiser K., Devito J., Jones C. G., Marentes A., Perez R., Umeh L., et al. (2015). Effects of anthropogenic noise on endocrine and reproductive function in white’s treefrog, Litoria caerulea. Conserv. Physiol. 3 (1), cou061. doi: 10.1093/conphys/cou061
Kelley S., Farrell T. M., Lind C. M. (2021). Validating the use of a quick-read glucometer to assess the glycemic response to short-term capture stress in two species of snake, nerodia sipedon and sistrurus miliarius. Ichthyol. Herpetol. 109 (2), 436–442. doi: 10.1643/h2020102
Kernbach M. E., Miller C., Alaasam V., Ferguson S., Francis C. D. (2021). Introduction to the symposium: Effects of light pollution across diverse natural systems. Integr. Comp. Biol. 61 (3), 1089–1097. doi: 10.1093/icb/icab157
Kight C. R., Swaddle J. P. (2011). How and why environmental noise impacts animals: an integrative, mechanistic review. Ecol. Lett. 14 (10), 1052–1061. doi: 10.1111/j.1461-0248.2011.01664.x
Kyba C. C., Kuester T., Sánchez de Miguel A., Baugh K., Jechow A., Hölker F., et al. (2017). Artificially lit surface of Earth at night increasing in radiance and extent. Sci. Adv. 3 (11), e1701528.
Malisch J. L., Harris B. N., Sherrer S. M., Lewis K. A., Shepherd S. L., McCarthy P. C., et al. (2020). In the wake of COVID-19, academia needs new solutions to ensure gender equity. Proc. Natl. Acad. Sci. 117 (27), 15378–15381.
Mancera K. F., Murray P. J., Lisle A., Dupont C., Faucheux F., Phillips C. J. (2017). The effects of acute exposure to mining machinery noise on the behaviour of eastern blue-tongued lizards (Tiliqua scincoides). Anim. Welfare 26 (1), 11–24. doi: 10.7120/09627286.26.1.011
Metcalfe N. B., Alonso-Alvarez C. (2010). Oxidative stress as a life-history constraint: the role of reactive oxygen species in shaping phenotypes from conception to death. Funct. Ecol. 24 (5), 984–996. doi: 10.1111/j.1365-2435.2010.01750.x
Mills S. C., Beldade R., Henry L., Laverty D., Nedelec S. L., Simpson S. D., et al. (2020). Hormonal and behavioural effects of motorboat noise on wild coral reef fish. Environ. pollut. 262, 114250. doi: 10.1016/j.envpol.2020.114250
Neuman-Lee L. A., Fokidis B. H., Spence A. R., van der Walt M., Smith G. D., Durham S., et al. (2015). Food restriction and chronic stress alter energy use and affect immunity in an infrequent feeder. Funct. Ecol. 29, 1453–1462. doi: 10.1111/1365-2435.12457
Neuman-Lee L. A., Hudson S. B., Webb A. C., French S. S. (2020). Investigating the relationship between corticosterone and glucose in a reptile. J. Exp. Biol. 223 (2), jeb203885. doi: 10.1242/jeb.203885
Nichols T. A., Anderson T. W., Širović A. (2015). Intermittent noise induces physiological stress in a coastal marine fish. PloS One 10 (9), e0139157. doi: 10.1371/journal.pone.0139157
Nykaza E. T., Pater L. L., Melton R. H., Luz G. A. (2009). Minimizing sleep disturbance from blast noise producing training activities for residents living near a military installation. J. Acoust. Soc. Am. 125 (1), 175–184.
Ouyang J. Q., Davies S., Dominoni D. (2018). Hormonally mediated effects of 1 artificial light at night on behavior and fitness: linking endocrine mechanisms with function. J. Experiemental Biol. 221, jeb156893. doi: 10.1242/jeb.156893
Pater L. L., Grubb T. G., Delaney D. K. (2009). Recommendations for improved assessment of noise impacts on wildlife. J. Wildl. Manag. 73 (5), 788–795.
Patricelli G. L., Blickley J. L. (2006). Avian communication in urban noise: causes and consequences of vocal adjustment. Auk 123, 639–649. doi: 10.1093/auk/123.3.639
Popper A. N., Hastings M. C. (2009). The effects of human-generated sound on fish. Integr. Zool. 4, 43–52. doi: 10.1111/j.1749-4877.2008.00134.x
Racic A., Tylan C., Langkilde T. (2020). Effects of temperature on plasma corticosterone in a native lizard. Sci. Rep. 10, 16315. doi: 10.1038/s41598-020-73354-z
R Core Team (2019). R: a language and environment for statistical computing, version 3.0. 2 Vol. 2013 (Vienna, Austria: R Foundation for Statistical Computing).
Ricart-Jané D., Rodríguez-Sureda V., Benavides A., Peinado-Onsurbe J., López-Tejero M. D., Llobera M. (2002). Immobilization stress alters intermediate metabolism and circulating lipoproteins in the rat. Metabolism 51 (7), 925–931. doi: 10.1053/meta.2002.33353
Rojas-Morales P., Pedraza-Chaverri J., Tapia E. (2020). Ketone bodies, stress response, and redox homeostasis. Redox Biol. 29, 101395. doi: 10.1016/j.redox.2019.101395
Romero L. M., Beattie U. K. (2022). Common myths of glucocorticoid function in ecology and conservation. J. Exp. Zoology Part A: Ecol. Integr. Physiol. 337 (1), 7–14.
Romero L. M., Reed J. M. (2005). Collecting baseline corticosterone samples in the field: is under 3 min good enough? Comp. Biochem. Physiol. Part A: Mol. Integr. Physiol. 140 (1), 73–79.
Romero L. M., Wingfield J. C. (2001). Regulation of the hypothalamic-pituitary-adrenal axis in free-living pigeons. J. Comp. Physiol. B 171, 231–235.
Royston J. P. (1982). An extension of Shapiro and wilk’s W test for normality to large samples. J. R. Stat. Society Ser. C 31 (2), 115–124.
Sala O. E., Stuart Chapin F. I. I. I., Armesto J. J., Berlow E., Bloomfield J., Dirzo R., et al. (2000). Global biodiversity scenarios for the year 2100. Science 287 (5459), 1770–1774.
Santulli A., Modica A., Messina C., Ceffa L., Curatolo A., Rivas G., et al. (1999). Biochemical responses of European Sea bass (Dicentrarchus labrax l.) to the stress induced by off shore experimental seismic prospecting. Mar. pollut. Bull. 38–12, 1105–1114.
Shannon G., Crooks K. R., Wittemyer G., Fristrup K. M., Angeloni L. M. (2016). Road noise causes earlier predator detection and flight response in a free-ranging mammal. Behav. Ecol. 27 (5), 1370–1375.
Sheriff M. J., Dantzer B., Delehanty B., Palme R., Boonstra R. (2011). Measuring stress in wildlife: techniques for quantifying glucocorticoids. Oecologia 166 (4), 869–887.
Slabbekoorn H., Bouton N., van Opzeeland I., Coers A., ten Cate C., Popper A. N. (2010). A noisy spring: the impact of globally rising underwater sound levels on fish. Trends Ecol. Evol. 25, 419–427. doi: 10.1016/j.tree.2010.04.005
Slabbekoorn H., Ripmeester E. A. (2008). Birdsong and anthropogenic noise: implications and applications for conservation. Mol. Ecol. 17, 72–83. doi: 10.1111/j.1365-294X.2007.03487.x
Tan W. C., Herrel A., Rödder D. (2023). A global analysis of habitat fragmentation research in reptiles and amphibians: what have we done so far? Biodiver. Conserv. 1–30 doi: 10.1007/s10531-022-02530-6
Tennessen J. B., Parks S. E., Langkilde T. (2014). Traffic noise causes physiological stress and impairs breeding migration behaviour in frogs. Conserv. Physiol. 2 (1). doi: 10.1093/conphys/cou032
Todd B. D., Andrews K. M. (2008). Response of a reptile guild to forest harvesting. Conserv. Biol. 22 (3), 753–761. doi: 10.1111/j.1523-1739.2008.00916.x
Todd B. D., Willson J. D., Gibbons J. W. (2010). The global status of reptiles and causes of their decline. Ecotoxicol. amphibians reptiles 47, 67. doi: 10.1201/EBK1420064162-c3
Touzot M., Teulier L., Lengagne T., Secondi J., Théry M., Libourel P.-A., et al. (2019). Artificial light at night disturbs the activity and energy 15 allocation of the common toad during the breeding period. Conserv. Physiol. 7. doi: 10.1093/conphys/coz002
Wale M. A., Simpson S. D., Radford A. N. (2013). Noise negatively affects foraging and antipredator behaviour in shore crabs. Anim. Behav. 86, 111–118. doi: 10.1016/j.anbehav.2013.05.001
Warren P. S., Katti M., Ermann M., Brazel A. (2006). Urban bioacoustics: it’s not just noise. Anim. Behav. 71, 491–502. doi: 10.1016/j.anbehav.2005.07.014
Warwick C., Arena P., Lindley S., Jessop M., Steedman C. (2013). Assessing reptile welfare using behavioural criteria. In Pract. 35 (3), 123–131. doi: 10.1136/inp.f1197
Webb A. C., Iverson J. B., Knapp C. R., DeNardo D. F., French S. S. (2019). Energetic investment associated with vitellogenesis induces an oxidative cost of reproduction. J. Anim. Ecol. 88, 461–472. doi: 10.1111/1365-2656.12936
Wilson B. S., Wingfield J. C. (1992). Correlation between female reproductive condition and plasma corticosterone in the lizard uta stansburiana. Copeia, 691–697.
Keywords: conservation, hormones, flyovers, metabolism, noise pollution, physiology, reproduction, whiptail
Citation: Kepas ME, Sermersheim LO, Hudson SB, Lehmicke AJJ, French SS and Aubry LM (2023) Behavior, stress and metabolism of a parthenogenic lizard in response to flyover noise. Front. Amphib. Reptil. Sci. 1:1129253. doi: 10.3389/famrs.2023.1129253
Received: 21 December 2022; Accepted: 08 February 2023;
Published: 29 March 2023.
Edited by:
Anssi Laurila, Uppsala University, SwedenReviewed by:
Pablo Burraco, University of Glasgow, United KingdomCopyright © 2023 Kepas, Sermersheim, Hudson, Lehmicke, French and Aubry. This is an open-access article distributed under the terms of the Creative Commons Attribution License (CC BY). The use, distribution or reproduction in other forums is permitted, provided the original author(s) and the copyright owner(s) are credited and that the original publication in this journal is cited, in accordance with accepted academic practice. No use, distribution or reproduction is permitted which does not comply with these terms.
*Correspondence: Lise M. Aubry, bGlzZS5hdWJyeUBjb2xvc3RhdGUuZWR1
†These authors have contributed equally to this work
Disclaimer: All claims expressed in this article are solely those of the authors and do not necessarily represent those of their affiliated organizations, or those of the publisher, the editors and the reviewers. Any product that may be evaluated in this article or claim that may be made by its manufacturer is not guaranteed or endorsed by the publisher.
Research integrity at Frontiers
Learn more about the work of our research integrity team to safeguard the quality of each article we publish.