- 1School of Agricultural Technology, King Mongkut’s Institute of Technology Ladkrabang, Bangkok, Thailand
- 2King Mongkut Chaokhunthahan Hospital, King Mongkut’s Institute of Technology Ladkrabang, Bangkok, Thailand
- 3Department of Biochemistry and Molecular Biology, Saitama University, Saitama, Japan
- 4School of Science, King Mongkut’s Institute of Technology Ladkrabang, Bangkok, Thailand
This study investigates the inhibitory effects of Diaporthe sp. isolate EC010 extract on barnyardgrass (Echinochloa crus-galli) seed germination and growth. Application of sequential extraction techniques to Diaporthe sp. mycelium resulted in partial separation of the phytotoxic compounds. The ethyl acetate (EtOAc) fraction most greatly reduced seed germination (81.01%), root length (89.18%), and shoot length (84.74%) compared to the control. Chemical characterization using gas chromatography-mass spectrometry revealed major constituents of linoleic acid, butyl ester (9.69%), hexadecanoic acid (7.99%), and 14-pentadecenoic acid (7.86%). With regard to physiological and biochemical indexes, treated seeds exhibited lower imbibition, significantly decreased α-amylase (EC 3.2.1.1) activity (p<0.05), and increased accumulation of malondialdehyde (85.52%) and hydrogen peroxide (141.10%). Moreover, activity of the antioxidant enzymes superoxide dismutase (EC 1.15.1.1) and guaiacol peroxidase (EC 1.11.1.7) was upregulated (67.24 and 61.62%, respectively), while catalase (EC 1.11.1.6) activity was downregulated (-33.75%). The inference is that an imbalance in ROS levels combined with reduced antioxidant potential drives the gradual accumulation of oxidative damage in seed cells and consequent loss of seed viability. All told, these results confirm the Diaporthe extract to induce oxidative stress and inhibit antioxidant enzymes. This study clearly demonstrates the oxidative damage associated with Diaporthe allelochemicals.
1 Introduction
Herbicides appear to be a cost-effective way to control weeds and contribute to crop productivity. However, the overuse of synthetic herbicides has led to the spread of herbicide-resistant biotypes (Saini and Singh, 2019). This resistance reduces the efficacy of existing herbicides, necessitating the exploration of alternative weed control strategies. Integrated weed management (IWM) approaches, which combine various weed control methods, offer a strategic way to reduce reliance on synthetic herbicides (Nazarkov and Gerasimova, 2023). In this context, natural herbicides derived from allelochemicals are of interest as potential complementary tools in IWM (Saini and Singh, 2019; Gao and Su, 2024). These natural products can be integrated with other weed control methods to achieve broad-spectrum weed control (Bailey, 2014) and, due to their multiple modes of action, can help prevent herbicide resistance (Hazrati et al., 2017). Compared to synthetic options, natural herbicides generally have lower toxicity and shorter environmental persistence (Gao and Su, 2024). By expanding research in this area, the current study aims to explore and discover new sources containing toxic compounds that could enhance weed control, ultimately contributing to the development of effective natural herbicides. Diaporthe is a large fungal genus belonging to the Diaporthales and Ascomycota, one for which new species are continually being reported (Huang et al., 2021; Sun et al., 2021). Notably, Diaporthe fungi are important sources of active natural products, with many reports of terpenes (Luo et al., 2021; Zhang et al., 2021), alkaloids, anthraquinones (Tian et al., 2018; Xu et al., 2021), and other metabolites with unique structures having been identified from this genus. In the past 20 years, more than 200 such compounds have been demonstrated to possess significant antitumor, antibacterial, herbicidal, and other biological activities. Consequently, reduced use of chemical products can be achieved by using natural herbicides containing natural fungal compounds or fungal active components. Eco-friendly herbicides have been developed using these chemicals as potential candidates.
Weed physiology during exposure to allelochemicals has lately become an area of research interest, with studies to date having highlighted impacts to significant metabolic processes such as those related to cell division, nutrient uptake, antioxidants, stress-mediated hormones, and other metabolites that control seed germination and growth (Radhakrishnan et al., 2018). It has been reported that application of herbicides induces the generation of reactive oxygen species (ROS) in plants, including superoxide radicals (O2•−), hydroxyl radicals (OH•), and hydrogen peroxide (H2O2) (Wu et al., 2021; Dmitrieva et al., 2024). This accumulation of ROS can induce oxidative stress, which has adverse consequences for proteins, DNA, and lipids (Choudhary et al., 2020). Especially, ROS action on membrane lipids results in lipid peroxidation, which in turn causes cell membrane damage (Laosinwattana et al., 2018). Accordingly, plants have a mechanism for defending from oxidative stress via the antioxidant enzyme system. Antioxidant enzymes scavenge or eliminate a variety of free radicals, including those generated during biological processes. The main antioxidant enzymes are superoxide dismutase (SOD), catalase (CAT), and guaiacol peroxidases (POD) (Mishra et al., 2023). SOD maintains the cellular levels of O2•− within physiological concentrations by converting O2•− to H2O2. As H2O2 may then oxidize cell membranes and cellular components to OH•, its removal is a crucial concern. CAT is an H2O2 decomposing enzyme that metabolizes H2O2 to O2 and H2O (Fujita and Hasanuzzaman, 2022). POD then provides additional mechanisms for maintaining redox balance and removal of toxic metabolites (Zhu et al., 2023).
In a previous study, we collected 21 fungi from weed-infected tissues and screened them for herbicidal activity. Among them, Diaporthe sp. isolate EC010 (GenBank accession No. OR143425) showed herbicidal effects of its secondary metabolites on target plants, including pre-emergence herbicidal effects in the form of inhibition of Amaranthus tricolor (dicotyledonous weed) seed germination. We hypothesized the Diaporthe extract to induce osmotic stress and thereby restrict both amylase activity, which is required for energy supply to root and shoot growth, and mitotic activity (Manichart et al., 2023a). In this study, we investigated the oxidative damage caused by Diaporthe sp. extract on the monocotyledonous weed barnyardgrass (Echinochloa crus-galli) and studied how treated plants respond via the antioxidant enzyme system. Moreover, we identified the chemical components of the extract.
2 Material and methods
2.1 Fungal culture and isolation of fungal allelochemicals
The fungi used in this study (Diaporthe sp. EC010) were initially collected by Manichart, Laosinwattana (Manichart et al., 2023a) in September 2021 from the Ladkrabang district of Bangkok, Thailand. The isolated fungi were cultured via submerged fermentation in potato dextrose broth medium. After the culture period, the biomass was collected and incubated in a hot air oven (45°C). The obtained mycelia were sequentially extracted using organic solvents with increasing polarity, according to Poonpaiboonpipat, Krumsri (Poonpaiboonpipat et al., 2021). Briefly, the dried mycelia were soaked with hexane for 24 hours, then the resulting mixture was filtered through filter paper, and the remaining residue was re-extracted. Next, the solutions were combined and evaporated to obtain the crude hexane extract fraction. The leftover residue was then extracted with ethyl acetate (EtOAc) and ethanol (EtOH) following the same process, resulting in EtOAc and EtOH extract fractions. The phytotoxicity of all extract fractions was assessed using a seed germination assay.
2.2 Seed germination assay
Firstly, each sticky fungus crude extract was dissolved in the corresponding solvent (hexane, EtOAc, or EtOH) at concentrations of 0.625, 1.25, and 2.50 mg/mL. The concentrations were selected based on preliminary experiments that showed these concentrations affected seed germination. Four replicates were maintained per treatment for each of the organic solvent in a completely randomized design (CRD); treatments with distilled water were used as negative controls. For the germination tests, according to Teerarak, Laosinwattana (Teerarak et al., 2010), 5 mL of each extract solution was added to a Petri dish (9-cm diameter) containing a double layer of germination paper and allowed to evaporate to complete dryness at room temperature. Then, the germination paper in the Petri dish was moistened with 5 mL of distilled water. Twenty healthy seeds of barnyardgrass were placed in each dish. The dishes were kept in a growth chamber (LAC-1075-N, Longyue, Shanghai) with a temperature of 27 ± 2°C, 12/12 h light/dark photoperiod, and relative humidity of about 80%. The germination rate of the grass seeds and the length of seedling roots and shoots (cm) were assessed after seven days of treatment. The optimal crude fraction with the highest inhibitory efficacy on germination and seedling growth was selected for further experiments.
2.3 Chemical characterization via GC-MS
Components of the Diaporthe EtOAc crude extract were identified using GC-MS. The extract was diluted in ethyl acetate before being analyzed on an Agilent 6890 N gas chromatograph equipped with an Agilent 5973 mass detector and an HP-5 silica capillary column (30 m x 0.25 mm ID, 0.25 m film thickness). The column temperature program started at 40°C for 3 minutes, then increased to 100°C at a rate of 10°C/min, and then further to 260°C at a rate of 5°C/min, which was held for 5 minutes. The flow rate was 1 mL/min, with helium used as the carrier gas. The MS analysis was performed with a detection range of 30-500 amu. The sample (0.2 µm) was injected using a 50:1 split ratio. The injector and detector were maintained at respective temperatures of 250°C and 270°C. Individual compounds were identified by comparison of MS profiles to a reference library (National Institute of Standards and Technology, NIST, 2014). A percentage peak area relative to the total peak area was used to express the relative amounts of the various components of the total crude extract. Peaks of less than 0.1% area were excluded, whereas all those comprising more than 0.1% were included.
2.4 Seed imbibition and α-amylase activity
The EtOAc crude extract was evaluated for its effect on seed imbibition. Seed imbibition was carried out according to Turk and Tawaha (2003) with modification. In brief, the original grass seed weight (W1) was recorded, and the seeds were then immersed in the fungal extract for a designated incubation period of 12, 24, or 36 hours. Since barnyardgrass takes approximately 36 hours to complete the germination process (Takao et al., 2011), these time points were selected to monitor the physiological response at each stage of germination. After incubation, the seeds were washed and weighed (W2) again and water absorption values were determined using the formula:
The activity of α-amylase (EC 3.2.1.1) was determined using the 3,5-dinitrosalicylic acid (DNS) test. α-amylase activity was measured as reported by Sadasivam and Manickam (1996). For the enzyme extraction, the treated seeds were grained with 0.1 M CaCl2 (4 mL) in an ice bath, and centrifuged at 9600 × g for 20 min at a temperature of 4°C. This yielded crude enzyme. Subsequently, the reaction was carried out by adding 1.0 mL of crude enzyme to 1.0 mL of 0.5% soluble starch. The mixture was incubated, and then 1.0 mL of DNS reagent was added, after which the reaction solution was immediately placed in a boiling water bath for 5 minutes. Finally, light absorption at 560 nm was measured and used to calculate the α-amylase activity, represented in μmol maltose/min/g (fresh weight, FW).
2.5 Measurement of MDA and H2O2 contents
The thiobarbituric acid reactive substance (TBARS) assay was used to determine lipid peroxidation based on estimating malondialdehyde (MDA) content. The sample (0.5 g FW) was ground with 6.0 mL of 3.0% trichloroacetic acid (TCA) and centrifuged for 20 minutes at 4°C at 9600 × g. The supernatant (1.0 mL) was then mixed with 2.0 mL of 0.5% thiobarbituric acid (TBA) in 20% TCA. This reaction mixture was heated in a boiling water bath for 20 minutes before cooling on ice for 5 minutes (He et al., 2014). Finally, the absorbance was measured at 532 and 600 nm, and an extinction coefficient of 155 m/M·cm was used to calculate the MDA content. Hydrogen peroxide (H2O2) content was calculated following Velikova, Yordanov (Velikova et al., 2000). The treated seeds (0.5 g FW) were homogenized with 6.0 mL of 3.0% TCA and centrifuged (9600 × g) for 20 minutes. Then, 1.0 mL of supernatant was added to 3.0 mL of reaction mixture (10 mM phosphate buffer pH 7.0 and 500 mM potassium iodine). After 15 minutes, the absorbance at 390 nm was measured.
2.6 Extraction and determination of antioxidant enzyme activity
The crude enzyme extract was prepared according to the procedure described by Mir, John (Mir et al., 2018). Briefly, 6.0 mL of 50 mM phosphate buffer containing polyvinylpolypyrrolidone (PVP) and 0.5 mM ethylenediaminetetraacetic acid (EDTA) was used to extract the treated barnyardgrass seeds (1.0 g FW). The homogenates were centrifuged, and the resulting crude enzyme extracts were used to test enzyme activity. The absorbance values of soluble proteins were determined colorimetrically at 595 nm using the Bradford method (Bradford, 1976). All the biochemical indexes obtained were determined in four replicates. Superoxide dismutase (SOD), catalase (CAT), and guaiacol peroxidases (POD) were determined via colorimetric assay following the method described by Chen and Zhang (2016). SOD (EC 1.15.1.1) activity was determined at 560 nm using nitro-blue tetrazolium (NBT) photoreduction. The reaction mixture was composed of 100 mM potassium phosphate (pH 7.8) containing 130 mM methionine, 750 μM NBT, 100 mM EDTA and 20 μM riboflavin. Enzyme extract (1.0 mL) was added to the reaction mixture, except in the case of the control. The mixture was then incubated in light for 30 minutes and the specific activity determined, expressed in SOD unit/mg protein. One unit of SOD is defined as the amount that inhibits the photoreduction of NBT by 50%. Similarly, to determine CAT (EC 1.11.1.6) activity, the crude enzyme extract (1.0 mL) was added to a CAT reaction mixture, which included phosphate buffer (100 mM, pH 7.0) and H2O2 (10 mM). The change in absorbance because of H2O2 consumption was immediately monitored at 240 nm at every 15 seconds for 1 minute, looking for steady average alteration. The enzyme activity was then calculated and represented as unit/mg protein. One unit of CAT is defined as the amount of enzyme that decreases the absorbance at 240 nm by 0.1 per minute. Finally, guaiacol was used to measure POD (EC 1.11.1.7) activity. In this assay, 1.0 mL of enzyme extract was added to a mixture of phosphate buffer (100 mM, pH 7.0), EDTA (0.1 mM), guaiacol (0.5%), and H2O2 (1.0 mM). The increase in absorbance caused by guaiacol oxidation was immediately measured at 470 nm every 15 seconds for 1 minute. The determined enzyme activity was expressed as unit/mg protein. One unit of POD is defined as the amount of enzyme that increases the absorbance at 470 nm by 0.01 per minute.
2.7 Statistical analysis
Experiment was arranged in a completely randomized design with four replicates and were repeated two times. Each sample was collected in four replicates for biochemical analyses. The results are shown as means. All data were analyzed using the SAS software and subjected to analysis of variance (ANOVA) and a comparison of means by Tukey’s multiple range tests (p≤ 0.05). Means followed by the same letter(s) are not different.
3 Results and discussion
3.1 Effect on E. crus-galli germination and early growth
Diaporthe sp. EC010 powdered mycelia were weighed and subjected to sequential extraction. The highest extraction yield was obtained from the EtOH fraction, followed by the EtOAc and hexane fractions respectively (data not shown). Table 1 shows the effect of these extracts on E. crus-galli germination and seedling growth. For all extracts, germination percentage decreased as the extract concentration increased. The EtOAc fraction had significantly greater impact on barnyardgrass germination compared to the other solvents; at the highest tested concentration of 2.50 mg/mL, the respective inhibition percentages were 81.01%, 24.05%, and 12.66% for the EtOAc, EtOH, and hexane fractions (Figure 1). Apart from its impact on seed germination, the EtOAc fraction also had an effect on the growth of E. crus-galli seedlings. Physical examination of the shoot and root lengths showed that the EtOAc fraction treatment decreased both of them across the concentration range. Notably, grass seedling roots were extremely restricted compared to the shoots (Laosinwattana et al., 2018; Somala et al., 2022). Because the root of the E. crus-galli seedlings soaked in Petri dishes containing the extract, the inhibitory effect of the extract on the root of the seedlings was found to be greater than that on the shoot. Teerarak, Charoenying (Teerarak et al., 2012) similarly studied the effect of Aglaia odorata EtOAc extract in wettable powder formulation on E. crus-galli germination and initial growth, in which treated seeds showed lower imbibition and α-amylase activity. Regarding the differing results of the three extracts tested here, differences in inhibitory efficacy may be caused by several factors such as composition, solvent solubility, and solvent polarity. Thus, inhibitory activity is well-established as varying across extracts made using different solvents (Li et al., 2006; Luthria et al., 2007; Garcia et al., 2010). These reports support that it is imperative to use suitable solvent solutions when performing selective extraction on natural sources in order to get fractions with high allelopathic potential and high crude extract yield. As the assay results indicated the EtOAc fraction to have the most powerful inhibitory efficacy, that fraction was used in the subsequent experiments.
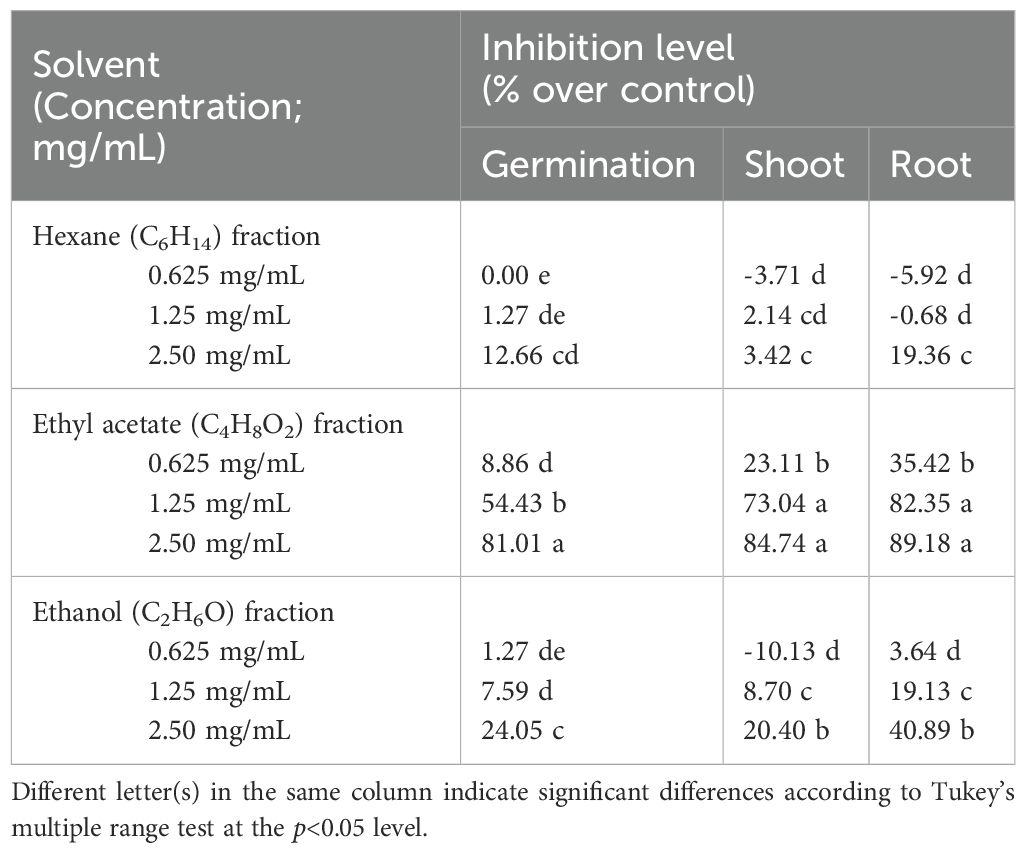
Table 1. Respective effects of sequential Diaporthe solvent extracts on the germination and growth of Echinochloa crus-galli seeds.
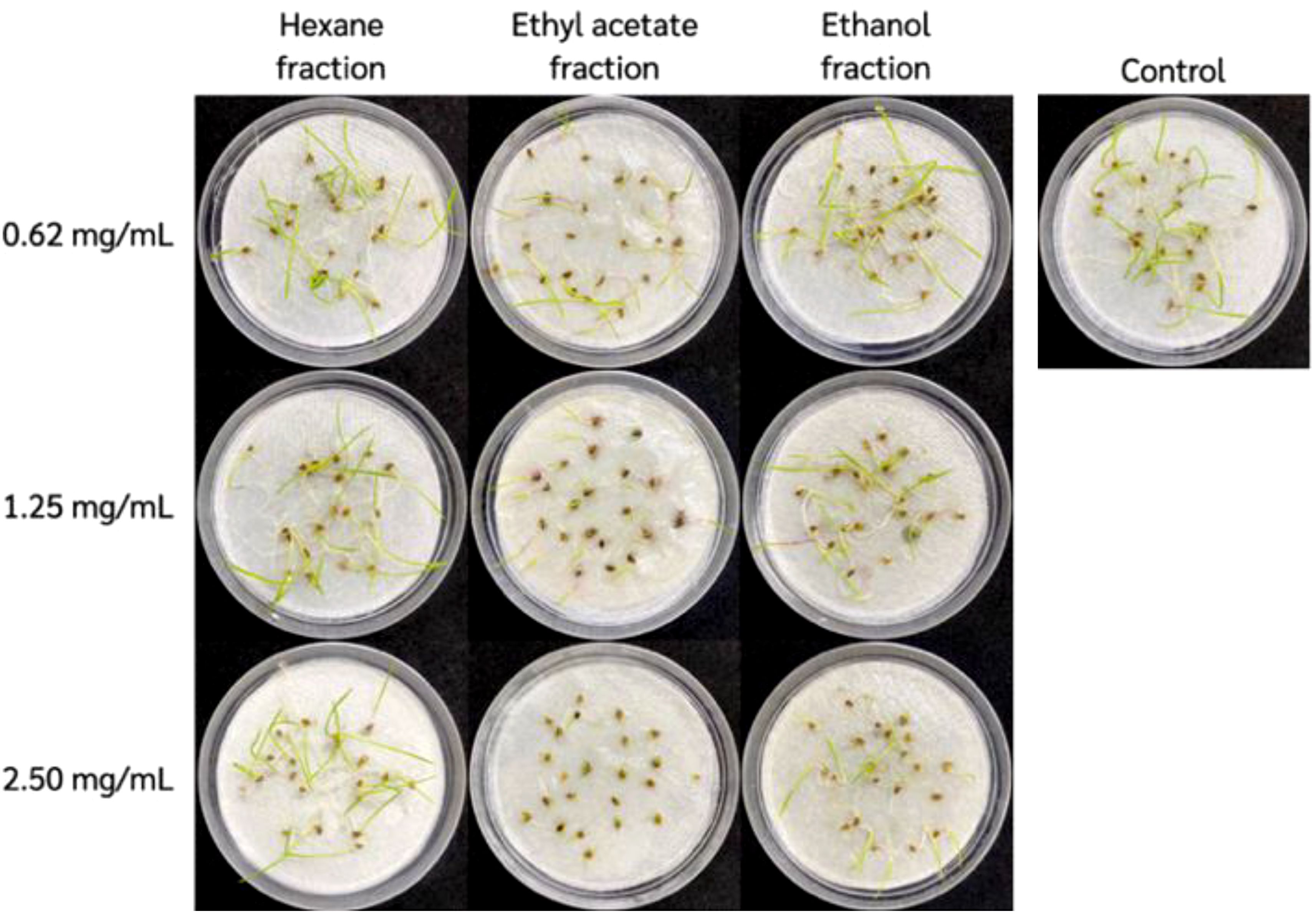
Figure 1. Inhibitory effects observed during bioassays with sequential solvent extracts of Diaporthe sp. mycelia.
3.2 Chemical constituents of the Diaporthe EtOAc crude extract
Table 2 lists the chemical components identified by GC-MS in the Diaporthe mycelia EtOAc crude extract. This analysis revealed the extract to contain a variety of phytocompounds (Figure 2), with 40 identified components collectively accounting for 86.44% of the total crude extract. The main constituents (Figure 3) were linoleic acid, butyl ester (9.69%), hexadecenoic acid (7.99%), and 14-pentadecenoic acid (7.86%). These findings are consistent with the prior reports of da Rosa, Sauzem (da Rosa et al., 2021) and dos Reis, da Rosa (dos Reis et al., 2019), who found fatty acid esters and diaza-compounds to be the major chemical constituents of Diaporthe schini extracts. Notably, the specific activities of metabolic enzymes (Winter et al., 2011; Tanapichatsakul et al., 2019) and species diversity may lead to chemical constituent differentiation. The phytotoxicity observed in this study could be associated with these identified chemicals and possible synergies among them.
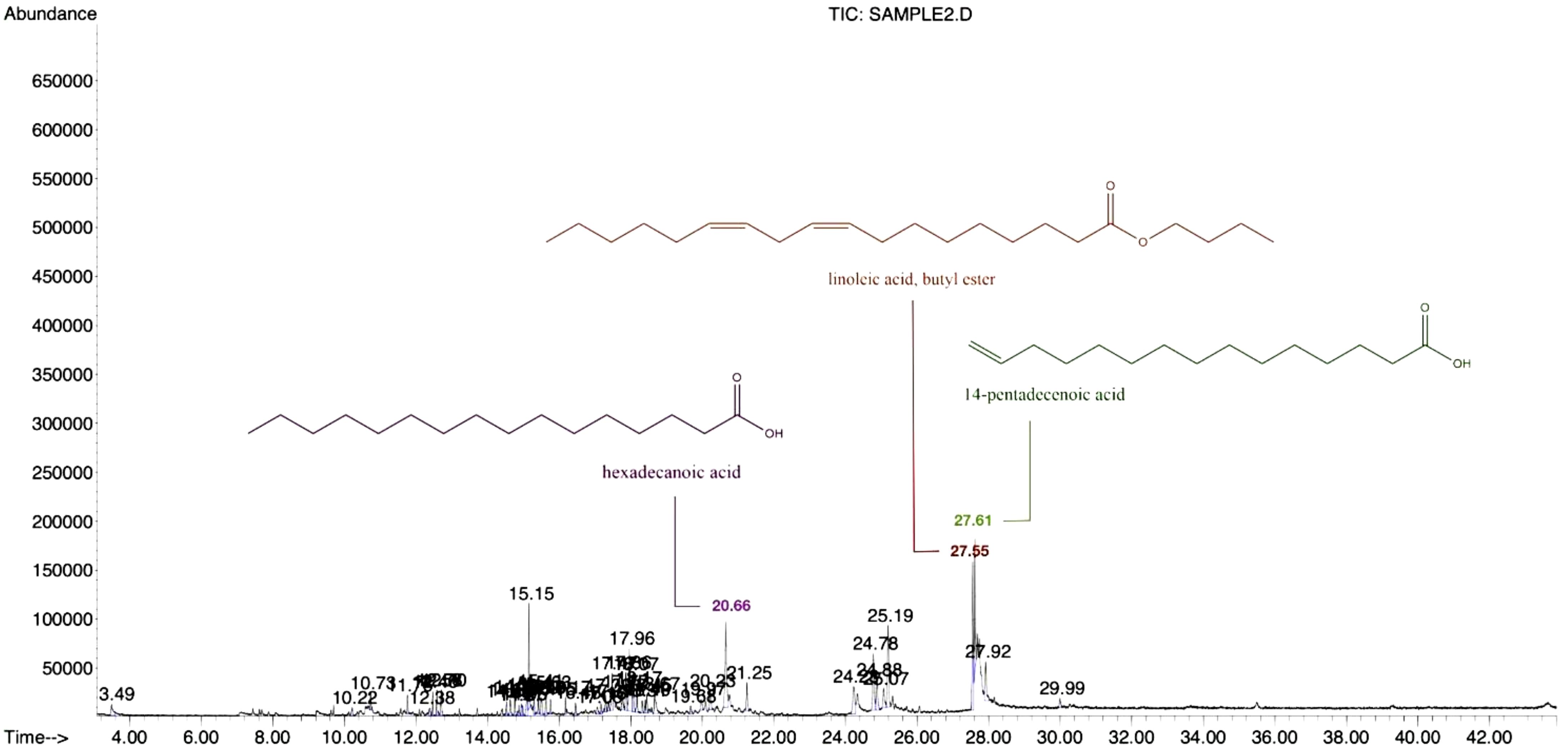
Figure 2. GC-MS chromatogram and chemical structure of the main compounds of the Diaporthe sp. EtOAc crude extract showing the retention times (min) of the peaks.
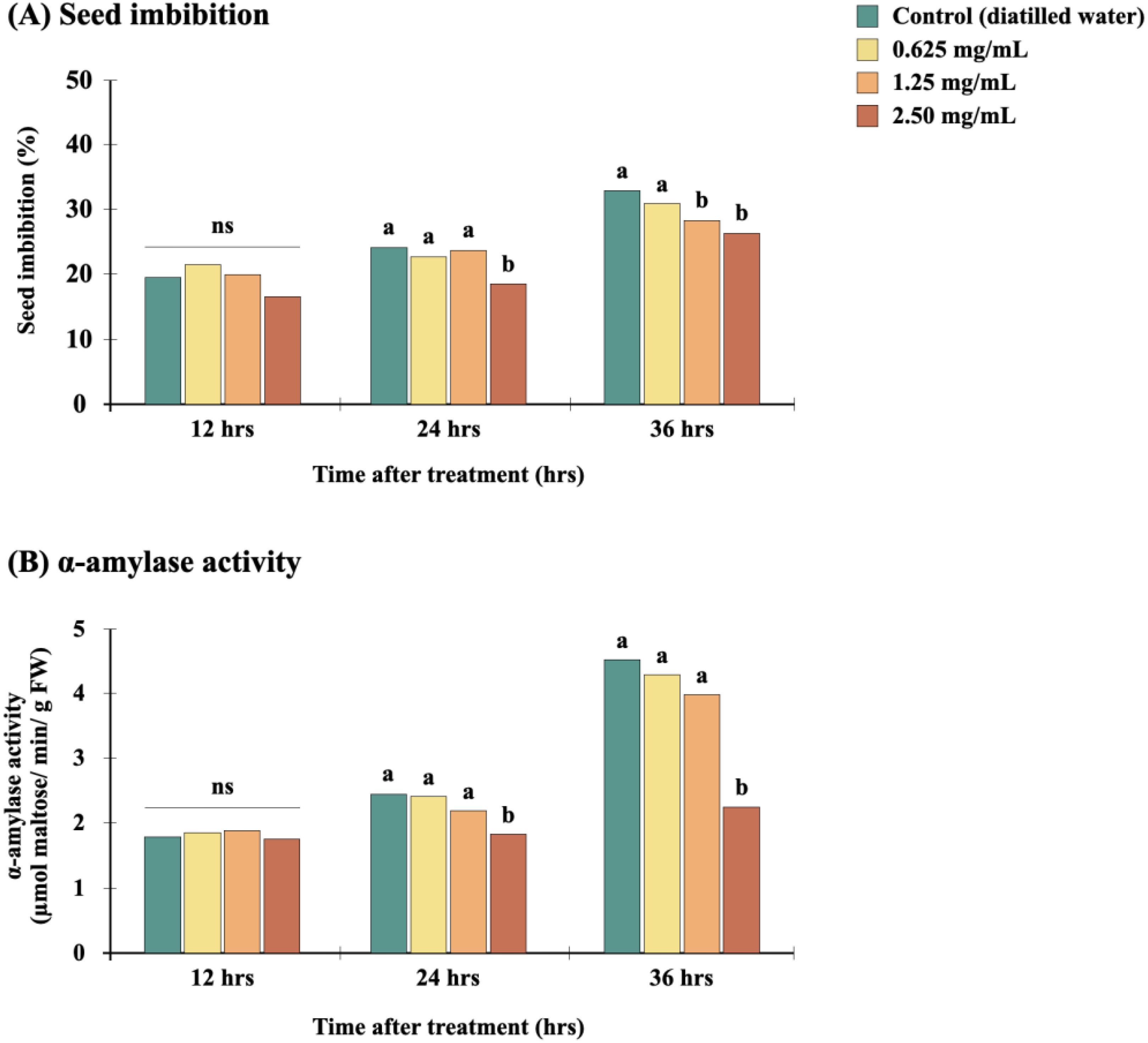
Figure 3. Effect of the Diaporthe sp. EtOAc crude extract on imbibition (A) and α-amylase activity (B) of E. crus-galli seeds. For a given exposure time, bars with different letters (a, b) among treatments are significantly different at p<0.05 according to Tukey’s multiple range test. “ns” indicates no significant difference. Data are mean values (n=4).
3.3 Effect on seed imbibition and α-amylase activity
We first investigated whether seed imbibition, the initial phase in the seed germination process, is disrupted by the Diaporthe EtOAc crude extract. In the first 12 hours following immersion of grass seeds in the extract or distilled water, the absorbing rate was high and not significantly different (p>0.05) from the control. From 12 to 24 hours, the seeds absorbed more water than in the first stage. Then, from 24 to 36 hours, Diaporthe extract clearly disrupted seed imbibition, depending on the treatment concentration and duration (Figure 3A). This can be attributed to the extract changing the water potential, which caused osmotic stress (Manichart et al., 2023a). Decreased imbibition suggests that water and mineral nutrient uptake is limited, which restricts seedling growth (Kamran et al., 2020), as supported by previously published studies (Teerarak et al., 2012; Laosinwattana et al., 2018; Manichart et al., 2023b).
We further investigated the effect of the Diaporthe extract on α-amylase activity, shown in Figure 3B. During seed germination, the main energy source is the endosperm starch and its breakdown. This process depends upon several enzymes, including α-glucosidase, debranching enzyme, and the α- and β-amylases (Amato et al., 2023), of which α-amylase functions throughout the germination process to break down starch into smaller molecules, thereby providing the nutrients and energy required for germination (Li et al., 2019). After treating grass seeds with Diaporthe extract, α-amylase was extracted from the seeds and its activity determined by in vitro generation of maltose. As a result, degradation of α-amylase activity was inhibited by all concentration of the extract correlate to the germination (Table 1). Overall, α-amylase activity was decreased in extract-treated grass seeds compared to controls. Moreover, the extract inhibited α-amylase activity in a dose-dependent manner, with the highest tested concentration (2.50 mg/mL) demonstrating a remarkably extensive decrease of α-amylase activity at 36 hours after treatment (p<0.05). This finding is compatible with the seed imbibition assay results, and aligns with the literature on the herbicidal effects of fungal extracts from Alternaria brassicicola and Diaporthe sp. isolate EC010 on amaranth, a dicotyledonous weed plant (Manichart et al., 2023a; Manichart et al., 2023b). According to the current study’s findings, one of herbicidal properties of this extract is the inhibition of α-amylase activity. Because starch fails to break down into tiny molecules to support growth and development, this impact may in turn result in inhibition of seed germination and seedling growth.
3.4 Effect on MDA, ROS generation, and activities of antioxidant enzymes
The degree of ROS activity in plant cells is contingent on the ability of ROS to move across cell membranes. Among ROS, H2O2 is the most stable and easily moves through membranes over relatively long distances, even in dry seeds. Ratajczak, Małecka (Ratajczak et al., 2015) and Kurek, Plitta-Michalak (Kurek et al., 2019) demonstrated a significant correlation between H2O2 levels and reduced germination (r = -0.9471, p = 0.004139). Accordingly, in this study, we focus on the accumulation of H2O2 in E. crus-galli seeds as a window into the role of oxidative stress in the mechanism of action of the Diaporthe sp. EtOAc fraction (Figures 4A, B). Plant cell membranes are composed of fatty acids and other lipids, and thus free lipids could be released from degraded cell membranes as the result of oxidative action (Scrivanti et al., 2003). Lipid peroxidation of fatty acids ultimately leads to accumulation of MDA (Marnett, 1999). In the present study, seed MDA and H2O2 content were observed to be significantly elevated after 24 and 36 hours of exposure to 2.50 mg/mL of the fungal extract, with 84.47% and 85.52% increases in MDA and 137.17% and 141.10% increases in H2O2 concentration, respectively. Thus, treatment with the extract significantly increased H2O2 production and caused lipid peroxidation, resulting in induced oxidative damage to the E. crus-galli seeds (Oracz et al., 2009; Ma et al., 2016). H2O2 has been considered both a beneficial (Oracz et al., 2009; Bahin et al., 2011) and detrimental factor with regard to cellular events in seed germination. As it regulates gene expression and phytohormone signaling (abscisic acid, gibberellins, auxins, and ethylene) (Bailly, 2019), a moderate level of H2O2 is beneficial and promotes germination, whereas excessive H2O2 content induces oxidative damage, which prevents or delays germination (Kurek et al., 2019). This dual role has been observed in cotton seeds, for which higher levels of H2O2 and lipid peroxidation correlated with reduced germination (Goel and Sheoran, 2003), and in aging sunflower seeds by Kibinza, Vinel (Kibinza et al., 2006), who established a linear association between H2O2 content and germination. Likewise, declining viability of wheat seeds was found to be dependent on both H2O2 production and the level of lipid peroxidation (Kong et al., 2015), which modifies membrane permeability and thereby decreases seed viability.
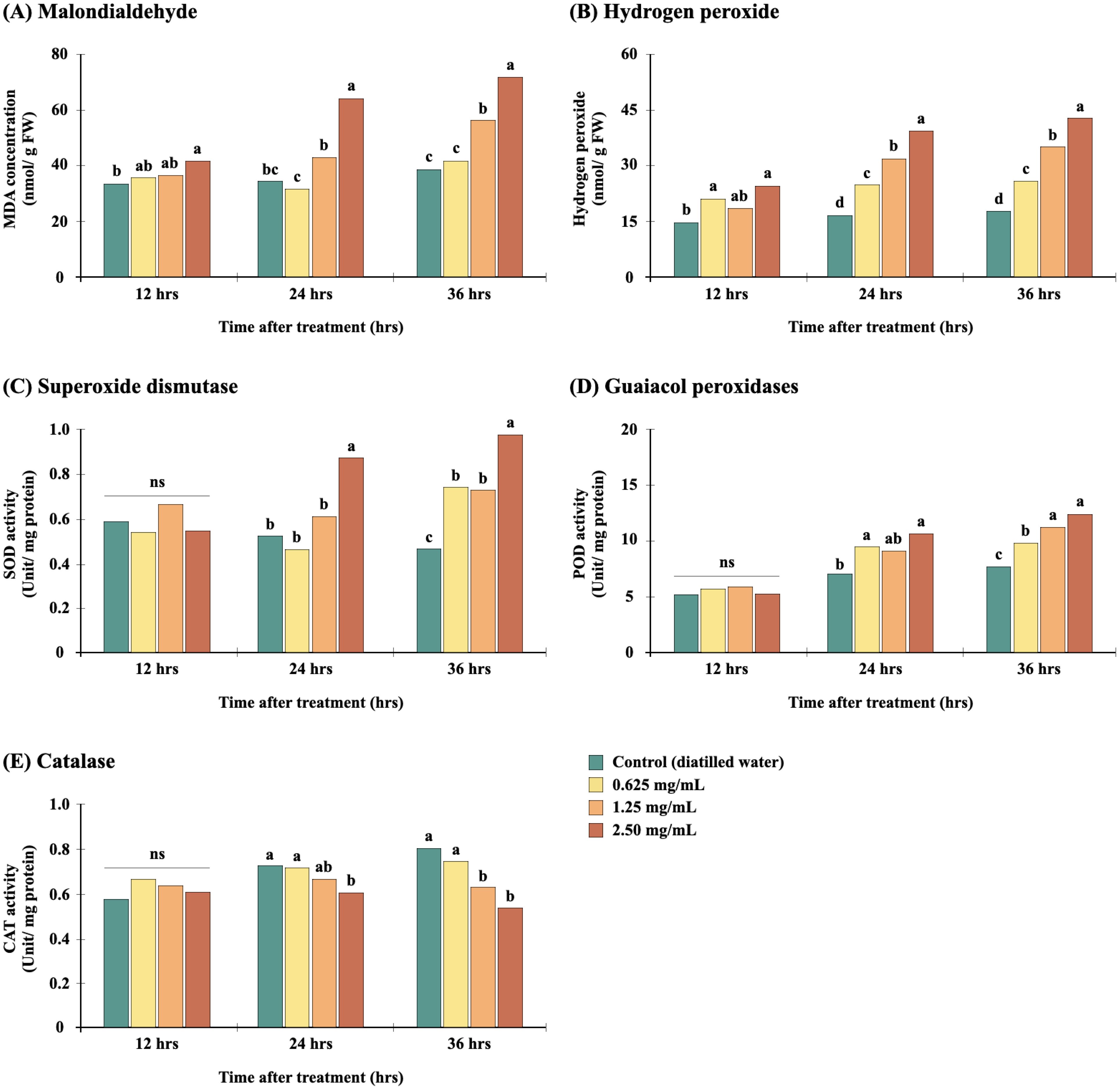
Figure 4. Effect of the Diaporthe sp. EtOAc crude extract on MDA (A) and H2O2 (B) content and SOD (C), POD (D) and CAT (E) activity in E. crus-galli. For a given exposure time, different letters (a, b) above bars indicate significant differences at p<0.05. “ns” indicates no significant difference. Data are mean values (n=4).
Antioxidant enzymes play an important role in redox regulation by balancing ROS levels. We found SOD activity to be significantly increased in E. crus-galli seed exposed to the Diaporthe sp. extract (Figure 4C), reaching 67.24% over control (p<0.05) at 36 hours after germination. POD activity also consistently increased as the germination period progressed (Figure 4D), with the maximum dose producing increases of 49.87% and 61.62% at 24 and 36 hours, respectively. Meanwhile, unlike SOD and POD, CAT activity showed a decreasing tendency in a dose-dependent manner (Figure 4E), indicating the extract to downregulate CAT enzymatic activity. As SOD and POD are involved in ROS scavenging (Shuai et al., 2011; Bhardwaj et al., 2021), their higher activities in treated E. crus-galli seeds may indicate induction of these antioxidant enzymes to help cells cope with the extract-induced oxidative stress and minimize free radical development (Narayan et al., 2024). A previous study suggested higher proline activity in Amaranthus tricolor treated with Diaporthe extract (Manichart et al., 2023a). Proline also serves as a free radical scavenger, protecting against protein denaturation and membrane degradation (Kumar et al., 2018; Kamran et al., 2020). Nonetheless, as the Diaporthe extract in this study demonstrated negative effects on seed germination regardless of the concentration applied, the induced SOD and POD was not sufficient to inhibit the proliferation of ROS and MDA. The significant decrease in CAT activity likely exacerbated oxidative bursts. In a similar study of sunflower seeds, CAT enzymatic activity was observed to decrease under accelerated oxidative stress (Kibinza et al., 2011). Moreover, Ratajczak, Małecka (Ratajczak et al., 2015) reported decreased activity of CAT in beech seeds and a strong negative correlation (r = -0.9177) of CAT activity with the level of H2O2. In our hypothetical model, shown in Figure 5, the decrease in CAT activity might have resulted in ROS accumulation, leading to lipid peroxidation and enzyme inactivation, which could in turn promote structural and functional damage to proteins and carbohydrates and modification or disruption of DNA structure (Sharma et al., 2012). Such events would explain the reduced germination percentage observed in extract-treated seeds.
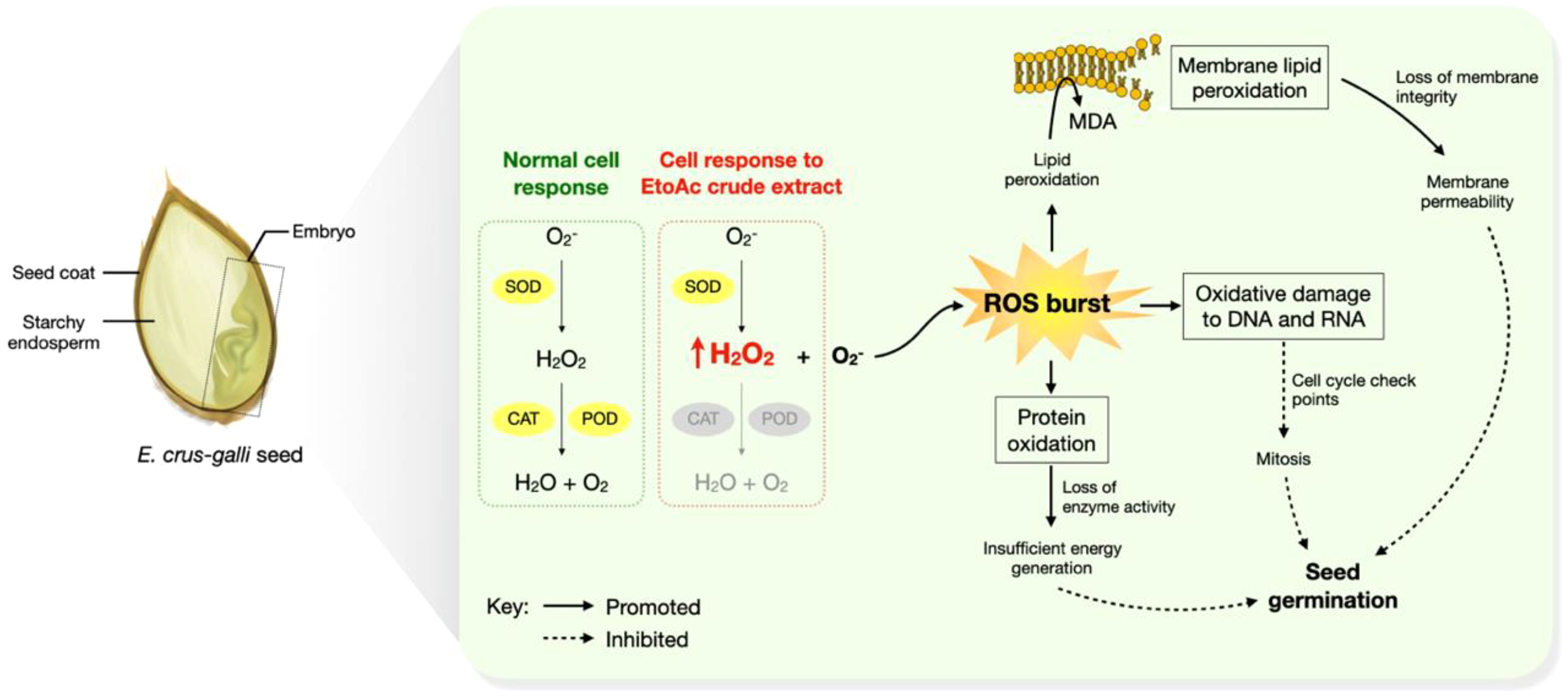
Figure 5. A hypothetical model of the mechanism of seed germination inhibition by the Diaporthe sp. EtOAc crude extract.
Our study has demonstrated the potential of fungal allelochemicals in inhibiting weed growth through oxidative damage, contributing valuable insights to the limited literature on this topic. Future work will focus on optimizing the formulation of the extract, investigating its selectivity, and conducting comprehensive field trials. We will also assess potential environmental impacts to ensure the safety and sustainability of this approach. This pioneering study serves as a foundation for the development of fungus-derived herbicides as an alternative weed control management strategy.
4 Conclusion
This study explores the inhibitory effects of Diaporthe sp. strain EC010 crude extracts on barnyardgrass germination and growth. The ethyl acetate fraction most strongly reduced seed germination and grass development. GC-MS of that fraction identified its major constituents to include linoleic acid, butyl ester (9.69%), hexadecanoic acid (7.99%), and 14-pentadecenoic acid (7.86%). Treated E. crus-galli seeds exhibited lower imbibition, reduced α-amylase activity, increased MDA, and H2O2 accumulation. They also demonstrated higher SOD and POD activities but lower CAT activity, which may correlate with delayed germination and seedling growth. All told, this study constitutes the investigation of oxidative damage caused by Diaporthe extract. This research highlights the potential of Diaporthe extract as a candidate for pre-emergence herbicidal products. Although the findings are promising, they are based on laboratory assays and focus on a single weed species. Further studies are essential to determine the efficacy of Diaporthe extracts under field conditions, evaluate their effects on crop species, soil health, and environmental factors, and understand their roles in integrated weed management strategies.
Data availability statement
The original contributions presented in the study are included in the article/supplementary material. Further inquiries can be directed to the corresponding author.
Author contributions
NS: Conceptualization, Investigation, Project administration, Writing – original draft. NM: Formal Analysis, Methodology, Writing – original draft. CL: Conceptualization, Funding acquisition, Resources, Supervision, Writing – review & editing. PW: Formal Analysis, Validation, Writing – review & editing. KY: Formal Analysis, Validation, Writing – review & editing. MT: Formal Analysis, Investigation, Methodology, Writing – original draft. NC: Formal Analysis, Validation, Writing – review & editing.
Funding
The author(s) declare that financial support was received for the research, authorship, and/or publication of this article. The authors thank National Science, Research and Innovation Fund (NSRF; Grant number RE-KRIS/FF67/026), Thailand for the financial support.
Conflict of interest
The authors declare that the research was conducted in the absence of any commercial or financial relationships that could be construed as a potential conflict of interest.
Publisher’s note
All claims expressed in this article are solely those of the authors and do not necessarily represent those of their affiliated organizations, or those of the publisher, the editors and the reviewers. Any product that may be evaluated in this article, or claim that may be made by its manufacturer, is not guaranteed or endorsed by the publisher.
References
Amato G., Caputo L., Francolino R., Martino M., De Feo V., De Martino L. (2023). Origanum heracleoticum essential oils: chemical composition, phytotoxic and alpha-amylase inhibitory activities. Plants. 12, 866. doi: 10.3390/plants12040866
Bahin E., Bailly C., Sotta B., Kranner I., Corbineau F., Leymarie J. (2011). Crosstalk between reactive oxygen species and hormonal signalling pathways regulates grain dormancy in barley. Plant Cell Environ. 34, 980–993. doi: 10.1111/j.1365-3040.2011.02298.x
Bailey K. L. (2014). The bioherbicide approach to weed control using plant pathogens. Integrated Pest Management: Elsevier; p, 245. doi: 10.1016/B978-0-12-398529-3.00014-2
Bailly C. (2019). The signalling role of ROS in the regulation of seed germination and dormancy. Biochem. J. 476, 3019–3032. doi: 10.1042/BCJ20190159
Bhardwaj R. D., Singh N., Sharma A., Joshi R., Srivastava P. (2021). Hydrogen peroxide regulates antioxidant responses and redox related proteins in drought stressed wheat seedlings. Physiol. Mol. Biol. Plants 27, 151–163. doi: 10.1007/s12298-021-00937-z
Bradford M. M. (1976). A rapid and sensitive method for the quantitation of microgram quantities of protein utilizing the principle of protein-dye binding. Analytical Biochem. 72, 248–254. doi: 10.1016/0003-2697(76)90527-3
Chen T., Zhang B. (2016). Measurements of proline and malondialdehyde content and antioxidant enzyme activities in leaves of drought stressed cotton. Bio-protocol. 6, e1913. doi: 10.21769/BioProtoc.1913
Choudhary A., Kumar A., Kaur N. (2020). ROS and oxidative burst: Roots in plant development. Plant Diversity. 42, 33–43. doi: 10.1016/j.pld.2019.10.002
da Rosa B. V., Sauzem G. D. S., Kuhn R. C. (2021). Obtaining antioxidant compounds from the endophytic fungus Diaporthe schini using heat- and ultrasound-assisted extraction. Braz. J. Chem. Engineering. 38, 189–195. doi: 10.1007/s43153-021-00089-3
Dmitrieva V. A., Tyutereva E. V., Voitsekhovskaja O. V. (2024). What can reactive oxygen species (ROS) tell us about the action mechanism of herbicides and other phytotoxins? Free Radical Biol. Med. 220, 92–110. doi: 10.1016/j.freeradbiomed.2024.04.233
dos Reis C. M., da Rosa B. V., da Rosa G. P., do Carmo G., Morandini L. M. B., Ugalde G. A., et al. (2019). Antifungal and antibacterial activity of extracts produced from Diaporthe schini. J. Biotechnol. 294, 30–37. doi: 10.1016/j.jbiotec.2019.01.022
Fujita M., Hasanuzzaman M. (2022). Approaches to enhancing antioxidant defense in plants. Antioxidants (Basel). 11 (5), 925. doi: 10.3390/antiox11050925
Gao W. T., Su W. H. (2024). Weed management methods for herbaceous field crops: A review. Agronomy. 14, 486. doi: 10.3390/agronomy14030486
Garcia L. M. Z., de Oliveira T. F., Soares P. K., Bruns R. E., Scarminio I. S. (2010). Statistical mixture design—Principal component determination of synergic solvent interactions for natural product extractions. Chemometrics Intelligent Lab. Systems. 103, 1–7. doi: 10.1016/j.chemolab.2010.04.016
Goel A., Sheoran I. (2003). Lipid peroxidation and peroxide-scavenging enzymes in cotton seeds under natural ageing. Biol. Plantarum. 46, 429–434. doi: 10.1023/A:1024398724076
Hazrati H., Saharkhiz M. J., Niakousari M., Moein M. (2017). Natural herbicide activity of Satureja hortensis L. essential oil nanoemulsion on the seed germination and morphophysiological features of two important weed species. Ecotoxicology Environ. Safety. 142, 423–430. doi: 10.1016/j.ecoenv.2017.04.041
He J., Ren Y., Chen X., Chen H. (2014). Protective roles of nitric oxide on seed germination and seedling growth of rice (Oryza sativa L. ) under cadmium stress. Ecotoxicology Environ. Saf. 108, 114–119. doi: 10.1016/j.ecoenv.2014.05.021
Huang L., Zhang X., Yang Y., Zou H., Fang B., Liu W. (2021). High-quality genome resource of Diaporthe destruens causing foot rot disease of sweet potato. Plant Disease. 105, 3279–3281. doi: 10.1094/PDIS-07-20-1473-A
Kamran M., Xie K., Sun J., Wang D., Shi C., Lu Y., et al. (2020). Modulation of growth performance and coordinated induction of ascorbate-glutathione and methylglyoxal detoxification systems by salicylic acid mitigates salt toxicity in choysum (Brassica parachinensis L.). Ecotoxicology Environ. Saf. 188, 109877. doi: 10.1016/j.ecoenv.2019.109877
Kibinza S., Bazin J., Bailly C., Farrant J. M., Corbineau F., Bouteau H. (2011). Catalase is a key enzyme in seed recovery from ageing during priming. Plant Science. 181, 309–315. doi: 10.1016/j.plantsci.2011.06.003
Kibinza S., Vinel D., Côme D., Bailly C., Corbineau F. (2006). Sunflower seed deterioration as related to moisture content during ageing, energy metabolism and active oxygen species scavenging. Physiologia Plantarum. 128, 496–506. doi: 10.1111/j.1399-3054.2006.00771.x
Kong L., Huo H., Mao P. (2015). Antioxidant response and related gene expression in aged oat seed. Front. Plant Science. 6, 158. doi: 10.3389/fpls.2015.00158
Kumar A., Kumar A., Kumar P., Lata C., Kumar S. (2018). Effect of individual and interactive alkalinity and salinity on physiological, biochemical and nutritional traits of marvel grass. Indian J. Exp. Biol. 56, 573–581.
Kurek K., Plitta-Michalak B., Ratajczak E. (2019). Reactive oxygen species as potential drivers of the seed aging process. Plants. 8, 174. doi: 10.3390/plants8060174
Laosinwattana C., Wichittrakarn P., Teerarak M. (2018). Chemical composition and herbicidal action of essential oil from Tagetes erecta L. Leaves Ind. Crops Products. 126, 129–134. doi: 10.1016/j.indcrop.2018.10.013
Li B., Smith B., Hossain M. M. (2006). Extraction of phenolics from citrus peels: I. Solvent extraction method. Separation Purification Technology. 48, 182–188. doi: 10.1016/j.seppur.2005.07.005
Li Q., Yang A., Zhang W.-H. (2019). Higher endogenous bioactive gibberellins and α-amylase activity confer greater tolerance of rice seed germination to saline-alkaline stress. Environ. Exp. Botany. 162, 357–363. doi: 10.1016/j.envexpbot.2019.03.015
Luo X. W., Chen C. M., Li K. L., Lin X. P., Gao C. H., Zhou X. F., et al. (2021). Sesquiterpenoids and meroterpenoids from a mangrove derived fungus Diaporthe sp. SCSIO 41011. Natural product Res. 35, 282–288. doi: 10.1080/14786419.2019.1627355
Luthria D. L., Biswas R., Natarajan S. (2007). Comparison of extraction solvents and techniques used for the assay of isoflavones from soybean. Food Chem. 105, 325–333. doi: 10.1016/j.foodchem.2006.11.047
Ma Z., Marsolais F., Bykova N. V., Igamberdiev A. U. (2016). Nitric oxide and reactive oxygen species mediate metabolic changes in barley seed embryo during germination. Front. Plant Sci. 7. doi: 10.3389/fpls.2016.00138
Manichart N., Laosinwattana C., Somala N., Teerarak M., Chotsaeng N. (2023a). Physiological mechanism of action and partial separation of herbicide-active compounds from the Diaporthe sp. extract on Amaranthus tricolor L. Sci. Rep. 13, 18693. doi: 10.1038/s41598-023-46201-0
Manichart N., Somala N., Laosinwattana C. (2023b). Allelopathic potential of secondary metabolites produced by Alternaria brassicicola and physiological mechanisms on Amaranthus tricolor. Int. J. Agric. Technol. 19, 1115–1126.
Marnett L. J. (1999). Lipid peroxidation-DNA damage by malondialdehyde. Mutat. Research/Fundamental Mol. Mech. Mutagenesis 424, 83–95. doi: 10.1016/s0027-5107(99)00010-x
Mir M. A., John R., AlYemeni M. N., Alam P., Ahmad P. (2018). Jasmonic acid ameliorates alkaline stress by improving growth performance, ascorbate glutathione cycle and glyoxylase system in maize seedlings. Sci. Rep. 8, 2831. doi: 10.1038/s41598-018-21097-3
Mishra N., Jiang C., Chen L., Paul A., Chatterjee A., Shen G. (2023). Achieving abiotic stress tolerance in plants through antioxidative defense mechanisms. Front. Plant Sci. 14. doi: 10.3389/fpls.2023.1110622
Narayan H., Kumar U., Chowdhury T., Swain P., Barik M., Nayak A. K. (2024). Effect of salinity stress on growth, chlorophyll, antioxidant enzymes and nutrient content in Azolla spp. Aquat. Botany. 192, 103750. doi: 10.1016/j.aquabot.2024.103750
Nazarkov M., Gerasimova I. (2023). Dynamics of weeds and integrated weed control in crop rotation with cereals. Bulgarian J. Crop Science/Rastenievdni Nauki. 60 (2), 17–25.
Oracz K., Hayat E. M. B., Kranner I., Bogatek R., Corbineau F., Bailly C. (2009). The mechanisms involved in seed dormancy alleviation by hydrogen cyanide unravel the role of reactive oxygen species as key factors of cellular signaling during germination. Plant Physiol. 150, 494–505. doi: 10.1104/pp.109.138107
Poonpaiboonpipat T., Krumsri R., Kato-Noguchi H. (2021). Allelopathic and Herbicidal Effects of Crude Extract from Chromolaena odorata (L.) RM King and H. Rob. on Echinochloa crus-galli and Amaranthus viridis. Plants 10, 1609. doi: 10.3390/plants10081609
Radhakrishnan R., Alqarawi A. A., Allah E. F. (2018). Bioherbicides: Current knowledge on weed control mechanism. Ecotoxicology Environ. Safety. 158, 131–138. doi: 10.1016/j.ecoenv.2018.04.018
Ratajczak E., Małecka A., Agnieszka B. Z., Kalemba E. M. (2015). The production, localization and spreading of reactive oxygen species contributes to the low vitality of long-term stored common beech (Fagus sylvatica L.) seeds. J. Plant Physiol. 174, 147–156. doi: 10.1016/j.jplph.2014.08.021
Sadasivam S., Manickam A. (1996). Biochemical Methods New Age International (P) Ltd (New Delhi: Publishers).
Saini R., Singh S. (2019). Use of natural products for weed management in high-value crops: An overview. Am. J. Agric. Res. 25 (4), 1–13.
Scrivanti L. R., Zunino M. P., Zygadlo J. A. (2003). Tagetes minuta and Schinus areira essential oils as allelopathic agents. Biochem. Systematics Ecology. 31, 563–572. doi: 10.1016/S0305-1978(02)00202-8
Sharma P., Jha A. B., Dubey R. S., Pessarakli M. (2012). Reactive oxygen species, oxidative damage, and antioxidative defense mechanism in plants under stressful conditions. J. Bot. 2012, 217037. doi: 10.1155/2012/217037
Shuai L., Yuan Y., Chen P., Lin S. (2011). Correlation between H2O2 scavenging system and flavonoids accumulation of Scutellaria baicalensis. Zhongguo Zhong Yao Za Zhi 36, 1707–1710.
Somala N., Laosinwattana C., Teerarak M. (2022). Formulation process, physical stability and herbicidal activities of Cymbopogon nardus essential oil-based nanoemulsion. Sci. Rep. 12, 10280. doi: 10.1038/s41598-022-14591-2
Sun W., Huang S., Xia J., Zhang X., Li Z. (2021). Morphological and molecular identification of Diaporthe species in south-western China, with description of eight new species. MycoKeys. 77, 65–95. doi: 10.3897/mycokeys.77.59852
Takao L. K., Ribeiro J. P. N., Lima M. I. S. (2011). Allelopathic effects of Ipomoea cairica (L. ) Sweet Crop weeds. Acta Botanica Brasilica 25, 858–864. doi: 10.1590/S0102-33062011000400012
Tanapichatsakul C., Khruengsai S., Monggoot S., Pripdeevech P. (2019). Production of eugenol from fungal endophytes Neopestalotiopsis sp. and Diaporthe sp. isolated from Cinnamomum loureiroi leaves. PeerJ. 7, e6427. doi: 10.7717/peerj.6427
Teerarak M., Charoenying P., Laosinwattana C. (2012). Physiological and cellular mechanisms of natural herbicide resource from Aglaia odorata Lour. on bioassay plants. Acta Physiologiae Plantarum. 34, 1277–1285. doi: 10.1007/s11738-011-0923-5
Teerarak M., Laosinwattana C., Charoenying P. (2010). Evaluation of allelopathic, decomposition and cytogenetic activities of Jasminum officinale L. f. var. grandiflorum (L.) Kob. on bioassay plants. Bioresource Technology. 101, 5677–5684. doi: 10.1016/j.biortech.2010.02.038
Tian W., Liao Z., Zhou M., Wang G., Wu Y., Gao S., et al. (2018). an unusual asymmetric bisanthraquinone with cage-like skeleton from the endophytic fungus Diaporthe sp. Fitoterapia. 128, 253–257. doi: 10.1016/j.fitote.2018.05.032
Turk M. A., Tawaha A. (2003). Allelopathic effect of black mustard (Brassica nigra L.) on germination and growth of wild oat (Avena fatua L.). Crop Prot. 22, 673–677. doi: 10.1016/S0261-2194(02)00241-7
Velikova V., Yordanov I., Edreva A. (2000). Oxidative stress and some antioxidant systems in acid rain-treated bean plants: Protective role of exogenous polyamines. Plant Science. 151, 59–66. doi: 10.1016/S0168-9452(99)00197-1
Winter J. M., Behnken S., Hertweck C. (2011). Genomics-inspired discovery of natural products. Curr. Opin. Chem. Biol. 15, 22–31. doi: 10.1016/j.cbpa.2010.10.020
Wu J., Zhai Y., Monikh F. A., Daniel A. L., Grillo R., Vijver M. G., et al. (2021). The Differences between the effects of a nanoformulation and a conventional form of atrazine to lettuce: physiological responses, defense mechanisms, and nutrient displacement. J. Agric. Food Chem. 69, 12527–12540. doi: 10.1021/acs.jafc.1c01382
Xu T. C., Lu Y. H., Wang J. F., Song Z. Q., Hou Y. G., Liu S. S., et al. (2021). Bioactive secondary metabolites of the genus Diaporthe and anamorph Phomopsis from terrestrial and marine habitats and endophytes: 2010–2019. Microorganisms. 9, 217. doi: 10.3390/microorganisms9020217
Zhang Q., Ma W. G., Zhao Q., Zhao Y. Y., Huang Z. P., Xu Y. X., et al. (2021). [amp]]alpha;-pyrone derivatives from endophytic fungus Diaporthe sp. RJ-41. Biochem. Systematics Ecology. 94, 104198. doi: 10.1016/j.bse.2020.104198
Keywords: allelochemicals, barnyardgrass, Diaporthe sp., oxidative damage, phytotoxicity
Citation: Somala N, Manichart N, Laosinwattana C, Wichittrakarn P, Yoneyama K, Teerarak M and Chotsaeng N (2024) Oxidative damage in Echinochloa crus−galli seeds exposed to Diaporthe sp. (Diaporthales, Ascomycota) fungal extract during germination. Front. Agron. 6:1456168. doi: 10.3389/fagro.2024.1456168
Received: 28 June 2024; Accepted: 06 November 2024;
Published: 25 November 2024.
Edited by:
Thomas R. Butts, Purdue University, United StatesReviewed by:
Adnan Akhter, University of the Punjab, PakistanAnaytullah Siddique, Lovely Professional University, India
Copyright © 2024 Somala, Manichart, Laosinwattana, Wichittrakarn, Yoneyama, Teerarak and Chotsaeng. This is an open-access article distributed under the terms of the Creative Commons Attribution License (CC BY). The use, distribution or reproduction in other forums is permitted, provided the original author(s) and the copyright owner(s) are credited and that the original publication in this journal is cited, in accordance with accepted academic practice. No use, distribution or reproduction is permitted which does not comply with these terms.
*Correspondence: Chamroon Laosinwattana, Y2hhbXJvb24ubGFAa21pdGwuYWMudGg=