- 1Plant Interaction Laboratory, Department of Biological and Forensic Sciences, Fayetteville State University, Fayetteville, NC, United States
- 2U.S. Department of Agriculture (USDA)-Agricultural Research Service (ARS) and Department of Entomology, Kansas State University, Manhattan, KS, United States
- 3Jack Britt High School, Fayetteville, NC, United States
The Hessian fly (HF, Mayetiola destructor) is one of the most destructive pests of wheat and wheat-related cereals. Wheat resistance and/or susceptibility to HF are often affected by the levels of phytohormones in plants. In this study, we tested the impact of phytohormones on Molly wheat resistance to HF biotype GP by externally applying phytohormones, including salicylic acid (SA), jasmonic acid (JA), 12-oxophytodienoic acid (OPDA), and auxin (indole acetic acid, IAA) to wheat seedlings under heat conditions. Our results indicated that the impact of externally applied phytohormones on wheat resistance to HF depends on the timing of phytohormone application and/or HF larval density at HF feeding sites in the plants. The early application of SA, OPDA, and IAA enhanced wheat resistance to HF under heat stress at low larval density, while the delayed application of SA, OPDA, and IAA did not affect wheat resistance to HF at high larval density.
Introduction
Phytohormones salicylic acid (SA), jasmonic acid (JA), and 12-oxophytodienoic acid (OPDA) play important roles in plant resistance to insects (Halitschke and Baldwin, 2004; Loake and Grant, 2007; Erb et al., 2012). Depending on specific plant-insect interactions, these phytohormones can act either individually or interactively in plants’ response to insects. Generally, SA regulates plant defenses against minimal-damaging insects such as aphids (Moran and Thompson, 2001; Loake and Grant, 2007), while JA regulates plants’ defense against more mechanically damaging insects (Halitschke and Baldwin, 2004). SA induced by insect feeding antagonizes JA mediated plant defenses against insect attack (Costarelli et al., 2020). Nevertheless, previous studies have found that JA contributes to plant resistance against sucking insects such as soybean resistance to soybean aphid (Aphis glycines Matsumura) (Chapman et al., 2018; Yates-Stewart et al., 2020). As a precursor for JA synthesis, OPDA, can also induce plants’ defense against insects via JA-independent pathways (Stintzi et al., 2001; Willmann, 2002; Louis et al., 2015; Varsani et al., 2019) and regulates gene expression in concert with JA to fine-tune the expression of defense genes (Stintzi et al., 2001). On the other hand, the phytohormone Auxin (Indole acetic acid, IAA) affects plant-parasite interactions in an opposite way. Generally, IAA suppresses plant defenses, resulting in increased pathogen virulence (Spoel and Dong, 2008) and host susceptibility to pathogens and insects (Tooker and de Moraes, 2011; Kunkel and Harper, 2018). SA, JA, OPDA, and IAA also play important roles in plants’ responses to heat stress. The levels of these phytohormones change in responses to heat stress in Arabidopsis and several other plant species (Dat et al., 1998; Clarke et al., 2009; Currie et al., 2014; Li et al., 2021). The involvement of phytohormones in both insect infestation and heat stress suggests that phytohormones may play significant roles in the expression of plant resistance/susceptibility to insects under heat conditions. Nevertheless, previous studies have focused on the impact of phytohormones on plant responses to either insects or heat stress individually, but not in combination, leaving a knowledge gap on how phytohormones affect plant resistance to insects under heat conditions.
Wheat (Triticum aestivum) is one of the most important staple crops. The Hessian fly (HF, Mayetiola destructor) is one of the most notorious pests affecting wheat, causing substantial yield losses worldwide (Tadesse et al., 2022). HF adult females lay eggs on the leaves of wheat plants. Each female can deposit ~200 eggs (Stuart et al., 2012). Those eggs hatch into larvae within a few days depending on temperature and humidity. HF attack plants only at the larval stage. The larvae of HF have piercing sucking mouth parts (Hatchett et al., 1990; Harris et al., 2006). Upon hatching, neonates crawl down to the base and live between leaf sheaths by establishing a permanent feeding site (Stuart et al., 2012). The interaction between wheat and HF follows a typical gene-for-gene style (Hatchett and Gallun, 1970). A virulent HF larva can establish feeding in a susceptible wheat plant, usurp the biosynthesis machinery of the susceptible plant to acquire nutrients required for its own growth and development, complete its life cycle, stunt the plant, and eventually cause the death of the plant (Harris et al., 2006; Subramanyam et al., 2015). Attacks from an avirulent HF larva to a resistant wheat plant containing the corresponding R gene, however, induce rapid and fierce defense responses from the plant (Giovanini et al., 2006; Kosma et al., 2010; Khajuria et al., 2013; Subramanyam et al., 2013), resulting in the death of the attacking insect (Shukle et al., 1990). Wheat resistance has been the most effective pest management strategy against HF. To date, 37 resistance genes have been identified in wheat and wheat-related species (Liu et al., 2005; Zhao et al., 2020), and many of them were deployed in wheat cultivars to protect wheat against HF infestation (Zhao et al., 2020). Nevertheless, most, if not all, HF resistance genes in wheat are temperature-sensitive (Chen et al., 2014). Under high-temperature, a resistant wheat plant can become susceptible in an otherwise incompatible wheat-HF interaction (Zhu et al., 2020).
We have been studying wheat-HF interaction under normal and heat conditions. Our previous results have demonstrated that the increased accumulations of SA, JA, OPDA, and IAA at HF feeding sites in wheat plants were correlated with wheat resistance/susceptibility (Zhu et al., 2010, 2011), and that externally applying SA and OPDA enhanced plant resistance to HF under normal temperature and heat conditions (Zhu et al., 2012; Underwood et al., 2014; Cheng et al., 2018). In the current study, we investigated the combined impact of phytohormones and heat stress on wheat resistance to HF. We found that under heat stress, the effect of phytohormones is dependent on the timing of phytohormone application and/or larval density at the HF feeding site of a wheat plant.
Materials and methods
Plants and insects
The wheat cultivar ‘Molly’ and an avirulent HF biotype GP were used in the study. Molly contains the HF resistance gene H13 (Patterson et al., 1994), and exhibits resistance to HF biotype GP at 27°C or below (Zhu et al., 2020).
Plant preparation and infestation
Plant preparation and insect infestation were conducted as described previously (Zhu et al., 2020) with slight modifications. Briefly, Molly seeds were first germinated in petri dishes. 15 germinated wheat seeds were planted in each pot of 10 cm in diameter. The plants were placed in a growth chamber programmed at 20°C and 14L:10D photoperiod before infestation and after heat treatment. Plants that significantly lagged in development were removed from the pots before infestation, resulting in 10 plants in each pot to be infested by HF adults. At the 1.5-leaf stage, 5—8 female and two male HF adults were transferred onto plants confined within each plastic cage with a mesh screen on the top. These flies immediately started to lay eggs on wheat leaves. HF eggs hatched into larvae in ~ 72 hours. The neonate then crawled down to the base of a plant, living between the first and second leaf sheaths and attacking plants at the second leaf sheath (feeding sites). The time when HF initiated attacks was designated as 0-time. To determine the 0-time, the infested plants were pulled from random pots, dissected, and observed under a dissection microscope starting at 72 hours following infestation. The time when 50% of checking plants contained at least one larva was deemed as 0-time.
Experimental treatment and design
Six treatments were included in each experiment (Table 1). Plants in all treatments were infested with HF. Treatment one was used as the control with plants growing at 20°C. Treatment two was subjected to 30°C heat stress for 24 hours. Treatments three, four, five, and six were subjected to the combination of heat stress and external application of phytohormones SA, JA, OPDA, and IAA, respectively. Two consecutive experiments, the early treatment experiment and the late treatment experiment, were conducted under the same growth and infestation conditions to control the HF larval density at feeding sites, following the Randomized complete block design (RCBD). Four biological replicates were included in the early treatment experiment, and five biological replicates were included in the late treatment experiment.
Phytohormone application and heat treatment
Two millimolar of SA, JA, OPDA, and IAA solutions in 0.02% Silwet L-77 solution (PH=7) was made prior to heat treatment and phytohormone applications. The SA solution was made from SA powder with 99% purity (Sigma-Alrich, St. Louis, MO). JA solution was made from liquid JA with ≥ 98% purity (Cayman Chemical, Ann Arbor, MI). OPDA solution was made from liquid OPDA with 99% purity (Cayman Chemical, Ann Arbor, MI). IAA solution was made from IAA powder with ≥ 98% purity (Thermo Fisher Scientific, Waltham, MA). And Silwet L-77 solution was made from liquid Silwet L-77 (PhytoTech Labs, Lenexa, KS). A 4.5ml solution containing each phytohormone was sprayed thoroughly onto the foliage of wheat plants using a 50 ml spray bottle (Target, Cary, NC). The concentration of phytohormones was chosen based on previous studies (Zhu et al., 2012; Underwood et al., 2014; Cheng et al., 2018). The amount of phytohormone solutions to spray onto wheat plants was determined based on our preliminary experimental results. In the early treatment experiment, phytohormones were applied at 0-time, while in the late treatment experiment, phytohormones were applied at 24 h after 0-time. Plants without phytohormone applications were sprayed with 4.5 ml 0.02% Silwet L-77 solution. Immediately after phytohormone or Silwet applications, the plants for heat treatment were placed in a growth chamber set at 30°C for 24 hours and returned to 20°C afterward until the time for phenotyping.
Phenotyping
Phenotyping was conducted seven days after 0-time. Each plant of each replicate for each treatment was dissected and examined under a dissection microscope. The numbers of dead larvae and live larvae at the HF feeding site of each plant in each replicate of each treatment were counted (Supplementary Table S1). A plant was considered resistant if it contained only dead larva(e) and susceptible if it contained any live larva(e). The mean number of total larvae per plant was calculated using the formular ‘Total larvae number per replicate/Number of plants containing larvae per replicate’. The percentage of susceptible plants was calculated using the formular ‘Number of susceptible plants per replicate/Number of plants containing larvae per replicate’. The percentage of live larvae was calculated using the formular ‘Number of live larvae per replicate/Number of total larvae per replicate’.
Statistical analysis of data
The normal homogeneity of the variances (Bartlett’s test) was tested for all the data. The result indicated that all the response variables met the normality assumption. One-way analysis of variance (ANOVA) was conducted on the percentage of susceptible plants, the percentage of live larvae, and the mean number of larvae per plant using the International Business Machines Corporation (IBM) Statistical Package for the Social Sciences (SPSS) Statistics (IBM SPSS Statistics, 2022). The means were compared among treatments, and the significance of differences was determined using the least significant difference (LSD) at α < 0.05.
Results
Differences in larval density between early and late treatment experiments
To control the larval density, we conducted two experiments, the early treatment experiment and the late treatment experiment, under the same growth condition but different timing in application of phytohormone and heat stress. In both experiments, each pot (replicate) contained 10 plants at the time when HF adults were released onto plants (Table 2). As expected, low larval density was achieved in the early treatment experiment with mean larvae number per plant ranging from 1.6 to 2.7, while high larval density was achieved in the late treatment experiment with mean larvae number per plant ranging from 21 to 25 (Table 2). Significant differences in larval density among treatment were not found in either the early treatment experiment (F = 1.116, df = 5, P = 0.38) or the late treatment experiment (F = 2.197, df = 5, P = 0.089) (Table 2).
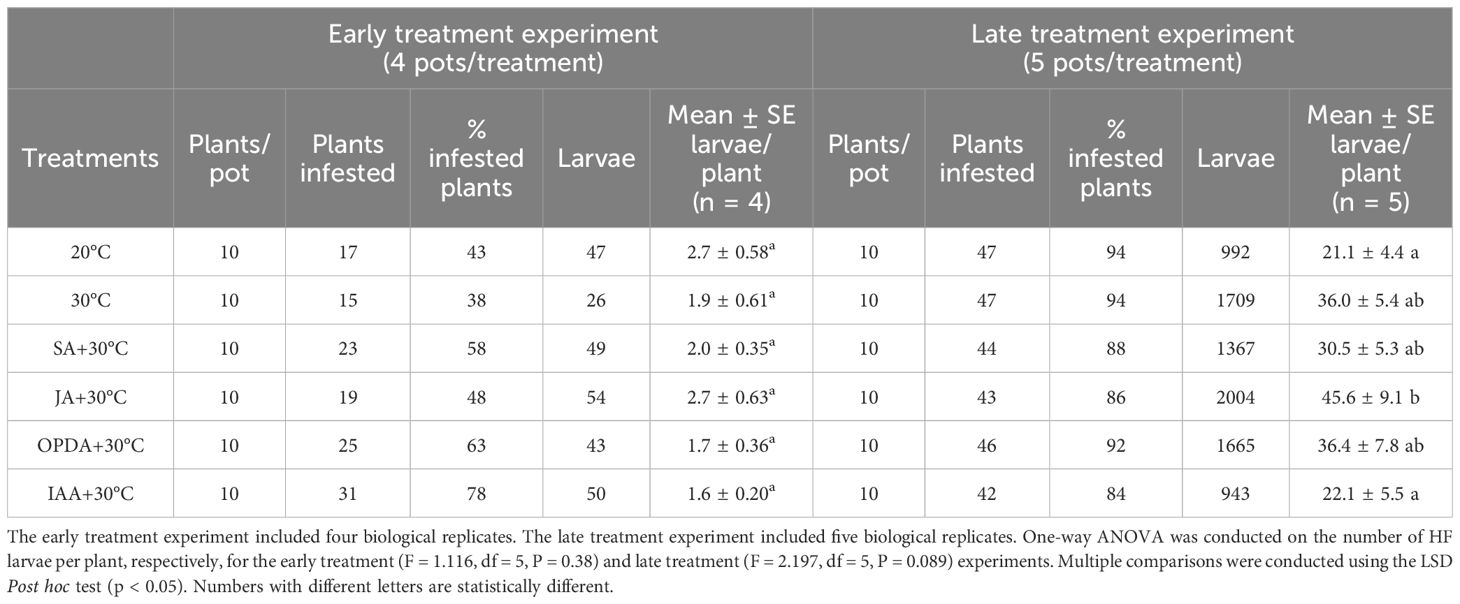
Table 2 Number of plants per replicate (pot) per treatment (Plants/pot), total number of plants infested with HF larvae (Plants infested), percentage of plants infested with HF larvae (% infested plants), total number of larvae in HF larvae infested plants (Larvae) per treatment, mean number of larvae per HF infested plant per treatment with standard error (Mean ± SE larvae/plant) in the early and late treatment experiments, respectively.
Differential impact of phytohormone applications on percentage of susceptible plants
Percentage of susceptible plants represents the susceptibility of wheat plants to HF (Cheng et al., 2018; Zhu et al., 2020). As expected, in both experiments, all or nearly all control plants growing at 20°C exhibited resistance to HF infestation, whereas plants exposed to heat stress displayed significantly higher percentages of susceptible plants (Figure 1), indicating that heat stress at 30°C for 24 hours weakened wheat resistance to HF regardless of the timing of heat stress application and larval density at feeding sties (Table 1). Nevertheless, the comparisons between heat-stressed plants and plants subjected to the combination of heat stress and phytohormone application displayed different results between the early treatment and late treatment experiments. In the early treatment experiment, the combined application of heat stress with SA, OPDA, or IAA, respectively, resulted in the decreased percentage of susceptible plants (Figure 1A), indicating that the application of these phytohormones enhanced wheat resistance to HF under heat conditions. In contrast, in the late treatment experiment, no significant difference was found in the percentage of susceptible plants between plants heat-stressed alone and plants subjected to the combination of heat stress and hormonal treatment with SA, OPDA, and IAA individually (Figure 1B), indicating that the application of these phytohormones onto wheat plants did not alter wheat susceptibility to HF under the heat condition. The application of JA, however, did not affect the percentage of susceptible plants under heat conditions in both experiments.
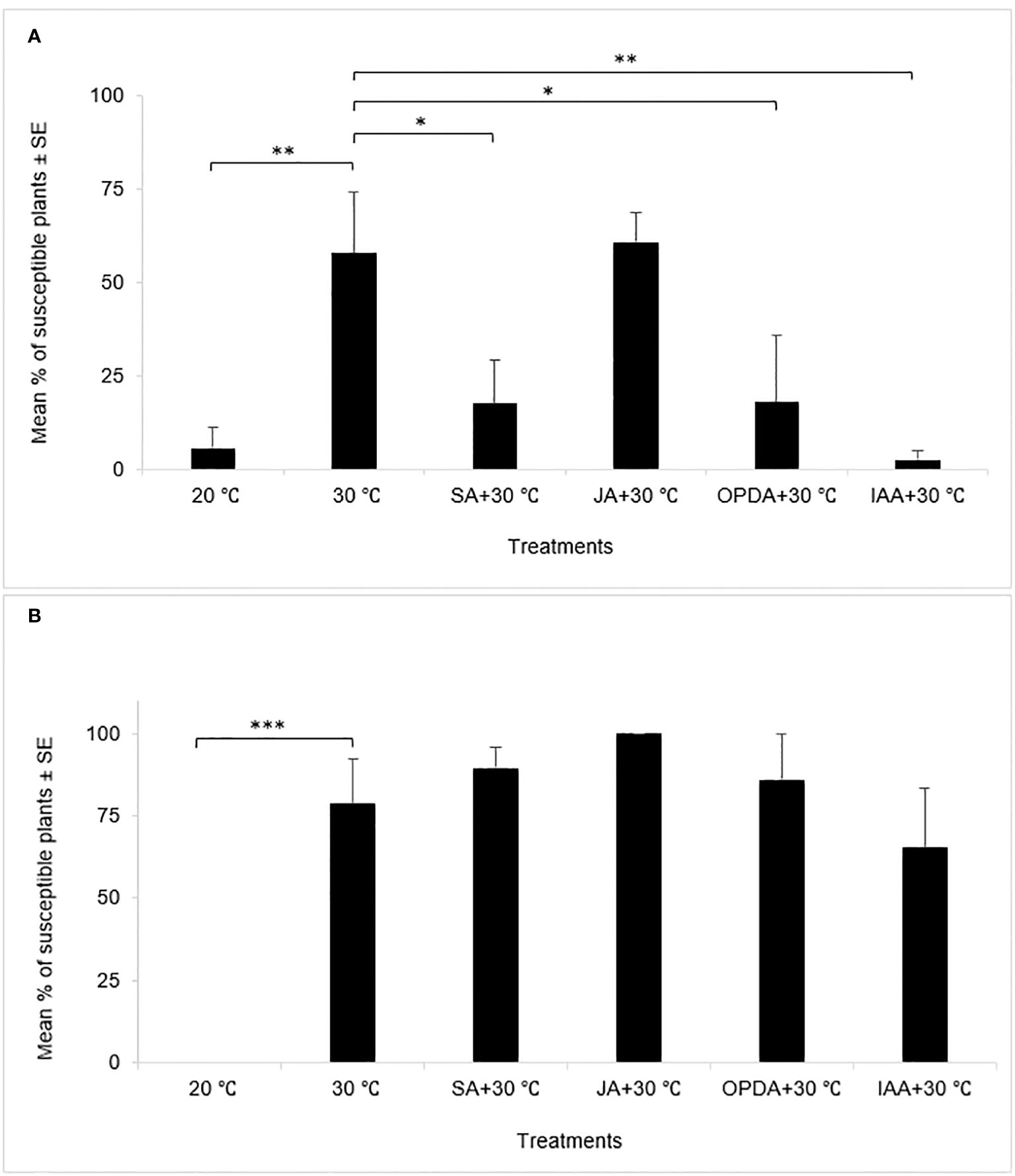
Figure 1 Mean percentage of susceptible plants in the early treatment [(A), n=4] and late treatment [(B), n = 5] experiments, respectively. *: p < 0.05, **: p < 0.01, ***: p < 0.001.
Differential impact of phytohormone application on percentage of live larvae
The percentage of live larvae represents the survival rate of HF larvae and is an important criterion to indicate the intensity of plant resistance responses (Zhu et al., 2012). A higher percentage of live larvae indicates weaker plant resistance responses (Currie et al., 2014). In our current study, the percentage of live larvae exhibited similar patterns as the percentage of susceptible plants in both experiments. Specifically, plants exposed to heat stress alone displayed higher percentages of live larvae than control plants under 20°C in both experiments (Figure 2), indicating that heat stress at 30°C for 24 hours weakened wheat resistance responses to HF regardless of the timing of heat treatments. In the early treatment experiment, the combined application of heat stress with SA, OPDA, or IAA, respectively, onto wheat plants resulted in significantly smaller percentage of live larvae compared to the plants under heat stress alone (Figure 2A), while in the late treatment experiment, no significant difference was found in the percentage of live larvae between the plants exposed to heat stress alone and the plants subjected to the combination of heat stress and SA, OPDA, and IAA, respectively (Figure 2B), indicating that the external application of these phytohormones did not affect the resistance responses of plants to HF infestation. Again, JA did not affect the percentage of live larvae in plants under heat stress in both experiments.
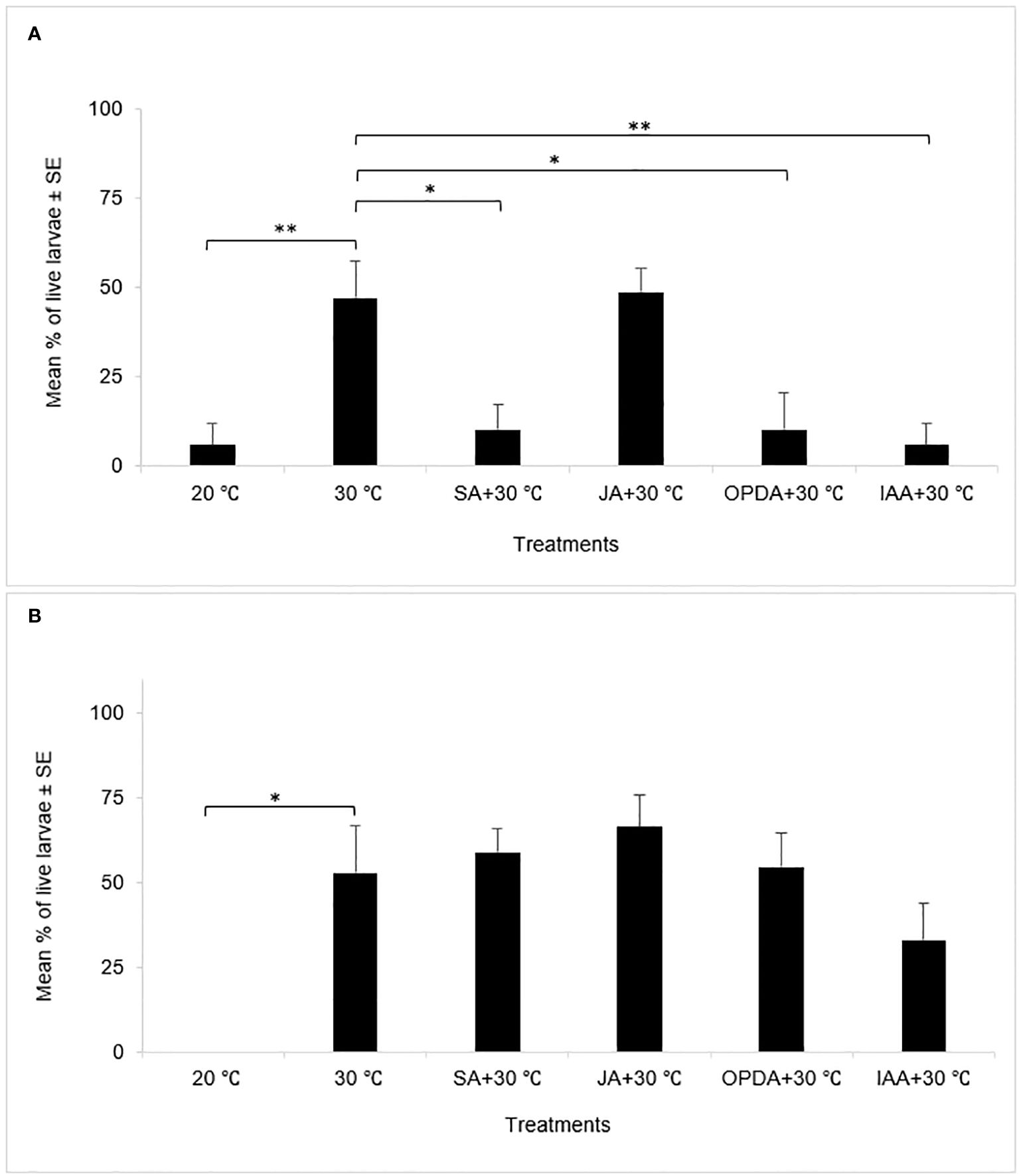
Figure 2 Mean percentages of live larvae in the early treatment [(A), n = 4] and late treatment [(B), n = 5] experiments, respectively. *: p < 0.05, **: p < 0.01.
Discussion
We successfully achieved differential larval densities at HF feeding sites between the early and late treatment experiments. Yet, the low larval density in the early treatment experiment was not due to the effect of phytohormone and/or heat stress since no significant differences was found in larval density among all the treatments regardless of heat stress and/or phytohormone applications (Table 2). Rather, the low larval density was caused by the toxic effect of Silwet L-77 to HF. The toxicity of Silwet L-77 solution to insect eggs, nymphs, and adults has been documented. 0.05% Silwet L-77 solution killed all nymphs and 47% adult Asian citrus psyllid (Diaphorina citri Kuwayama) (Srinivasan et al., 2008). Eggs of Pacific spider mite (Tetranychus pacificus McGregor) was highly susceptible to 0.1% Silwet L-77 with mortality > 99.4% (Tipping et al., 2003). The application of 0.02% surfactant Silwet L-77 onto wheat foliage in our early treatment experiment at 0-time when only a few HF larvae reached the base of wheat plants likely killed most if not all unhatched HF eggs and newly hatched larvae before their arrival at the feeding sites, causing considerable reduction in the number of plants infested with HF larvae and the number of HF larvae at feeding sites (Table 2; Supplementary Table S1), while in the late treatment experiment, the spray of Silwet L-77 solution was delayed for 24 hours, which allowed more eggs to hatch and larvae to migrate to feeding sites, resulting in higher larval density (Table 2; Supplementary Table S1).
Our results also indicated that the application of phytohormones SA, OPDA, and IAA, respectively, enhanced wheat resistance in the early treatment experiment when larval density at HF feeding site was low (Figures 1A, 2A), but did not affect wheat resistance in the late treatment experiment when the larval density was high (Figures 1B, 2B). The differential impact of SA, OPDA, and IAA between early and late treatment experiments may be caused by the difference in the timing of the phytohormone application and/or the difference in the larval density of wheat plants. Phytohormones are signaling molecules that induce rapid defense responses in plant resistance against parasites (Varsani et al., 2019; Ding and Ding, 2020). Highly enhanced accumulation of SA and OPDA were observed at the HF feeding sites in resistance plants during the incompatible wheat-HF interaction (Zhu et al., 2010). The external application of SA solution onto wheat foliage upregulated the expression of wheat defense-response genes (Sardesai et al., 2005; Subramanyam et al., 2006). Moreover, the application of SA and OPDA solutions, respectively, reduced HF larval survival in wheat plants (Zhu et al., 2012). These findings demonstrated that SA and OPDA play important roles in wheat resistance to HF. The effective defense of wheat against HF, however, is time-sensitive and requires rapid mobilization of membrane lipids and timely synthesis of defense related compounds, which was often delayed during the compatible interactions (Zhu et al., 2012; Khajuria et al., 2013). That said, the early application of those phytohormones onto wheat plants in the early treatment experiment likely resulted in early and rapid activation of defense responses that provide better protection to the heat stressed wheat plants against infesting HF larvae, while in the late treatment experiment, the 24 hour delay in application of the phytohormones, may result in delayed defense responses that are ‘too little, too late’ in helping the wheat plants fight against feeding HF larvae under heat conditions. Our current results are consistent with our previous findings that the application of SA and OPDA solution, respectively, at 0-time enhanced wheat resistance to HF under heat conditions (Underwood et al., 2014; Cheng et al., 2018). Further study will be necessary to investigate the impact of different timing in phytohormone application on wheat resistance to HF and other insects. It is also likely that the difference in HF larval density played a role in the differential impact of phytohormones on wheat resistance to HF under heat conditions between the early and late treatment experiments. Density-dependency of plant resistance to insects has been observed in several plant species previously (Kroes et al., 2015; Kersch-Becker and Thaler, 2019). A larger number of feeding insects can induce greater physiological and biochemical changes in plants than a smaller number of feeding insects (Shiojiri et al., 2010). Under normal temperature, wheat resistance to HF is largely density independent. A single virulent HF larva can deactivate plant defense and render a wheat plant susceptible (Byers and Gallun, 1972). Wheat plants subjected to heat stress, however, undergo a myriad of changes physiologically and biochemically (Currie et al., 2014; Zhu et al., 2020, Yuan et al., 2022) and become more susceptible to HF (Liu et al., 2013; Cheng et al., 2018). The external application of phytohormones may induce defense responses that could counteract the susceptibility responses induced by heat stress to a certain degree if the larval density is low and the susceptibility responses is weak but was unable to help plants regain resistance when infesting larvae population is high and susceptibility responses is strong. Of particular interest is the observation that external application of IAA also enhanced wheat resistance to HF under heat conditions at low larval density (Figures 1A, 2A). Higher level of endogenous IAA was found in susceptible plants. We, therefore, considered IAA to be linked with wheat susceptibility to HF (Zhu et al., 2010). Nevertheless, the role of IAA in plant-parasite interactions can be complicated. Previous studies have found that IAA could either suppress plant defenses, resulting in increased insect and pathogen virulence (Wang et al., 2007; Spoel and Dong, 2008; Tooker and de Moraes, 2011; Kunkel and Harper, 2018) or enhance plant resistance to insects via its influence on the levels and balance of other defense-related phytohormones, such as SA and JA (Ding et al., 2008), resulting in an altered hormone signaling network and enhanced production of defensive compounds (Mine et al., 2018). Further research is needed to fully comprehend the role of IAA in wheat resistance to HF under normal and heat conditions. We also found that the external application of JA did not influence wheat resistance to HF under heat conditions, regardless of HF larval density at feeding site and the timing in phytohormone application. Such results suggested that JA may not play significant roles in wheat resistance to HF. This finding is largely in alignment with previous findings that OPDA and SA but not JA were significantly induced in resistant wheat plants during the incompatible interaction (Zhu et al., 2010), that the external application of SA, OPDA, respectively, but not the combination of JA and OPDA reduced the survival rate of HF larvae in the susceptible plants (Zhu et al., 2012), and that the external application of SA and JA solution, respectively, onto wheat plants upregulated different set of genes related to wheat plants’ responses to HF (Sardesai et al., 2005). Though a precursor for JA synthesis, OPDA and JA control different aspects of plant resistance to insects (Bosch et al., 2014). OPDA can activate plant defense responses in the absence of JA (Stintzi et al., 2001).
In summary, our results indicated that the impact of externally applied phytohormones on wheat resistance to HF infestation depends on the timing of phytohormone application and/or HF larval density at feeding sites. The early applications of SA, OPDA, and IAA enhanced wheat resistance to HF under heat stress at low larval density, while the delayed applications of SA, OPDA, and IAA did not affect wheat resistance to HF infestation at high larval density. These findings contribute to our understanding of the complexity of the impact of the externally applied phytohormones, on plant resistance to insect pests in relation to timing of phytohormone application, insect density, and plant responses to abiotic stresses.
Data availability statement
The original contributions presented in the study are included in the article/Supplementary Material. Further inquiries can be directed to the corresponding author.
Ethics statement
The manuscript presents research on animals that do not require ethical approval for their study.
Author contributions
MM: Investigation, Methodology, Writing – original draft, Writing – review & editing. LZ: Conceptualization, Funding acquisition, Methodology, Project administration, Writing – original draft, Writing – review & editing. JJ: Investigation, Writing – review & editing. MC: Methodology, Resources, Writing – review & editing. DC: Investigation, Writing – review & editing. NR: Investigation, Writing – review & editing. CG: Investigation, Writing – review & editing. HP: Investigation, Writing – review & editing. JW: Investigation, Writing – review & editing.
Funding
The author(s) declare financial support was received for the research, authorship, and/or publication of this article. The research is supported by NSF-HBCU-UP-EIR Award # 2100425.
Acknowledgments
The authors thank Dr. Subhashree Subramanyam at Purdue University, Department of Agronomy, for providing ‘Molly’ wheat seeds, Dr. Shubo Han in the Department of Chemistry, Physics, and Materials Science, Fayetteville State University, NC, USA, for his kind assistance in phytohormone solution preparation.
Conflict of interest
The authors declare that the research was conducted in the absence of any commercial or financial relationships that could be construed as a potential conflict of interest.
Publisher’s note
All claims expressed in this article are solely those of the authors and do not necessarily represent those of their affiliated organizations, or those of the publisher, the editors and the reviewers. Any product that may be evaluated in this article, or claim that may be made by its manufacturer, is not guaranteed or endorsed by the publisher.
Author disclaimer
Mention of trade names or commercial products in this publication is solely for the purpose of providing specific information and does not imply recommendation or endorsement by the U.S. Department of Agriculture. The USDA is an equal opportunity provider and employer.
Supplementary material
The Supplementary Material for this article can be found online at: https://www.frontiersin.org/articles/10.3389/fagro.2024.1331871/full#supplementary-material
References
Bosch M., Louwrance P. W., Gershenzon J., Wasternack C., Hause B., Schaller A., et al. (2014). Jasmonic acid and its precursor 12-oxophytodienoic acid control different aspects of constitutive and induced herbivore defenses in tomato. Plant Physiol. 166)1, 396–410. doi: 10.1104/pp.114.237388
Byers R. A., Gallun R. L. (1972). Ability of the Hessian fly to stunt winter wheat. 1. Effect of larval feeding on elongation of leaves. J. Econ. Entomol. 65, 955–958. doi: 10.1093/jee/65.4.955
Chapman K. M., Marchi-Werle L., Hunt T. E., Louis J. (2018). Abscisic and jasmonic acids contribute to soybean tolerance to the soybean aphid (Aphis glycines matsumura). Sci. Rep. 8, 15148. doi: 10.1038/s41598-018-33477-w
Chen M. S., Wheeler S., Wang H., Whitworth R. J. (2014). Impact of temperatures on Hessian fly (Diptera: Cecidomyiidae) resistance in selected wheat cultivars (Poales: Poaceae) in the Great Plains region. J. economic entomology 107, 1266–1273. doi: 10.1603/EC13357
Cheng G., Chen M. S., Zhu L. (2018). 12-Oxo-Phytodienoic acid enhances wheat resistance to Hessian fly (Diptera: Cecidomyiidae) under heat stress. J. Econ. Entomol. 111, 917–922. doi: 10.1093/jee/tox374
Clarke S. M., Cristescu S. M., Miersch O., Harren F. J. M., Wasternack C., Mur L. A. J. (2009). Jasmonates act with salicylic acid to confer basal thermotolerance in Arabidopsis thaliana. New Phytol. 182, 175–187. doi: 10.1111/j.1469-8137.2008.02735.x
Costarelli A., Bianchet C., Ederli L., Salerno G., Piersanti S., Rebora M., et al. (2020). Salicylic acid induced by herbivore feeding antagonizes jasmonic acid mediated plant defenses against insect attack. Plant Signaling Behav. 15. doi: 10.1080/15592324.2019.1704517
Currie Y., Moch J., Underwood J., Kharabsheh H., Quesenberry A., Miyagi R., et al. (2014). Transient heat-stress compromises the resistance of wheat seedlings to Hessian fly (Diptera: Cecidomyiidae) infestation. J. Econ. Entomol. 107, 389–395. doi: 10.1603/EC13261
Dat J. F., López-Delgado H., Foyer C. H., Scott I. M. (1998). Parallel changes in H2O2 and catalase during thermotolerance induced by salicylic acid or heat acclimation in mustard seedlings. Plant Physiol. 116, 1351–1357. doi: 10.1104/pp.116.4.1351
Ding P., Ding Y. (2020). Stories of salicylic acid: a plant defense hormone. Trends Plant science. 25, 549–565. doi: 10.1016/j.tplants.2020.01.004
Ding X., Cao Y., Huang L., Zhao J., Xu C., Li X., et al. (2008). Activation of the indole-3-acetic acid-amido synthetase GH3-8 suppresses expansin expression and promotes salicylate- and jasmonate-independent basal immunity in rice. Plant Cell. 20, 228–240. doi: 10.1105/tpc.107.055657
Erb M., Meldau S., Howe G. A. (2012). Role of phytohormones in insect-specific plant reactions. Trends Plant Sci. 17, 250–259. doi: 10.1016/j.tplants.2012.01.003
Giovanini M. P., Puthoff D. P., Nemacheck J. A., Mittapalli O., Saltzmann K. D., Ohm H. W., et al. (2006). Gene-for-gene defense of wheat against the Hessian fly lacks a classical oxidative burst. MPMI. 19, 1023–1033. doi: 10.1094/MPMI-19-1023
Halitschke R., Baldwin I. T. (2004). Jasmonates and related compounds in plant-insect interactions. J. Plant Growth Regul. 23, 238–245. doi: 10.1007/BF02637264
Harris M., Freeman T., Rohfritsch O., Anderson K., Payne S., Moore J. (2006). Virulent Hessian fly (Diptera: Cecidomyiidae) larvae induce a nutritive tissue during compatible interactions with wheat. Ann. Entomol. Soc Am. 99, 305–316. doi: 10.1603/0013-8746(2006)099[0305:VHFDCL]2.0.CO;2
Hatchett J. H., Gallun R. L. (1970). Genetics of the ability of the Hessian fly, Mayetiola destructor, to survive on wheat having different genes for resistance. Ann. Entomol. Soc. Am. 63, 1400–1407.
Hatchett J. H., Kreitner G. L., Elzinga R. J. (1990). Larval mouthparts and feeding mechanism of the Hessian fly (Diptera: Cecidomyiidae). Ann. Entomol. Soc. Am. 83, 1137–1147.
Kersch-Becker M. F., Thaler J. S. (2019). Constitutive and herbivore-induced plant defences regulate herbivore population processes. J. Anim. Ecol. 88, 1079–1088. doi: 10.1111/1365-2656.12993
Khajuria C., Wang H., Liu X., Wheeler S., Reese J. C., El Bouhssini M., et al. (2013). Mobilization of lipids and fortification of cell wall and cuticle are important in host defense against Hessian fly. BMC Genomics. 14, 1–16. doi: 10.1186/1471-2164-14-423
Kosma D. K., Nemacheck J. A., Jenks M. A., Williams C. E. (2010). Changes in properties of wheat leaf cuticle during interactions with Hessian fly. Plant J. 63, 31–43. doi: 10.1111/j.1365-313X.2010.04229.x
Kroes A., van Loon J. J., Dicke M. (2015). Density-dependent interference of aphids with caterpillar-induced defenses in Arabidopsis: involvement of phytohormones and transcription factors. Plant Cell Physiol. 56, 98–106. doi: 10.1093/pcp/pcu150
Kunkel B. N., Harper C. P. (2018). The roles of auxin during interactions between bacterial plant pathogens and their hosts. J. Exp. Bot. 69, 245–254. doi: 10.1093/jxb/erx447
Li N., Euring D., Cha J. Y., Lin Z., Lu M., Huang L. J., et al. (2021). Plant hormone-mediated regulation of heat tolerance in response to global climate change. Front. Plant Sci. 11. doi: 10.3389/fpls.2020.627969
Liu X. M., Brown-Guedira G. L., Hatchett J. H., Owuoche J. O., Chen M. S. (2005). Genetic characterization and molecular mapping of a Hessian fly-resistance gene transferred from T. turgidum ssp. dicoccum to common wheat. Theor. Appl. Genet. 111, 1308–1315. doi: 10.1007/s00122-005-0059-3
Liu X., Khajuria C., Li J., Trick H. N., Huang L., Gill B. S., et al. (2013). Wheat Mds-1 encodes a heat-shock protein and governs susceptibility towards the Hessian fly gall midge. Nat. Commun. 4, 2070. doi: 10.1038/ncomms3070
Loake G., Grant M. (2007). Salicylic acid in plant defense–the players and protagonists. Curr. Opin. Plant Biol. 10, 466–472. doi: 10.1016/j.pbi.2007.08.008
Louis J., Basu S., Varsani S., Castano-Duque L., Jiang V., Williams W. P., et al. (2015). Ethylene contributes to maize insect resistance1-mediated maize defense against the phloem sap-sucking corn leaf aphid. Plant Physiol. 169, 313–324. doi: 10.1104/pp.15.00958
Mine A., Seyfferth C., Kracher B., Berens M. L., Becker D., Tsuda K. (2018). The defense phytohormone signaling network enables rapid, high-amplitude transcriptional reprogramming during effector-triggered immunity. Plant Cell. 30, 1199–1219. doi: 10.1105/tpc.17.00970
Moran P. J., Thompson G. A. (2001). Molecular responses to aphid feeding in arabidopsis in relation to plant defense pathways. Plant Physiol. 125, 1074–1085. doi: 10.1104/pp.125.2.1074
Patterson F. L., Mass F. B. III, Foster J. E., Ratcliffe R. H., Cambron S., Safranski G., et al. (1994). Registration of eight Hessian fly resistant common winter wheat germplasm lines (Carol, Erin, Flynn, Iris, Joy, Karen, Lola, and Molly). Crop Sci. 34, 315–316. doi: 10.2135/cropsci1994.0011183X003400010084x
Sardesai N., Subramanyam S., Nemacheck J., Williams C. E. (2005). Modulation of defense-response gene expression in wheat during Hessian fly larval feeding. J. Plant Interact. 1, 39–50. doi: 10.1080/17429140500309498
Shiojiri K., Ozawa R., Kugimiya S., Uefune M., van Wijk M., Sabelis M. W., et al. (2010). Herbivore-specific, density-dependent induction of plant volatiles: honest or “cry wolf” signals? PLoS One 5 (8), e12161. doi: 10.1371/journal.pone.0012161
Shukle R., Grover P., Foster J. (1990). Feeding of Hessian fly (Diptera: Cecidomyiidae) larvae on resistant and susceptible wheat. Environ. Entomol. 19, 494–500.
Spoel S. H., Dong X. (2008). Making sense of hormone crosstalk during plant immune responses. Cell Host Microbe 3, 348–351. doi: 10.1016/j.chom.2008.05.009
Srinivasan R., Hoy M. A., Singh R., Rogers M. E. (2008). Laboratory and field evaluations of Silwet L-77 and kinetic alone and in combination with imidacloprid and abamectin for the management of the Asian citrus psyllid, Diaphorina citri (Hemiptera: Psyllidae). Fla. Entomol. 91, 87–100. doi: 10.1653/0015-4040(2008)091[0087:LAFEOS]2.0.CO;2
Stintzi A., Weber H., Reymond P., Browse J., Farmer E. E. (2001). Plant defense in the absence of jasmonic acid: the role of cyclopentenones. Proc. Natl. Acad. Sci. U.S.A. 98, 12837–12842. doi: 10.1073/pnas.211311098
Stuart J. J., Chen M. S., Shukle R., Harris M. O. (2012). Gall midges (Hessian flies) as plant pathogens. Annu. Rev. Phytopathol. 50, 339–357. doi: 10.1146/annurev-phyto-072910-095255
Subramanyam S., Sardesai N., Minocha S. C., Zheng C., Shukle R. H., Williams C. E. (2015). Hessian fly larval feeding triggers enhanced polyamine levels in susceptible but not resistant wheat. BMC Plant Biol. 15, 1–16. doi: 10.1186/s12870-014-0396-y
Subramanyam S., Sardesai N., Puthoff D. P., Meyer J. M., Nemacheck J. A., Gonzalo M., et al. (2006). Expression of two wheat defense-response genes, Hfr-1 and Wci-1, under biotic and abiotic stresses. Plant Sci. 170, 90–103. doi: 10.1016/j.plantsci.2005.08.006
Subramanyam S., Zheng C., Shukle J. T., Williams C. E. (2013). Hessian fly larval attack triggers elevated expression of disease resistance dirigent-like protein-encoding gene, HfrDrd, in resistant wheat. Arthropod Plant Interact. 7, 389–402. doi: 10.1007/s11829-013-9253-4
Tadesse W., El-Hanafi S., El-Fakhouri K., Imseg I., Rachdad F. E., El-Gataa Z., et al. (2022). Wheat breeding for Hessian fly resistance at ICARDA. Crop J. 10, 1528–1535. doi: 10.1016/j.cj.2022.07.021
Tipping C., Bikoba V., Chander G. J., Mitcham E. J. (2003). Efficacy of Silwet L-77 against several arthropod pests of table grape. J. Econ. Entomol. 96, 246–250. doi: 10.1093/jee/96.1.246
Tooker J. F., de Moraes C. M. (2011). Feeding by a gall-inducing caterpillar species alters levels of indole-3-acetic and abscisic acid in Solidago altissima (Asteraceae) stems. Arthropod Plant Interact. 5, 115–124. doi: 10.1007/s11829-010-9120-5
Underwood J., Moch J., Chen M. S., Zhu L. (2014). Exogenous salicylic acid enhances the resistance of wheat seedlings to Hessian fly (Diptera: Cecidomyiidae) infestation under heat stress. J. Economic Entomology. 107, 2000–2004. doi: 10.1603/EC14223
Varsani S., Grover S., Zhou S., Koch K. G., Huang P. C., Kolomiets M. V., et al. (2019). 12-oxo-phytodienoic acid acts as a regulator of maize defense against corn leaf aphid. Plant Physiol. 79, 1402–1415. doi: 10.1104/pp.18.01472
Wang L., Halitschke R., Kang J. H., Berg A., Harnisch F., Baldwin I. T. (2007). Independently silencing two JAR family members impairs levels of trypsin proteinase inhibitors but not nicotine. Planta 226, 159–167. doi: 10.1007/s00425-007-0477-3
Willmann M. R. (2002). Plant defense in the absence of jasmonic acid. Trends Plant Sci. 7, 8–9. doi: 10.1016/S1360-1385(01)02218-X
Yates-Stewart A. D., Pekarcik A., Michel A., Blakeslee J. J. (2020). Jasmonic acid-isoleucine (JA-ile) is involved in the host-plant resistance mechanism against the soybean aphid (Hemiptera: aphididae). J. Econ. Entomol. 113)6, 2972–2978. doi: 10.1093/jee/toaa221
Yuan J., O’Neal J., Brown D., Zhu L. (2022). Impact of heat stress on expression of wheat genes responsive to Hessian fly infestation. Plants 11 (11), 1402. doi: 10.3390/plants11111402
Zhao L., Abdelsalam N. R., Xu Y., Chen M. S., Feng Y., Kong L., et al. (2020). Identification of two novel Hessian fly resistance genes H35 and H36 in a hard winter wheat line SD06165. Theor. Appl. Genet. 133, 2343–2353. doi: 10.1007/s00122-020-03602-3
Zhu L. C., Chen M. S., Liu X. (2011). Changes in phytohormones and fatty acids in wheat and rice seedlings in response to hessian fly (Diptera: cecidomyiidae) infestation. J. Econ. Entomol 104, 1384–1392. doi: 10.1603/EC10455
Zhu L., Liu X., Chen M. S. (2010). Differential accumulation of phytohormones in wheat seedlings attacked by avirulent and virulent hessian fly (Diptera: cecidomyiidae) larvae. J. Economic Entomology. 103, 178–185. doi: 10.1603/EC09224
Zhu L., Liu X., Wang H., Khajuria C., Reese J. C., Whitworth R. J., et al. (2012). Rapid mobilization of membrane lipids in wheat leaf sheaths during incompatible interactions with Hessian fly. Mol. Plant Microbe Interact. 25, 920–930. doi: 10.1094/MPMI-01-12-0022-R
Keywords: larval density, impact, phytohormones, Hessian fly, resistance, wheat, heat stress
Citation: Maldani M, Zhu L, Jackson J, Chen M-S, Capers D, Rania N, Gore C, Pankey H and Walker J (2024) Impact of phytohormones on wheat resistance to Hessian fly under heat stress. Front. Agron. 6:1331871. doi: 10.3389/fagro.2024.1331871
Received: 01 November 2023; Accepted: 16 July 2024;
Published: 31 July 2024.
Edited by:
Elena Costi, University of Modena and Reggio Emilia, ItalyReviewed by:
Shubha Subramanyam, Agricultural Research Service (USDA), United StatesJoshua Blakeslee, The Ohio State University, United States
Copyright © 2024 Maldani, Zhu, Jackson, Chen, Capers, Rania, Gore, Pankey and Walker. This is an open-access article distributed under the terms of the Creative Commons Attribution License (CC BY). The use, distribution or reproduction in other forums is permitted, provided the original author(s) and the copyright owner(s) are credited and that the original publication in this journal is cited, in accordance with accepted academic practice. No use, distribution or reproduction is permitted which does not comply with these terms.
*Correspondence: Lieceng Zhu, bHpodUB1bmNmc3UuZWR1