- 1Forage Seed and Cereal Research Unit, Agricultural Research Service (USDA), Corvallis, OR, United States
- 2Department of Natural Resources and Environment, University of New Hampshire, Durham, NH, United States
- 3Center of Soil Biogeochemistry and Microbial Ecology (Soil BioME), University of New Hampshire, Durham, NH, United States
Introduction: Understanding how nutrient inputs affect nitrogen (N) transformations and storage is vital for developing sustainable agroecosystems. Organic N inputs, such as crop residues (e.g., cover crop biomass and stover) and animal manures, can accelerate N cycling by increasing mineralization and immobilization rates to provide crops with more opportunities to intercept N as it moves through bioavailable pools. We aimed to understand how organic and synthetic soil amendments inhibit or promote N mineralization, immobilization, and nitrification rates.
Methods: We conducted a meta-analysis of peer-reviewed studies to assess N transformation rates in agroecosystems. Specifically, we targeted studies employing 15N pool dilution methods to quantify N mineralization, immobilization, and nitrification rates in response to organic and synthetic soil amendments.
Results and discussion: Our findings indicate that adding synthetic, manure, and crop derived residues as soil amendments increased mineralization by 60%, 135%, and 214%, respectively, relative to the unamended controls. While manure and residue produced similar mineralization rates, residue amendments induced significantly higher immobilization rates than synthetic and manure amendments – a sevenfold and fourfold increase, respectively. Furthermore, only residue N amendments enhanced the ammonium (NH4+) pool size, while synthetic and manure amendments resulted in no change in NH4+ pool size. These results suggest that residue amendments encourage tighter coupling of the carbon (C) and N cycles compared to manure or synthetic amendments by delivering C rich substrates (e.g., C:N ratio >20:1) to soil microbes. This tighter coupling with residue amendments leads to faster mineralization-immobilization processes and larger NH4+ pools than those observed with manure or synthetic amendments. As such, residue amendments encourage soil N recycling between inorganic and organic forms, which is crucial to supporting crop N needs throughout the growing season while minimizing N losses.
1 Introduction
Nitrogen (N) is a limiting resource for aboveground biomass production (Vitousek and Howarth, 1991; LeBauer and Treseder, 2008) and is essential for promoting crop yield (Xu et al., 2012; Lassaletta et al., 2014). Growers use N-rich fertilizers to supply bioavailable N and optimize yields. However, most crops only use roughly 50% of the N supplied via external inputs (Allison, 1955; Robertson and Vitousek, 2009; Yan et al., 2019), resulting in high environmental N losses (Drinkwater and Snapp, 2007; Robertson and Vitousek, 2009; Butterbach-bahl et al., 2013; Paustian et al., 2016). To address this issue, there is growing interest in using alternative N sources such as crop residues and animal manures that supplement organic N pools. This strategy aims to reduce dependence on inorganic N sources that are highly reactive and vulnerable to losses (Bodirsky et al., 2014).
Organic amendments are a promising means to regulate N availability and potentially improve long-term soil fertility. Organic amendments facilitate carbon (C) and N cycling by furnishing organic C and N to the system, providing an alternative N source that reengages the microbial community’s role in N transformations. Most N in organic inputs must undergo microbial transformation to become bioavailable (Schimel and Bennett, 2004) with the balance between N mineralization and immobilization dependent on the stoichiometric ratio of both the microbial community and their substrate (Mooshammer et al., 2014b). Compost, and especially manure, often have low C:N (e.g., 5-20:1) and may thus provide microbes with excess N that is subsequently released in the process of N mineralization. In contrast, crop residues with high C:N (e.g., >30:1) may require additional N from the soil environment to maintain ideal stoichiometry resulting in N immobilization. However, crop residues can range from those with low C:N (e.g., clover) to others with high C:N (e.g., wheat chaff, corn stover). Therefore, the range in organic amendment quality, whether manure or crop residues, can cause high variability in N transformations in the soil (Trinsoutrot et al., 2000).
N mineralization and immobilization are key transformations affecting crop N availability and system-level crop N use efficiency (NUE) in agroecosystems. Thus, a deep understanding of how different organic and synthetic fertilizer amendments influence mineralization-immobilization dynamics will help inform the development of optimal fertilizer strategies. N isotope pool dilution may provide unique insights into both gross and net rates of N transformations and how they are impacted by organic and inorganic fertilizer inputs. Historically, researchers estimated N cycling rates by tracking changes in NH4+ or NO3- pools, which only offers insight into net fluxes. Net transformation rates and pool sizes do not fully represent N availability, potentially obscuring rapid shifts between inorganic and organic forms of N. Rapid N transformations due to soil organic matter (SOM) turnover deliver frequent small doses of bioavailable N to the soil (Nguyen et al., 2016; Soldatova et al., 2024), which can support plant growth, create a more efficient N cycle, and minimize N loss in natural ecosystems (Robertson and Groffman, 2024). This continual turnover and supply of N provides an approach to managing agroecosystems that reduces reliance on large and inefficient inorganic N pools (Drinkwater et al., 2017; Drinkwater and Snapp, 2022). Isotope pool dilution techniques give a more detailed view by measuring rates of both N production and consumption, reflecting microbial activity’s impact on N dynamics and providing additional insights into crop N availability.
Isotope pool dilution is a standard method used to quantify gross rates of N cycling based on the movement of a 15N tracer through the soil (Kirkham and Bartholomew, 1954). At the beginning of the incubation (t0), a known quantity of the 15N label is added to the NH4+ or NO3- pool. Then after a defined incubation period at t1, after the production of new NH4+ or NO3- dilutes the 15N pool with 14N, the difference in isotope enrichment between t0 and t1 informs calculations that estimate gross rates of N immobilization and mineralization. Before the widespread use of this method, and still today in many studies, researchers estimated N mineralization and nitrification rates by determining the change in overall NH4+ or NO3- pool size, providing just an estimate of net flux. Pool dilution methods capture the combined influence of microbial consumptive and productive processes, accounting for gross N production (i.e., mineralization) and consumption (i.e., immobilization) (Murphy et al., 2003). Pool dilution methods thus link microbial communities to organic N dynamics and cycling rates of key bioavailable N species. In agricultural soils, pool dilution techniques reveal the impact of management on microbial activity and N cycling, aiding in understanding crop N availability. For example, some agroecosystems appear to maintain yields despite low standing inorganic N pools, likely due to increased NUE (Fageria and Baligar, 2005; Xu et al., 2012; Lassaletta et al., 2014). One explanation for this may be faster processes of mineralization and immobilization. Rapid N cycling (e.g., Osterholz et al., 2017) can help maintain small inorganic N pool sizes, boost crop NUE, and maintain adequate crop N uptake (Grandy et al., 2022).
Twenty years have passed since Booth et al. (2005) published a meta-analysis that examined controls on N cycling in global terrestrial ecosystems with studies that utilized pool dilution methods. The authors identified ecosystem type as a primary moderator and treated agricultural systems uniformly without considering local conditions or specific management factors. Then, Gardner and Drinkwater (2009) used 15N crop recovery (via tracer experiments) to examine the efficiency of crop nutrient uptake from organic and inorganic N inputs to the soil. They found that organic inputs tended to have higher 15N input recovery in crops than synthetic inputs, highlighting the potential for organic nutrient inputs to supply plant N more efficiently than inorganic fertilizer inputs. A more recent meta-analysis (Mahal et al., 2018) showed that conservation agricultural practices like adding organic amendments can substantially increase potential net N mineralization but does not distinguish among types of N inputs. Understanding how different nutrient management practices affect microbial N processing and the balance of N production and consumption could inform practices that optimize crop yield, while minimizing environmental N losses.
To quantify broad patterns of N transformation rates in response to different soil N amendments, we conducted a meta-analysis that assessed 124-216 paired comparisons (depending on N transformation reported) from 18 studies that matched our criteria. We selected studies that exclusively employed 15N pool dilution methods to measure gross N transformation rates under zero (control) N amendments and synthetic or organic N amendments (treatment). We aimed to determine whether organic inputs enhance gross rates of mineralization and immobilization, as these N recycling processes can support plant available N during the growing season and reduce inorganic N losses.
2 Materials and methods
2.1 Data collection
We collected data from peer-reviewed publications from 1984 up to February 2024. Our meta-analysis used the online databases ISI Web of Knowledge and Google Scholar to search for relevant studies. We performed four searches using the asterisk (*) as a wildcard to broaden the scope of our search terms, with “min” defining broad usage of the term “mineralization,” employing the following combinations: (i) [Nitrogen min* AND soil AND pool dilution] OR [Nitrogen min* AND soil AND isotop*] AND [agri*]; (ii) [Gross nitrogen min* AND isotop*] AND [Gross nitrogen min* AND pool dilution]; (iii) [Nitrogen min* AND soil AND pool dilution] OR [Nitrogen min* AND soil AND isotop*]; (iv) nitrogen mineralization AND agri* AND soil AND pool dilution. We also screened published reviews and meta-analyses for publications that the search engines may have missed. We collected 512 studies that matched our search terms. We implemented the following criteria to screen the final database of studies: (i) studies were performed in an agroecosystem; (ii) gross N mineralization rates were measured by pool dilution methods and reported for both the reference (control) and experimental treatment; (iii) studies manipulated N amendments (organic and/or synthetic); (iv) studies reported values for non-amended soils; (v) the means, standard deviations or standard errors, and sample sizes were reported in text, figures, or tables.
In total, there were 26 studies that fulfilled our criteria. A total of five studies were removed from the final pool because they did not specify an unamended control. Finally, three more studies were removed from the final pool because the field manipulations received a one-time basal application of fertilizer at the beginning of the growing season. Therefore, the final pool of studies was further reduced from 26 studies to 18 studies (Table 1; Suppplementary Figure 1). All studies measured gross N mineralization rates, but N immobilization and nitrification rates were not consistently reported across all studies. We considered N mineralization, immobilization, nitrification rates, and NH4+ pool size the target response variables measured in the control and treatment groups. Throughout this manuscript, we will refer to mineralization, immobilization, and nitrification rates collectively as “N transformation rates” when discussing the broader context of N cycling to highlight their interconnected roles in soil N dynamics.
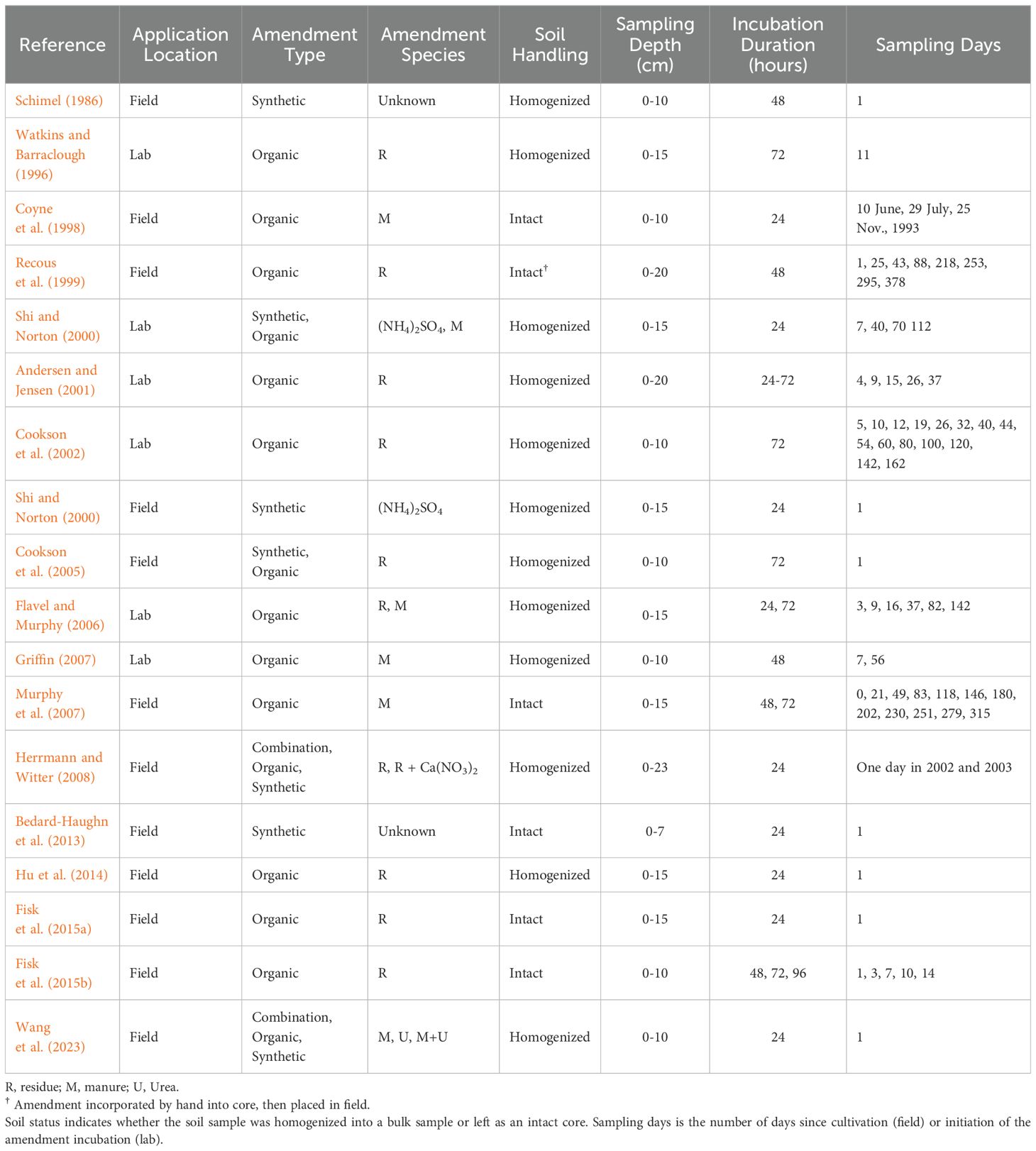
Table 1. Collection of studies included in the meta-analysis displaying amendment type and amendment species reported by each study.
The reference (control) group consisted of zero soil amendments and the experimental group consisted of soil amendments. There were three broad categories of amendment types that were applied as treatments in reference to the control: synthetic amendments, organic amendments, and in some cases, a combination of the two (referred to as a combination effect throughout this manuscript). Synthetic amendments were defined as inorganic mineral fertilizers, including urea, commonly applied to agricultural fields. Although urea’s chemical composition is technically organic, we classified it as a synthetic amendment due its industrial production and similarities to some inorganic fertilizers, such as rapid N availability and high N loss potential (Kirova-Yordanova, 2017). Organic amendments were defined as N-containing crop residues, green manures, and animal-based manures and were pooled together to obtain an overall effect size for organic amendments. When considering soil amendment type as a moderating variable, we subdivided it into two categories, plant derived amendments (e.g., crop residues and green manures) and animal derived amendments (e.g., pig slurry, cow manure). Plant and animal derived amendments will be referred to as “crop residues” and “manure,” respectively, throughout the manuscript. Often, there were multiple comparisons derived from each study (e.g., no amendment vs low amendment, no amendment vs high amendment) and we treated these comparisons as distinct comparisons within studies. In addition, several studies in our database conducted incubations with destructive harvests at multiple time points. We also considered each of these comparisons as distinctive datapoints but accounted for their non independence in our analysis (Nakagawa and Santos, 2012) (see below).
We extracted data presented in figure format with WebPlotDigitizer opensource software (Rohatgi, 2020). We converted N inputs (regardless of inorganic or organic species) to reflect a kg N ha-1 basis. If a study lacked the necessary information to calculate N contributions of residues (e.g., did not report the N content of residues or provide sufficient details on residue characteristics), we used C:N reported in the literature to fill information gaps and estimate N application rate via residue as accurately as possible. Specifically, three studies – Cookson et al. (2005) and Fisk et al. (2015a and 2015b) – did not provide enough information for precise calculations. In Cookson et al. (2005), the authors described the residue as “grass hay,” and given the wheat-based cropping system in this study, we referenced C:N values for wheat straw from the literature, which range from ~40-130:1 (Thomsen and Sørensen, 2006; Wu et al., 2010; Gan et al., 2011; Zhang et al., 2018). As a reasonable approximation for “grass hay” we assigned a C:N of 50:1 to this study to estimate N’s contribution from residues. For Fisk et al. (2015a, 2015b), residue applications occurred across multiple years with different crops in each year: barley straw in 2003, canola straw in 2006, and oat straw in 2010 – for both studies – and oat straw in 2012 for Fisk et al. (2015b). Barley straw C:N ranges from ~15-150:1 (Chantigny et al., 2001; Havstad et al., 2010; Naz et al., 2017; Reichel et al., 2022), canola straw C:N ranges from ~33-141:1 (Singh et al., 2006; Chico-Santamarta et al., 2011; Gan et al., 2011), and oat straw C:N ranges from 46-83:1 (Papavizas et al., 1962; Wu et al., 2010; Zhao et al., 2018). These wide ranges of C:N reflect variation due to crop variety, maturity at harvest, and climatic factors (e.g., moisture and temperature). Therefore, to estimate a representative N contribution from the three crop inputs, we used a 60:1 C:N in our calculations. This ratio represents a midpoint value within the ranges reported in the literature and aligns more closely with the narrower and lower range of oat straw, the most recent crop in rotation. In addition to amendment types, we also collected data on sample- and site-level descriptors to understand how environmental and edaphic characteristics may influence N transformation rates. These descriptors included total soil C and N, soil C:N, pH, sampling depth, incubation temperature, pool dilution incubation duration, and experimental duration.
The small sample size of some of our observations for synthetic and combined amendments may give more weight to organic amendments in the overall analysis. However, we reduced the number of publications examining the effects of synthetic amendments on N transformation rates due to the absence of a proper control group with no soil amendment. Consequently, we excluded these studies from our analysis, leading to a smaller sample size of paired observations. Furthermore, our extensive literature synthesis found limited studies investigating the combined effects of applying synthetic and organic amendments. Given that growers are likely to utilize a combination of synthetic and organic inputs to address soil fertility needs, more research is needed to assess gross N transformation rates under these input regimes.
Because of the limited number of studies that used pool dilution techniques and matched our criteria, our analysis included lab and field studies where the researchers manipulated soil amendments, which allowed us to broaden our dataset. While lab-based studies do not fully represent N cycling dynamics in the field, they do provide insight into potential soil responses to synthetic and organic inputs and were thus included. Additionally, even though field studies tend to have higher variances in a meta-analysis than lab studies, the field studies only contribute to a small portion of the overall heterogeneity in the variances (Hillebrand and Gurevitch, 2014). Therefore, overall conclusions from a meta-analysis that includes both lab and field studies are unlikely to be impacted by the differences in variances (Hillebrand and Gurevitch, 2014).
2.2 Meta-data analysis
We used the natural-log transformed ratio of the means (RR) as the effect size metric to quantify differences between the unamended and amended samples in our target studies, which is a common approach to calculating effect size in meta-analyses of ecological research (Hedges et al., 1999; Lajeunesse, 2011; Koricheva et al., 2013)
where the natural log of the RR is the proportional change in the means of treatment group () and control or reference, no amendment group (), The corresponding variances were calculated as:
where is the standard deviation and are the standard deviations and sample size of and . This method of calculating variances down-weights studies with large variances (Hedges et al., 1999; Lajeunesse, 2011). In the instance where variance was not reported and could not be calculated based on available information (n = 2), standard deviations were imputed based on the ratio of standard deviations and means from all studies that reported both values (Lajeunesse, 2013; Bowles et al., 2016).
We used the metafor package (Viechtbauer, 2010) to compute RRs and the corresponding confidence intervals. To account for non-independence of multiple observations within a study, we assigned publication ID as a random effect in our random-effects and mixed-effects meta-regression models. All meta-analyses were performed with the rma function, and the models were fit by using restricted maximum likelihood estimation. To obtain an overall effect size for each N transformation and NH4+ pool size, we constructed random effects model with publication ID as the random effect.
To examine the influence of different amendment types on N transformation rates, we conducted a meta-regression by including amendment type as a moderator and publication ID as the random effect. Specifically, we tested different moderators including amendment type (synthetic, organic, or combination) and amendment species (amendment species: residue, manure, residue + manure, synthetic fertilizer, organic + synthetic fertilizer), to explore the influence of broad amendment categories (organic vs synthetic fertilizer) and their subcategories on N transformation rates. This approach allowed us to assess not only whether synthetic or organic amendments had a larger effect but also the extent that specific amendment species influenced N transformation rates and NH4+ pool size. We conducted an omnibus test for each moderator to test for between-group heterogeneity for moderator levels and moderators were considered to influence N transformation rates if the Qm value was significant (p< 0.05) (Viechtbauer, 2010; Koricheva et al., 2013). For simplicity of interpretation, we transformed lnRRs and 95% CIs to reflect percent change from zero:
Transformed values of RR > 0 indicated a positive effect of soil amendments on N transformation rates in comparison to zero amendments, while values of transformed RR<0 indicated a negative effect of soil amendments on N transformation rates in comparison to zero amendments. We assessed publication bias by visually inspecting the funnel plot for asymmetry and applying the trim-and-fill method to detect and adjust for any potentially missing studies (Koricheva et al., 2013). We evaluated the overall heterogeneity of the model by performing the meta-analysis without any moderators (Viechtbauer, 2010).
We computed Pearson rank correlation coefficients to describe the relationships between N transformation rates, NH4+ pool size, amendment application rate, and site level characteristics using the rstatix package (Kassambara, 2019). Correlations were computed using the non-back transformed RR values. To minimize the impact of skewness and outliers and improve linearity between the variables, we applied log-transformations on amendment application rate, soil C, and soil N. To visualize the relationships between amendment application rate, mineralization, immobilization, and NH4+ pool size by amendment category (i.e., organic, synthetic, combination), we plotted regressions fitted with linear models and kernel density plots of the distributions using the GGally package (Schloerke et al., 2024).
All figures were generated using the packages ggplot2 (Wickham, 2016) and Ggally (Schloerke et al., 2024). All statistical analyses were performed in the statistical computing software R (version 4.3.3, R Core Team, 2020). Flow diagram of study selection (Supplementary Figure 1) was created using the PRISMA Flow Diagram tool (Haddaway et al., 2022).
3 Results
Eighteen studies measuring gross N mineralization rates with 15N isotope pool dilution methods showed that soil amendments increased N mineralization, immobilization, and nitrification rates in agricultural soils. Overall, amendments enhanced N mineralization rates by 172% (p< 0.001), N immobilization rates by 147% (p< 0.001), and nitrification rates by over 200% (p< 0.001; Figure 1) relative to zero amendments. N transformation rates were generally low in the control group and high in the experimental group across all studies (Table 2).
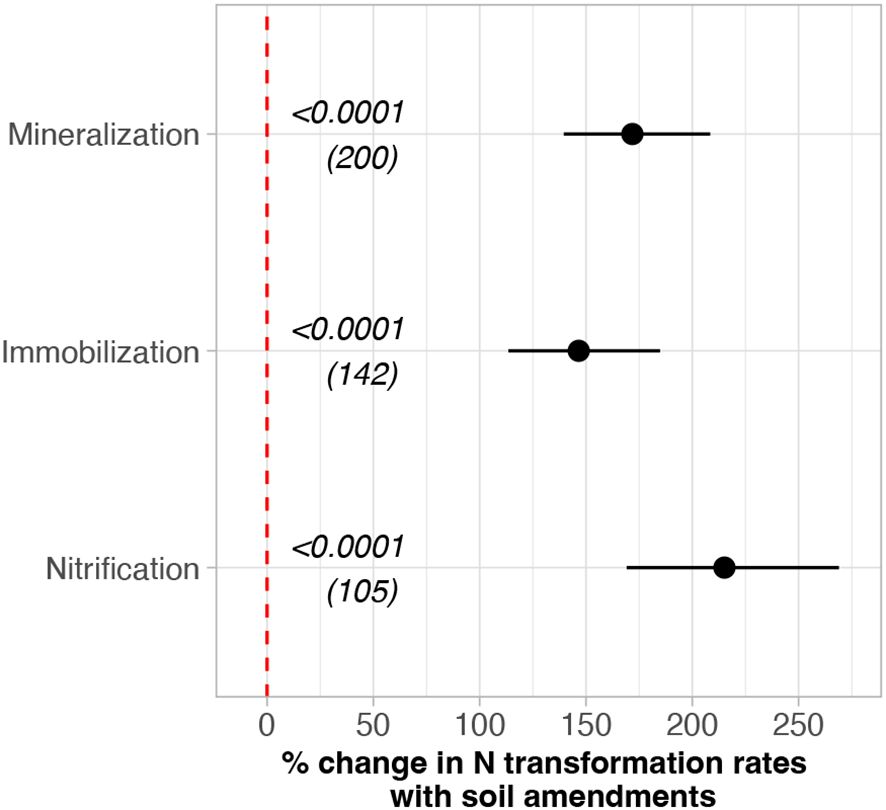
Figure 1. Overall amendment effects (% change) on N transformation rates in the soil. A separate meta-analysis was run for each N transformation rates. Amendment effects were calculated as the back-transformed response ratios (RR). Bars represent 95% confidence intervals. Effects are considered significant if the CIs do not overlap with zero. Numbers in parentheses indicate the number of paired comparisons.
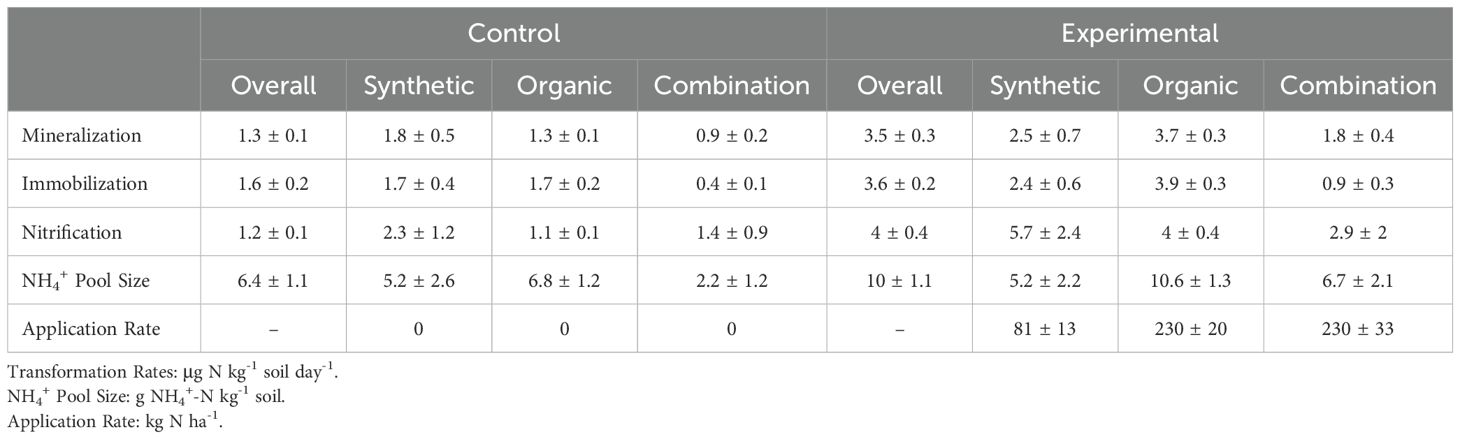
Table 2. Overall and moderator level means and standard errors for N transformation rates, NH4+ pool size, and amendment application rate for control and experimental treatments across all studies.
The addition of soil amendments, regardless of amendment type, facilitated an overall increase in mineralization rates (Supplementary Table 2). Organic amendments increased N mineralization rates by 187% (p< 0.0001; CI lower = 151, CI upper = 229), and synthetic amendments increased N mineralization rates by 60% (p = 0.05; CI lower = 1, CI upper = 154). Combining organic and synthetic amendments increased N mineralization rates by 137% (p< 0.001; CI lower = 50, CI upper = 275). Organic amendments also increased N immobilization rates by 164% (p< 0.0001; CI lower = 127, CI upper =208) and nitrification rates by 220% (p< 0.0001; CI lower = 171, CI upper =227). Synthetic amendments did not affect N immobilization rates but did increase nitrification rates by 204% (p = 0.005; CI lower = -13, CI upper = 106). Combined amendments increased immobilization rates by 129% (p = 0.01; CI lower = 22, CI upper = 330) but did not influence nitrification rates.
No differences in N mineralization rates were observed between the residue and manure amendments (Figure 2A). However, residue resulted in a 240% increase in immobilization rates (p< 0.0001; Figure 2B), while manure amendments resulted in a 57% increase in immobilization rates (p< 0.001; Figure 2B). Residue amendments also had a larger impact on nitrification rates than manure, with a 306% (p< 0.0001; Figure 2C) and 37% (p = 0.05; Figure 2C) change, respectively. Within the combination treatments, both residue + manure and organic + synthetic amendment categories resulted in significant increases in mineralization rates but were not different from each other (Figure 2A). While the organic + synthetic combination treatment increased immobilization rates (p = 0.001), it was not statistically different from the residue + manure treatment (Figure 2B).
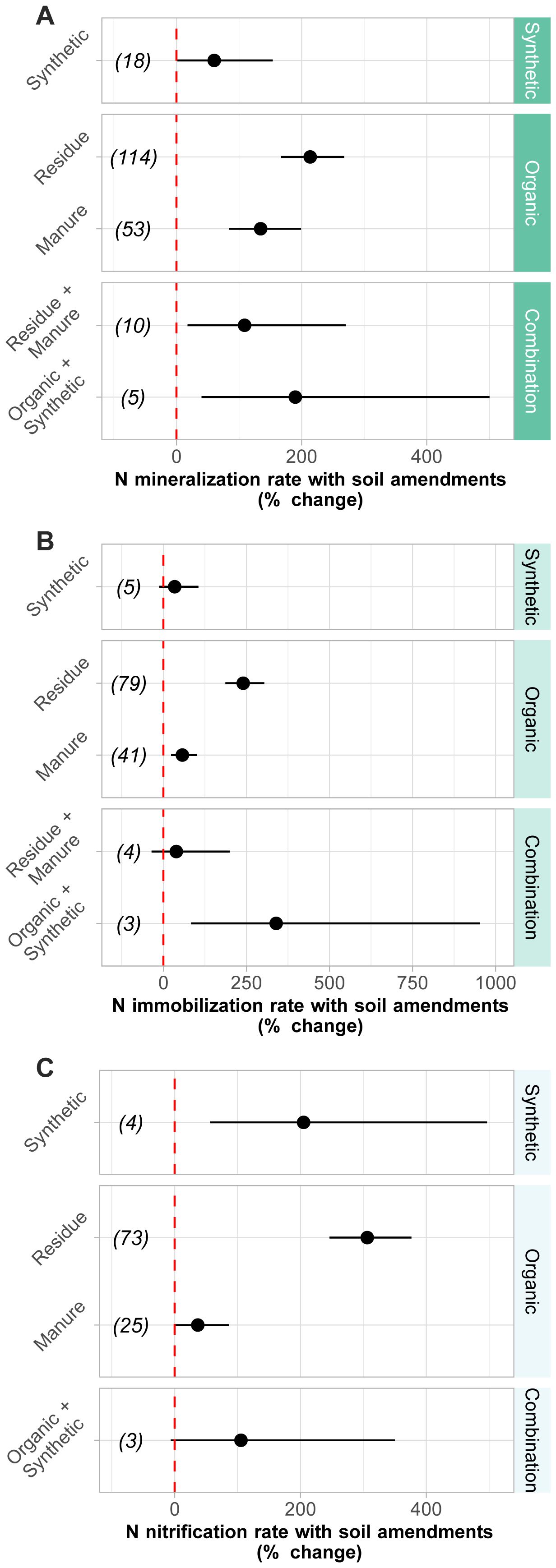
Figure 2. Meta-analysis results of the change in (A) mineralization (p < 0.001), (B) immobilization (p < 0.001), and (C) nitrification (p < 0.001) rates in response to different soil amendment types. Numbers in parentheses indicate the number of paired comparisons. Individual moderator parameters are significantly different from one another if the 95% CI do not overlap. Overall effects of each moderator parameter are considered significant if 95% CI do not overlap with zero. Amendment effects were calculated as the back-transformed response ratios (RR). Bars represent 95% confidence intervals.
Mostly, NH4+ pool size did not change with soil amendment treatment type. However, NH4+ increased by 83% under residue amendments relative to zero amendments (p< 0.0001; Figure 3). Additionally, the (i.e., amendment effect on NH4+ pool size) correlated weakly with the overall amendment application rate; specifically, organic amendments were weakly and negatively correlated with , while synthetic and combination amendments had no relationship with the (Figure 4D). Organic amendments, on average, stimulated mineralization at a rate of nearly three times that of synthetic amendments (Table 2). However, neither organic nor synthetic amendment application rate significantly correlated with (i.e., amendment effect on mineralization rates) or i.e., amendment effect on immobilization rates) (Figures 4A, B). These findings imply that even though organic amendments often contributed more to the NH4+ pool than synthetic amendments, the overall quantity of N applied via amendment does not drive differences in N transformation rates.
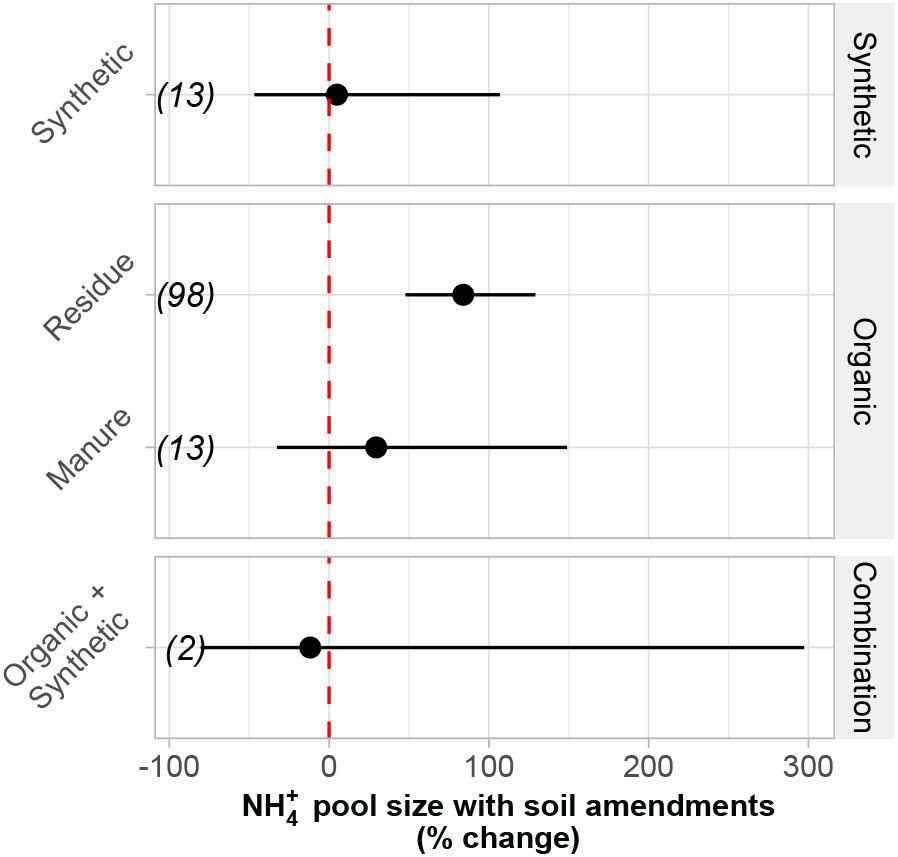
Figure 3. Change in NH4+ pool concentration in response to different soil amendments. Numbers in parentheses indicate the number of paired comparisons. Individual moderator parameters are significantly different from one another if the 95% CI do not overlap. Overall effects of each moderator parameter are considered significant if 95% CI do not overlap with zero. Amendment effects were calculated as the back-transformed response ratios (RR). Bars represent 95% confidence intervals.
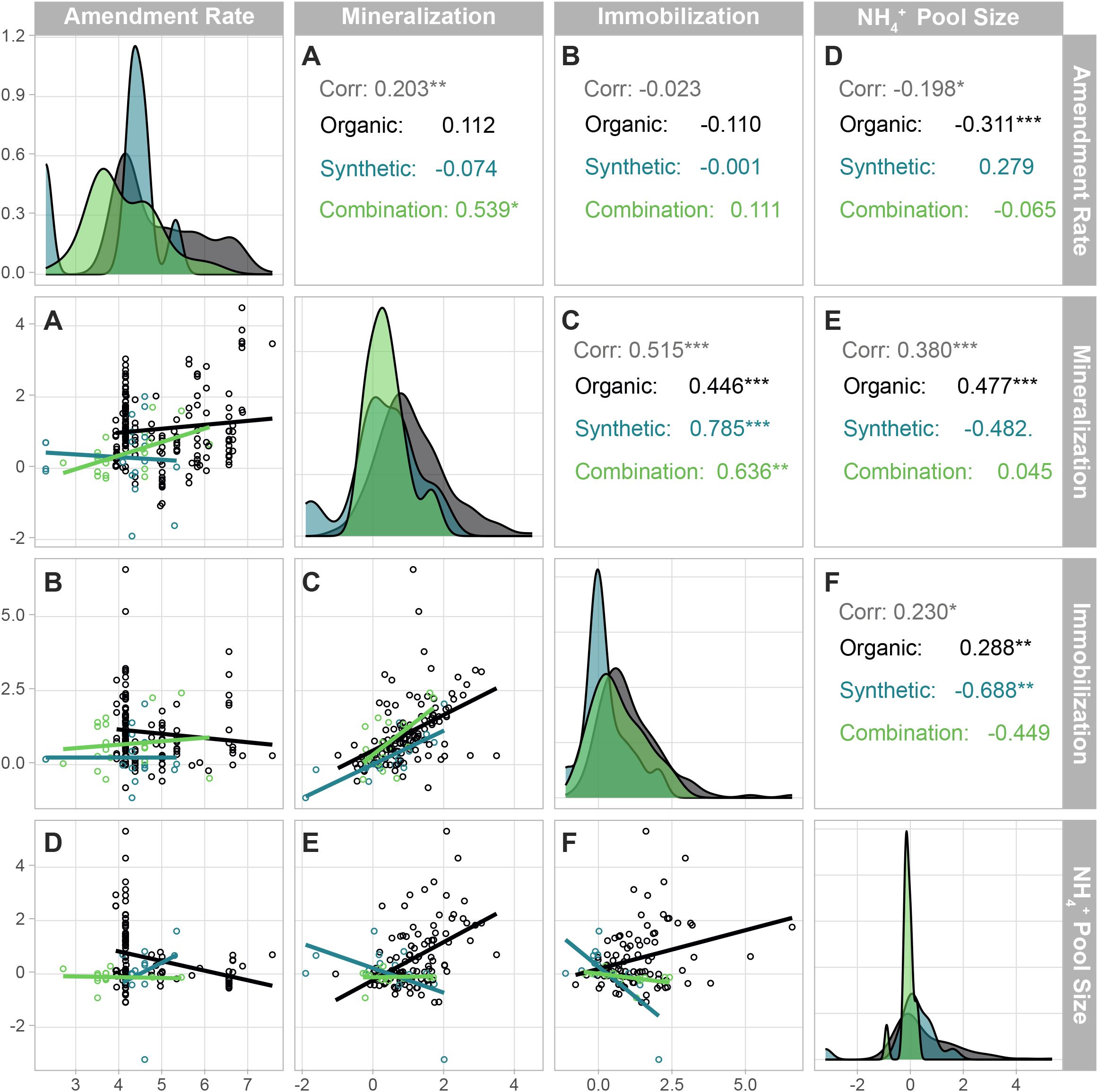
Figure 4. Correlation matrix showing the correlations (lower triangle) and the distribution of the data (diagonal) for each amendment type. Pearson correlations between amendment application rate, mineralization rate, immobilization rate, and NH4+ pool size are displayed in the lower triangle of the matrix. The correlation coefficients for each correlation combination are displayed in the upper triangle of the matrix. “Corr” (the overall correlation coefficient irrespective of amendment type) is displayed on top (gray color) and the individual correlations for each amendment type are displayed underneath. The 1-to-1 diagonal shows the distribution of the spread of the data for each variable, separated into “Organic” (black), “Synthetic” (blue), or “Combination” (green) amendment types. Asterisks represent correlation significance: ***<0.0001, **<0.01, *<0.05. Letters correspond to correlation pairings, and match correlations to their respective correlation coefficients: (A) mineralization and amendment rate, (B) immobilization and amendment rate, (C) mineralization and immobilization, (D) NH4+ pool size and amendment rate, (E) mineralization and NH4+ pool size, and (F) immobilization and NH4+ pool size.
However, Pearson correlations revealed strong relationships between the and (Figure 4E) and and (Figure 4F). Additionally, there was a strong relationship between AEmin and AE imb (Figure 4C). Unamended soil properties from each study, including soil C and N, C:N, and soil pH, showed minimal correlation with N transformation rate RRs (Supplementary Table 2). While correlated with and , its correlation with soil pH and C was weak and negative, and we found no significant correlation with soil N or the C:N. demonstrated weak, negative correlations with soil pH and the C:N but not with soil C or N. Finally, (i.e., amendment effect on nitrification rates) showed a moderate, negative correlation with soil pH and C:N, and weak correlations with soil C and N.
4 Discussion
Understanding the influence of soil amendments on N cycling dynamics within agroecosystems is vital for developing effective nutrient management strategies. These strategies rely on optimizing internal N cycling and require balancing external inorganic and organic N inputs to the system. Our meta-analysis examined the change in N transformation rates via pool dilution methods in response to different organic and synthetic soil amendments (i.e., ) and highlights the role that organic amendments, such as cow manure and crop residues, play in enhancing N cycling rates. Specifically, residue-based amendments increased mineralization () rates more than synthetic amendments and increased immobilization () rates more than both synthetic and manure amendments (Figure 2). In contrast, neither manure nor synthetic amendments induced a significant .
We found that manure and inorganic fertilizer contribute to an overall increase in mineralization rates of organic N to inorganic forms, increasing plant available N. However, high mineralization rates and large inorganic N pools also lead to environmental N losses (Galloway et al., 2008; Erisman et al., 2013). Our meta-analysis indicates that crop residue amendments produced higher N mineralization and immobilization rates than manure and synthetic N sources. Mineralization (i.e., microbial N production) releases NH4+ making it available for plant uptake or susceptible to volatilization (i.e., NH3 release) or nitrification, which is prone to leaching NO3- (Erisman et al., 2013). Immobilization (i.e., microbial N consumption) reduces plant-accessible N but lowers the risk of N losses from the system. Thus, boosting immobilization and mineralization provides a potential means to increase soil N storage and plant N uptake (Schimel and Bennett, 2004; Daly et al., 2021). Ultimately, strategically combining crop residues, manure, and inorganic fertilizers may help optimize the opportunities for crop N uptake without excess soil N vulnerable to offsite losses (Vanlauwe and Giller, 2006; Drinkwater and Snapp, 2022; Grandy et al., 2024).
N amendments – synthetic, organic, or a combination – increased mineralization rates by roughly 60-215%, while residue was the only N amendment to substantially increase immobilization and nitrification rates. Our results contradict the findings of (Booth et al., 2005), who found that the overall effect of fertilizer treatments (inorganic and organic) did not affect mineralization rates, immobilization rates, or nitrification rates. However, the authors did not distinguish between organic and inorganic amendments in the determination of the overall effect size, therefore it is not possible to ascertain whether organic amendments indeed increased N transformation rates in their analysis. Furthermore, the authors reported that individual studies using organic fertilizer sources resulted in significant increases in mineralization rates. Our study parsed apart the amendment effect (i.e., synthetic vs. organic amendment) on N transformations and, consistent with existing research (e.g., Kumar and Goh, 2003; Diacono and Montemurro, 2010; Chen et al., 2014a), found that organic matter incorporation into agricultural soils can stimulate mineralization rates, as reflected in our findings of a strong organic amendment effect.
A recent meta-analysis conducted by Mahal et al. (2018) found that, on average, cropping systems that received N amendments – regardless of form – had higher potentially mineralizable N (PMN) relative to the non-amended controls. Specifically, the authors found that compost and manure amendments increased PMN by 52% and 78%, respectively, while inorganic amendments did not induce changes in PMN. We found a slight increase in mineralization rates with added synthetic amendments but a much larger one with crop residues and manure. Our findings and those from Mahal et al. (2018) suggest that the contribution of synthetic fertilizer N enhances the inorganic N pool but has relatively less or no impact on N mineralization.
Synthetic amendments significantly increased both N mineralization and nitrification rates but had no detectable effect on immobilization rates. This finding suggests that, from a microbial perspective, there is an excess of available N with respect to C. Applying synthetic fertilizers often enhances crop productivity, resulting in increased crop residue inputs to soil and higher microbial biomass than crops receiving low or no fertilizer (Geisseler and Scow, 2014). This potentially high rate of residue inputs coupled with an active microbial community in a strongly N-enriched environment will induce microbial N spillover (Mooshammer et al., 2014a). Even if N fertilization has no effect or inhibits decomposition rates (Grandy et al., 2013), microbes may still release inorganic N during decomposition (Breza et al., 2023).
Soil amendment stoichiometry is a major driver of microbial decomposition processes and N cycling and governs how organic inputs influence soil nutrient dynamics (Keiblinger et al., 2010; Chen et al., 2014b). Substrates with low C:N (e.g., manure and green manure) fuel the microbial community by providing easily accessible forms of C balanced with available N, thereby increasing mineralization rates and short-term N release into the soil (Flavel and Murphy, 2006; Myrold and Bottomley, 2015). In contrast, high C:N inputs like crop residues contribute to the SOM pool both in the short and long term, providing C resources over extended periods and sustaining bioavailable N through mineralization-immobilization (Nguyen et al., 2016). Incorporating high quality crop residues with low to medium C:N (e.g., 20-30:1) may enhance SOM content in the short term (Ding et al., 2006; Tiemann et al., 2015), creating a fresh pool of substrates that can immediately stimulate microbial activity (Wang et al., 2015). For example, a study from our database (Herrmann and Witter, 2008) tested the immobilization potential of various N inputs to quantify the contribution of amendments to net NH4+ availability. The authors found a linear relationship between immobilization rates and microbial respiration rates irrespective of amendment C:N, suggesting the background SOM pool of their soils contained enough C to facilitate NH4+ immobilization. Over the long term, residue inputs contribute toward building the stable SOM pool that may ultimately drive immobilization rates (Yan et al., 2019). However, substrate quality is a key component in dictating N transformation rates, and distinguishing between whether the N input itself, the long-term accumulation of inputs, or an interaction of the two is a stronger driving force remains uncertain.
The existing organic N pool is an essential source of NH4+, and implementing management strategies that build this pool (e.g., crop residue inputs) can contribute to increased N transformation rates and soil NH4+ concentrations (Farzadfar et al., 2021). While growers typically rely on inorganic N application rates to supply most or all plant-available N, the turnover of in situ organic matter can also be a significant N source (Näsholm et al., 2000; Snapp and Fortuna, 2003; Geisseler et al., 2009). We found that synthetic N inputs and manure amendments did not alter NH4+ pool size relative to the unamended control. Yet, we found that residue inputs increased the NH4+ pool size by roughly 75% compared to no inputs. Moreover, there was a weak negative relationship between N application rate and NH4+ pool size, but only for organic amendments. These results suggest that amendment type facilitates changes in NH4+ pool size rather than the quantity of N applied, per se, via external N inputs. As such, more N amendments do not necessarily mean an increase in the NH4+ pool. Local edaphic factors like clay content (Vogel et al., 2015), soil temperature and moisture levels (Guntiñas et al., 2012; Robertson and Groffman, 2024), and pH (Averill and Waring, 2018) can all influence N transformations rates and NH4+ storage in the soil. However, within the context of our analysis, the increase in mineralization rates indicates greater NH4+ production, but high immobilization rates under residue amendments suggest that microbial metabolic requirements may restrict the persistence of the NH4+ pool. Therefore, with residue amendments, C and N cycling may remain more tightly coupled (Gardner and Drinkwater, 2009; King and Hofmockel, 2017; Stella et al., 2019) where microbes assimilate NH4+ based on greater C availability (Mooshammer et al., 2014a).
While this meta-analysis detected compelling patterns in N transformation rates in response to different N amendments, there are limitations and considerations, primarily due to the number of studies included in the analysis. Although we initially identified about 40 viable studies, our selection criteria reduced the final number to 18 studies (see PRISMA flow diagram, Supplementary Figure 1). As such, the number of studies that manipulated synthetic or organic amendments was imbalanced, with six of the studies manipulating synthetic amendments and 12 manipulating organic amendments. Additionally, of the six studies on synthetic N, only two used the same fertilizer type, while the others did not report the chemical composition. The under-reporting of synthetic amendments limits our ability to fully understand their impact. Altogether, we conducted the amendment comparisons at a very high level (e.g., organic vs. synthetic), with organic amendments separated into plant residues or animal-derived manures and synthetic amendments as a single category, reflecting the minimal available data. These aspects of our study suggest some caution in generalizing the findings, particularly for synthetic amendments. Nonetheless, there is value in understanding how broad patterns of soil respond to inputs, even if specific responses vary depending on the context.
5 Conclusions
This meta-analysis demonstrated that organic-based soil amendments, especially crop residues, enhanced N mineralization, immobilization, and nitrification rates and increase the NH4+ pool size. While manure and synthetic N fertilizers also increased N mineralization rates, neither amendment altered immobilization or nitrification rates. This suggests that these amendments result in high N bioavailability, prompting microbial release of excess N, which can support crop production but also might increase potential N offsite movement. Applying N amendments that allow for slower N release and balance mineralization-immobilization dynamics over longer periods of time may help support production goals and reduce excess N and microbial spillover, thereby improving N retention within the agroecosystem. The effect of combining organic and synthetic amendments on N transformation rates remains ambiguous. However, future research could expand on these findings by examining gross N mineralization rates under different agricultural contexts and investigating how interactions between synthetic and organic nutrients influence these transformations. These insights have the potential to deepen our understanding of N cycling dynamics and help refine input strategies, contributing to climate smart solutions that maintain agricultural productivity and increase sustainability.
Data availability statement
The raw data supporting the conclusions of this article will be made available by the authors, without undue reservation.
Author contributions
LB: Conceptualization, Data curation, Formal analysis, Investigation, Methodology, Funding acquisition, Visualization, Writing – original draft, Writing – review & editing. AG: Conceptualization, Funding acquisition, Supervision, Writing – original draft, Writing – review & editing.
Funding
The author(s) declare financial support was received for the research, authorship, and/or publication of this article. This work was partially funded by the National Science Foundation Graduate Research Fellowship Program (DGE 1450271). Partial funding was also provided by the New Hampshire Agricultural Experiment Station. This work is supported by the USDA National Institute of food and Agriculture Hatch Project #70003624 and the state of New Hampshire.
Acknowledgments
We greatly appreciate T. Hennas’s efforts in data collection and extraction. We would also like to thank A.B. Daly, T. Bowles, S. Frey, and R. Smith for manuscript feedback. We are thankful for the support from the New Hampshire Agricultural Experiment Station. Finally, we are grateful to the reviewers and editors for their thorough and constructive feedback.
Conflict of interest
The authors declare that the research was conducted in the absence of any commercial or financial relationships that could be construed as a potential conflict of interest.
Publisher’s note
All claims expressed in this article are solely those of the authors and do not necessarily represent those of their affiliated organizations, or those of the publisher, the editors and the reviewers. Any product that may be evaluated in this article, or claim that may be made by its manufacturer, is not guaranteed or endorsed by the publisher.
Supplementary material
The Supplementary Material for this article can be found online at: https://www.frontiersin.org/articles/10.3389/fagro.2025.1472749/full#supplementary-material
Abbreviations
Amendment Effect, the natural-log transformed ratio of the means (i.e., response ratio; RR) quantifying the differences between amended and unamended soils; AEΔ, Percent change in transformation rates (or NH4+ pool size) with respect to zero (i.e., back-transformed RR); AEmin, Amendment effect on mineralization rates; AEimb, Amendment effect on immobilization rates; AEnit, Amendment effect on nitrification rates; , Amendment effect on NH4+ pool size.
References
Allison F. E. (1955). The enigma of soil nitrogen balance sheets. Plant Soil 7, 213–250. doi: 10.1016/S0065-2113(08)60339-9
Andersen M. K., Jensen L. S. (2001). Low soil temperature effects on short-term gross n mineralisation–immobilisation turnover after incorporation of a green manure. Soil Biol. Biochem. 33 (4–5), 511–521. doi: 10.1016/S0038-0717(00)00192-9
Averill C., Waring B. (2018). Nitrogen limitation of decomposition and decay: How can it occur? Glob. Chang. Biol. 24, 1417–1427. doi: 10.1111/gcb.13980
Bedard-Haughn A., Comeau L.-P., Sangster A. (2013). Gross nitrogen mineralization in pulse-crop rotations on the northern great plains. Nutr. Cycl Agroecosyst 95 (2), 159–174. doi: 10.1007/s10705-013-9555-z
Bodirsky B. L., Popp A., Lotze-Campen H., Dietrich J. P., Rolinski S., Weindl I., et al. (2014). Reactive nitrogen requirements to feed the world in 2050 and potential to mitigate nitrogen pollution. Nat. Commun. 5, 3858. doi: 10.1038/ncomms4858
Booth M. S., Stark J. M., Rastetter E. (2005). Controls on nitrogen cycling in terrestrial ecosystems: A synthetic analysis of literature data. Ecol. Monogr. 75, 139–157. doi: 10.1890/03-8021
Bowles T. M., Jackson L. E., Malina L., Cavagnaro T. R., Nuñez M. (2016). Ecological intensification and arbuscular mycorrhizas: a meta-analysis of tillage and cover crop effects. J. Appl. Ecol. 54, 1785–1793. doi: 10.1111/1365-2664.12815
Breza L. C., Mooshammer M., Bowles T. M., Jin V. L., Schmer M. R., Thompson B., et al. (2023). Complex crop rotations improve organic nitrogen cycling. Soil Biol. Biochem. 177, 108911. doi: 10.1016/j.soilbio.2022.108911
Butterbach-bahl K., Baggs E. M., Dannenmann M., Kiese R., Zechmeister-boltenstern S. (2013). Nitrous oxide emissions from soils: how well do we understand the processes and their controls? Phil. Trans. R Soc. B 368, 1–20. doi: 10.1098/rstb.2013.0122
Chantigny M. H., Rochette P., Angers D. A. (2001). Short-term C and N dynamics in a soil amended with pig slurry and barley straw: a field experiment. Can. J. Soil Sci. 81, 131–137. doi: 10.4141/S00-046
Chen B., Liu E., Tian Q., Yan C., Zhang Y. (2014a). Soil nitrogen dynamics and crop residues. A review. Agron. Sustain. Dev. 34, 429–442. doi: 10.1007/s13593-014-0207-8
Chen R., Senbayram M., Blagodatsky S., MyaChina O., Dittert K., Lin X., et al. (2014b). Soil C and N availability determine the priming effect: Microbial N mining and stoichiometric decomposition theories. Global Change Biol. 20, 2356–2367. doi: 10.1111/gcb.12475
Chico-Santamarta L., Humphries A. C., Chaney K., White D. R., Magan N., Godwin R. J. (2011). Microbial changes during the on-farm storage of canola (oilseed rape) straw bales and pellets. Biomass Bioenergy 35, 2939–2949. doi: 10.1016/j.biombioe.2011.03.025
Cookson W. R., Abaye D. A., Marschner P., Murphy D. V., Stockdale E. A., Goulding K. W. T. (2005). The contribution of soil organic matter fractions to carbon and nitrogen mineralization and microbial community size and structure. Soil Biol. Biochem. 37, 1726–1737. doi: 10.1016/j.soilbio.2005.02.007
Cookson W. R., Cornforth I. S., Rowarth J. S. (2002). Winter soil temperature (2–15°C) effects on nitrogen transformations in clover green manure amended or unamended soils; a laboratory and field study. Soil Biol. Biochem. 34 (10), 1401–1415. doi: 10.1016/S0038-0717(02)00083-4
Coyne M. S., Zhai Q., MacKown C. T., Barnhisel R. I. (1998). Gross nitrogen transformation rates in soil at a surface coal mine site reclaimed for prime farmland use. Soil Biol. Biochem. 30 (8–9), 1099–1106. doi: 10.1016/S0038-0717(97)00202-2
Daly A. B., Jilling A., Bowles T. M., Buchkowski R. W., Frey S. D., Kallenbach C. M., et al. (2021). A holistic framework integrating plant-microbe-mineral regulation of soil bioavailable nitrogen. Biogeochemistry 154, 211–229. doi: 10.1007/s10533-021-00793-9
Diacono M., Montemurro F. (2010). Long-term effects of organic amendments on soil fertility. A review. Agron. Sustain. Dev. 30, 401–422. doi: 10.1051/agro/2009040
Ding G., Liu X., Herbert S., Novak J., Amarasiriwardena D., Xing B. (2006). Effect of cover crop management on soil organic matter. Geoderma 130, 229–239. doi: 10.1016/j.geoderma.2005.01.019
Drinkwater L. E., Schipanski M., Snapp S., Jackson L. E. (2017). “Ecologically Based Nutrient Management,” in Agricultural Systems (London, UK: Elsevier), 203–257. doi: 10.1016/B978-0-12-802070-8.00007-4
Drinkwater L. E., Snapp S. S. (2007). Nutrients in agroecosystems: rethinking the management paradigm. Adv. Agron. 92, 163–186. doi: 10.1016/S0065-2113(04)92003-2
Drinkwater L. E., Snapp S. S. (2022). Advancing the science and practice of ecological nutrient management for smallholder farmers. Front. Sustain. Food Syst. 6. doi: 10.3389/fsufs.2022.921216
Erisman J. W., Galloway J. N., Seitzinger S., Bleeker A., Dise N. B., Petrescu A. M. R., et al. (2013). Consequences of human modification of the global nitrogen cycle. Philos. Trans. R. Soc. B: Biol. Sci. 368, 20130116. doi: 10.1098/rstb.2013.0116
Fageria N. K., Baligar V. C. (2005). Enhancing nitrogen use efficiency in crop plants. Adv. Agron. 88, 97–185. doi: 10.1016/S0065-2113(05)88004-6
Farzadfar S., Knight J. D., Congreves K. A. (2021). Soil organic nitrogen: an overlooked but potentially significant contribution to crop nutrition. Plant Soil 462, 7–23. doi: 10.1007/s11104-021-04860-w
Fisk L. M., Barton L., Jones D. L., Glanville H. C., Murphy D. V. (2015a). Root exudate carbon mitigates nitrogen loss in a semi-arid soil. Soil Biol. Biochem. 88, 380–389. doi: 10.1016/j.soilbio.2015.06.011
Fisk L. M., Maccarone L. D., Barton L., Murphy D. V. (2015b). Nitrapyrin decreased nitrification of nitrogen released from soil organic matter but not amoA gene abundance at high soil temperature. Soil Biol. Biochem. 88, 214–223. doi: 10.1016/j.soilbio.2015.05.029
Flavel T. C., Murphy D. V. (2006). Carbon and nitrogen mineralization rates after application of organic amendments to soil. J. Environ. Qual. 35, 183–193. doi: 10.2134/jeq2005.0022
Galloway J. N., Townsend A. R., Erisman J. W., Bekunda M., Cai Z., Freney J. R., et al. (2008). Transformation of the nitrogen cycle: recent trends, questions, and potential solutions. Science 320, 889–892. doi: 10.1126/science.1136674
Gan Y. T., Liang B. C., Liu L. P., Wang X. Y., McDonald C. L. (2011). C:N ratios and carbon distribution profile across rooting zones in oilseed and pulse crops. Crop Pasture Sci. 62, 496. doi: 10.1071/CP10360
Gardner J. B., Drinkwater L. E. (2009). The fate of nitrogen in grain cropping systems : A meta-analysis of 15N field experiments. Ecol Appl 19, 2167–2184. doi: 10.1890/08-1122.1
Geisseler D., Horwath W. R., Doane T. A. (2009). Significance of organic nitrogen uptake from plant residues by soil microorganisms as affected by carbon and nitrogen availability. Soil Biol. Biochem. 41, 1281–1288. doi: 10.1016/j.soilbio.2009.03.014
Geisseler D., Scow K. M. (2014). Long-term effects of mineral fertilizers on soil microorganisms - A review. Soil Biol. Biochem. 75, 54–63. doi: 10.1016/j.soilbio.2014.03.023
Grandy A. S., Daly A. B., Bécu R., Cardinael R., Fontaine S., Jilling A., et al. (2024). A microbial framework for nitrogen cycling solutions in agroecosystems. One Earth. 7(12), 2103–2107. doi: 10.1016/j.oneear.2024.11.018
Grandy A. S., Daly A. B., Bowles T. M., Gaudin A. C. M., Jilling A., Leptin A., et al. (2022). The nitrogen gap in soil health concepts and fertility measurements. Soil Biol. Biochem. 175, 108856. doi: 10.1016/j.soilbio.2022.108856
Grandy A. S., Salam D. S., Wickings K., McDaniel M. D., Culman S. W., Snapp S. S. (2013). Soil respiration and litter decomposition responses to nitrogen fertilization rate in no-till corn systems. Agriculture Ecosyst. Environ. 179, 35–40. doi: 10.1016/j.agee.2013.04.020
Griffin T. S. (2007). Estimates of gross transformation rates of dairy manure n using 15 n pool dilution. Commun. Soil Sci. Plant Anal. 38 (11–12), 1451–1465. doi: 10.1080/00103620701378409
Guntiñas M. E., Leirós M. C., Trasar-Cepeda C., Gil-Sotres F. (2012). Effects of moisture and temperature on net soil nitrogen mineralization: A laboratory study. Eur. J. Soil Biol. 48, 73–80. doi: 10.1016/j.ejsobi.2011.07.015
Haddaway N. R., Page M. J., Pritchard C. C., McGuinness L. A. (2022). PRISMA2020: An R package and Shiny app for producing PRISMA 2020-compliant flow diagrams, with interactivity for optimised digital transparency and Open Synthesis. Campbell Systematic Rev. 18, e1230. doi: 10.1002/cl2.1230
Havstad L. T., Aamlid T. S., Henriksen T. M. (2010). Decomposition of straw from herbage seed production: Effects of species, nutrient amendment and straw placement on C and N net mineralization. Acta Agriculturae Scandinavica Section B - Soil Plant Sci. 60, 57–68. doi: 10.1080/09064710802616548
Hedges L. V., Gurevitch J., Curtis P. S. (1999). The meta-analysis of response ratios in experimental ecology. Ecology 80, 1150. doi: 10.2307/177062
Herrmann A. M., Witter E. (2008). Predictors of gross N mineralization and immobilization during decomposition of stabilized organic matter in agricultural soil. Eur. J. Soil Sci. 59, 653–664. doi: 10.1111/j.1365-2389.2008.01023.x
Hillebrand H., Gurevitch J. (2014). Meta-analysis results are unlikely to be biased by differences in variance and replication between ecological lab and field studies. Oikos 123, 794–799. doi: 10.1111/oik.01288
Hu Y.-L., Wu F.-P., Zeng D.-H., Chang S. X. (2014). Wheat straw and its biochar had contrasting effects on soil c and n cycling two growing seasons after addition to a black chernozemic soil planted to barley. Biol. Fertil Soils 50 (8), 1291–1299. doi: 10.1007/s00374-014-0943-6
Kassambara A. (2023). rstatix: Pipe-Friendly Framework for Basic Statistical Tests. R package version 0.7.2, Available online at: https://CRAN.R-project.org/package=rstatix.
Keiblinger K. M., Hall E. K., Wanek W., Szukics U., Hämmerle I., Ellersdorfer G., et al. (2010). The effect of resource quantity and resource stoichiometry on microbial carbon-use-efficiency. FEMS Microbiol. Ecol. 73, 430–440. doi: 10.1111/j.1574-6941.2010.00912.x
King A. E., Hofmockel K. S. (2017). Diversified cropping systems support greater microbial cycling and retention of carbon and nitrogen. Agriculture Ecosyst. Environ. 240, 66–76. doi: 10.1016/j.agee.2017.01.040
Kirkham D., Bartholomew W. V. (1954). Equations for following nutrient transformations in soil, utilizing tracer data1. Soil Sci. Soc. America J. 18, 33. doi: 10.2136/sssaj1954.03615995001800010009x
Kirova-Yordanova Z. (2017). Exergy-based estimation and comparison of urea and ammonium nitrate production efficiency and environmental impact. Energy 140, 158–169. doi: 10.1016/j.energy.2017.08.086
Koricheva J., Gurevitch J., Mengersen K. (Eds.) (2013). Handbook of Meta-analysis in Ecology and Evolution (Princeton: Princeton University Press). doi: 10.2307/j.ctt24hq6n
Kumar K., Goh K. M. (2003). Nitrogen release from crop residues and organic amendments as affected by biochemical composition. Commun. Soil Sci. Plant Anal. 34, 2441–2460. doi: 10.1081/CSS-120024778
Lajeunesse M. J. (2011). On the meta-analysis of response ratios for studies with correlated and multi-group designs. Ecology 92, 2049–2055. doi: 10.1890/11-0423.1
Lajeunesse M. J. (2013). “Power Statistics for Meta-analysis,” in Handbook of Meta-analysis in Ecology and Evolution (Princeton, New Jersey: Princeton University Press), 348–363.
Lassaletta L., Billen G., Grizzetti B., Anglade J., Garnier J. (2014). 50 year trends in nitrogen use efficiency of world cropping systems: The relationship between yield and nitrogen input to cropland. Environ. Res. Lett. 9, 105011. doi: 10.1088/1748-9326/9/10/105011
LeBauer D. S., Treseder K. K. (2008). Nitrogen limitation of net primary productivity in terrestrial ecosystems is globally distributed. Ecology 89, 371–379. doi: 10.1890/06-2057.1
Mahal N. K., Castellano M. J., Miguez F. E. (2018). Conservation agriculture practices increase potentially mineralizable nitrogen: A meta-analysis. Soil Sci. Soc. America J. 82, 1270–1278. doi: 10.2136/sssaj2017.07.0245
Mooshammer M., Wanek W., Hammerle I., Fuchslueger L., Hofhansl F., Knoltsch A., et al. (2014a). Adjustment of microbial nitrogen use efficiency to carbon:nitrogen imbalances regulates soil nitrogen cycling. Nat. Commun. 5, 3694. doi: 10.1038/ncomms4694
Mooshammer M., Wanek W., Zechmeister-Boltenstern S., Richter A. A. (2014b). Stoichiometric imbalances between terrestrial decomposer communities and their resources: mechanisms and implications of microbial adaptations to their resources. Front. Microbiol. 5. doi: 10.3389/fmicb.2014.00022
Murphy D. V., Recous S., Stockdale E. A., Fillery I. R. P., Jensen L. S., Hatch D. J., et al. (2003). Gross nitrogen fluxes in soil: theory, measurement, and application of 15N pool dilution techniques. Adv. Agron. 79, 69–118. doi: 10.1016/S0065-2113(02)79002-0
Murphy D. V., Stockdale E. A., Poulton P. R., Willison T. W., Goulding K. W. T. (2007). Seasonal dynamics of carbon and nitrogen pools and fluxes under continuous arable and ley-arable rotations in a temperate environment. Eur. J. Soil Sci. 58 (6), 1410–1424. doi: 10.1111/j.1365-2389.2007.00946.x
Myrold D. D., Bottomley P. J. (2015). “Nitrogen Mineralization and Immobilization,” in Agronomy Monographs. Eds. Schepers J. S., Raun W. R. (American Society of Agronomy, Crop Science Society of America, Soil Science Society of America, Madison, WI, USA), 157–172. doi: 10.2134/agronmonogr49.c5
Nakagawa S., Santos E. S. A. (2012). Methodological issues and advances in biological meta-analysis. Evol. Ecol. 26, 12553–1274. doi: 10.1007/s10682-012-9555-5
Näsholm T., Huss-Danell K., Högberg P. (2000). Uptake of organic nitrogen in the field by four agriculturally important plant species. Ecology 81, 1155–1161. doi: 10.1890/0012-9658
Naz A. A., Reinert S., Bostanci C., Seperi B., Leon J., Böttger C., et al. (2017). Mining the global diversity for bioenergy traits of barley straw: genomewide association study under varying plant water status. GCB Bioenergy 9, 1356–1369. doi: 10.1111/gcbb.12433
Nguyen T. T., Cavagnaro T. R., Thanh Ngo H. T., Marschner P. (2016). Soil respiration, microbial biomass and nutrient availability in soil amended with high and low C:N residue – Influence of interval between residue additions. Soil Biol. Biochem. 95, 189–197. doi: 10.1016/j.soilbio.2015.12.020
Osterholz W. R., Rinot O., Shaviv A., Linker R., Liebman M., Sanford G., et al. (2017). Predicting gross nitrogen mineralization and potentially mineralizable nitrogen using soil organic matter properties. Soil Sci. Soc. America J. 81, 1115–1126. doi: 10.2136/sssaj2017.02.0055
Papavizas G. C., Davey C. B., Woodard R. S. (1962). Comparative effectiveness of some organic amendments and fungicides in reducing activity and survival of rhizoctonia solani in soil. Can. J. Microbiol. 8, 915–922. doi: 10.1139/m62-119
Paustian K., Lehmann J., Ogle S., Reay D., Robertson G. P., Smith P. (2016). Climate-smart soils. Nature 532, 49–57. doi: 10.1038/nature17174
R Core Team (2020). R: a language and environment for statistical computing. R Foundation for Statistical Computing, Vienna, Austria. Available online at: https://www.R-project.org/.
Recous S., Aita C., Mary B. (1998). In situ changes in gross n transformations in bare soil after addition of straw. Soil Biol. Biochem. 31 (1), 119–133. doi: 10.1016/S0038-0717(98)00113-8
Reichel R., Kamau C. W., Kumar A., Li Z., Radl V., Temperton V. M., et al. (2022). Spring barley performance benefits from simultaneous shallow straw incorporation and top dressing as revealed by rhizotrons with resealable sampling ports. Biol. Fertil Soils 58, 375–388. doi: 10.1007/s00374-022-01624-1
Robertson G. P., Groffman P. M. (2024). “Nitrogen transformations,” in Soil Microbiology, Ecology and Biochemistry (Amsterdam, Netherlands: Elsevier), 407–438. doi: 10.1016/B978-0-12-822941-5.00014-4
Robertson G. P., Vitousek P. M. (2009). Nitrogen in agriculture: balancing the cost of an essential resource. Annu. Rev. Environ. Resour. 34, 97–125. doi: 10.1146/annurev.environ.032108.105046
Rohatgi A. (2020). WebPlotDigitizer version 5.2. Available online at: https://automeris.io
Schimel D. S. (1986). Carbon and nitrogen turnover in adjacent grassland and cropland ecosystems. Biogeochemistry 2, 345–357. doi: 10.1007/BF02180325
Schimel J. P., Bennett J. (2004). Nitrogen mineralization: challenges of a changing paradigm. Ecology 85, 591–602. doi: 10.1890/03-8002
Schloerke B., Cook D., Larmarange J., Briatte F., Marbach F., Thoen E., et al. (2024). GGally: extension to “ggplot2”. R package version 2.2.1. Available online at: https://ggobi.github.io/ggally/.
Shi W., Norton J. M. (2000). Microbial control of nitrate concentrations in an agricultural soil treated with dairy waste compost or ammonium fertilizer. Soil Biol. Biochem. 32 (10), 1453–1457. doi: 10.1016/S0038-0717(00)00050-X
Singh B. P., Rengel Z., Bowden J. W. (2006). Carbon, nitrogen and sulphur cycling following incorporation of canola residue of different sizes into a nutrient-poor sandy soil. Soil Biol. Biochem. 38, 32–42. doi: 10.1016/j.soilbio.2005.03.025
Snapp S. S., Fortuna A. M. (2003). Predicting nitrogen availability in irrigated potato systems. HortTechnology 13, 598–604. doi: 10.21273/horttech.13.4.0598
Soldatova E., Krasilnikov S., Kuzyakov Y. (2024). Soil organic matter turnover: Global implications from δ13C and δ15N signatures. Sci. Total Environ. 912, 169423. doi: 10.1016/j.scitotenv.2023.169423
Stella T., Mouratiadou I., Gaiser T., Berg-Mohnicke M., Wallor E., Ewert F., et al. (2019). Estimating the contribution of crop residues to soil organic carbon conservation. Environ. Res. Lett. 14, 094008. doi: 10.1088/1748-9326/ab395c
Thomsen I. K., Sørensen P. (2006). Tillage-induced N mineralization and N uptake in winter wheat on a coarse sandy loam. Soil Tillage Res. 89, 58–69. doi: 10.1016/j.still.2005.06.011
Tiemann L. K., Grandy A. S., Atkinson E. E., Marin-Spiotta E., Mcdaniel M. D. (2015). Crop rotational diversity enhances belowground communities and functions in an agroecosystem. Ecol. Lett. 18, 761–771. doi: 10.1111/ele.12453
Trinsoutrot I., Recous S., Bentz B., Lineres M., Cheneby D., Nicolardot B., et al. (2000). Biochemical quality of crop residues and carbon and nitrogen mineralization kinetics under nonlimiting nitrogen conditions. Soil Sci. Soc. America J. 64, 918–926. doi: 10.2136/sssaj2000.643918x
Vanlauwe B., Giller K. (2006). Popular myths around soil fertility management in sub-Saharan Africa. Agriculture Ecosyst. Environ. 116, 34–46. doi: 10.1016/j.agee.2006.03.016
Viechtbauer W. (2010). Conducting meta-analysis in R with the metafor package. J. Stat. Software 36, 1–48. doi: 10.18637/jss.v036.i03.
Vitousek P. M., Howarth R. W. (1991). Nitrogen limitation on land and in the sea : how can it occur? 13, 87–115. doi: 10.1007/BF00002772
Vogel C., Heister K., Buegger F., Tanuwidjaja I., Haug S., Schloter M., et al. (2015). Clay mineral composition modifies decomposition and sequestration of organic carbon and nitrogen in fine soil fractions. Biol. Fertil Soils 51, 427–442. doi: 10.1007/s00374-014-0987-7
Wang H., Boutton T. W., Xu W., Hu G., Jiang P., Bai E. (2015). Quality of fresh organic matter affects priming of soil organic matter and substrate utilization patterns of microbes. Sci. Rep. 5, 10102. doi: 10.1038/srep10102
Wang J., Wu L., Xiao Q., Huang Y., Liu K., et al. (2023). Long-term manuring enhances soil gross nitrogen mineralization and ammonium immobilization in subtropical area. Agriculture Ecosyst. Environ. 348, 108439. doi: 10.1016/j.agee.2023.108439
Watkins N., Barraclough D. (1996). Gross rates of n mineralization associated with the decomposition of plant residues. Soil Biol. Biochem. 28 (2), 169–175. doi: 10.1016/0038-0717(95)00123-9
Wickham H. (2016). ggplot2: elegant graphics for data analysis. Springer-Verlag New York. Available online at: https://ggplot2.tidyverse.org.
Wu X., Yao W., Zhu J., Miller C. (2010). Biogas and CH4 productivity by co-digesting swine manure with three crop residues as an external carbon source. Bioresource Technol. 101, 4042–4047. doi: 10.1016/j.biortech.2010.01.052
Xu G., Fan X., Miller A. J. (2012). Plant nitrogen assimilation and use efficiency. Annu. Rev. Plant Biol. 63, 153–182. doi: 10.1146/annurev-arplant-042811-105532
Yan M., Pan G., Lavallee J. M., Conant R. T. (2019). Rethinking sources of nitrogen to cereal crops. Global Change Biol. 1–9, 191–199. doi: 10.1111/gcb.14908
Zhang H., Lopez P. C., Holland C., Lunde A., Ambye-Jensen M., Felby C., et al. (2018). The multi-feedstock biorefinery – Assessing the compatibility of alternative feedstocks in a 2G wheat straw biorefinery process. GCB Bioenergy 10, 946–959. doi: 10.1111/gcbb.12557
Keywords: gross nitrogen transformations, gross nitrogen mineralization, gross nitrogen immobilization, 15N, isotope pool dilution, organic amendment, synthetic amendment, meta-analysis
Citation: Breza LC and Grandy AS (2025) Organic amendments tighten nitrogen cycling in agricultural soils: a meta-analysis on gross nitrogen flux. Front. Agron. 7:1472749. doi: 10.3389/fagro.2025.1472749
Received: 30 July 2024; Accepted: 03 January 2025;
Published: 10 February 2025.
Edited by:
Joel Reyes-Cabrera, Texas A&M University Kingsville, United StatesReviewed by:
Savita Singh, Babu Shivnath Agrawal College, IndiaNatasja Van Gestel, Texas Tech University, United States
Copyright © 2025 Breza and Grandy. This is an open-access article distributed under the terms of the Creative Commons Attribution License (CC BY). The use, distribution or reproduction in other forums is permitted, provided the original author(s) and the copyright owner(s) are credited and that the original publication in this journal is cited, in accordance with accepted academic practice. No use, distribution or reproduction is permitted which does not comply with these terms.
*Correspondence: Lauren C. Breza, bGF1cmVuLmJyZXphQHVzZGEuZ292