- 1Instituto de Biotecnología y Biología Molecular (IBBM)-Facultad de Ciencias Exactas, Universidad Nacional de La Plata y CCT-La Plata, CONICET, La Plata, Argentina
- 2Cátedra de Genética-Facultad de Ciencias Agrarias y Forestales, Universidad Nacional de La Plata, La Plata, Argentina
- 3Laboratorio de Investigación y Desarrollo de Métodos Analíticos, Universidad Nacional de La Plata y CIC-PBA, La Plata, Argentina
Greenhouse gas emissions, such as N2O from excessive N-fertilizer use, are of concern. Symbiotic N2-fixation by pulses such as soybean might mitigate this issue, for which inoculants carrying locally adapted Bradyrhizobium strains are recommended. In the frame of this goal, enhancing the quality control of these inoculants is required on two key aspects: determining the presence of nosZ, for the strains being able to reduce N2O, and assessing N2-fixation potential. Previously it was demonstrated that, in soybean leaves, N-contents are well correlated with chlorophyll contents. However, no such correlations were made with either N obtained from N2-fixation or with nodules mass, which is an indicator of nodular activity. Here we aimed to leverage the correlation between N and chlorophyll levels to develop a simple and non-destructive laboratory method to be applied in quality control of inoculants, able to assess the N2-fixing capacity of rhizobial strains. To establish such correlations, we cultivated soybeans in vermiculite with N-free nutrient solution, and inoculated them with various Bradyrhizobium field isolates that displayed a range of symbiotic N2-fixing capacities. Subsequently, we measured chlorophyll with a portable chlorophyllometer, and correlated these measures with symbiotic parameters. Moreover, we tested for the presence of nosZ by PCR. We observed significant correlations between chlorophyll and shoot nitrogen obtained from N2-fixation and, in addition, we corroborated that chlorophyll contents were significantly correlated also with nodules mass. Two B. diazoefficiens strains stood out and possessed nosZ. In contrast, B. elkanii and B. japonicum isolates displayed lower chlorophyll and symbiotic performance, and lacked nosZ. Our findings highlight the potential of measuring chlorophyll contents and testing for the presence of nosZ as two straightforward techniques that may enhance laboratory tests for quality control, enabling selection of superior and safe locally isolated strains for soybean inoculants without increased production costs.
1 Introduction
Global warming is becoming evident year after year, whereby it is urgent to strengthen measures to mitigate greenhouse gas emissions. Among the most contaminant human activities is the irrational use of N-fertilizers, which, being applied in up to 10-fold excess in several crops, leach into the soil and decompose producing N2O gas with strong greenhouse and global warming effects (Erisman et al., 2013; Aryal et al., 2022). Therefore, measures intended to mitigate the extended use of N-fertilizers are urgent. It is well known that biological N2 fixation may replace N-fertilizers in legume crops, and even incorporate N to the soil (Imran et al., 2021). Soybean [Glycine max (L.) Merr.], the most important grain legume worldwide, is a host for diverse N2-fixing bacteria and, in particular, Bradyrhizobium diazoefficiens, B. elkanii, and B. japonicum are commonly used as inoculants in vast cropping areas. However, mineralized N from decomposing roots, nodules, and aerial biomass incorporated as green manure to the soil may undergo denitrification by soil microbiota and release N2O if the pathway is not completed until total reduction to N2. Under low-oxygen conditions, such as temporary soil flooding, Bradyrhizobium spp. may use NO3− as electron acceptor in a reaction catalyzed by nitrate reductase (encoded in the napEDABC operon) that renders NO2− as reduced product. In turn, NO2− is reduced to NO in a reaction catalyzed by nitrite reductase (NirK) and, in a next step, NO is reduced to N2O in a reaction catalyzed by nitric oxide reductase (encoded in norCBQD). In particular, nirK and the norCBQD operon are under direct or undirect control of FixK2, a global regulator that controls genes responsive to microoxic conditions (Mesa et al., 2003). The presence of these genes is not even in bradyrhizobia: while the B. japonicum group (that includes B. japonicum and B. diazoefficiens) possesses these genes, the B. elkanii group in general lack them, although in two B. elkanii strains denitrification activity was detected (Obando et al., 2019). Of concern is the lack of the last denitrification step in several strains of Bradyrhizobium that do not possess nosZ, the gene encoding N2O reductase, which catalyzes the reduction of N2O to N2. It was reported that inoculation with such strains of Bradyrhizobium spp. may produce N2O due to incomplete denitrification, by difference with those that possess the nosZ gene, which are able to perform the complete denitrification pathway toward N2 (Siqueira et al., 2017; Obando et al., 2019; Mania et al., 2020). Therefore, inoculation of soybean with nosZ+ strains lead to a significant reduction of N2O emission from this crop in comparison with plots inoculated with nosZ− strains (Akiyama et al., 2016).
In addition, for N2 fixation to be efficient, a positive net balance between N incorporated by the plant from atmosphere and from soil must be achieved. In Argentina, the third-largest producer of soybeans, it was estimated that soybean fixes 2.54 Tg of N per growth cycle, contributing 17% of total symbiotic N2 fixation globally. Since, in the same conditions, 2.43 Tg N are exported within seeds at harvest, it was estimated that the net average gain of N by this crop system is 0.11 Tg (Collino et al., 2015). However, this balance depends on various factors, including environmental conditions, plant cultivars, and the prevalence of Bradyrhizobium spp. genotypes in the soil. Although soybean crops in Argentina are widely inoculated with the elite strain B. japonicum E109 (Torres et al., 2015), other strains are prevalent inside nodules, suggesting a poor adaptability of E109 to the conditions of agricultural soils in Argentina (Iturralde et al., 2019). In addition, E109 is known as a nosZ− strain (Obando et al., 2019) whereby its use as a universal inoculant in Argentina may be harmful regarding N2O emissions. Therefore, it would be of interest to select new strains, better adapted to local conditions, and safer from the point of view of greenhouse gas production, to be incorporated in next-generation inoculants. This process requires better laboratory methods for quality control, able to measure and register the N2-fixing potential and the presence of nosZ with simple, low cost and, if possible, non-destructive technologies.
Several methods for estimating N2-fixation are known from long ago (Burris, 1972), but many are cumbersome, difficult to apply in non-destructive or field conditions, or require costly equipment. One popular method is the acetylene reduction assay, but it was criticized because exposure of nodulated roots to acetylene causes a rapid decline in nitrogenase activity, which was attributed to the cessation of ammonia production by nitrogenase and increase of oxygen diffusion resistance by the nodules (Minchin and Witty, 1989). Furthermore, the magnitude of this decline depends on environmental conditions, rhizobial species or strains, whereby this method is not recommended for comparative studies. Another method involves measuring H2 evolution, which is a by-product of the nitrogenase reaction (Witty and Minchin, 1998), but it is restricted to strains lacking the ability to recycle H2 for generation of extra ATP (Carter et al., 1978). Other methods involve direct measurement of total N accumulated by plants growing hydroponically without added combined N or the measurement of N2-fixation export products, such as ureides in the case of soybean (Herridge, 1982). However, these methods are laborious and often destructive, limiting their application in serial measurements or selection procedures involving individual plants. Alternative methods, such as the use of the 15N isotope, can be applied in field trials but are also destructive and costly (Chalk and Ladha, 1999). Thus, perhaps due to unavailability of rapid, nondestructive, simple, and reliable tests for N2-fixation, the inoculants industry seldom measures this important symbiotic parameter as part of their laboratory protocols for quality control of its strains (Penna et al., 2011; Herrmann and Lesueur, 2013; Herridge et al., 2014; de Souza et al., 2019).
As an alternative, indirect estimation of N2-fixation could be achieved by measuring parameters related to photosynthesis, as it is known that the N-status of leaves is correlated with the rate of photosynthesis in different plant species. The majority of N allocated to leaves is integrated into the photosynthetic apparatus; for instance, it was calculated that 54% of leaf N is related with photosynthesis pool, with RuBisCO alone accounting for 20% (Evans and Clarke, 2019). This might be explained by the relatively low catalytic efficiency of RuBisCO, thus requiring a very large amount of enzyme per unit leaf area for achieving a sufficient net photosynthesis rate (Tcherkez et al., 2006). Therefore, gross leaf photosynthesis and CO2 uptake rate are positively correlated with leaf N concentration in soybean (Boote et al., 1978; Lugg and Sinclair, 1981; Boon-Long et al., 1983; Buttery and Buzzell, 1988).
A non-destructive and rapid method for estimating photosynthesis rate in soybean is the measurement of leaf chlorophyll content using a portable chlorophyllometer (Ma et al., 1995; Castelli et al., 1996). This instrument measures leaf transmittance in the red and infrared wavelengths, allowing for determination of relative chlorophyll content spectrophotometrically using the infrared measure as a self-correction. Although the relationship between chlorophyll content and chlorophyllometer readings is non-linear (Markwell et al., 1995; Uddling et al., 2007), the measurements are being interpreted as a reflection of a plant’s nutritional status in agronomic research. However, previous investigations have failed to consider the role of nodulation in this relationship. Several experiments have shown no response in photosynthesis rate to different levels of N-fertilization in soybean (Moreira et al., 2015). The presence of N2-fixing bacteria may have masked the responses to varying N-fertilization levels. Furthermore, a positive correlation between chlorophyll and leaf N content has been observed in field studies, although it was not strong enough to be used as a predictor of leaf N content (Fritschi and Ray, 2007). Nevertheless, the nodulation state of these plants was not reported by the authors.
The above studies have mostly focused on soybean plants in the pod-filling state, and photosynthesis rate and leaf N content may differ in earlier vegetative stages due to factors such as changes in leaf mass per unit area. In soybean plants grown hydroponically for 23-30 days after germination (around V2 stage), a significant positive correlation was observed between N content per unit leaf area and light-saturated photosynthesis (Adams et al., 2018). However, the nodulation state of these plants again was not reported. Therefore, it remains unclear whether photosynthesis-related parameters can be used as predictors of N2-fixation by root nodule bacteria in soybean. To demonstrate such a relationship, a panel of Bradyrhizobium strains with different N2-fixing capacities should be used instead of different N-fertilizer doses, and indeed the photosynthetic capacity of plants inoculated with such a panel of strains should be measured in the absence of any externally added N-nutrient.
Therefore, in this report we conducted measurements of various symbiotic parameters and chlorophyll relative contents as determined with a chlorophyllometer in soybean plants nodulated by different strains of Bradyrhizobium spp. isolated from an agricultural field at the Province of Buenos Aires, Argentina, in order to assess the correlation among these measures. We found that the chlorophyllometer readings had a good predictive power for the N2-fixing quality of the different strains. In addition, we made use of a PCR protocol to detect the presence of nosZ in these strains. These approaches could be incorporated into laboratory protocols for quality control of inoculant strains to aid in the production of safe and efficient inoculants and supports the recommendation of new strains adapted to local conditions, which may be of value to farmer communities engaged in soybean crop production.
2 Materials and methods
2.1 Bacterial strains, plants, and culture conditions
We obtained B. diazoefficiens USDA 110T from Deane Weber, ARS-USDA, United States, B. japonicum E109 from Alejandro Perticari, IMYZA-INTA, Argentina, B. elkanii USDA 76T, and B. japonicum USDA 6T from Esperanza Martínez-Romero, Centro de Ciencias Genómicas, UNAM, México, B. diazoefficiens SEMIA 5080, and B. japonicum SEMIA 5079 from Mariangela Hungria, EMBRAPA, Brazil, and Azospirillum argentinense Az39T from Fabricio Cassán, UNRC, Argentina. We maintained all these strains, as well as the Bradyrhizobium spp. isolated in this study (see Section 3.1) in glycerol stocks as described (Iturralde et al., 2019). For routine stocks, we grew the bacteria in YMA (Vincent, 1970), and for plants inoculation we grew them in a liquid medium that contained (in g l−1 distilled water): glucose, 5; mannitol, 5; sodium gluconate, 5; yeast extract, 2.2; CaCl2, 0.9; MgCl2, 0.3; K2HPO4, 1.1 and KH2PO4, 0.9. Soybean Bioceres 4.12 RR was provided by Bioceres S.A., Argentina. For nodulation experiments, plants were germinated and cultivated in N-free modified Fåhraeus nutrient solution (MFS, Lodeiro et al., 2000) as already described (Iturralde et al., 2019).
2.2 Isolation of soybean-nodulating rhizobia
We obtained soil samples in January 2021 from an experimental field of Facultad de Ciencias Agrarias y Forestales (Faculty of Agricultural and Forestry Sciences), UNLP, located at Los Hornos (LH), Province of Buenos Aires, Argentina (34°59´10.5” S; 58°59´58.9” W), and from the garden of one of the authors (ARL) located at Mar del Plata (MP), Province of Buenos Aires, Argentina (38°4’ 23.0” S; 57°33’34.5” W). The LH soil was chosen because it was under experimentation conducted by the Faculty of Agricultural and Forestry Sciences to evaluate different soybean inoculants and other crops systems since at least the previous 10 years before sampling whereby it was presumed that this soil would contain a soybean-nodulating allochthonous population derived from previously inoculated rhizobia (Iturralde et al., 2019). The MP soil has no previous history of soybean and, therefore, allochthonous soybean-nodulating rhizobia were not expected here. From 5 sites of each location, we collected soil samples at the vertices of a zigzag and in the middle of the plot and stored the samples in plastic bags. All samples were kept refrigerated and transported to the laboratory as soon as possible. To obtain the soybean-nodulating population from both soil samples, we extracted the bacteria from soils and used soybean as trap-plants as described (Iturralde et al., 2019). We purified the isolates by streaking in YMA plates until exhaustion and, after culturing at 28°C for approximately 5 days, we picked isolated colonies from the end of the streak, and streaked them again to obtain new colonies. We repeated this process three consecutive times. Afterwards, we inoculated soybean plants to pass the isolates through nodules and reisolated them again. Finally, we analyzed their genotypes by DNA fingerprint and determined the species by gene sequencing (see Section 2.3).
2.3 DNA extraction, gene sequencing and genotypic analysis
We isolated genomic DNA from each individual bacterial strain as described (Iturralde et al., 2019). We used the same PCR conditions, primers, and electrophoresis already described for BOX-A1R DNA-fingerprint (Iturralde et al., 2019) and analyzed each DNA fingerprint lane for the presence or absence of bands with the GelCompare II 4.0 software (Applied Maths, Kortrijk, Belgium). We optimized the band pattern with a 1.5% optimization and 1.7% tolerance. We obtained the cladogram with the unweighted pair-group method with arithmetic mean (UPGMA) (Sneath and Sokal, 1973) and evaluated the distances among branches with the Jaccard coefficient (Jaccard, 1912). For DNA sequencing, we obtained DNA fragments corresponding to 16S rRNA (V1-V9), atpD, glnII, and recA genes by PCR using the primers and amplification conditions already described (Iturralde et al., 2019). Then, we send the DNA fragments to Macrogen (Korea) for their sequencing with an ABI 3730xl DNA sequencer. We deposited the obtained sequences in GenBank with the accession numbers detailed in Supplementary Table 1. We analyzed the 16S rRNA and atpD-glnII-recA genes with DNASTAR Lasergene (DNAStar, Inc.) and compared these sequences with related sequences from GenBank with NCBI BLAST. Then, we aligned the sequences with MUSCLE and constructed phylogenetic trees using MEGA 11 with neighbor joining method (Tamura et al., 2021). For analysis of DNA sequence data, we selected the DNA evolution model using the above-mentioned software. Furthermore, we used the Tamura 3-parameter model with gamma distribution (T92+G) to obtain the cladograms for 16S rRNA and concatenated atpD-glnII-recA genes. We grouped the sequences by maximum likelihood and evaluated the support for tree nodes by bootstrap analyses with 1,000 samplings (Felsenstein, 1985; Hedges, 1992). For nosZ detection, an internal 200-bp fragment from this gene was obtained by PCR using the primers and cycle conditions already described (Itakura et al., 2013).
2.4 Analysis of plant growth-promoting activities
We assayed phosphate solubilization by measuring the ratio of the diameter of the solubilization halo to the diameter of the colony in NBRIP agar medium (Nautiyal, 1999). For siderophore production we cultured the strains in CAS (chromium azurol S) agar medium (Schwyn and Neilands, 1987) and detected siderophore production by the overlay method described by Pérez-Miranda et al. (2007). For detection and quantification of indoleacetic acid (IAA) production we centrifuged YM-grown bacteria at 8,220 × g for 20 min and filtered the supernatant through 0.22 μm cellulose filters (Millipore, USA). Then, we quantified IAA in the filtered supernatants by high-performance liquid chromatography (HPLC) using purified IAA (Sigma-Aldrich, Buenos Aires, Argentina) as standard, according to Jensen et al. (1995).
2.5 Microscopy
We heat-fixed bacterial cells to slides and carried out Gram staining with crystal violet and safranin. We washed the slides and dried them for optical microscopy, and observed isolated cells at 1000 × using a Nikon Eclipse E400 microscope.
2.6 Plant assays
To determine the most probable numbers of rhizobia in each soil sample we proceeded according to Vincent (1970). To inoculate plants for symbiotic studies, we suspended single bacterial colonies from YMA plates in 1 ml sterile MFS and deposited this suspension onto each seedling after planting in the vermiculite pots. Seven seedlings per strain were inoculated. As negative controls, we inoculated a set of plants with 1 ml of sterile MFS with neither bacteria nor externally added combined-N, and as reference we used B. japonicum E109 inoculated in separate pots. This experimental approach ensured that all N in negative control plants was from seed protein reserves, while all extra N in inoculated plants came from N2-fixation. Then, we cultured the plants for 28 days in the conditions already described. After this growth period, we separated the shoot, the root, and the nodules from each plant. We counted the total number of nodules of each plant, and then we dried all the materials at 60°C up to weight constancy. For determination of total N, we sent the dried materials to C & D Laboratory (Buenos Aires, Argentina) for Kjeldahl analysis.
2.7 Estimation of chlorophyll contents
We estimated leaf chlorophyll contents with a SPAD-502 chlorophyllometer (Minolta, Japan) reading each leaflet of a trifoliate leaf at V1 and V2 stages. Then, we combined the readings of each leaflet, and expressed the results in relative soil-plant analysis development (SPAD) units as the average for each trifoliate leaf.
2.8 Statistical analysis
For principal component analysis (PCA) we used InfoStat software (Di Rienzo et al., 2009) and for the symbiotic parameters we employed the analysis of variance (ANOVA) followed by Tukey test to identify significant mean differences with a threshold of p< 0.05 as already described (Iturralde et al., 2019). We estimated the correlations between the different symbiotic variables and chlorophyll contents by Pearson correlation using Excel. The significance of the correlation coefficients obtained was calculated using the Student´s t-test.
3 Results
3.1 Genotypic analysis and classification
We obtained 21 isolates from soil extracts using soybean as trap-plant: 16 isolates from the LH site (MPN: 7.6·103 rhizobia·g-1soil) and 5 isolates from the MP site (MPN: 6.0·101 rhizobia·g-1 soil). All isolates grew in YMA supplemented with Congo Red, producing slow-growth, white mucous colonies with smooth borders, typical of Bradyrhizobium spp. In addition, all isolates were Gram-negative rods. Genetic diversity was analyzed through BOX-A1R DNA-fingerprints (Supplementary Figure 1) and a dendrogram, based on UPGMA algorithm and Dice coefficient, was constructed using B. diazoefficiens USDA 110T, B. japonicum E109, B. elkanii USDA 76T, B. japonicum USDA 6T, B. diazoefficiens SEMIA 5080, and B. japonicum SEMIA 5079 as reference strains (Figure 1). By using a 40% similarity cutoff we grouped the isolates in three main clades. Clade I contained 10 isolates and the three B. japonicum reference strains; clade II contained 4 isolates along with the two B. diazoefficiens reference strains, and clade III grouped the remaining 7 isolates together with the B. elkanii reference strain. In addition, we analyzed the distribution of the isolates using a 70% cutoff, which was considered that may group genotypes sharing the same parental genotype in Bradyrhizobium spp. (Loureiro et al., 2007). This analysis rendered 7 groups of isolates plus 2 isolates that remained alone. Only one isolate was similar to B. diazoefficiens USDA 110 and another to B. elkanii USDA 76 according to this criterion, while no isolates displayed substantial similarity to B. japonicum E109 (Figure 1).
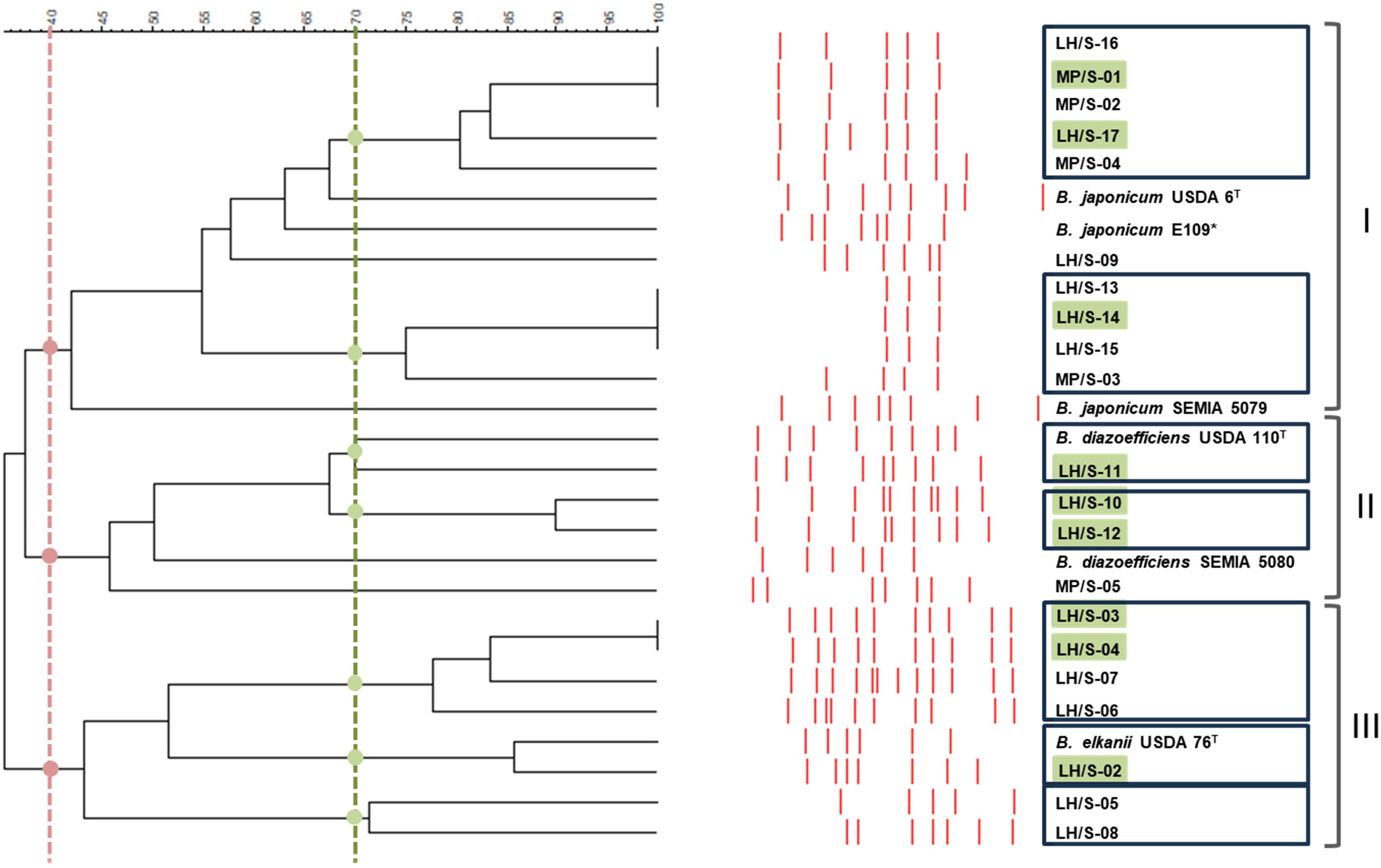
Figure 1 DNA-fingerprint of the soybean-nodulating isolates and reference strains obtained with BOX-A1R primer. Clades (numbered at the right) were defined at 40% similarity (red lines, dots, and brackets). The isolates with more than 70% similarity are presumed as sharing the same parental genotype (boxed). Isolates denoted with a shadowed box are those picked for further species identification and characterization. T indicates type strains; the asterisk indicates the reference strain.
Subsequently, we selected 9 isolates as representative of the three main clades for species determination and nosZ detection. Eight isolates were from LH showing differences in the DNA fingerprint banding pattern and one isolate was from MP.
We sequenced the 16S rRNA genes from these 9 isolates in order to achieve a taxonomic approximation. We observed that isolates LH/S-02, LH/S-03 and LH/S-04 showed a close relationship with several B. elkanii strains as well as B. jicamae PAC68T, B. valentinum LmjM3, B. icense LMTR 13T, B. embrapense SEMIA 6208T and B. lablabi CCBAU 23086T, while LH/S-10, LH/S-11 and LH/S-12 were close to B. diazoefficiens SEMIA5080 and USDA110T strains, and LH/S-14, LH/S-17 and MP/S-01 were close to B. japonicum USDA6T, SEMIA5079 and E109 strains (Figure 2). These relationships between isolates and reference species were in close agreement with those observed in the DNA fingerprint.
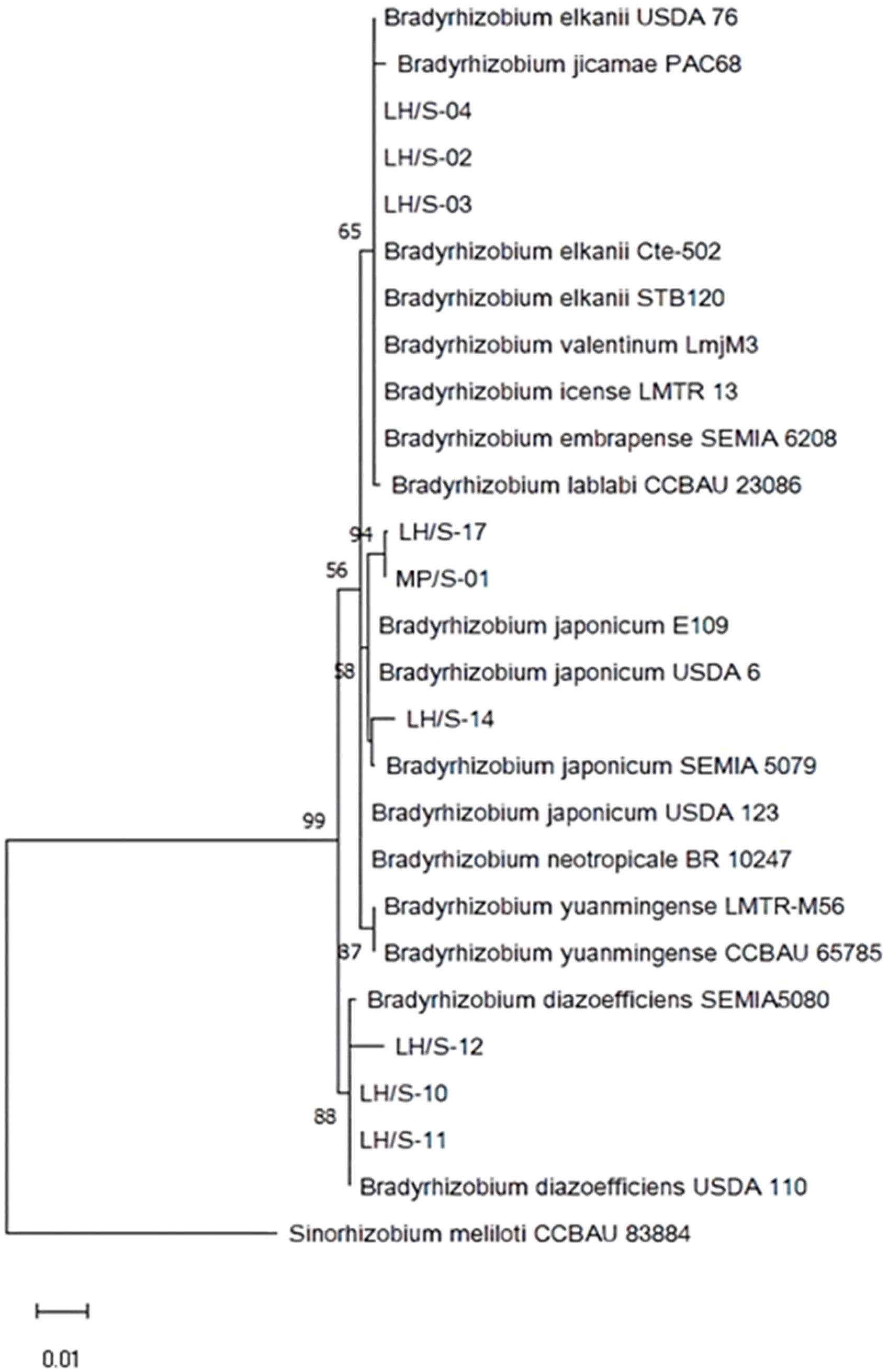
Figure 2 Maximum likelihood cladogram obtained from 16S rRNA gene of soybean-nodulating isolates and type/reference strains. Bootstrap values greater than 50% calculated for 1000 replications are indicated at internodes. Branch lengths are shown to scale, indicating evolutionary distance used to infer the phylogenetic tree. Sinorhizobium meliloti CCBAU 83884 was included as outgroup. T indicates type strain.
Since 16S ribosomal RNA gene sequences lack sufficient information to discriminate between B. diazoefficiens and B. japonicum, we compared the concatenated recA-atpD-glnII sequences to refine the phylogenetic analysis (Menna et al., 2009; Delamuta et al., 2013). We performed the multilocus sequence analysis by considering only the complete aligned sequences of the soybean-nodulating strains and the type/reference strains sequences (size in parentheses) retrieved from GenBank: 16S rRNA (1,135 bp), recA (434 bp), atpD (356 bp), glnII (282 bp). This analysis confirmed the results obtained with 16S rRNA sequence comparisons and BOX-A1R DNA fingerprinting, indicating that three of the Bradyrhizobium spp. isolates were related to B. japonicum, three to B. diazoefficiens, and the remaining three to B. elkanii (Figure 3).
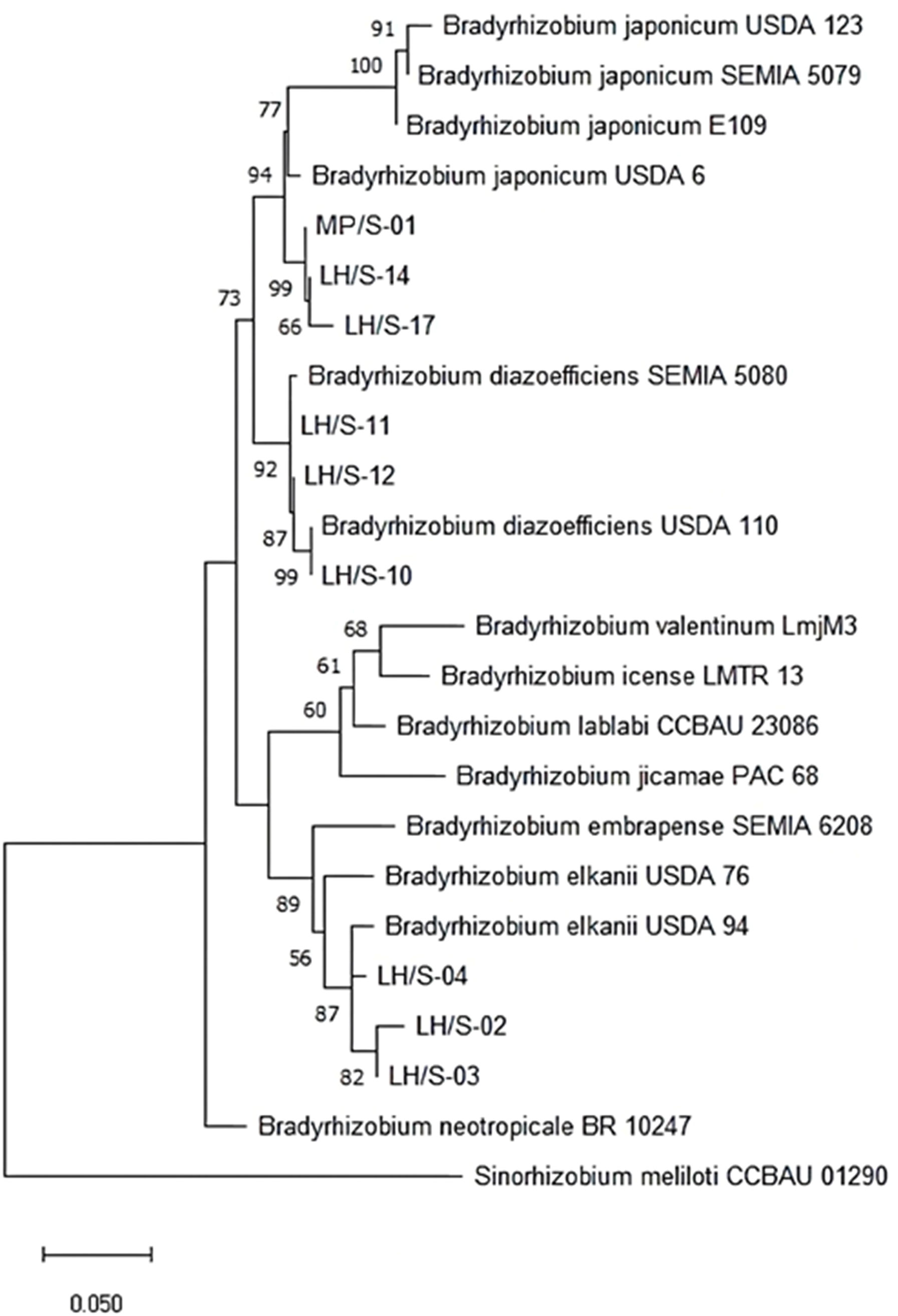
Figure 3 Maximum likelihood cladogram obtained for the concatenated atpD-glnII-recA fragment sequences to distinguish B. diazoefficiens, B. japonicum and B. elkanii among picked soybean-nodulating isolates in comparison with type/reference strains. Bootstrap values greater than 50% calculated for 1000 replications are indicated at internodes. Branch lengths are shown to scale, indicating evolutionary distance used to infer the phylogenetic tree. Sinorhizobium meliloti CCBAU 01290 was included as outgroup. T indicates type strain.
To detect the nosZ gene that encodes the N2O reductase we used two sets of primers: one specific for nosZ and the other specific for the housekeeping gene recA as positive control for the integrity of DNA templates. Our PCR analysis indicated that, as reported previously, B. diazoefficiens USDA 110 is nosZ+ since the target 200-bp nosZ fragment was amplified, while B. japonicum E109 is nosZ−. These results are in agreement with the complete genomic sequences of these strains (Kaneko et al., 2002; Torres et al., 2015). In turn, the isolates were also diverse in this respect: while the three B. diazoefficiens isolates were nosZ+, all B. japonicum and B. elkanii were nosZ− (Figure 4).
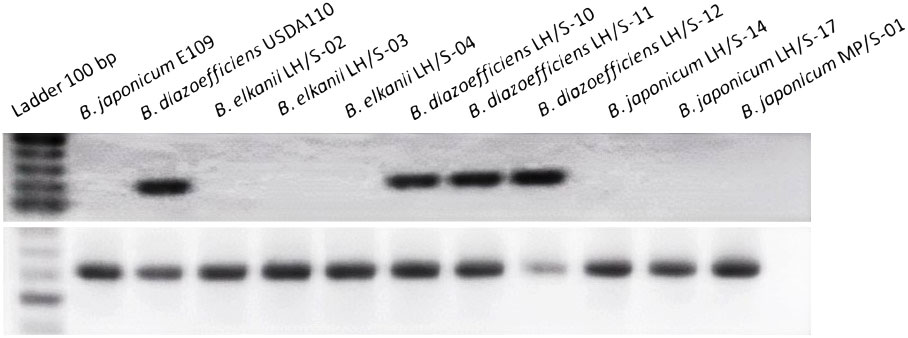
Figure 4 Detection of the 200-bp amplicon of nosZ by PCR in the soybean-nodulating field isolates (upper panel). As internal control for the integrity of the DNA templates, the housekeeping recA 650-bp amplicon was obtained (lower panel).
In summary, we observed a remarkable diversity of Bradyrhizobium strains from a relatively small soil area, which included B. diazoefficiens, B. elkanii and B. japonicum, but only the former species was positive for the presence of nosZ. In the next sections, we studied whether this genotypic diversity provides a sufficient range of plant-growth promoting and N2-fixing performances so as to calculate possible correlations with chlorophyll contents in soybean leaves.
3.2 Plant growth-promoting activities
None of the 9 selected isolates were able to solubilize phosphate or produce siderophores. To evaluate the IAA production and secretion in vitro, we measured the concentration of IAA in culture supernatants by HPLC using purified IAA as standard, and the IAA-producing strain Azospirillum argentinense Az-39 (Molina et al., 2018; Dos Santos Ferreira et al., 2022) as positive control (Supplementary Figure 2). The isolate B. elkanii LH/S-02 secreted 1.9 ± 0.86 μg · ml−1, B. elkanii LH/S-03 secreted 1.66 ± 0.39 μg · ml−1 and B. elkanii LH/S-04 secreted 0.55 ± 0.02 μg · ml−1 IAA. By comparison, A. argentinense Az39 secreted 4.95± 1.29 μg · ml−1 IAA in the same conditions. None of the other isolates were able to secrete IAA in detectable amounts. These results indicated that, among the isolates analyzed, production and secretion of IAA was a property of B. elkanii, not shared with B. diazoefficiens or B. japonicum. Therefore, the plant growth-promoting activities studied in this section are not expected to have an impact on the correlations with chlorophyll contents.
3.3 Symbiotic interaction between the selected isolates and soybean
To assess the symbiotic proficiency of our selected isolates we conducted a series of experiments in the greenhouse, where individual plants were inoculated with each isolate, a set of plants was inoculated with B. japonicum E109 as reference strain, and another set of plants was kept uninoculated. We measured the following parameters: numbers of nodules per plant, individual nodule dry weight, shoot and root fresh and dry weights, shoot/root fresh and dry weight ratios, N contents per biomass unit in dry shoots, and chlorophyll contents in leaves. The detailed results are presented in Supplementary Table 2 and here we will focus on the most relevant relationships.
To include all variables mentioned and their relationships, we performed a principal component analysis (PCA). As shown in Figure 5, the principal component (PC) 1 explained 49.1% of total variance, while the PC 2 explained 32.7%. Thus, both components rescued 81.8% of variance. The stronger correlations may be observed among the symbiotic variables individual nodule dry weight, number of nodules per plant and N contents per biomass unit, and among the biomass variables shoot dry weight and root dry weight. Both groups of variables point in the direction of PC 1, where chlorophyll contents and shoot fresh weight have the highest relationship. On the other hand, shoot/root ratio (dry) is not correlated with shoot dry weight and root dry weight, but it has correlation with the symbiotic variables individual nodule dry weight, number of nodules per plant and N contents per biomass unit. There was also no correlation between shoot fresh weight and root fresh weight, and, in addition, shoot/root ratio (fresh) was important for both PC 1 and PC 2, but nearly opposite to shoot fresh weight and root fresh weight (Figure 5).
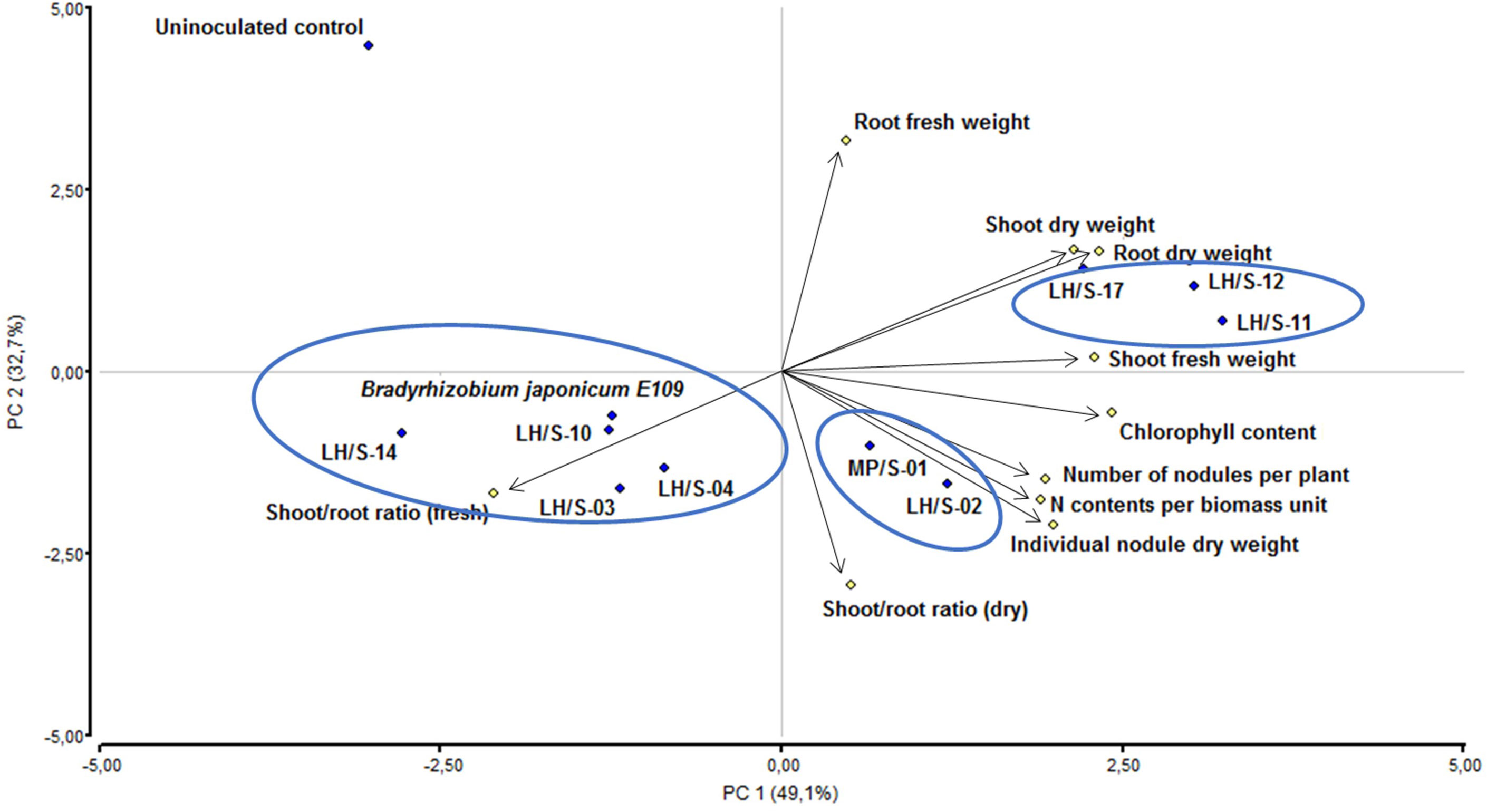
Figure 5 Principal components analysis (PCA) of number of nodules per plant, individual nodule dry weight, shoot and root fresh weight, shoot and root dry weight, shoot/root fresh and dry weight ratios, N contents per biomass unit in dry shoots, and chlorophyll contents in leaves of soybean plants inoculated with the field soybean-nodulating isolates and B. japonicum E109 as reference strain. Principal components PC 1 and PC 2 explained 49.1% and 32.7%, respectively, of the total variance.
The isolates formed three groups: one composed by B. elkanii LH/S-03 and LH/S-04, B. japonicum LH/S-14 and E109, and B. diazoefficiens LH/S-10, which was characterized by shoot/root ratio (fresh), another composed by B. diazoefficiens LH/S-11 and LH/S-12, and B. japonicum LH/S-17, which was characterized by the biomass variables shoot dry weight, root dry weight and shoot fresh weight, and the third one composed by B. elkanii LH/S-02 and B. japonicum MP/S-01, which was characterized by the symbiotic variables individual nodule dry weight, number of nodules per plant and N contents per biomass unit. These last two groups were also characterized by chlorophyll contents (Figure 5).
We calculated the total N-contents of shoots (hereafter referred to as shoot N) from N contents per biomass unit and shoot dry weight and expressed the results in mg N·plant−1, the total nodules dry weight by multiplying the number of nodules per plant by individual nodule dry weight and expressed it in mg plant−1, and finally we expressed chlorophyll contents in SPAD units. Since these plants did not receive N in the plant nutrient solution, shoot N reflects the N obtained by N2 fixation from atmosphere, while total nodules dry weight is an indicator of nodular activity. As shown in Figures 6A–C, we observed a wide diversity of chlorophyll contents, shoot N, and total nodules dry weight among the plants inoculated with the different isolates, indicating substantial differences in photosynthetic and N2-fixation capacities. This result allowed us to study the possible correlation between each of the symbiotic variables with photosynthesis, evaluated through chlorophyll contents. As shown in Figures 6D, E we indeed observed statistical significant correlations between the pairs of variables analyzed. For the pair shoot N vs chlorophyll contents the Pearson correlation coefficient was 0.81 and for total nodules dry weight vs chlorophyll contents it was 0.61 (both significant with p < 0.005).
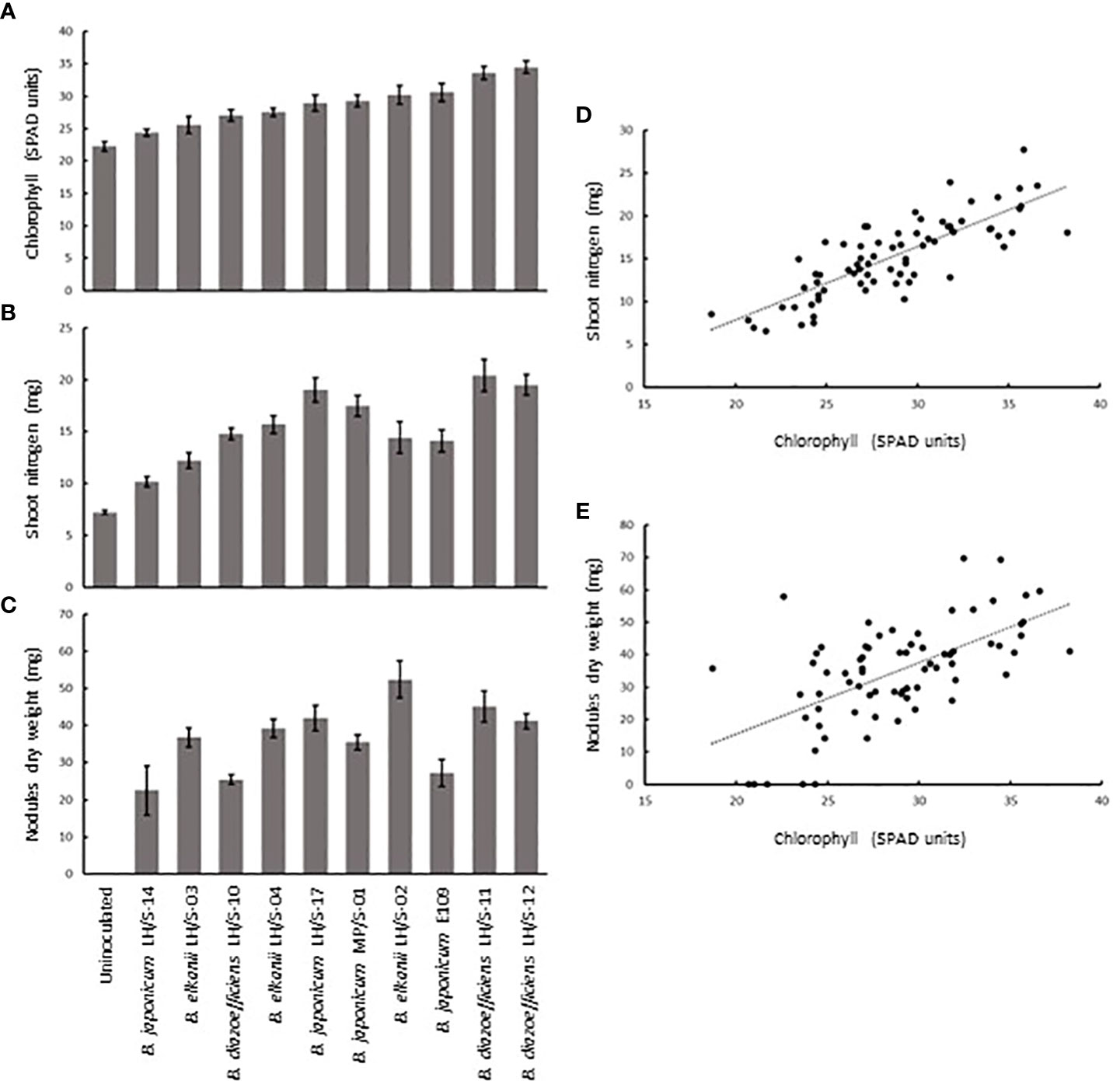
Figure 6 Diversity of symbiotic-related values and their correlations with chlorophyll contents in soybeans inoculated with the different soybean-nodulating field isolates, B. japonicum E109 as reference strain, and an uninoculated control. (A) Chlorophyll contents in leaves. (B) Shoot nitrogen accumulated per plant. (C) Total nodules dry weight per plant. (D) Correlation between shoot nitrogen and chlorophyll contents. (E) Correlation between total nodules dry weight and chlorophyll contents. Error bars in (A-C) are standard errors of the mean. In (D), r = 0.81. In (E), r = 0.61. Both correlation coefficients were significant with p< 0.005.
If we order the isolates in thirds from higher to lower value (excluding B. japonicum E109), we can appreciate that the top third as regards shoot N is integrated by B. diazoefficiens LH/S-11 (1st), B. diazoefficiens LH/S-12 (2nd) and B. japonicum LH/S-17 (3rd), while the top third as regards total nodules dry weight contains B. elkanii LH/S-02 (1st), B. diazoefficiens LH/S-11 (2nd), and B. diazoefficiens LH/S-12 (3rd). On the other hand, the top third as regards chlorophyll contents is composed by B. diazoefficiens LH/S-12 (1st), B. diazoefficiens LH/S-11 (2nd), and B. elkanii LH/S-02 (3rd). By comparing with the PCA in Figure 5, we can see that the isolates LH/S-11, LH/S-12, and LH/S-17 are situated in the same region, characterized by biomass production variables, while LH/S-02 is characterized by symbiotic variables. Hence, the measurement of chlorophyll contents using the chlorophyllometer correctly identified B. diazoefficiens LH/S-11 and B. diazoefficiens LH/S-12 as the most prominent isolates regarding their symbiotic potential among the 9 isolates analyzed. Therefore, this may serve as a simple, non-destructive method for estimating N2-fixation at laboratory scale for quality control protocols at inoculants industry.
4 Discussion
By using soybean as trap-plant we obtained 21 isolates from soil samples taken at Los Hornos (LH) and Mar del Plata (MP), two locations separated by 370 km in the Province of Buenos Aires, Argentina. Soybean was cultivated in LH for experimental purposes since the last 10 years, while the MP samples come from a garden where soybean was never planted. By using BOX-A1R DNA fingerprint we grouped these isolates in three genotypic clades, each one represented by reference strains of B. japonicum (clade I), B. diazoefficiens (clade II) and B. elkanii (clade III). Then, we determined the species to which 9 selected isolates belong by sequencing the 16S rRNA, recA, atpD, and glnII genes. By comparing this information with the genotypical distribution among the clades, a good agreement for species determination was observed among all these genetic classification criteria (see Figures 1–3). The origin of LH isolates may be the inoculants applied years before, although the genotypic pattern of B. japonicum E109, the most used strain in inoculants in Argentina, was not represented among the isolated genotypes. In turn, the source of MP isolates is unclear, although the most plausible explanation is that they may originate from bacteria inadvertently carried by the author owner of this garden. Anyway, these results underscore the high adaptability of Bradyrhizobium spp. to soils, both from the point of view of its persistence in soil and genotypic plasticity, as reported previously in Brazilian soils (Loureiro et al., 2007). The MP/S-01 strain that we choose for the studies has a DNA fingerprint band pattern very similar to LH/S-14, but they differed in symbiotic behavior. While LH/S-14 was among the worst as revealed by PCA, shoot N accumulation and chlorophyll contents, this isolate was curious in that it produced very few nodules, although with high individual nodule dry weight. Nodule size is normally related with nodular activity, while number of nodules is regulated by the host plant in a process known as autoregulation of nodulation, which is mediated by plant signals that include CLE peptides and microRNA (Zhang et al., 2021). In this process, the plant progressively inhibits new nodulation after the first nodules were formed, according to their activity. If individual nodules formed by LH/S-14 are abnormally highly active —as suggested by their size— autoregulation of nodulation might act soon to render a very low number of nodules per plant, which together are not enough for a substantial amount of N fixed. By contrast, MP/S-01 had a behavior not so bad, being the 4th among the isolates in shoot N and chlorophyll contents and the 7th in total nodules dry weight (for details, see Supplementary Table 2). In addition, there were 7 single nucleotide differences between the 16S rDNA sequences obtained from LH/S-14 and MP/S-01 PCR products (not shown), whereby the similarity in DNA fingerprint band pattern observed between these isolates seems not to indicate genetic identity. At present, the genetic bases of the different behaviors between these isolates regarding nodulation are uncertain.
The genetic diversity observed among our isolates was reflected in disparate symbiotic performances, especially regarding total nodules dry weight and shoot N. The presence of such a diversity in symbiotic performance of local isolates was already described (Damirgi et al., 1967; Ellis et al., 1984; Keyser and Cregan, 1987; Hirsch, 1996; Rodríguez-Echeverría et al., 2003; Abaidoo et al., 2007; Melchiorre et al., 2011; Iturralde et al., 2019), and set the basis for the hypothesis that local strains raise a competitive barrier to nodulation by elite inoculant strains (Dowling and Broughton, 1986; Triplett and Sadowsky, 1992; Pérez-Giménez et al., 2011; Checcucci et al., 2017; Onishchuk et al., 2017; Mendoza-Suárez et al., 2021). Moreover, we confirmed the previous observations that elite genotypes are absent from local populations, even if the elite strains are continuously used as inoculants, indicating that they possess a poor adaptability to local soil conditions (Loureiro et al., 2007; Melchiorre et al., 2011; Iturralde et al., 2019). Therefore, it seems that the fitness landscape of bradyrhizobia in soils is complex, and might include adaptation to soil properties, to local microbiota, and to host plants.
The diversity of symbiotic performance enabled us to search for correlations between symbiotic parameters and chlorophyll contents. We observed high correlations between shoot N and chlorophyll contents as well as total nodules dry weight and chlorophyll contents, both of which, along with PCA, allowed the identification of B. diazoefficiens LH/S-11 and LH/S-12 as the most promising isolates. These correlations might be due to the fact that, in our experimental setup, plants were cultivated in N-free nutrient solution, and therefore were forced to acquire all their N from N2-fixation. Given that leaf N is primarily allocated to the photosynthetic apparatus (Evans and Clarke, 2019), a correlation between N2-fixation and photosynthesis is manifested. This correlation allowed us to setup a laboratory protocol for the estimation of N2-fixation by means of chlorophyll measurements with a portable chloropyllometer, a simple and non-destructive method.
Since differences in performing the whole denitrification process from oxidized N-compounds to N2 are known between the different Bradyrhizobium species (Siqueira et al., 2017; Obando et al., 2019; Mania et al., 2020), we also looked for the presence of the nosZ gene encoding the N2O reductase in our isolates as an additional and independent quality criterion. In agreement with previous reports (Siqueira et al., 2017; Obando et al., 2019), we observed that the three B. diazoefficiens isolates possessed at least one copy of nosZ, while the B. elkanii and B. japonicum isolates did not. Therefore, we may expect that inoculation of soybean with B. diazoefficiens LH/S-11 and LH/-12 may prevent N2O emissions caused by the rhizobia from this crop, and even reduce such emissions if the inoculation technology fosters high nodules occupation by the inoculated strain in competition with soybean-nodulating strains from the soil microbiota (López-García, 2009; Akiyama et al., 2016).
The B. elkanii isolate LH/S-02 was the only one of this species that possessed a good symbiotic performance, near B. diazoefficiens LH/S-11 and LH/S-12. This isolate shared with the other two B. elkanii the property of producing and secreting IAA, which was not detected in the other species tested here. In addition, LH/S-02 was the top producer of IAA, although at less than half the level of A. argentinense Az39, which is a well-known plant growth promoting rhizobacterium in part due to this capacity (Molina et al., 2018). Nevertheless, IAA production and secretion were observed in vitro, whereby we cannot extrapolate it to the situation in the rhizosphere or inside plant tissues (e.g., infection threads or nodules), but, since IAA was regarded as participating in nodule development (Gao et al., 2021), the possibility exists that the good performance of LH/S-02 be due in part to this capability. On the other hand, none of the isolates produced siderophores or solubilized phosphate at appreciable levels, by difference with other Bradyrhizobium spp. field isolates reported elsewhere (Ibny et al., 2019; Bünger et al., 2021; Bharti et al., 2023; Lamrabet et al., 2023).
5 Conclusion
The symbiotic performance of a soybean crop growing in a soil populated by local, allochtonous genotypes of soybean-nodulating rhizobia would depend on the local strains more than on the elite strains inoculated at sowing. Therefore, it is advisable to use good strains adapted to the local conditions instead of a single foreign elite strain. Our results suggest that, to select and assess the quality of such strains, the use of a chlorophyllometer and the PCR-determination of the presence of nosZ are advisable methods to incorporate in laboratory tests for routine quality control protocols at the inoculants industry. On the one hand, the chlorophyllometer is a small, portable equipment with a moderate cost and no need for consumables, which makes it suitable for small and medium-sized inoculants enterprises, and on the other hand, PCR is already a routine method in most laboratories and inoculants industries. Therefore, these methods may allow to select and cultivate new strains on the basis of their quality as N2-fixers and their safety as full denitrifiers without impacting on the inoculants production cost. Hence, selecting strains from local populations and adding these two techniques to the routine laboratory analysis of inoculants quality are three simple measures that may render substantial benefits for profiting N2-fixation while, at the same time, mitigating N2O greenhouse gas emissions. Undertaking this simple strategy may positively impact on soil fertility-sustainable and climate-friendly soybean agriculture, while maintaining the low cost of inoculation.
Data availability statement
The datasets presented in this study can be found online at https://sedici.unlp.edu.ar. The accession number(s) can be found in the Supplementary Material.
Author contributions
DB: Data curation, Formal analysis, Investigation, Methodology, Validation, Visualization, Writing – review & editing. EF: Investigation, Methodology, Validation, Visualization, Writing – review & editing. TS: Investigation, Methodology, Writing – review & editing. RB: Investigation, Methodology, Writing – review & editing. CC: Conceptualization, Investigation, Methodology, Validation, Visualization, Writing – review & editing. EM: Conceptualization, Investigation, Methodology, Validation, Writing – review & editing. JP-G: Conceptualization, Investigation, Methodology, Supervision, Validation, Visualization, Writing – review & editing. AL: Conceptualization, Funding acquisition, Investigation, Methodology, Project administration, Resources, Supervision, Validation, Visualization, Writing – original draft, Writing – review & editing.
Funding
The author(s) declare financial support was received for the research, authorship, and/or publication of this article. This work was supported by Agencia Nacional de Promoción de la Investigación, el Desarrollo Tecnológico y la Innovación (Argentina) [grant number PICT2020-3911]. The funder had no role in study design; in the collection, analysis and interpretation of data; in the writing of the report; and in the decision to submit the article for publication.
Acknowledgments
The authors are grateful to Luciana Cayuela and Silvana Stongiani for laboratory technical assistance, Ulises Mancini for help with revision of DNA sequences, and Claudio Mazo for assistance in plant experiments. DB, EF-C, TS, and RB are fellows of CONICET (Argentina). CC, EM, JP-G and AL are members of the Scientific Researcher Career of CONICET (Argentina). The content of this manuscript may be found online as preprint in BioRxiv: https://doi.org/10.1101/2023.10.26.564198.
Conflict of interest
The authors declare that the research was conducted in the absence of any commercial or financial relationships that could be construed as a potential conflict of interest.
Publisher’s note
All claims expressed in this article are solely those of the authors and do not necessarily represent those of their affiliated organizations, or those of the publisher, the editors and the reviewers. Any product that may be evaluated in this article, or claim that may be made by its manufacturer, is not guaranteed or endorsed by the publisher.
Supplementary material
The Supplementary Material for this article can be found online at: https://www.frontiersin.org/articles/10.3389/fagro.2024.1336433/full#supplementary-material
Supplementary Figure 1 | Genotypic characterization of the soybean-nodulating isolates.
Supplementary Figure 2 | HPLC chromatogram of cellular extracts from the B. elkanii isolates.
References
Abaidoo R. C., Keyser H. H., Singleton P. W., Dashiell K. E., Sanginga N. (2007). Population size, distribution, and symbiotic characteristics of indigenous Bradyrhizobium spp. that nodulate TGx soybean genotypes in Africa. Appl. Soil Ecol. 35, 57–67. doi: 10.1016/j.apsoil.2006.05.006
Adams M. A., Buckley T. N., Salter W. T., Buchmann N., Blessing. C. H., Turnbull T. L. (2018). Contrasting responses of crop legumes and cereals to nitrogen availability. New Phytol. 217, 1475–1483. doi: 10.1111/nph.14918
Akiyama H., Hoshino Y. T., Itakura M., Shimomura Y., Wang Y., Yamamoto A., et al. (2016). Mitigation of soil N2O emission by inoculation with a mixed culture of indigenous Bradyrhizobium diazoefficiens. Sci. Rep. 6, 32869. doi: 10.1038/srep32869
Aryal B., Gurung R., Camargo A. F., Fongaro G., Treichel H., Mainali B., et al. (2022). Nitrous oxide emission in altered nitrogen cycle and implications for climate change. Environ. pollut. 314, 120272. doi: 10.1016/j.envpol.2022.120272
Bharti A., Maheshwari H. S., Garg S., Anwar K., Pareek A., Satpute G., et al. (2023). Exploring potential soybean bradyrhizobia from high trehalose-accumulating soybean genotypes for improved symbiotic effectiveness in soybean. Int. Microbiol. 26, 973–987. doi: 10.1007/s10123-023-00351-3
Boon-Long P., Egli D. B., Leggett J. E. (1983). Leaf N and photosynthesis during reproductive growth in soybeans. Crop Sci. 23, 617–620. doi: 10.2135/cropsci1983.0011183X002300040005x
Boote K. J., Gallaher R. N., Robertson W. K., Hinson K., Hammond L. C. (1978). Effect of foliar fertilization on photosynthesis, leaf nutrition, and yield of soybeans. Agron. J. 70, 787–791. doi: 10.2134/agronj1978.00021962007000050022x
Bünger W., Sarkar A., Grönemeyer J. L., Zielinski J., Revermann R., Hurek T., et al. (2021). Root nodule rhizobia from undomesticated shrubs of the dry woodlands of Southern Africa can nodulate Angolan teak Pterocarpus angolensis, an important source of timber. Front. Microbiol. 12. doi: 10.3389/fmicb.2021.611704
Burris R. H. (1972). Nitrogen fixation-assay methods and techniques. Methods Enzymol. 24, 415–431. doi: 10.1016/0076-6879(72)24088-5
Buttery B. R., Buzzell R. I. (1988). Soybean leaf nitrogen in relation to photosynthetic rate and yield. Can. J. Plant Sci. 68, 793–795. doi: 10.4141/cjps88-092
Carter K. R., Jennings N. T., Hanus J., Evans H. J. (1978). Hydrogen evolution and uptake by nodules of soybeans inoculated with different strains of Rhizobium japonicum. Can. J. Microbiol. 24, 307–311. doi: 10.1139/m78-051
Castelli F., Contillo R., Miceli F. (1996). Non-destructive determination of leaf chlorophyll content in four crop species. J. Agron. Crop Sci. 177, 275–283. doi: 10.1111/j.1439-037X.1996.tb00246.x
Chalk P. M., Ladha J. K. (1999). Estimation of legume symbiotic dependence: an evaluation of techniques based on 15N dilution. Soil Biol. Biochem. 31, 1901–1917. doi: 10.1016/S0038-0717(99)00095-4
Checcucci A., DiCenzo G. C., Bazzicalupo M., Mengoni A. (2017). Trade, diplomacy, and warfare: the quest for elite rhizobia inoculant strains. Front. Microbiol. 8. doi: 10.3389/fmicb.2017.02207
Collino D. J., Salvagiotti F., Perticari A., Piccinetti C., Ovando G., Urquiaga S., et al. (2015). Biological nitrogen fixation in soybean in Argentina: relationships with crop, soil, and meteorological factors. Plant Soil 392, 239–252. doi: 10.1007/s11104-015-2459-8
Damirgi S. M., Frederick L. R., Anderson I. C. (1967). Serogroups of Rhizobium japonicum in soybean nodules as affected by soil types. Agron. J. 59, 10–12. doi: 10.2134/agronj1967.00021962005900010004xW
Delamuta J. R. M., Ribeiro R. A., Ormeño-Orrillo E., Melo I. S., Martínez-Romero E., Hungria M. (2013). Polyphasic evidence supporting the reclassification of Bradyrhizobium japonicum group Ia strains as Bradyrhizobium diazoefficiens sp. nov. Int. J. Syst. Evol. Microbiol. 63, 3342–3351. doi: 10.1099/ijs.0.049130-0
de Souza G. K., Sampaio J., Longoni L., Ferreira S., Alvarenga S., Beneduzi A. (2019). Soybean inoculants in Brazil: an overview of quality control. Braz. J. Microbiol. 50, 205–211. doi: 10.1007/s42770-018-0028-z
Di Rienzo J. A., Casanoves F., Balzarini M. G., González L., Tablada M., Robledo C. W. (2009) InfoStat, Universidad Nacional de Córdoba, Argentina. Available at: https://www.infostat.com.ar/index.php?mod=page&id=15 (Accessed 14 May 2023).
Dos Santos Ferreira N., Coniglio A., Puente M., Sant'Anna F. H., Maroniche G., García J., et al. (2022). Genome-based reclassification of Azospirillum brasilense Az39 as the type strain of Azospirillum argentinense sp. nov. Int. J. Syst. Evol. Microbiol. 72, 005475. doi: 10.1099/ijsem.0.005475
Dowling D. N., Broughton W. J. (1986). Competition for nodulation of legumes. Annu. Rev. Microbiol. 40, 131–157. doi: 10.1146/annurev.mi.40.100186.001023
Ellis R., Ham G. E., Schmidt E. L. (1984). Persistence and recovery of Rhizobium japonicum inoculum in a field soil. Agron. J. 76, 573–576. doi: 10.2134/agronj1984.00021962007600040015x
Erisman J. W., Galloway J. N., Seitzinger S., Bleeker A., Dise N. B., Petrescu A. R., et al. (2013). Consequences of human modification of the global nitrogen cycle. Philos. Trans. R. Soc 368, 20130116. doi: 10.1098/rstb.2013.0116
Evans J. R., Clarke V. C. (2019). The nitrogen cost of photosynthesis. J. Exp. Bot. 70, 7–15. doi: 10.1093/jxb/ery366
Felsenstein J. (1985). Confidence limits on phylogenies: an approach using the bootstrap. Evolution 39, 783–791. doi: 10.1111/j.1558-5646.1985.tb00420.x
Fritschi F. B., Ray J. D. (2007). Soybean leaf nitrogen, chlorophyll content, and chlorophyll a/b ratio. Photosynthetica 45, 92–98. doi: 10.1007/s11099-007-0014-4
Gao Z., Chen Z., Cui Y., Ke M., Xu H., Xu Q., et al. (2021). GmPIN-dependent polar auxin transport is involved in soybean nodule development. Plant Cell 33, 2981–3003. doi: 10.1093/plcell/koab183
Hedges S. B. (1992). The number of replications needed for accurate estimation of the bootstrap p-value in phylogenetic studies. Mol. Biol. Evol. 9, 366–369. doi: 10.1093/oxfordjournals.molbev.a040725
Herridge D. F. (1982). Relative abundance of ureides and nitrate in plant tissues of soybean as a quantitative assay of nitrogen fixation. Plant Physiol. 70, 1–6. doi: 10.1104/pp.70.1.1
Herridge D. F., Hartley E., Gemell L. G. (2014). Rhizobial counts in peat inoculants vary among legume inoculant groups at manufacture and with storage: implications for quality standards. Plant Soil 380, 327–336. doi: 10.1007/s11104-014-2087-8
Herrmann L., Lesueur D. (2013). Challenges of formulation and quality of biofertilizers for successful inoculation. Appl. Microbiol. Biotechnol. 97, 8859–8873. doi: 10.1007/s00253-013-5228-8
Hirsch P. R. (1996). Population dynamics of indigenous and genetically modified rhizobia in the field. New Phytol. 133, 159–171. doi: 10.1111/j.1469-8137.1996.tb04351.x
Ibny F. Y. I., Jaiswal S. K., Mohammed M., Dakora F. D. (2019). Symbiotic effectiveness and ecologically adaptive traits of native rhizobial symbionts of Bambara groundnut (Vigna subterranea L. Verdc.) in Africa and their relationship with phylogeny. Sci. Rep. 9, 12666. doi: 10.1038/s41598-019-48944-1
Imran A., Hakim S., Tariq M., Nawaz M. S., Laraib I., Gulzar U., et al. (2021). Diazotrophs for lowering nitrogen pollution crises: looking deep into the roots. Front. Microbiol. 12. doi: 10.3389/fmicb.2021.637815
Itakura M., Uchida Y., Akiyama H., Hoshino Y. T., Shimomura Y., Morimoto S., et al. (2013). Mitigation of nitrous oxide emissions from soils by Bradyrhizobium japonicum inoculation. Nat. Clim. Change 3, 208–212. doi: 10.1038/nclimate1734
Iturralde E. T., Covelli J. M., Alvarez F., Pérez-Giménez J., Arrese-Igor C., Lodeiro A. R. (2019). Soybean-nodulating strains with low intrinsic competitiveness for nodulation, good symbiotic performance, and stress-tolerance isolated from soybean-cropped soils in Argentina. Front. Microbiol. 10. doi: 10.3389/fmicb.2019.01061
Jaccard P. (1912). The distribution of flora in the alpine zone. New Phytol. 11, 37–50. doi: 10.1111/j.1469-8137.1912.tb05611.x
Jensen J. B., Egsgaard H., Van Onckelen H., Jochimsen B. U. (1995). Catabolism of indole-3-acetic acid and 4-and 5-chloroindole-3-acetic acid in Bradyrhizobium japonicum. J. Bacteriol. 177, 5762–5766. doi: 10.1128/jb.177.20.5762-5766.1995
Kaneko T., Nakamura Y., Sato S., Minamisawa K., Uchiumi T., Sasamoto S., et al. (2002). Complete genomic sequence of nitrogen-fixing symbiotic bacterium Bradyrhizobium japonicum USDA110. DNA Res. 9, 189–197. doi: 10.1093/dnares/9.6.189
Keyser H. H., Cregan P. B. (1987). Nodulation and competition for nodulation of selected soybean genotypes among Bradyrhizobium japonicum serogroup 123 isolates. Appl. Environ. Microbiol. 53, 2631–2635. doi: 10.1128/aem.53.11.2631-2635.1987
Lamrabet M., Chaddad Z., Bouhnik O., Alami S., Kaddouri K., Bennis M., et al. (2023). Different species of Bradyrhizobium from symbiovars genistearum and retamae nodulate the endemic Retama dasycarpa in the High Atlas Mountains. FEMS Microbiol. Ecol. 99, fiad038. doi: 10.1093/femsec/fiad038
Lodeiro A. R., González P., Hernández A., Balagué L. J., Favelukes G. (2000). Comparison of drought tolerance in nitrogen-fixing and inorganic nitrogen-grown common beans. Plant Sci. 154, 31–41. doi: 10.1016/s0168-9452(99)00246-0
López García S. L., Perticari A., Piccinetti C., Ventimiglia L., Arias N., De Battista J. J., et al (2009). In-furrow inoculation and selection for higher motility enhances the efficacy of Bradyrhizobium japonicum nodulation. Agron. J. 101, 357–363. doi: 10.2134/agronj2008.0155x
Loureiro M., Kaschuk G., Alberton O., Hungria M. (2007). Soybean [Glycine max (L.) Merrill] rhizobial diversity in Brazilian oxisols under various soil, cropping, and inoculation managements. Biol. Fert. Soils 43, 665–674. doi: 10.1007/s00374-006-0146-x
Lugg D. G., Sinclair T. R. (1981). Seasonal changes in photosynthesis of field-grown soybean leaflets. 2. Relation to nitrogen content. Photosynthetica 15, 138–144.
Ma B. L., Morrison M. J., Voldeng H. D. (1995). Leaf greenness and photosynthetic rates of soybean. Crop Sci. 35, 1411–1414. doi: 10.2135/cropsci1995.0011183X003500050025x
Mania D., Woliy K., Degefu T., Frostegård Å. (2020). A common mechanism for efficient N2O reduction in diverse isolates of nodule-forming bradyrhizobia. Environ.Microbiol. 22, 17–31. doi: 10.1111/1462-2920.14731
Markwell J., Osterman J. C., Mitchell J. L. (1995). Calibration of the Minolta SPAD-502 leaf chlorophyll meter. Photosynth. Res. 46, 467–472. doi: 10.1007/BF00032301
Melchiorre M., de Luca M. J., González-Anta G., Suárez P., López C., Lascano R., et al. (2011). Evaluation of bradyrhizobia strains isolated from field-grown soybean plants in Argentina as improved inoculants. Biol. Fert. Soils 47, 81–89. doi: 10.1007/s00374-010-0503-7
Mendoza-Suárez M., Andersen S. U., Poole P. S., Sánchez-Cañizares C. (2021). Competition, nodule occupancy, and persistence of inoculant strains: key factors in the Rhizobium-legume symbioses. Front. Plant Sci. 12. doi: 10.3389/fpls.2021.690567
Menna P., Barcellos F. G., Hungria M. (2009). Phylogeny and taxonomy of a diverse collection of Bradyrhizobium strains based on multilocus sequence analysis of the 16S rRNA gene, ITS region and glnII, recA, atpD and dnaK genes. Int. J. Syst. Evol. Microbiol. 59, 2934–2950. doi: 10.1099/ijs.0.009779-0
Mesa S., Bedmar E. J., Chanfon A., Hennecke H., Fischer H. M. (2003). Bradyrhizobium japonicum NnrR, a denitrification regulator, expands the FixLJ-FixK2 regulatory cascade. J. Bacteriol. 185, 3978–3982. doi: 10.1128/JB.185.13.3978-3982.2003
Minchin F. R., Witty J. F. (1989). “Limitations and errors in gas exchange measurements with legume nodules,” in Applications of Continuous and Steady-State Methods to Root Biology. Developments in Plant and Soil Sciences, vol. 34 . Eds. Torrey J. G., Winship L. J. (Dordrecht: Springer), 79–95. doi: 10.1007/978-94-009-2237-2_5
Molina R., Rivera D., Mora V., López G., Rosas S., Spaepen S., et al. (2018). Regulation of IAA biosynthesis in Azospirillum brasilense under environmental stress conditions. Curr. Microbiol. 75, 1408–1418. doi: 10.1007/s00284-018-1537-6
Moreira A., Moraes L. A. C., Schroth G., Mandarino J. M. G. (2015). Effect of nitrogen, row spacing, and plant density on yield, yield components, and plant physiology in soybean-wheat intercropping. Agron. J. 107, 2162–2170. doi: 10.2134/agronj15.0121
Nautiyal C. S. (1999). An efficient microbiological growth medium for screening phosphate solubilizing microorganisms. FEMS Microbiol. Lett. 170, 265–270. doi: 10.1111/j.1574-6968.1999.tb13383.x
Obando M., Correa-Galeote D., Castellano-Hinojosa A., Gualpa J., Hidalgo A., Alché J. D., et al. (2019). Analysis of the denitrification pathway and greenhouse gases emissions in Bradyrhizobium sp. strains used as biofertilizers in South America. J. Appl. Microbiol. 127, 739–749. doi: 10.1111/jam.14233
Onishchuk O. P., Vorobyov N. I., Provorov N. A. (2017). Nodulation competitiveness of nodule bacteria: Genetic control and adaptive significance. Appl. Biochem. Microbiol. 53, 131–139. doi: 10.1134/S0003683817020132
Penna C., Massa R., Olivieri F., Gutkind G., Cassán F. (2011). A simple method to evaluate the number of bradyrhizobia on soybean seeds and its implication on inoculant quality control. AMB Express. 1, 21. doi: 10.1186/2191-0855-1-21
Pérez-Giménez J., Quelas J. I., Lodeiro A. R. (2011). “Competition for nodulation,” in Soybean/Book 3. Physiology and Biochemistry. Ed. El-Shemy H. A. (Rijeka, Croatia: InTech Open Access Publisher), 139–166. doi: 10.5772/18034
Pérez-Miranda S., Cabirol N., George-Téllez R., Zamudio-Rivera L. S., Fernández F. J. (2007). O-CAS, a fast and universal method for siderophore detection. J. Microbiol. Methods 70, 127–131. doi: 10.1016/j.mimet.2007.03.023
Rodríguez-Echeverría S., Pérez-Fernández M. A., Vlaar S., Finnan T. (2003). Analysis of the legume-rhizobia symbiosis in shrubs from central western Spain. J. Appl. Microbiol. 95, 1367–1374. doi: 10.1046/j.1365-2672.2003.02118.x
Schwyn B., Neilands J. (1987). Universal chemical assay for detection and determination of siderophores. Anal. Biochem. 160, 47–56. doi: 10.1016/0003-2697(87)90612-9
Siqueira A. F., Minamisawa K., Sánchez C. (2017). Anaerobic reduction of nitrate to nitrous oxide is lower in Bradyrhizobium japonicum than in Bradyrhizobium diazoefficiens. Microbes Environ. 32, 398–401. doi: 10.1264/jsme2.ME17081
Tamura K., Stecher G., Kumar S. (2021). MEGA11: molecular evolutionary genetics analysis version 11. Mol. Biol. Evol. 38, 3022–3027. doi: 10.1093/molbev/msab120
Tcherkez G. G., Farquhar G. D., Andrews T. J. (2006). Despite slow catalysis and confused substrate specificity, all ribulose bisphosphate carboxylases may be nearly perfectly optimized. Proc. Natl. Acad. Sci. U.S.A. 103, 7246–7251. doi: 10.1073/pnas.0600605103
Torres D., Revale S., Obando M., Maroniche G., Paris G., Perticari A., et al. (2015). Genome sequence of Bradyrhizobium japonicum E109, One of the most agronomically used nitrogen-fixing rhizobacteria in Argentina. Genome Announc. 3, e01566–14. . doi: 10.1128/genomeA.01566-14
Triplett E. W., Sadowsky M. J. (1992). Genetics of competition for nodulation of legumes. Annu. Rev. Microbiol. 46, 399–428. doi: 10.1146/annurev.mi.46.100192.002151
Uddling J., Gelang-Alfredsson J., Piiki K., Pleijel H. (2007). Evaluating the relationship between leaf chlorophyll concentration and SPAD-502 chlorophyll meter readings. Photosynth. Res. 91, 37–46. doi: 10.1007/s11120-006-9077-5
Vincent J. M. (1970). “A manual for the practical study of root-nodule bacteria,” in International Biological Programme (Oxford: Blackwell Scientific).
Witty J. F., Minchin F. R. (1998). Methods for the continuous measurement of O2 consumption and H2 production by nodulated legume root systems. J. Exp. Bot. 49, 1041–1047. doi: 10.1093/jxb/49.323.1041
Keywords: Bradyrhizobium, soybean, chlorophyllometer, nitrogen fixation, nitrous oxide
Citation: Brignoli D, Frickel-Critto E, Sandobal TJ, Balda RS, Castells CB, Mongiardini EJ, Pérez-Giménez J and Lodeiro AR (2024) Quality control of Bradyrhizobium inoculant strains: detection of nosZ and correlation of symbiotic efficiency with soybean leaf chlorophyll levels. Front. Agron. 6:1336433. doi: 10.3389/fagro.2024.1336433
Received: 15 November 2023; Accepted: 02 January 2024;
Published: 22 January 2024.
Edited by:
Mohammad Bagher Hassanpouraghdam, University of Maragheh, IranReviewed by:
Kailash Chand Kumawat, Sam Higginbottom University of Agriculture, Technology and Sciences, IndiaJohn M. Maingi, Kenyatta University, Kenya
Becky Nancy Aloo, University of Eldoret, Kenya
Copyright © 2024 Brignoli, Frickel-Critto, Sandobal, Balda, Castells, Mongiardini, Pérez-Giménez and Lodeiro. This is an open-access article distributed under the terms of the Creative Commons Attribution License (CC BY). The use, distribution or reproduction in other forums is permitted, provided the original author(s) and the copyright owner(s) are credited and that the original publication in this journal is cited, in accordance with accepted academic practice. No use, distribution or reproduction is permitted which does not comply with these terms.
*Correspondence: Aníbal R. Lodeiro, bG9kZWlyb0BiaW9sLnVubHAuZWR1LmFy