- 1Department of Biotechnology, Indira Gandhi National Tribal University, Amarkantak, India
- 2Department of Physics, School of Advanced Sciences, Vellore Institute of Technology, Vellore, India
Alkaline stress imposes significant constraints on agriculture by reducing nutrient availability and inhibiting plant growth. This study examines the physiological and biochemical responses of chickpea (Cicer arietinum L.) seedlings to alkaline stress, with implications for improving crop resilience. Chickpea seedlings were subjected to combined Na₂CO₃ and NaHCO₃ treatments, and changes in growth, root morphology, and nutrient uptake were evaluated. Alkaline stress led to substantial reductions in growth metrics (shoot and root length, fresh and dry weights), root-to-shoot ratio, and lateral root number, indicating pronounced root damage. This damage was associated with elevated hydrogen peroxide (H₂O₂) levels, increased membrane damage, and reduced cell viability. In response to alkaline stress, chickpea roots accumulated osmolytes (proline, soluble sugars) and upregulated antioxidant enzymes (catalase, ascorbate peroxidase) as an adaptive response to mitigate osmotic and oxidative stress. Ion homeostasis was disrupted, with decreased uptake of essential nutrients like K, P, Mn, Fe, and Zn, while the uptake of Na, Mg, and Ca increased, disturbing nutrient balance. These findings underscore the need for strategies, such as genetic improvement to enhance alkaline stress tolerance in chickpea, contributing to improved crop performance in challenging soil conditions.
Introduction
By 2050, the global population is expected to reach approximately 9.1 billion, necessitating a significant increase in food production. Estimates indicate that food production must rise by 70% compared to 2009 levels to adequately meet the needs of this growing population (FAO, 2009). Rising temperatures, changing precipitation patterns, and extreme weather events caused by climate change can negatively impact crop yields and food production (Rosenzweig et al., 2001). In addition to these climate-driven challenges, abiotic stress in the form of salinity and alkalinity further limit plant growth and productivity worldwide (Fang et al., 2021). Salinity stress primarily results from the presence of neutral salts, such as sodium chloride (NaCl) and sodium sulfate (Na2SO4), while alkalinity stress stems from the presence of alkaline salts, including sodium bicarbonate (NaHCO3) and sodium carbonate (Na2CO3) (Liu et al., 2022). Though both forms of stress disrupt plant growth through distinct mechanisms, alkalinity has been shown to have a more detrimental impact on plant growth compared to salinity (Paz et al., 2012; Gong et al., 2014; Fang et al., 2021; Liu et al., 2022).
Alkalinity serves as the primary constraint on cropping activity, as the cultivated area of alkaline soils (37%) surpasses that of saline soils (23%) (Paz et al., 2012). Alkaline soils often associated with arid and semi-arid regions are found in various parts of the world, affecting agricultural productivity. Soils rich in carbonates are naturally alkaline, and such soils limit the growth of calcifuge plants. Dicotyledonous plants like peas, beans, or sunflowers face more pronounced root growth inhibition from HCO3− compared to monocotyledonous plants like barley and oats (Poschenrieder et al., 2018). Alkaline stress can lead to numerous detrimental impacts on plant growth, including seed germination, morphological development, and organ formation, ultimately resulting in a reduction in crop yield (Ma et al., 2023). Alkaline stress obstructs plant growth and development by causing ionic and osmotic stresses along with oxidative stress due to increased production of reactive oxygen species (ROS), which disrupt the physiological and biochemical metabolism of plants (Wang et al., 2022a). Moreover, alkaline stress significantly reduces photosynthesis rates and pigment levels, exerting a more severe impact on photosynthesis than salinity stress, which in turn leads to diminished biomass accumulation and crop yield, as photosynthesis directly influences both processes (Yang et al., 2009). Reactive oxygen species (ROS) arise as byproducts of normal metabolism, and high concentrations of ROS can damage cellular components and membranes. Abiotic stresses like heat, drought, and salinity increase ROS production, causing oxidative stress (Dubey et al., 2022). Similarly, alkaline stress triggers excessive buildup of reactive oxygen species (ROS), including hydrogen peroxide (H₂O₂) and superoxide radicals, which disrupt cellular processes and lead to oxidative damage, notably through lipid peroxidation of cellular membranes in plant tissues (Liu et al., 2022). This oxidative damage is evidenced by the accumulation of malondialdehyde (MDA), a byproduct of lipid peroxidation, which serves as a reliable indicator of membrane damage under stress conditions, as observed in alfalfa under alkaline stress (Song et al., 2017). To mitigate these effects, plants activate key antioxidant enzymes, such as catalase (CAT) and ascorbate peroxidase (APX). These enzymes play crucial roles in detoxifying excess H₂O₂ and safeguarding cellular components from oxidative damage. CAT rapidly converts H₂O₂ into water and oxygen in peroxisomes, while APX, with a higher affinity for H₂O₂, functions across various cellular compartments, utilizing ascorbate to reduce H₂O₂ levels (Sofo et al., 2015). In addition to enzyme-mediated ROS scavenging, plants adapt to alkaline stress by accumulating osmolytes, including proline and soluble sugars, which contribute to osmotic balance and ROS mitigation and maintain cellular functions (Gong et al., 2014; Hou et al., 2023). For example, in wheat, salt-alkali stress led to increased levels of proline and soluble sugars to counteract salt-alkaline conditions (Lin et al., 2012), Similarly, in transgenic rice seedlings, tolerance to alkaline stress has been linked to significantly higher accumulation of proline and soluble sugars compared to control plants (Feng et al., 2024).
Roots, as the primary organs responsible for water and nutrient uptake, also play a crucial role in stress perception and response, including under alkaline conditions. Alkaline stress has been shown to have a significant effect on plant roots through the induction of oxidative stress, causing impairment of root activity and the death of root cells (Guo et al., 2017; Zhang et al., 2017). Additionally, alkaline stress reduces root surface area, total root length, and root volume, limiting the plant’s capacity to absorb water and nutrients effectively. For example, in sensitive rice cultivars, alkaline conditions caused substantial reductions in root biomass, surface area, and tip numbers, all of which are critical for efficient water and nutrient uptake (Zhang et al., 2017; Fang et al., 2021). Elevated levels of alkaline salts reduce potassium (K⁺) content by promoting sodium (Na⁺) uptake, which leads to K⁺ efflux from plant cells and disrupts Na⁺/K⁺ homeostasis, impairing essential cellular functions (Wakeel, 2013). Additionally, in the rhizosphere, a high pH environment causes the precipitation of many nutrient ions, hindering their availability and uptake, thus disturbing ion homeostasis (Guo et al., 2010, Guo et al., 2022).
Chickpea (Cicer arietinum L.), the third most important pulse crop globally, is cultivated on approximately 12 million hectares, primarily in arid and semi-arid regions, where it is exposed to various abiotic stresses. While the effects of salinity on chickpea growth, physiology, and ion balance have been extensively studied (Flowers et al., 2010), the impact of alkaline stress remains underexplored. Unlike salinity, which primarily causes ion toxicity, alkaline stress involves high pH levels that hinder the uptake of essential nutrients and affect sodium (Na⁺) exclusion (Zhang et al., 2023). The combined effects of ion toxicity and elevated pH make alkaline stress particularly harmful to plant growth, significantly disrupting ion balance, especially Na⁺ and K⁺ homeostasis. In this study, chickpea seedlings were subjected to alkaline stress using a mixture of NaHCO₃ and Na₂CO₃, and parameters such as root growth, biochemical changes, and ion concentrations were analyzed to elucidate the mechanisms behind alkaline stress-induced damage and the adaptive responses of chickpea plants.
Materials and methods
Plant material and growth conditions
We selected healthy seeds of chickpea (RVG 203) (kindly provided by KVK, IGNTU) and surface-sterilized them using 70% ethanol for 1 minute, followed by 0.5% sodium hypochlorite for 1 minute. Subsequently, the seeds were washed with sterile Milli-Q water 5-6 times. Afterward, the seeds were incubated in sterile Milli-Q water for six hours and then placed on wet filter paper for germination in the dark at 28°C.
After two days, twenty uniformly germinated seedlings were selected and transferred to the control and alkaline stress medium separately. They were allowed to grow for 10 days in a controlled growth chamber at 28°C with a 12-hour photoperiod. After ten days of treatment, the seedlings were analyzed for growth parameters, physiological characteristics, and biochemical responses. All experiments were carried out thrice, with each experiment comprising three biological replicates.
Alkaline stress treatment
Alkaline stress was simulated by mixing sodium bicarbonate (NaHCO3) and sodium carbonate (Na2CO3) at a molar ratio of 9:1 and applying a concentration of 20 mM of the mixture to 0.5X Hoagland’s solution (pH 9.1, EC 2650 μs/cm). This concentration was determined after assessing plant growth inhibition across a range of concentrations from 0 to 40 mM, selecting the lowest concentration (20 mM) at which significant root damage and inhibition of plant growth were observed during the 10-day experimental period. The control treatment involved irrigating plants with 0.5X Hoagland’s solution (pH 6.5, EC 1200 μs/cm) without the addition of NaHCO3 and Na2CO3. The pH and EC of the hydroponic solutions were monitored daily, and the media was replaced with fresh media every two days.
Measuring seedling growth
After 10 days of growth in both control and alkaline stress conditions, the seedlings were gently removed from the growth medium, and their shoot length, root length, later root number, fresh weight of both shoot and root were measured. Subsequently, after drying in an oven, the dry weight of the seedlings was determined. Root-shoot ratio was calculated as the ratio of root DW to shoot DW.
Measurement of relative water content
Relative water content was determined following the method described by Dubey et al. (2022). Initially, the fresh weight (FW) of the seedlings was recorded. The seedlings were then placed in plastic bags, which were sealed after adding water to submerge them. These bags were kept at room temperature for 4 hours. Afterward, the seedlings were blot dried with paper towels to remove excess moisture, and the turgid weight (TW) was measured. Subsequently, the seedlings were dried in a hot air oven at 60°C for 4 days, and the dry weight (DW) was recorded. The relative water content (RWC) was calculated using the equation: RWC (%) = (FW – DW)/(TW – DW) x 100
Determination of root parameters
Root parameters, including total volume, total surface area, primary root volume, primary root surface area, lateral root volume, lateral root surface area, number of root tips, and number of branch points, in both control and treated plants, were determined using RhizoVision Explorer (Seethepalli et al., 2021).
Determination of H2O2 content
Hydrogen peroxide (H2O2) content was measured as follows: Root samples (500 mg) were homogenized on ice in 5 ml of 0.1% (w/v) trichloroacetic acid (TCA). The homogenate was centrifuged at 12,000 rpm for 15 minutes. The reaction mixture included 0.5 ml of 10 mM potassium phosphate buffer (pH 7.0), 1 ml of 1 M potassium iodide, and 0.5 ml of the supernatant. This mixture was incubated in the dark for one hour. Absorbance was then measured at 390 nm using a spectrophotometer. H2O2 content was determined using a standard curve and expressed as micromoles per gram of fresh weight (Dubey et al., 2022).
Estimation of lipid peroxidation
Lipid peroxidation in both control and alkaline-treated plants was assessed by measuring the concentration of malondialdehyde (MDA) in the root tissue of randomly selected plants from each group. MDA determination was performed following the method outlined by Heath and Packer (1968). Briefly, 200 mg of root tissue was homogenized in 2 ml of 0.1% TCA. The homogenate was then centrifuged at 10,000 g for 20 minutes. Subsequently, 0.5 ml of the supernatant was added to a reaction mixture containing 4 ml of 0.5% TBA in 20% TCA. The reaction mixture was heated in a water bath at 95°C for 30 minutes and then cooled immediately on ice. After cooling, the mixture was centrifuged at 10,000 g for 10 min. The absorbance was measured at 532 nm and 600 nm for the supernatant. The amount of MDA was estimated by using an extinction coefficient of 155mM-1cm-1.
Proline estimation
Proline content was quantified using a colorimetric method adapted from Abraham et al. (2010). Briefly, about 100 mg of root tissue was homogenized in 3% sulfosalicylic acid. The homogenate was centrifuged at 12,000 g for 5 minutes at room temperature. A 100 μL aliquot of the supernatant was mixed with 100 μL of 3% sulfosalicylic acid, 200 μL of glacial acetic acid, and 200 μL of acidic ninhydrin. This mixture was incubated at 96°C for 60 minutes and then rapidly cooled on ice to stop the reaction. The samples were extracted with toluene, and the absorbance was measured at 520 nm using toluene as a reference. Concentration of proline was calculated using a standard curve.
Estimation of total soluble sugar
Total soluble sugar content was determined following the protocol of Irigoyen et al. (1992). Freshly harvested root tissue (200 mg) was homogenized in 2 ml of 95% ethanol. The homogenate was centrifuged at 3500 g for 10 minutes. The alcoholic supernatant (0.1 ml) was mixed with 3 ml of freshly prepared anthrone solution (150 mg anthrone dissolved in 100 ml of 72% H2SO4) and heated in a boiling water bath for 10 minutes. The absorbance was measured at 625 nm after cooling. Total soluble sugar content was quantified using a glucose standard curve.
Membrane injury
To assess membrane integrity/injury, plant samples were randomly selected from both the control and treatment groups. Root samples were thoroughly washed with deionized water to eliminate any surface-adhered electrolytes. Root (1gm) samples were placed in individual 50-ml Falcon tubes containing 20 ml of deionized water. These tubes were then maintained at a constant temperature of 25°C for a duration of 1 hour. After this incubation period, the electrical conductivity (EC) was measured (referred to as R1). Following the initial measurement, the tissue samples were subjected to boiling in a water bath for 40 minutes. After boiling, the samples were allowed to cool to 25°C, and the EC was measured once again (referred to as R2). The Membrane Injury (MI) was subsequently calculated using the following formula: MI (%) = (R1/R2) × 100.
Root cell viability assay
Evans blue staining and quantification was performed as previously described (Kumar and Kirti, 2012). Samples were immersed in a 0.25% (wt/vol) solution of Evans blue under continuous agitation. Following staining, the root segments were thoroughly rinsed multiple times with Milli-Q water to eliminate any excess and unbound stain. For quantifying Evan’s Blue levels, the stained samples were ground in a 1% SDS solution and then centrifuged at 12,000 g for 10 minutes. The supernatant was subsequently collected, and its optical density (OD) was measured at 600 nm. The obtained OD values were then used to calculate the concentration of Evans blue in micrograms per gram of fresh weight (μg g-1 FW), utilizing a standard curve constructed with known Evans blue concentrations.
Estimation of catalase and ascorbate peroxidase enzyme activity
Catalase (CAT) and ascorbate peroxidase (APX) activities were measured according to the methods of Elavarthi and Martin (2010), as detailed by Dubey et al. (2022). Briefly, CAT activity was assessed by monitoring the decrease in absorbance at 240 nm as H2O2 decomposed, while APX activity was determined by measuring the reduction in absorbance of ascorbate at 290 nm.
Measurement of ion content
The quantification of ion content was performed utilizing inductively coupled plasma-mass spectrometry (ICP-MS) at the Central Research Facility, Indian Institute of Technology Delhi. Root samples, each weighing 200 mg, previously dried and ground, were subjected to digestion with 8 ml concentrated HNO3 at 200°C and 60 bar for a duration of 30 minutes within a Microwave Reaction System (manufactured by Anton Paar, model: Multiwave PRO). Subsequent to digestion, sample volume was adjusted to 40 ml with deionized water, and the resulting solution underwent filtration utilizing a 0.2-µm membrane. Elemental analysis was conducted using an ICP-MS instrument (Model: 7900, manufactured by Agilent Technologies), calibrated according to the manufacturer’s instructions using blank and multielement standards.
Data and statistical analysis
The data was analyzed using one-way analysis of variance (ANOVA), followed by means comparison using Tukey and Bonferroni tests to determine significance levels. Statistical analyses were performed using OriginPro 2021 software. Principal component analysis (PCA) of the ionome was performed using R software.
Results and discussion
Effect of alkaline stress on growth of chickpea seedlings
Alkaline stress adversely affects crop growth and yield, yet its impact on chickpea (Cicer arietinum L.), an economically and nutritionally vital legume, remains underexplored. Previous studies have shown detrimental effects of alkaline conditions on both monocot and dicot crops (Gong et al., 2014; Zhang et al., 2017). Alkaline stress significantly impaired chickpea seedling growth, causing notable decreases in shoot length, root length, shoot fresh weight, root fresh weight, shoot dry weight, root dry weight, and seedling relative water content (RWC) (Figures 1A–H). Alkaline stress reduced root length by 48% and shoot length by 30%. Root fresh weight and dry weight decreased by 62% and 45%, respectively, while shoot fresh weight and dry weight decreased by 45% and 31%. Growth reduction was more pronounced in the roots, as indicated by the substantial reduction in root length and biomass. Further, analysis of the root-shoot ratio, revealed a reduction under alkaline stress, indicating root injury (Figure 1I). Additionally, the total number of lateral roots was reduced by 66% (Figure 1J). Similar reductions in growth parameters under alkaline stress have been reported in other plant species, indicating a common response across diverse crops (Guo et al., 2010; Wang et al., 2022b; Ma et al., 2023). The findings underscore the detrimental effects of alkaline stress on chickpea seedlings. The substantial reduction in root length, biomass and number of lateral roots indicates adverse effect on the root system compromising water and nutrient acquisition and reducing overall biomass.
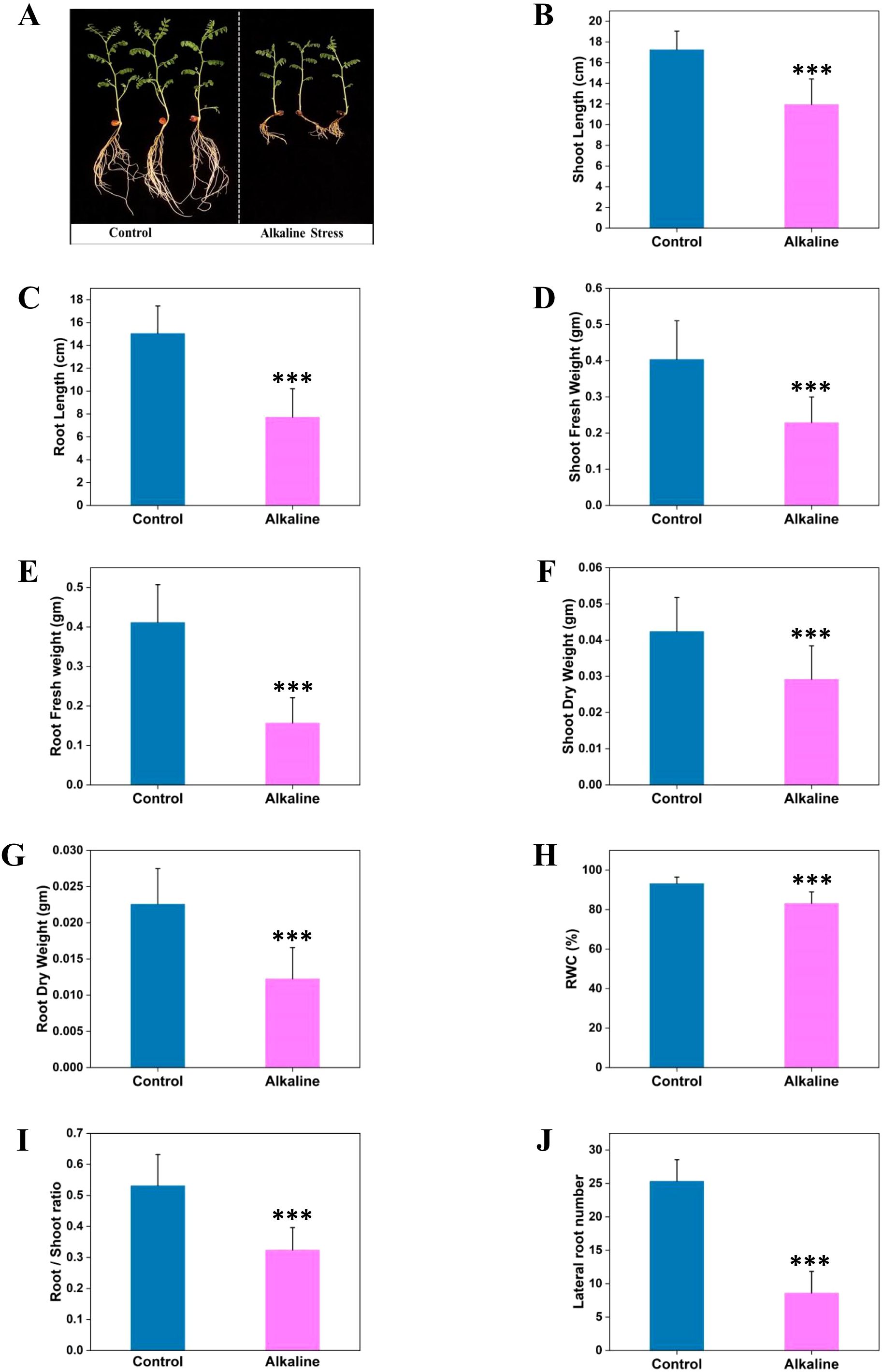
Figure 1. Impact of alkaline stress on chickpea seedling growth. (A) Representative images of ten-day-old chickpea seedlings grown under control and alkaline conditions. Growth parameters are quantified, including: shoot length (B), root length (C), shoot fresh weight (D), root fresh weight (E), shoot dry weight (F), root dry weight (G), relative water content (H), root-to-shoot ratio (I), and total lateral root number (J). Data are presented as mean values with error bars indicating ± SD. Asterisks denote statistically significant differences (***P < 0.001).
Impact of alkaline stress on root morphology and root traits of chickpea seedlings
Root architecture significantly impacts plant productivity, involving variables like topology, root length, and branching, which affect soil resource acquisition and anchorage (Fitter and Stickland, 1991). While salinity’s effects on chickpea root development are known (Flowers et al., 2010; Kaur et al., 2022), there are no studies on the impact of alkalinity on chickpea root morphology, biochemical properties, and ion homeostasis. Given that alkaline stress has a greater impact on roots, we analyzed various root morphological characteristics using RhizoVision software (Seethepalli et al., 2021).
Significant differences were observed in total surface area and total volume of roots between control and alkaline treatment groups (Figures 2A, B). While both primary and lateral root traits were negatively affected by alkaline stress, lateral root traits exhibited a more pronounced reduction (>80%) under alkaline stress (Figures 2E–H). For example, primary root surface area and volume were reduced by 66.19% and 67.46%, respectively, whereas lateral root surface area and volume were reduced by 82.31% and 81.85%, respectively (Figures 2C–F). Furthermore, total root tips and total branch points also showed a significant reduction (>80%) under alkaline stress compared to controls (Figures 2G, H), indicating hindered primary and lateral root growth in chickpea seedlings under alkaline stress.
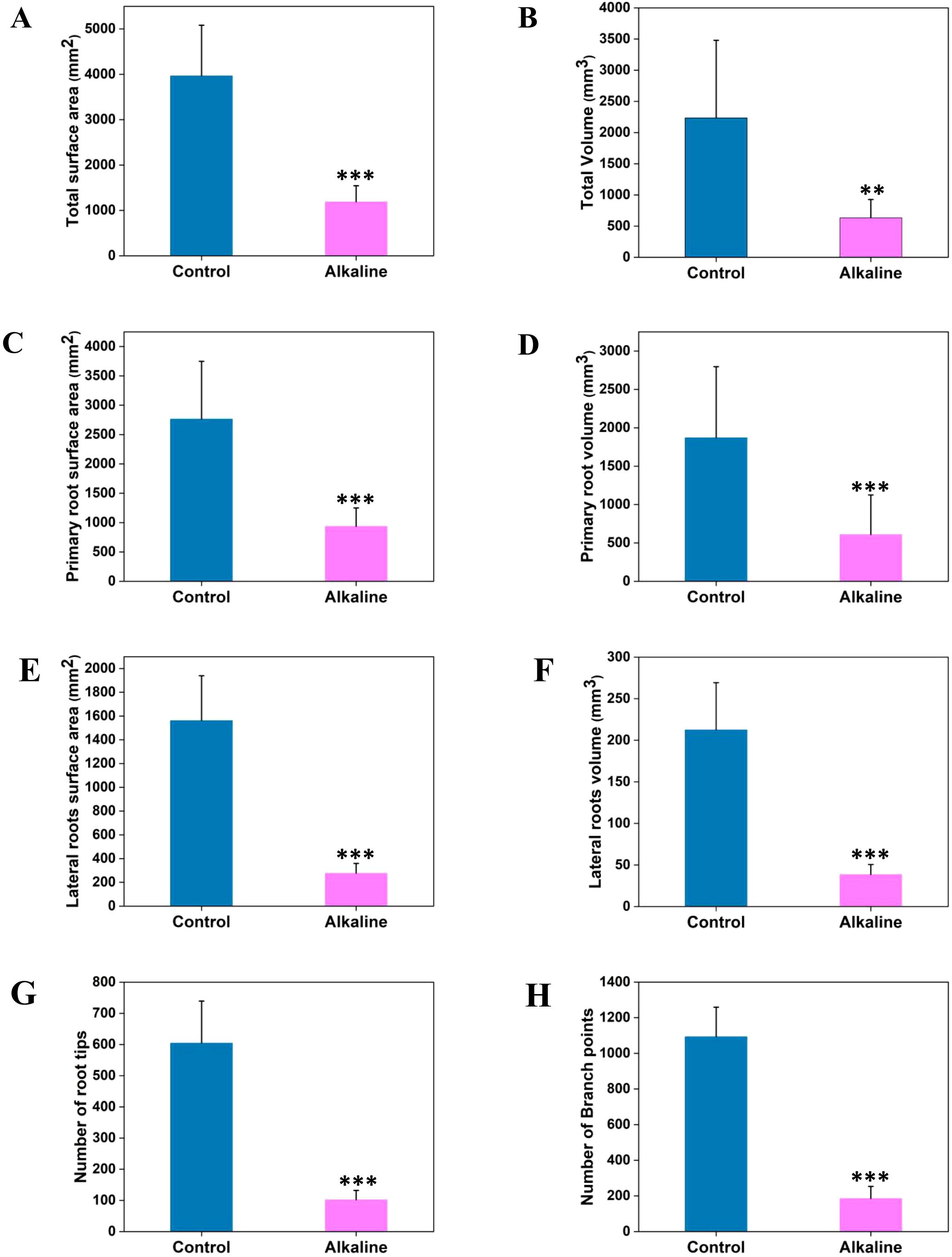
Figure 2. Root trait analysis of chickpea seedlings under control and alkaline stress using RhizoVision Explorer. Root traits of chickpea seedlings were analyzed from greyscale images obtained via RhizoVision Explorer software under control and alkaline conditions. The figure presents quantifications of the following root traits: total surface area (A), total volume (B), primary root surface area (C), primary root volume (D), lateral roots surface area (E), lateral roots volume (F), number of root tips (G), and number of branch points (H). Data are expressed as means ± SD (n = 25). Statistical significance is indicated by asterisks (**P < 0.01, ***P < 0.001).
The observed reduction in root-shoot ratio in chickpea (Figure 1I) indicates compromised root growth relative to shoot growth and root injury. Several studies have reported that alkaline stress restricts root system expansion, thereby reducing the root structure’s capacity for effective nutrient and water uptake (Zhang et al., 2016; Lu et al., 2022; Wang et al., 2022b). In rice, for example, exposure to alkaline conditions significantly reduced root surface area, length, and volume, effects attributed to limited cell expansion and structural damage in root tissues (Lv et al., 2013). Similarly, a notable reduction in lateral branching and root tip density under alkaline stress, compared to control conditions, suggests the loss of crucial adaptive root traits essential for resilience in stressful environments (Benjamin and Nielsen, 2006). The pronounced reduction in root traits over shoots indicates higher root susceptibility to alkaline stress.
Alkaline stress induces membrane damage and decreases root cell viability
Alkaline stress significantly affected the root cell membrane, increasing ion leakage by more than 4-fold (Figure 3A) and MDA concentration by 5-fold (Figure 3B) compared to control plants. These results clearly indicate membrane damage in chickpea roots under alkaline conditions. The observed increase in ion leakage suggests substantial membrane destabilization, likely due to disrupted ion homeostasis and oxidative stress (Zhang et al., 2017). Similarly, the 5-fold increase in MDA concentration indicates heightened lipid peroxidation, reflecting oxidative damage that typically escalates under stress conditions (Song et al., 2017). Root cell viability under alkaline conditions was assessed using Evans blue staining, which revealed a greater than 4-fold increase in dye uptake in stressed roots compared to controls (Figure 3C). The elevated Evans blue uptake in stressed chickpea roots strongly indicates a decline in cell viability, as the dye selectively permeates non-viable cells. This reduced viability reflects cellular death and loss of functional root tissue, which consequently limits root elongation and lateral root formation, as evidenced by the observed reductions in root morphology and branching (Figures 2A–H).
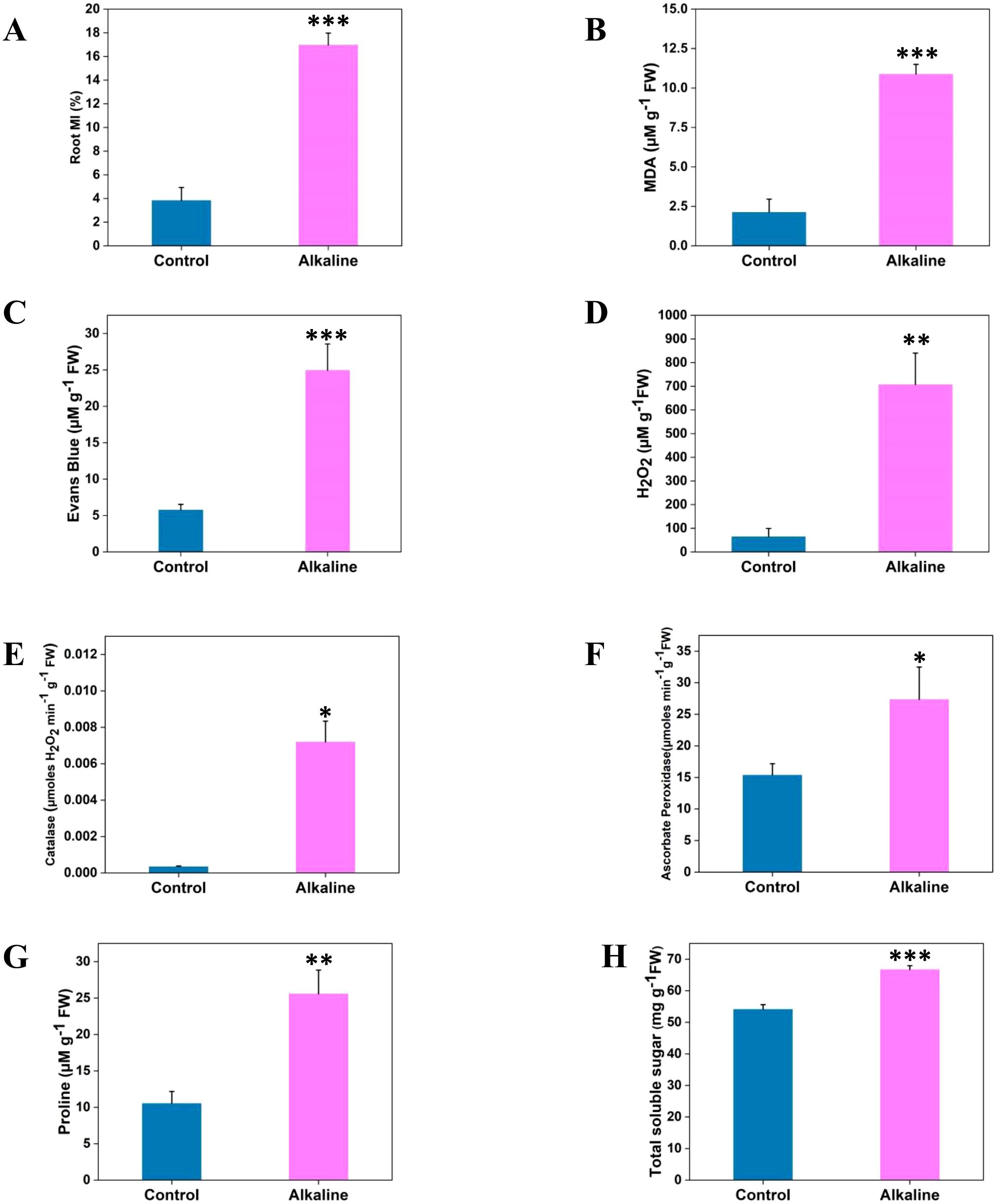
Figure 3. Physiological and biochemical responses of chickpea roots to alkaline stress. The figure presents quantitative analysis of the following physiological and biochemical parameters: (A) Electrolyte leakage, (B) Malondialdehyde (MDA) content, (C) Root cell viability, (D) Hydrogen peroxide (H₂O₂) content, (E) Catalase (CAT) activity, (F) Ascorbate peroxidase (APX) activity, (G) Proline content, and (H) Total soluble sugar content. Data are expressed as means ± SD from experiments conducted in triplicate. Statistical significance between control and alkaline stress conditions is denoted by *P < 0.05, **P < 0.01, ***P < 0.001.
Accumulation of H2O2 and antioxidant enzyme activity in roots under alkaline conditions
To investigate the underlying causes of membrane damage and cell death in chickpea roots under alkaline stress, we examined the primary agents contributing to this damage. Hydrogen peroxide (H₂O₂), a type of reactive oxygen species (ROS), is commonly generated during normal metabolic processes. However, when present at elevated concentrations, H₂O₂ can lead to oxidative damage in cellular components, including membrane lipids (Dubey et al., 2022). Quantification of H₂O₂ in chickpea roots under alkaline conditions revealed that its levels increased several-fold compared to control roots (Figure 3D). Additionally, the enzymatic activities of catalase (CAT) and ascorbate peroxidase (APX), key antioxidants were significantly elevated in stressed roots (Figures 3E, F).
The overaccumulation of H₂O₂ has been identified as a key factor contributing to sensitivity against alkaline stress, as demonstrated in recent studies on the Alkaline Tolerance 1 (AT1) locus. In sorghum and other monocots, functional AT1 negatively regulates the phosphorylation of aquaporins responsible for H₂O₂ transport, leading to its accumulation and increased oxidative damage under alkaline conditions. Knockouts of AT1 in crops such as sorghum, millet, rice, and maize have shown improved alkaline tolerance by reducing H₂O₂ accumulation and mitigating oxidative stress (Zhang et al., 2023).
This oxidative stress triggers upregulation of antioxidant enzymes, such as catalase (CAT) and ascorbate peroxidase (APX), activating innate defense mechanisms to counteract the oxidative stress. Both catalase (CAT), ascorbate peroxidase (APX) are involved in scavenging H2O2 (Dubey et al., 2022). However, while these enzymes mitigate some of the oxidative stress, the observed root damage and elevated H₂O₂ levels suggest that, despite the upregulation of CAT and APX activities, ROS levels remain high. This excess ROS can overwhelm defense mechanisms, leading to oxidative damage manifesting as cellular injury, cell death, and compromised root function under alkaline conditions (Zhang et al., 2017). The balance between ROS production and antioxidant activity ultimately determines the extent of cellular and structural damage under alkaline stress. Potent antioxidants like procyanidins can mitigate root damage and promote growth by reducing ROS accumulation, underscoring the importance of antioxidants in managing oxidative stress (Zhang et al., 2017).
Accumulation of proline and total soluble sugars under alkaline stress
Proline and total soluble sugars (TSS) accumulation is a common adaptive response in plants to osmotic stress, acting as osmoprotectants under stress conditions. Alkaline stress-induced osmotic stress led to increased accumulation of proline and soluble sugars in chickpea roots (Figures 3G, H). Specifically, alkaline-treated roots exhibited a notable increase of 59.53% in proline concentration and 18.8% in TSS concentration compared to controls (Figures 3G, H).
The higher levels of inorganic ions in the soil increases osmotic pressure, leading to osmotic stress and physiological drought in plants (Fang et al., 2021). The enhanced accumulation of proline and total soluble sugars (TSS) in chickpea roots under alkaline stress indicates an osmoprotective strategy to mitigate this stress. In response to Na+ influx, plant roots accumulate proline and soluble sugars, reducing osmotic pressure and improving water retention, uptake and transport (Verma et al., 2016). Similar increases in proline and TSS were reported in broomcorn millet and soybean roots under alkaline stress (Ma et al., 2023; Wang et al., 2022b). This adaptive response is consistent with broader plant mechanisms against abiotic stresses, with increased proline and TSS levels observed in plants under heat and salinity stress (Dubey et al., 2022; Liu et al., 2022).
Alkaline stress disturbs ion balance
Alkaline stress disrupts plant ion balance, leading to increased accumulation of sodium (Na⁺), calcium (Ca²⁺), and magnesium (Mg²⁺) in chickpea roots. Conversely, levels of potassium (K⁺), phosphorus (P), manganese (Mn), iron (Fe), cobalt (Co), zinc (Zn), and molybdenum (Mo) decrease significantly under alkaline stress compared to controls. Boron, nickel, and copper levels show little change between stressed and control roots (Figure 4A). Additionally, the Na+/K+ ratio substantially increases under alkaline stress (Figure 4B). Principal Component Analysis of ionomics data reveals total coefficients of variation for PC1 at 84.72% and PC2 at 10.16% (Figure 4C). Increased alkalinity due to bicarbonate levels reduces iron and potassium concentrations in various plant species, including barley, maize, and sorghum (Alhendawi et al., 1997). Similarly, alkaline stress elevates calcium and magnesium levels in wheat and alfalfa, consistent with our findings in chickpea (Guo et al., 2010; Wang et al., 2017).
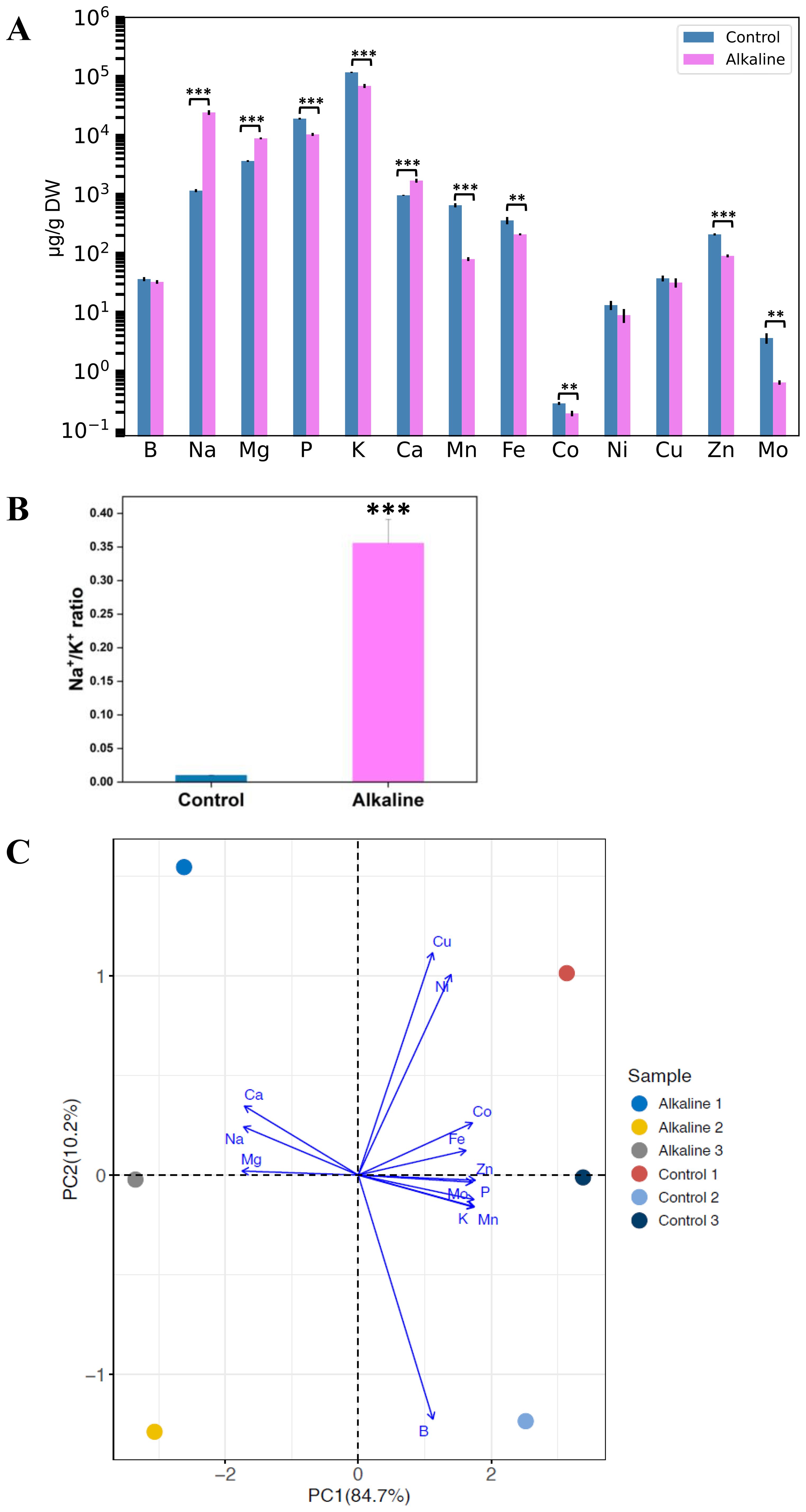
Figure 4. Ion concentration responses of chickpea roots to alkaline stress. (A) Elemental concentrations in chickpea roots under control and alkaline conditions, measured by ICP-MS. The y-axis is presented on a logarithmic scale for clearer visualization across the wide range of concentrations. (B) Na+/K+ ratio under control and alkaline conditions. (C) PCA illustrating differences in ionomic profiles between control and alkaline-treated roots. Data are presented as mean ± SD (n = 3). Significant differences are indicated as ***P < 0.001, **P < 0.01.
Alkaline stress induces osmotic stress and ion toxicity similar to salt stress, further impairing nutrient absorption at high pH levels and leading to nutrient imbalances, metabolic disorders, and disturbances in ion homeostasis (Guo et al., 2015; Lu et al., 2022). Potassium (K⁺) is crucial for enzyme activation, osmotic regulation, and cellular ion balance, supporting overall metabolic stability and numerous physiological functions in plants. Conversely, sodium (Na⁺) becomes toxic at elevated concentrations, disrupting metabolic processes and enzyme activities. Thus, maintaining an optimal Na⁺/K⁺ ratio is essential for plant health. In chickpea roots, alkaline stress significantly increases Na⁺ levels while reducing K⁺ levels, creating an Na⁺/K⁺ imbalance that negatively impacts nutrient absorption and cellular function. This stress-induced elevation in Na⁺ is partly due to competition with K⁺ for uptake pathways, as Na⁺ often replaces K⁺ in non-selective channels and high-affinity transporters, disrupting normal K⁺ absorption. Additionally, alkaline-induced membrane depolarization hinders K⁺ uptake and promotes its efflux, further reducing K⁺ levels in plant tissues (Wakeel, 2013).
Further, alkaline stress negatively affects nodulation in chickpea, evidenced by low nodulation percentages in alkaline soils (Singh et al., 2015). This reduction is attributed to an unfavorable habitat for rhizobia growth and multiplication and nutrient deficiencies. In addition to macronutrients like phosphorus, legume-rhizobia symbiosis requires various micronutrients, including boron, cobalt, copper, iron, manganese, molybdenum, nickel, and zinc, sometimes in higher amounts than required by the plant or bacteria alone (O’Hara, 2001). For instance, molybdenum, crucial for nitrogenase activity, can decrease legume productivity by impacting nodule development and function (O’Hara, 2001). Reduced levels of molybdenum and other micronutrients in chickpea roots in our study suggest that alkaline stress may hinder nodulation by impeding essential nutrient uptake.
Conclusions
This study reveals that alkaline stress significantly inhibits chickpea growth by reducing shoot and root biomass, disrupting root morphology, and impairing nutrient uptake. Alkaline stress also induces oxidative damage in chickpea roots, as evidenced by elevated hydrogen peroxide (H₂O₂) levels and decreased root cell viability. Additionally, alkaline stress disrupts ion balance, leading to increased sodium uptake and reduced uptake of essential nutrients, such as potassium, phosphorus, and iron, which are critical for plant health and productivity. In response to the stress, chickpea plants activate adaptive mechanisms, including the accumulation of osmolytes like proline and soluble sugars and the upregulation of antioxidant enzymes, which help partially mitigate cellular damage. However, the combined effects of osmotic stress, ion toxicity, and metabolic disturbances contribute to overproduction of reactive oxygen species (ROS), ultimately overwhelming the plant’s defense mechanisms and causing root damage.
While this research provides insights into chickpea responses to a combined Na₂CO₃ and NaHCO₃-induced alkaline environment, future studies could benefit from examining the specific effects of each salt to better understand distinct ionic and osmotic stress responses. Additionally, exploring genetic diversity for alkaline stress tolerance in chickpea could facilitate the development of resilient cultivars suited to these challenging soil conditions. Overall, this work contributes to a broader understanding of chickpea stress physiology and underscores the need for innovative approaches to enhance crop resilience against alkaline soils.
Data availability statement
The raw data supporting the conclusions of this article will be made available by the authors, without undue reservation.
Author contributions
KK: Formal analysis, Investigation, Writing – original draft. AJ: Investigation, Writing – original draft. UMKK: Formal analysis, Methodology, Software, Writing – review & editing. KRRK: Conceptualization, Formal analysis, Funding acquisition, Resources, Supervision, Writing – original draft, Writing – review & editing.
Funding
The author(s) declare that no financial support was received for the research, authorship, and/or publication of this article.
Acknowledgments
Chickpea seeds were kindly provided by Krishi Vigyan Kendra, IGNTU, Amarkantak.
Conflict of interest
The authors declare that the research was conducted in the absence of any commercial or financial relationships that could be construed as a potential conflict of interest.
Publisher’s note
All claims expressed in this article are solely those of the authors and do not necessarily represent those of their affiliated organizations, or those of the publisher, the editors and the reviewers. Any product that may be evaluated in this article, or claim that may be made by its manufacturer, is not guaranteed or endorsed by the publisher.
References
Abraham E., Hourton-Cabassa C., Erdei L., Szabados L. (2010). Methods for determination of proline in plants. Methods in Molecular Biology, 317–331. doi: 10.1007/978-1-60761-702-0_20
Alhendawi R. A., Römheld V., Kirkby E. A., Marschner H. (1997). Influence of increasing bicarbonate concentrations on plant growth, organic acid accumulation in roots and iron uptake by barley, sorghum, and maize. J. Plant Nutr. 20, 1731–1753. doi: 10.1080/01904169709365371
Benjamin J., Nielsen D. (2006). Water deficit effects on root distribution of soybean, field pea and chickpea. Field Crops Res. 97, 248–253. doi: 10.1016/J.FCR.2005.10.005
Dubey A., Kumar K., Srinivasan T., Kondreddy A., Kumar K. R. R. (2022). An invasive weed-associated bacteria confers enhanced heat stress tolerance in wheat. Heliyon 8, e09893. doi: 10.1016/j.heliyon.2022.e09893
Elavarthi S., Martin B. (2010). Spectrophotometric assays for antioxidant enzymes in plants. Methods Mol Biol. 639, 273–81. doi: 10.1007/978-1-60761-702-0_16
Fang S., Hou X., Liang X. (2021). Response mechanisms of plants under saline-alkali stress. Front. Plant Sci. 12. doi: 10.3389/fpls.2021.667458
FAO. (2009). How to Feed the World in 2050 (High-Level Expert Forum). Available at: www.fao.org.
Fitter A. H., Stickland T. R. (1991). Architectural analysis of plant root systems 2. Influence of nutrient supply on architecture in contrasting plant species. New Phytol. 118, 383–389. doi: 10.1111/j.1469-8137.1991.tb00019.x
Feng Z., Xu Y., Xie Z., Yang Y., Lu G., Jin Y., et al. (2024). Overexpression of Abscisic Acid Biosynthesis Gene OsNCED3 Enhances Survival Rate and Tolerance to Alkaline Stress in Rice Seedlings. Plants 13. doi: 10.3390/plants13121713
Flowers T. J., Gaur P. M., Gowda C. L. L., Krishnamurthy L., Srinivasan S., Siddique K. H. M., et al. (2010). Salt sensitivity in chickpea. Plant Cell Environ. 33, 490–509. doi: 10.1111/j.1365-3040.2009.02051.x
Gong B., Wen D., Bloszies S., Li X., Wei M., Yang F., et al. (2014). Comparative effects of NaCl and NaHCO3 stresses on respiratory metabolism, antioxidant system, nutritional status, and organic acid metabolism in tomato roots. Acta Physiologiae Plantarum. 36 (8), 2167–2181. doi: 10.1007/s11738-014-1593-x
Guo M., Li S., Tian S., Wang B., Zhao X. (2017). Transcriptome analysis of genes involved in defense against alkaline stress in roots of wild jujube (Ziziphus acidojujuba). PLoS One 12, e0185732. doi: 10.1371/journal.pone.0185732
Guo J., Lu X., Tao Y., Guo H., Wei M. (2022). Comparative ionomics and metabolic responses and adaptive strategies of cotton to salt and alkali stress. Front. Plant Sci. 13. doi: 10.3389/fpls.2022.871387
Guo R., Shi L., Ding X., Hu Y., Tian S., Yan D., et al. (2010). Effects of saline and alkaline stress on germination, seedling growth, and ion balance in wheat. Agron. J. 102, 1252–1260. doi: 10.2134/agronj2010.0022
Guo R., Yang Z., Li F., Yan C., Zhong X., Liu Q., et al. (2015). Comparative metabolic responses and adaptive strategies of wheat (Triticum aestivum) to salt and alkali stress. BMC Plant Biology 15. doi: 10.1186/s12870-015-0546-x
Heath R. L., Packer L. (1968). Photoperoxidation in isolated chloroplasts: I. Kinetics and stoichiometry of fatty acid peroxidation. Arch. Biochem. biophysics 125, 189–198. doi: 10.1016/0003-9861(68)90654-1
Hou R., Yang L., Wuyun T., Chen S., Zhang L. (2023). Genes related to osmoregulation and antioxidation play important roles in the response of Trollius chinensis seedlings to saline-alkali stress. Front. Plant Sci. 14. doi: 10.3389/fpls.2023.1080504
Irigoyen J. J., Einerich D. W., Sánchez-Díaz M. (1992). Water stress induced changes in concentrations of proline and total soluble sugars in nodulated alfalfa (Medicago sativa) plants. Physiologia plantarum 84, 55–60. doi: 10.1111/j.1399-3054.1992.tb08764.x
Kaur G., Sanwal S. K., Sehrawat N., Kumar A., Kumar N., Mann A. (2022). Getting to the roots of Cicer arietinum L. (chickpea) to study the effect of salinity on morpho-physiological, biochemical and molecular traits. Saudi J. Biol. Sci. 29, 103464. doi: 10.1016/j.sjbs.2022.103464
Kumar K. R. R., Kirti P. B. (2012). Novel role for a serine/arginine-rich splicing factor, AdRSZ21 in plant defense and HR-like cell death. Plant Mol. Biol. 80, 461–476. doi: 10.1007/s11103-012-9960-8
Lin J., Li X., Zhang Z., Yu X., Gao Z., Wang Y., et al. (2012). Salinity-alkalinity tolerance in wheat: seed germination, early seedling growth, ion relations and solute accumulation. Afr. J. Agric. Res. 7, 467–474. doi: 10.5897/AJAR11.1417
Liu D., Ma Y., Rui M., Lv X., Chen R., Chen X., et al. (2022). Is High pH the Key Factor of Alkali Stress on Plant Growth and Physiology? A Case Study with Wheat (Triticum aestivum L.) Seedlings. Agronomy 12, 1820. doi: 10.3390/agronomy12081820
Lu X., Min W., Shi Y., Tian L., Li P., Ma T., et al. (2022). Exogenous melatonin alleviates alkaline stress by removing reactive oxygen species and promoting antioxidant defence in rice seedlings. Front. Plant Sci. 13. doi: 10.3389/fpls.2022.849553
Lv B., Xiaowei L., Ma H., Sun Y., Lixing W., Jiang C., et al. (2013). Differences in growth and physiology of rice in response to different saline-alkaline stress factors. Agron. J. 105, 1119–1128. doi: 10.2134/AGRONJ2013.0017
Ma Q., Wang H., Wu E., Yuan Y., Feng Y., Zhao L., et al. (2023). Comprehensive physiological, transcriptomic, and metabolomic analysis of the response of Panicum miliaceum L. roots to alkaline stress. Land Degradation Dev. 34, 2912–2930. doi: 10.1002/ldr.4656
O’Hara G. W. (2001). Nutritional constraints on root nodule bacteria affecting symbiotic nitrogen fixation: a review. Aust. J. Exp. Agric. 41, 417. doi: 10.1071/ea00087
Paz R. C., Rocco R. A., Reinoso H., Menéndez A., Pieckenstain F. L., Ruíz O. A. (2012). Comparative study of alkaline, saline, and mixed saline–alkaline stresses with regard to their effects on growth, nutrient accumulation, and root morphology of lotus tenuis. J. Plant Growth Regulation. 31, 448–459. doi: 10.1007/s00344-011-9254-4
Poschenrieder C., Fernández J. A., Rubio L., Pérez L. M., Terés J., Barceló J. A. (2018). Transport and use of bicarbonate in plants: Current knowledge and challenges ahead. Int. J. Mol. Sci. 19, 1352. doi: 10.3390/ijms19051352
Rosenzweig C., Iglesias A., Yang X., Epstein P., Chivian E. (2001). Climate change and extreme weather events; implications for food production, plant diseases, and pests. Global Change Hum. Health 2, 90–104. doi: 10.1023/A:1015086831467
Seethepalli A., Dhakal K., Griffiths M., Guo H., Freschet G. T., York L. M. (2021). RhizoVision Explorer: open-source software for root image analysis and measurement standardization. AoB Plants 13 (6), plab056. doi: 10.1093/aobpla/plab056
Singh R. P., Manchanda G., Singh R. N., Srivastava A. K., Dubey R. C. (2015). Selection of alkalotolerant and symbiotically efficient chickpea nodulating rhizobia from North-West Indo Gangetic Plains. J. Basic Microbiol. 56, 14–25. doi: 10.1002/jobm.201500267
Sofo A., Scopa A., Nuzzaci M., Vitti A. (2015). Ascorbate peroxidase and catalase activities and their genetic regulation in plants subjected to drought and salinity stresses. Int. J. Mol. Sci. 16, 13561–13578. doi: 10.3390/ijms160613561
Song T., Xu H., Sun N., Jiang L., Tian P., Yong Y., et al. (2017). Metabolomic Analysis of Alfalfa (Medicago sativa L.) Root-Symbiotic Rhizobia Responses under Alkali Stress. Front. Plant Sci. 8. doi: 10.3389/fpls.2017.01208
Verma V., Ravindran P., Kumar P. P. (2016). Plant hormone-mediated regulation of stress responses. BMC Plant Biol. 16, 86. doi: 10.1186/s12870-016-0771-y
Wakeel A. (2013). Potassium-sodium interactions in soil and plant under saline-sodic conditions. J. Plant Nutr. Soil Sci. 176, 344–354. doi: 10.1002/jpln.201200417
Wang N., Fan X., Lin Y., Li Z., Wang Y., Zhou Y., et al. (2022a). Alkaline stress induces different physiological, hormonal and gene expression responses in diploid and autotetraploid rice. Int. J. Mol. Sci. 23, 5561. doi: 10.3390/ijms23105561
Wang X., Ren H., Wei Z., Wang Y., Ren W. (2017). Effects of neutral salt and alkali on ion distributions in the roots, shoots, and leaves of two alfalfa cultivars with differing degrees of salt tolerance. J. Integr. Agriculture/Journal Integr. Agric. 16, 1800–1807. doi: 10.1016/s2095-3119(16)61522-8
Wang G., Shen W., Zhang Z., Guo S., Hu J., Feng R., et al. (2022b). The effect of neutral salt and alkaline stress with the same na+ Concentration on root growth of soybean (Glycine max (L.) merr.) seedlings. Agronomy 12, 2708. doi: 10.3390/agronomy12112708
Yang C.-W., Xu H.-H., Wang L.-L., Liu J., Shi D.-C., Wang D.-L. (2009). Comparative effects of salt-stress and alkali-stress on the growth, photosynthesis, solute accumulation, and ion balance of barley plants. Photosynthetica 47, 79–86. doi: 10.1007/s11099-009-0013-8
Zhang H., Liu X., Zhang R. X., Huang Y., Wang M., Yang H., et al. (2017). Root Damage under Alkaline Stress Is Associated with Reactive Oxygen Species Accumulation in Rice (Oryza sativa L.). Front. Plant Sci. 8. doi: 10.3389/fpls.2017.01580
Zhang H., Yu F., Xie P., Sun S., Qiao X., Tang S., et al. (2023). A Gγ protein regulates alkaline sensitivity in crops. Science 379 (6638), eade8416. doi: 10.1126/science.ade8416
Keywords: alkaline soil, alkaline stress, chickpea, ionomics, legume, oxidative stress, root damage
Citation: Kumar K, Jaiswal A, Koppolu UMK and Kumar KRR (2024) Alkaline stress disrupts growth, biochemistry, and ion homeostasis of chickpea (Cicer arietinum L.) roots. Front. Agron. 6:1497054. doi: 10.3389/fagro.2024.1497054
Received: 16 September 2024; Accepted: 02 December 2024;
Published: 19 December 2024.
Edited by:
Naser A. Anjum, Aligarh Muslim University, IndiaCopyright © 2024 Kumar, Jaiswal, Koppolu and Kumar. This is an open-access article distributed under the terms of the Creative Commons Attribution License (CC BY). The use, distribution or reproduction in other forums is permitted, provided the original author(s) and the copyright owner(s) are credited and that the original publication in this journal is cited, in accordance with accepted academic practice. No use, distribution or reproduction is permitted which does not comply with these terms.
*Correspondence: Koppolu Raja Rajesh Kumar, ay5yYWphcmFqZXNoa3VtYXJAZ21haWwuY29t; a3Jya3VtYXJAaWdudHUuYWMuaW4=
†ORCID: Koppolu Raja Rajesh Kumar, orcid.org/0000-0001-5153-6671