- 1International Centre of Insect Physiology and Ecology, Nairobi, Kenya
- 2Department of Zoology and Entomology, University of Pretoria, Pretoria, South Africa
- 3Forestry and Agricultural Biotechnology Institute, University of Pretoria, Pretoria, South Africa
- 4Department of Life Sciences, South Eastern Kenya University, Kitui, Kenya
Plant-soil feedback can influence aboveground interactions between plants and herbivores by affecting plant chemistry. Such interactions can be utilized in pest management. However, cropping systems such as maize-legume intercropping (MLI) can influence these interactions which is not well understood. In this study, we explored effects of MLI systems on soil physico-chemical properties, maize growth, larval feeding and development of fall armyworm (Spodoptera frugiperda). We used sterile soil and soil conditioned by different MLI and maize-monoculture cropping systems to explore these interactions. Soil samples that included soil conditioned by different MLI and maize-monoculture cropping systems were collected from smallholder farmer fields in eastern Kenya, where different MLI and maize-monoculture cropping systems were being practiced. These soil samples were compared with sterile soils for physico-chemical properties using black oxidation and Walkley methods. Three-weeks-old maize plants grown in the different soil treatments in the greenhouse were used for larval feeding and development assays. Neonate S. frugiperda larvae were allowed to feed on maize leaf discs for 24 hours and another set of plants were inoculated with the neonates for 15 days and the larval survival and development monitored. Soil obtained from different maize-edible legume intercropping systems had a higher pH, electrical conductivity, nitrogen, organic carbon, potassium, phosphorus, calcium, magnesium, exchangeable acidity, copper, clay and silt compared to maize-monoculture and sterile soil. Maize plants grown in MLI soil had better growth parameters compared to those in maize-monoculture and sterile soils. A high correlation was found between pH and plant biomass, while no significant correlation with other physico-chemical properties was noted. There were significant differences in larval feeding by S. frugiperda neonates when exposed to constitutive and induced maize leaf discs with more leaf tissue fed on maize grown in maize-monoculture and sterile soil. When allowed to feed for 15 days, S. frugiperda larval weight and length were significantly lower on maize plants grown in soils conditioned by MLI than those grown in soil conditioned by maize-monoculture and sterile soils. Findings from this study show how conditioning soil by MLI systems improve soil health, maize growth and reduces S. spodoptera larval feeding and development.
1 Introduction
Global population will reach about nine billion by 2050, requiring a significant increase in crop production and yields to meet the high food demand amid ecological pressures (Van Dijk et al., 2021). However, crop production is limited by factors including poor soil fertility, diseases and pests which are exacerbated by changing climate (Midega et al., 2018; Chiriboga et al., 2021; Davidson-Lowe et al., 2021; Mutyambai et al., 2022). One pest that has become a global threat to the production of staple food is the fall armyworm, Spodoptera frugiperda J.E Smith (FAW) (Lepidoptera: Noctuidae). Fall armyworm is native to South America, whose invasion on the African continent was first reported in Nigeria in early 2016. But by January 2018, it had spread through most of sub-Saharan Africa (SSA) (Stokstad, 2017; FAO, 2018; Koffi et al., 2020). Fall armyworm is a polyphagous pest, known to attack over 350 plant species in the Americas (Montezano et al., 2018), with maize being the most preferred host for feeding and offspring development. The larval feeding on young maize leaves, whorls, tassels, and ears, leads to substantial damage even resulting in plant death in severe infestation levels. Plants weakened because of the defoliation and damage of cobs by the larvae are more susceptible to diseases and environmental stresses (Hailu et al., 2018; Mutyambai et al., 2022). The invasion of FAW in Africa and its subsequent spread around the world has become a significant source of concern due to its feeding on various staple and economically important crops, especially maize, sorghum, and millets, causing significant yield losses, in the absence of proper control measures (Chiriboga et al., 2021; Makgoba et al., 2021; Overton et al., 2021; Yan et al., 2022; Peter et al., 2023). The multivoltine nature of FAW coupled with rapid reproduction capacity and high dispersal ability has contributed to the successful invasion and establishment of FAW in SSA and beyond (Stokstad, 2017; De Groote et al., 2020). Surveys in Kenya showed that 82% of maize smallholder farmers had been affected by FAW, causing yield losses of up to 30%, reducing maize productivity by up to 1 million tonnes (De Groote et al., 2020; Mutyambai et al., 2022). In Ethiopia, Abro et al. (2021) estimated up to 36% yield loss of maize valued at US$ 200 million between 2017 and 2019. In spite of management efforts by farmers in Ethiopia, FAW caused up to 11.5% yield loss (Kassie et al., 2020). This reveals that there is an urgent need for effective control of FAW for food security and enhancing livelihoods of maize growers.
Several management practices have been implemented in SSA to tackle the menace of FAW. These include cultural practices, biological control using natural enemies and chemical control using insecticides (Hailu et al., 2018; Midega et al., 2018; Makgoba et al., 2021). When FAW invaded Africa, chemical control was the main strategy deployed by many African governments (Tambo et al., 2020; Chiriboga et al., 2021). Moreover, this approach was adopted by most smallholder maize farmers, who frequently applied insecticides. However, overuse of these synthetic insecticides is not a sustainable option as these pose adverse effects on human and environmental health including loss of pollinators, and pests’ natural enemies (Midega et al., 2018; Kumela et al., 2019; De Groote et al., 2020). Potential sustainable management strategies of FAW include agroecological-based approaches such as intercropping, conservation and augmentation of natural enemies as well as utilization of botanicals and beneficial microbes (Hailu et al., 2018; Midega et al., 2018; Kansiime et al., 2019; Makgoba et al., 2021). Habitat diversification through cropping systems such as push-pull technology and maize-legume intercropping (MLI) systems have been shown to reduce FAW infestation and increase maize yield where they are practiced compared to maize-monocultures (MMC) and other farmer practices (Hailu et al., 2018; Midega et al., 2018; Mutyambai et al., 2022; Librán-Embid et al., 2023). However, the impact of these cropping systems on the belowground soil properties and their subsequent effect on plant-soil feedbacks in relation to invasive pest herbivores like FAW is not well known. Hence, there is needed to understand these cropping systems interactions with belowground soil and its properties and plant-soil feedbacks mediated by the soil changes resulting from this cropping diversification to inform development of more effective and sustainable strategies for managing this pest in SSA.
Cultivation systems, such as MLI systems and crop rotation, have been shown to impact soil and regulate plant-soil interaction (Drinkwater et al., 2021; Wang et al., 2021). Recently, our understanding of how cropping systems impact crop output, plant growth, chemistry, and insect resistance has increased. For example, cover crop, push-pull cropping system and crop rotation strategies have significantly increased crop health and yield, soil health and offer a potential management option for the herbivore pests (Mutyambai et al., 2019; Ndayisaba et al., 2020; Davidson-Lowe et al., 2021; Jalli et al., 2021). In addition, diverse cropping strategies, such as cover-crop and cereal-legume intercropping systems exhibited significant expression of defense genes and emission of herbivore-induced volatiles and secondary metabolites, enabling plants to resist herbivore pests (Kaplan et al., 2018; Mutyambai et al., 2019; Davidson-Lowe et al., 2021). Some of the intercropped plants, like push-pull and maize-bean, release chemicals that attract insect herbivores away from the target or repel them from the area (Khan et al., 2012; Gordy et al., 2015; Peter et al., 2023). Moreover, companion crops improve soil health, increase organic content, mitigates erosion, inhibit the movement of larvae, prevent the laying of eggs, and provide a suitable habitat for natural enemies (Harrison and Bardgett, 2010; Khan et al., 2012; Sokame et al., 2020; Peter et al., 2023). Intercropping through simultaneous cultivation of different species masks volatile cues used by herbivores to locate hosts (Karban, 2011; Midega et al., 2018). According to Kaplan et al. (2018), plant species grown in a given soil have differential effects on subsequent plants that grow in the same soil. On the other hand, monoculture has been associated with detrimental impacts on plant soil feedback (PSF). This leads to negative consequences on plant development and pest resistance (Delgado and Gómez, 2016; Van der Putten et al., 2016), which is apparent in MMC systems (Ewel et al., 1991).
Plant-mediated effects affect soil composition through influence on nutrient accessibility, microbial communities, and organic composition, which are crucial for their growth and health. Different plant communities cause shifts in soil microbiota, affecting the performance of successive plants either positively or negatively (Agegnehu et al., 2016; Delgado & Gómez, 2016; Wang et al., 2021). This phenomenon, known as PSF, has been utilized as a cropping practice since the origin of agriculture (Harrison and Bardgett et al., 2010; Van der Putten et al., 2016; Sharma, 2022) to enhance agroecosystem services. However, only recently have ecologists become interested in PSF as a critical driver of plant dynamics and ecological processes (Mutyambai et al., 2019; Pervaiz et al., 2020; Wang et al., 2021). The intrinsic soil characteristics determine the entire agricultural output and the soil’s ability to support plant growth, which determines production potential (Agegnehu et al., 2016; Sharma, 2022). The soil’s physico-chemical, and biological characteristics make up these attributes, which give such systems their dynamism. The fertility of the soil is significantly influenced by soil physico-chemical characteristics, including soil organic matter, maximum water-holding capacity, electrical conductivity, bulk density, and pH (Van der Putten et al., 2016; Ndayisaba et al., 2020; Ndayisaba et al., 2022; Sharma, 2022). The soil is the main reservoir of nutrients and carbon, influencing soil health and fertility (Li et al., 2020; Wang et al., 2021). These outcomes improve our understanding of how various cropping systems impact ecosystem functioning, allowing us to create more effective and sustainable agricultural systems. Because of this, elucidating how different cropping systems alter soil-conditioning properties and reduce plant damage on maize plant becomes imperative. Studies that examine the underlying phenomenon in plant-soil interaction and its consequences have been largely ignored, despite the recent focus on the influence of PSF on soil physico-chemical characteristics and plant performance (Loreau et al., 2001; Pervaiz et al., 2020; Drinkwater et al., 2021).
This study aimed at investigating the effect of conditioning soil with different MLI systems on: (1) soil physico-chemical properties; (2) maize seed germination, plant growth and biomass; and (3) FAW larval feeding and development as a measure of direct resistance. We hypothesized that soil conditioned by different MLI systems exhibited better physico-chemical characteristics, mediates fast seed germination and enhance maize growth, and biomass compared to maize plants grown on MMC and S conditioned soil. We further hypothesized that FAW neonates fed less on plants grown in soil conditioned by different MLI systems compared to those grown in MMC conditioned and sterile soil.
2 Materials and methods
2.1 Study site and soil sample collection
Soil samples were collected from three counties in Kenya namely, Tharaka Nithi (N 00° 01’ 58.5” E 37° 47’ 23.1”; N 00° 18’ 51.9” E 37° 46’ 41.6”; 700 - 1113 meters above sea level (masl)), Embu (S 00° 30’ 07.4” E 37° 27’ 44.6”; S 00° 42’ 17.6” E 37° 29’ 32.7”; 1093 – 1541 masl), and Meru (N 00° 02’ 26.1” E 37° 45’ 55.5”; N 00° 01’ 48.6” E 37° 45’ 54.5”; 1110 – 1140 masl). These counties were selected based on the availability of different MLI and MMC cropping systems. Within each county, farms were chosen based on similarities in agronomical parameters, farm management, and minimum tillage to control weeds without applying synthetic fertilizers or pesticides. Rhizospheric soil samples were collected from different MLI and MMC cropping systems (Table 1) in farms that were already established. The major crops planted in these three counties include maize, millet, sorghum, black bean, common bean, pigeon pea, green gram, and cowpea, characterized by MLI systems. The climate in the area is sub-humid tropical with a bimodal rainfall distribution consisting of a long rain season (March-August) and a short season (October-December). The soils in the study area are mainly Acrisols and Nitisols. Four smallholder farms representing MLI and MMC cropping systems provided samples for each of the cropping systems in Table 1. The soil sampling was done randomly between maize and legume rows for intercrops and maize rows for MMC cropping system during the vegetative growth (4-5 weeks old) stage of the plants. Twelve samples were collected per smallholder farm (≈5-20 cm depth) using a soil auger after cleaning surface organic contents around the rows of the plants. The soils from each farm (12 points sample) were then mixed to form a composite and packaged in separate Khaki bags (Paper bags Ltd., Nairobi, Kenya) before transporting to the International Centre of Insect Physiology and Ecology (icipe) for analysis and experiments.
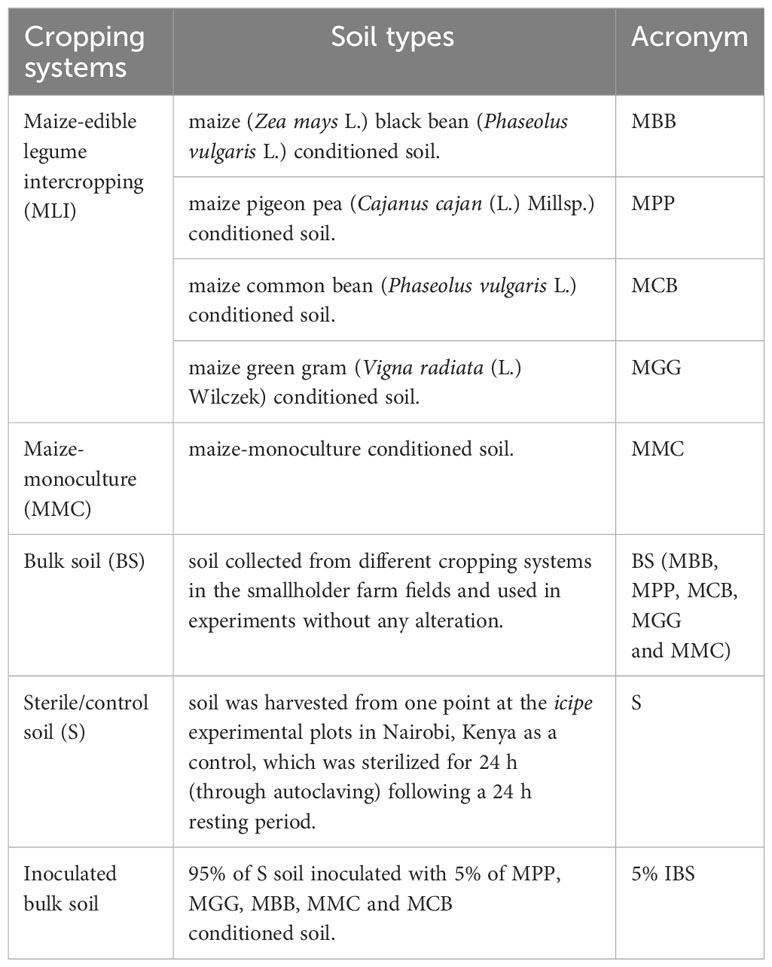
Table 1 Rhizospheric soil samples collected from different cropping systems in smallholder farm fields.
2.2 Soil physico-chemical properties
Soil samples collected from various cropping systems as bulk soil (BS) and sterile soil (S) as described above (Table 1) were analyzed for micronutrients at Société Générale de Surveillance (SGS) Kenya Ltd Multi-laboratory, Nairobi, before the onset of the experiments in the greenhouse. Soil micronutrients including nitrogen (N), phosphorus (P), sodium (Na), organic carbon (OC), calcium (Ca), potassium (K), and other soil parameters such as potential of hydrogen (pH), electrical conductivity (EC) were measured following the methodology developed by Okalebo et al. (2002) and Sparks et al. (1996). Soil texture (silt, clay, and sand) and trace elements such as zinc (Zn), boron (B), cupper (Cu), iron (Fe), and manganese (Mg) were analyzed through the Bouyoucos hydrometer method as described by Beretta et al. (2014).
2.3 Plants
Maize seeds (SC Duma 43), a variety commonly cultivated by small-scale farmers in Western and Eastern Kenya, were obtained from Simlaw Seeds, Nairobi, Kenya. The seeds were surface sterilized with 70% ethanol for 30 seconds and rinsed with distilled water before planting in a sterilized five-liter plastic container pot (previously sterilized in 70% ethanol) in a greenhouse (25 ± 2°C during the day, 19 ± 2°C at night, and L12:D12 photoperiod) at icipe. Two seeds were planted in each conditioned soil type (MLI, MMC and S soils) collected from the farms within 48 h after sample collection. To prevent soil cross-contamination, precautions such as wearing gloves while handling soil from different fields, during measurements, and when dealing with the plant and FAW neonates were taken.
2.4 Insects
Using the protocol described by Onyango and Ochieng’-Odero (1994), FAW larvae were reared at the Animal Rearing and Containment Unit (ARCU), icipe Duduville campus on a maize-based artificial diet and leaves in 1000 mL plastic jar, with their lids infused with steel wire for airflow. Optimal conditions for larvae rearing were 27 ± 2°C, 75 ± 5% relative humidity (RH), and L12:D12 photoperiod until they pupated (Mutua et al., 2022). To develop into adults (moths), pupae were kept in cages measuring 40 × 20 × 20 cm with wet cotton wool to provide moisture, while matured moths were then transferred to oviposition cages of 80 × 50 × 70 cm. The matured moths were provided with maize plants for mating and as an oviposition substrate. They were also continuously provided with a 10% honey solution. To induce egg production, they were exposed to maize leaves and damp cotton cloth, acting as a water source. The eggs were collected and placed in plastic containers to regenerate the colony. One-day-old unfed FAW neonates were used for the experiments. The neonates were collected using a fine-camel brush. Second-generation insects were utilized in all tests. To avoid genetic degradation, laboratory-reared insects were infused with field-collected insects every two months to maintain their phenological characteristics.
2.5 Germination of maize seeds
Bulk soils (BS) collected from the various cropping systems (Table 1) were used to investigate the impact of soil conditioning on seed germination time and rate. In addition, the S soil was inoculated with 5% of the BS soils collected from different cropping systems above to form another type 5% IBS which was also used for the experiment. The BS and 5% IBS soils (Table 1) were distributed in plastic container pots (5 L) that were pre-sterilized with 70% ethanol. Two maize seeds were sown in each pot and kept in a greenhouse (27 ± 2°C, 75 ± 5% relative humidity (RH), and L12:D12). The experiment was replicated four times for each treatment with four experimental units in each replicate. In total, 384 seeds planted for the experiment. The planted seeds were watered daily with sterilized distilled water (0.2 L), and the germination was observed and recorded on daily bases. The time taken for the seed to germinate was expressed as germination time, while the germination rate in each treatment was calculated as the proportion of germinated seed from the total seeds that were planted. Three days post germination, the weakest seedling in each pot was thinned out and only one seedling per pot was kept for the subsequent experiments. At the end, each treatment (each cropping system in each soil conditioned type) had 16 plants for the subsequent experiments.
2.6 Plant growth parameters
Maize plant growth parameters including the number of leaves, stem diameter, plant height, and chlorophyll content for each maize grown in each soil type were measured weekly for three weeks’ duration (W1, W2 and W3) (Chiriboga et al., 2021). Plant height was measured using a meter tap from the soil lines to the uppermost leaf’s arch. The chlorophyll content of the leaves was measured using a chlorophyll meter (SPAD-502) (Konica Minolta Sensing, Inc., Japan). Vernier callipers (Toolstream Ltd. BA22 BHZ, United Kingdom) were used to measure the diameter of the plant stem. After 21 days of growth, four plants from each soil type were cut at the baseline. Thereafter, the soil was removed from the shoots and roots by washing them with excess water and then dried with a paper towel. Maize plant biomass was determined by weighing fresh and dry root and shoot using a weighing balance (Kern & Sohn GmgH, D-72336 Balingen, Germany).
2.7 Larval feeding assay
A no-choice feeding assay was conducted in the laboratory to determine FAW leaf feeding on maize plants grown on BS and 5% IBS soils (Table 1). For each soil type, four maize plants that are three weeks old were selected. This is the age when maize is highly preferred and susceptible to FAW (De Lange et al., 2020). For each plant, leaf discs of 15 mm diameter were removed from the second youngest fully expanded maize leaf and used for the constitutive feeding experiment. The leaf disc was placed in a 30 mL transparent plastic cup (TPC) that was midway filled with technical agar #3 (TA3), to prevent leaf discs from desiccating. Ten unfed FAW neonates were placed on the leaf disc within the media TPC, sealed with parafilm to prevent the neonates from escaping (Mutyambai et al., 2019), and a slit was made with a sharp scalpel at the cup lid for air circulation. The neonates were allowed to feed for 24 h, and thereafter the leaf discs were photographed to assess the area consumed by the neonates using ImageJ software (National Institutes of Health, Bethesda, USA) (Schneider et al., 2012). A similar experiment was conducted after initial exposure of maize plant for 24 h (induced assay) with 10 FAW neonates in a greenhouse.
2.8 Growth and development of Spodoptera frugiperda larvae
This experiment was conducted to investigate the effects of maize plants grown on BS and 5% IBS soils (Table 1) on the fitness performance of FAW neonates under semi-field conditions in a greenhouse. Ten neonates were placed on a three-week-old undamaged maize whorl plant using a fine camel-hair brush, and allowed to feed for 15 days. The plants were placed on metal stands elevated above the ground and covered with sticky glue to prevent predators like ants from climbing up the stands. A 0.2 L of sterilized distilled water was used to water the 5 L pot daily at the base of each maize plant. After 15 days, larval survival rates, length, weight, and instar stage of development were recorded from each replicate per cropping system (Mutua et al., 2022). The experiment was replicated four times for each soil type.
2.9 Data analyses
All data were subjected to a normality test using Shapiro-Wilk test. Soil physico-chemical properties, plant growth, larval feeding and development data obtained from each soil type (Table 1) were analyzed using one-way ANOVA. Tukey’s post hoc test was used to carry out a pairwise comparison for mean separation at α = 0.05. The germination time data were analyzed using a generalized linear model (GLM) with Poisson distribution since they were not normally distributed. A two-sample (unpaired) Student’s t-test was used to compare constitutive and induced larval feeding for each soil type. Pearson correlation analysis was used to determine the relationship between soil physico-chemical properties and plant growth parameters. The principal component analysis (PCA) was conducted to determine the relationship between soil physico-chemical parameters and the different cropping systems. All analyses were carried out using R (v4.1.2.) statistical software packages (R Core Team, 2018).
3 Results
3.1 Soil physico-chemical properties
Bulk soils (BS) obtained from different cropping systems (MBB, MGG, MPP, MCB, MMC) and control (S) soils significantly varied in their physico-chemical characteristics (F5,30 = 44.27, P = 0.001; F5,30 = 2.610, P = 0.01; F5,30 = 1.128, P = 0.05; F5,30 = 2.708, P = 0.01; F5,30 = 6.132, P, 0.001; F5,30 = 2.218, P = 0.01; F5,30 = 4.574, P = 0.001; F5,30 = 2.232, P = 0.02; F5,30 = 2.653, P = 0.01; F5,30 = 17.200, P = 0.001; F5,30 = 13.600, P = 0.001; F5,30 = 2.685, P = 0.01; and F5,30 = 3.635, P = 0.01; for pH, EC, K, Ca, Mg, EA, Fe, Cu, N, OC, clay and silt; Table 2). There were significant differences across soil physico-chemical parameters in different cropping systems, except for Na, Mn, Zn, S and sand. We observed that the lowest pH was recorded on MMC and S soils. Overall, BS soils obtained from different MLI systems had a higher pH, EC, K, Ca, Mg and Cu soil parameters compared to MMC and S soil (Table 2).
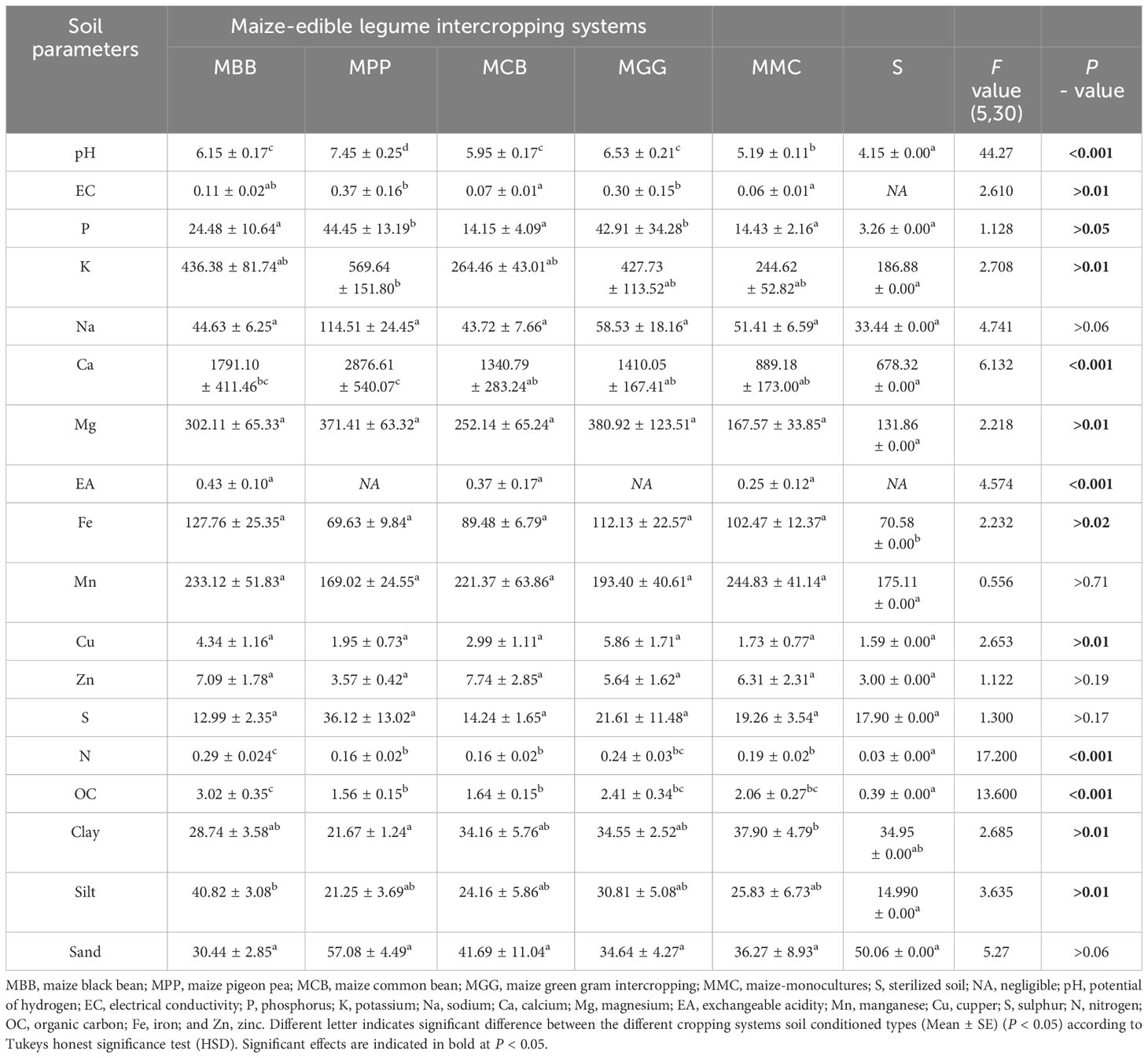
Table 2 Physico-chemical characteristics of soil conditioned by different maize-legume intercropping, maize-monoculture cropping systems and the sterilized soil control.
There is a strong correlation between soil conditioned by MLI and MMC cropping systems (principal component biplot (PCA)). A strong positive correlation was observed between soil conditioned by MBB and MPP on parameters such as S, Na, EC, K, pH, Ca and Mg (Figure 1). MGG and MCB cropping system correlated negatively with N, OC, sand and silt. These results indicate that MLI systems have a significant influence on soil properties, making them favorable for farm mechanization. In contrast, the MMC cropping system has no impact on these properties. It’s worth noting that PCA1 and PCA2, which collectively account for 58.2% of the total variance, played a vital role in elucidating the interactions between the selected soil properties (Figure 1).
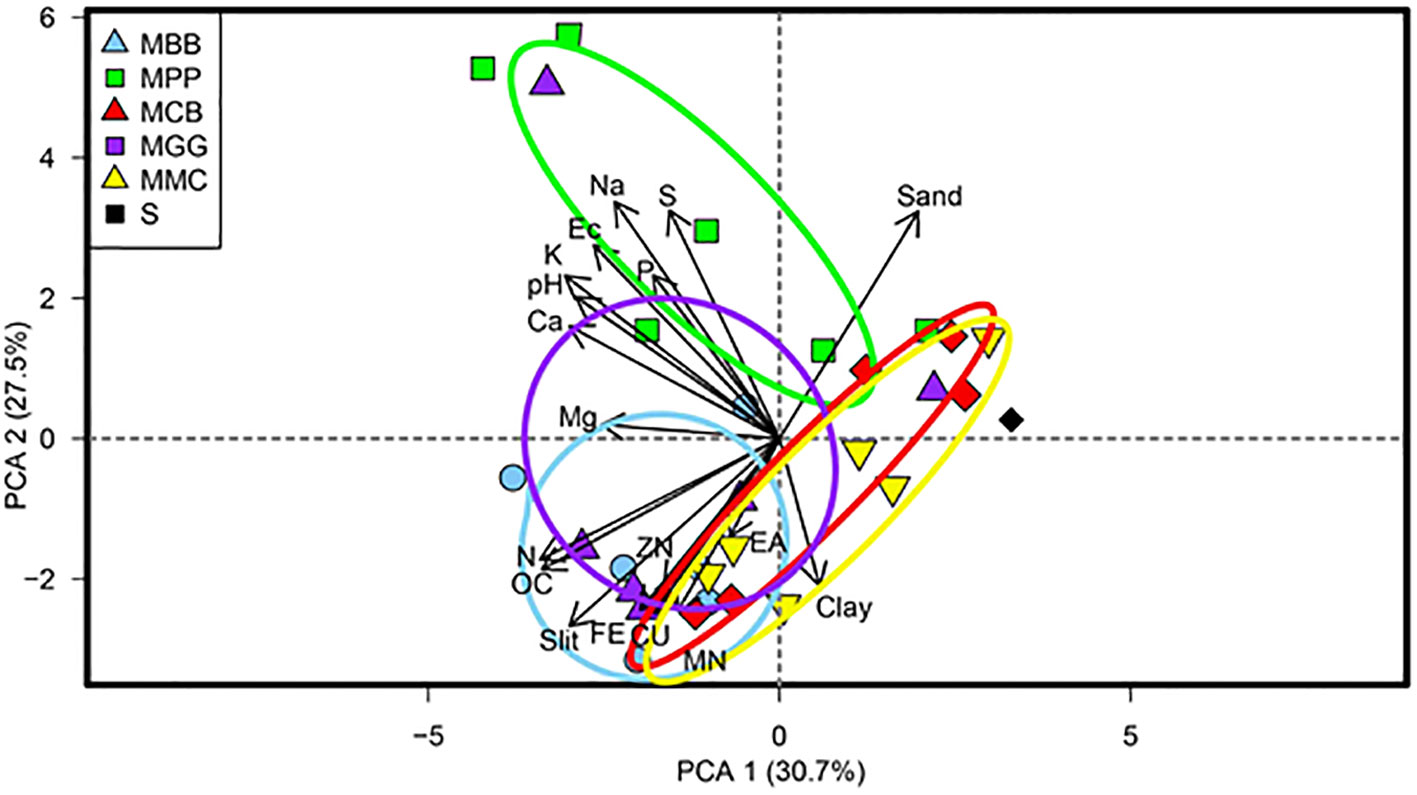
Figure 1 Principal component biplot, for the relationship between soil physico-chemical properties of different cropping systems soil conditioned. Principal components 1 = PCA1 and principal components 2 = PCA2. MBB, maize black bean; MPP, maize pigeon pea; MCB, maize common bean; MGG, maize green gram intercropping; MMC, maize-monocultures; S, sterilized soil; pH, potential of hydrogen; EC, electrical conductivity; P, phosphorus; K, potassium; Na, sodium; Ca, calcium; Mg, magnesium; EA, exchangeable acidity; Mn, manganese; Cu, cupper; S, sulphur; N, nitrogen; OC, organic carbon; Fe, iron; and Zn, zinc.
3.2 Germination rate and time of maize seeds
3.2.1 Germination rate
SC Duma 43 maize seeds planted in different cropping systems conditioned BS (MMP, MPP, MCB, MGG, MMC and S) significantly affected seed germination rate (ANOVA: F5,18 = 6.99, P < 0.001). The germination rate was significantly higher in MPP and MGG soils followed by MBB and MMC soils (Figure 2A). Similar trends were observed for S soil inoculated with 5% IBS where the germination rate was significantly higher in MPP and MGG soils (ANOVE: F5,18 = 4.89, P < 0.001) (Figure 2B).
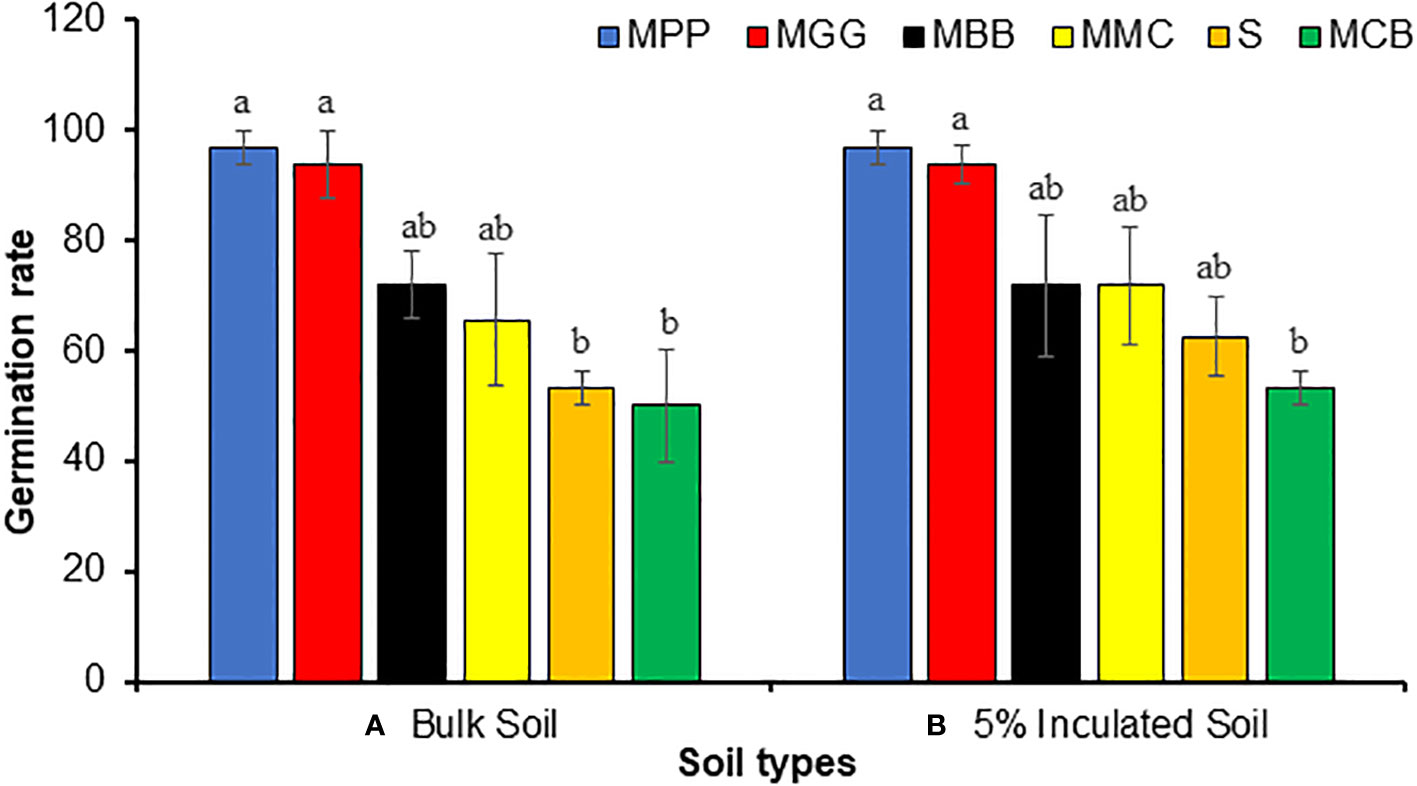
Figure 2 Mean (% ± SE) germination rate of SC Duma 43 maize seeds planted in soil conditioned by different cropping systems. (A) Bulk soils (BS) collected from smallholder farms with different cropping systems; MBB, maize black bean; MPP, maize pigeon pea; MCB, maize common bean; MGG, maize green gram intercropping; MMC, maize-monocultures; S, sterilized soil; (B), sterile soil inoculated with 5% soil conditioned by different BS cropping systems (5% IBS). Different letters above the bars indicate a significant difference (P < 0.05) between the means. N = 384 plants.
3.2.2 Germination time
The time taken for SC Duma 43 maize seeds to germinate significantly varied across BS. The germination time was significantly lower in MBB and MPP soils which was on average 4 days after planting (GLM: χ2 = 8.98, df = 135, P = 0.01) (Figure 3A). However, seeds planted in MMC and S soil took on average 6 days to germinate (Figure 3A). On the other hand, there was no significant difference in germination time for the seeds planted in 5% IBS (MPP, MGG, MBB, MMC, MCB) (GLM: χ2 = 12.31, df = 143, P = 0.589) (Figure 3B).
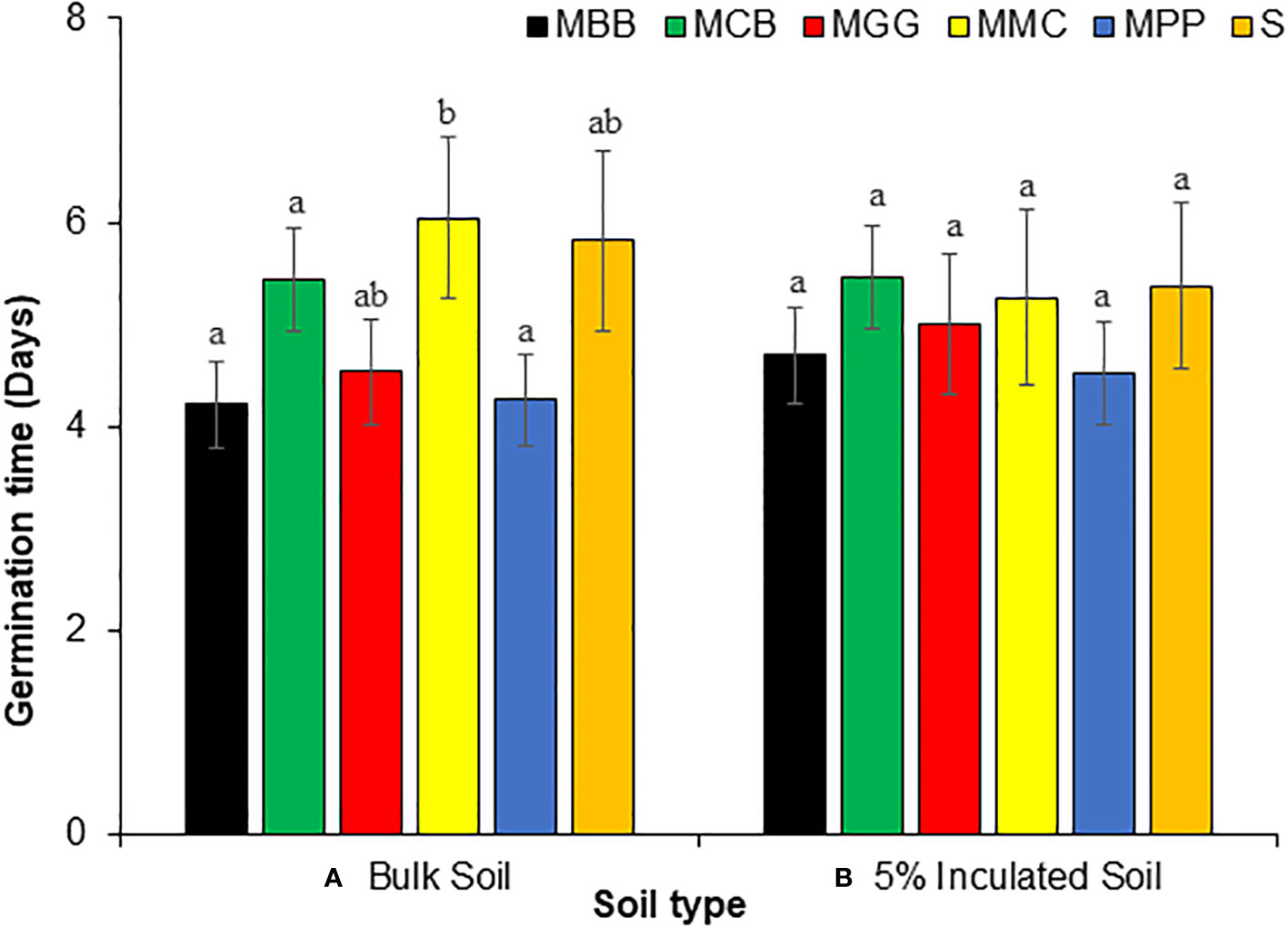
Figure 3 Germination time (in days) (Mean ± SE) taken by SC Duma 43 maize seeds grown in soils conditioned by different cropping systems. (A) Bulk soils (BS) collected from smallholder farms with different cropping systems; MBB, maize black bean; MPP, maize pigeon pea; MCB, maize common bean; MGG, maize green gram intercropping; MMC, maize-monocultures; S, sterilized soil; (B), sterile soil inoculated with 5% soil conditioned by different BS cropping systems (5% IBS). Different letters above the bars indicate a significant difference (P < 0.05) between the means. N = 384 plants.
3.3 Maize plant growth parameters
3.3.1 Plant height
There were significant differences in plant height for maize grown in BS and 5% IBS soils during the first week (W1), second week (W2) and third (W3) weeks after germination (ANOVA: F5,18 = 33.77, P < 0.001), (ANOVA: F5,18 = 21.37, P < 0.001) and (ANOVA: F5,18 = 33.77, P < 0.001), respectively (Figure 4). Among BS soils, maize planted in MCB soil were significantly higher in W1 and W2 after germination, compared to other soils (S, MMC, MBB, and MGG). However, during W3, the height of maize plants grown in MPP soil was significantly higher at ≈ 35 cm, followed by MCB and MBB soils (P < 0.001). Plant height in S, MMC and MMG soils were relatively similar and shorter across W3 duration. We observed similar trends in 5% IBS soils. In W1, the plant height was significantly higher in MCB followed by MPP soil, while it was lowest in MGG and S soil (P < 0.001). During W2, the plants were significantly taller in MPP followed by MCB and then MBB soils. Although plants in MGG soil were short during the first two weeks, they reached ≈ 30 cm in W3, accounting for the tallest level across the 5% IBS soils. Indeed, the plants in S soil were shorter across the three weeks regardless of soil type (BS and 5% IBS) or cropping system (Figure 4).
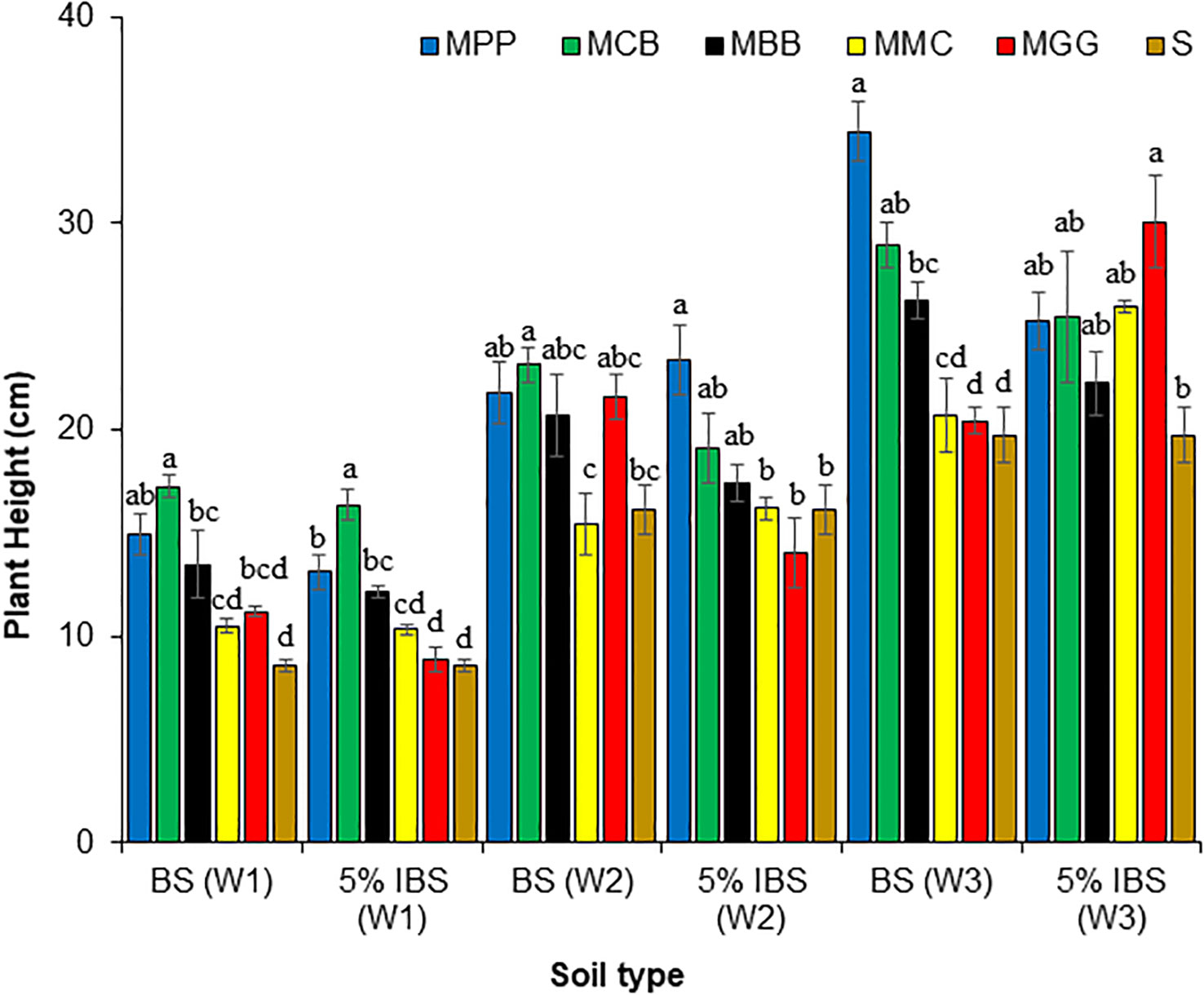
Figure 4 Maize plant height (cm) (Mean ± SE) in first (W1), second (W2), and third (W3) weeks for plants grown in soils conditioned by different cropping systems. Bulk soils (BS) collected from smallholder farms with different cropping systems with MBB, maize black bean; MPP, maize pigeon pea; MCB, maize common bean; MGG, maize green gram intercropping; MMC, maize-monocultures; S, sterilized soil; (5% IBS), sterile soil inoculated with 5% soil conditioned by different cropping systems. Different letters above the bars in each week (W1, W2, W3) for each soil’s conditioned cropping system type (BS and 5% IBS) indicate a significant difference (P < 0.05) between the treatments. N = 384 plants.
3.3.2 Plant diameter
There were significant differences in maize plant diameter between maize plants grown in BS and 5% IBS soils during W1, W2 and W3 after germination (ANOVA: F5,18 = 33.77, P < 0.001), (ANOVA: F5,18 = 21.37, P < 0.001) and (ANOVA: F5,18 = 33.77, P < 0.001), respectively (Figure 5). In BS, maize plant diameter was significantly wider (P < 0.001) for plants grown in MPP compared to MGG, S, and MMC soils during W1. In W2, the diameter of maize plants grown in MPP soil was statistically different from those grown in MMC, MBB and S (P < 0.001) soils. In W3, the diameter of maize plants grown in MBB soil was significantly wider compared to the diameters of plants grown in MMC, MGG and S soils (P < 0.001). We observed similar trends when the experiment was set in 5% IBS soil. The diameter of maize plants grown in 5% inoculated soil by MCB soil was significantly higher than plants grown in S soil (P < 0.001), but MCB was not significantly different from maize plant diameters grown in S soil inoculated with 5% of MGG, MBB, and MPP soils during W1 after germination (P < 0.05). During W2 and W3, there were no significant differences between maize plant diameters grown in all 5% IBS soils (P < 0.05, Figure 5).
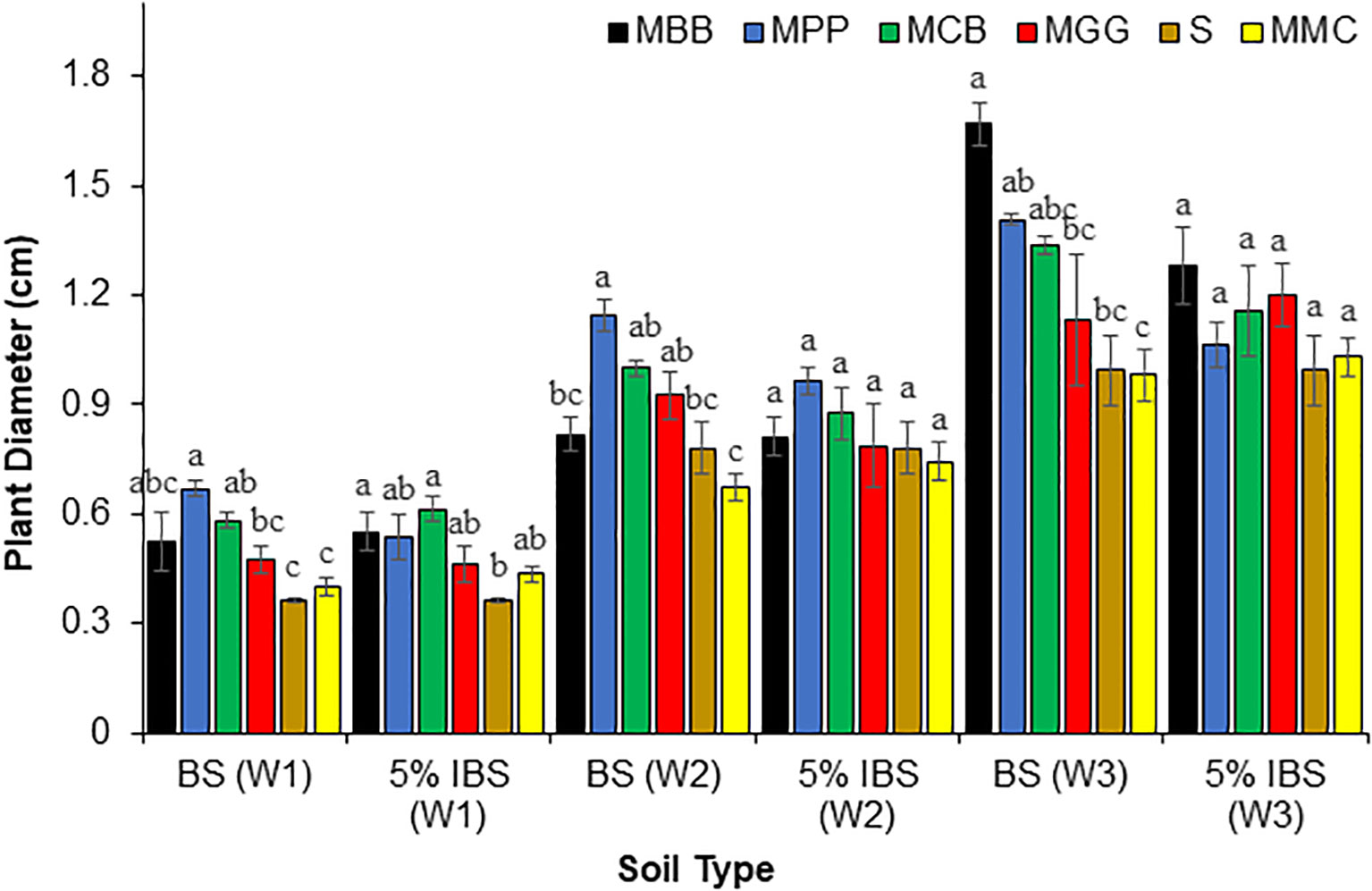
Figure 5 Diameter (cm) (Mean ± SE) of maize plants in the first (W1), second (W2), and third (W3) weeks for plants grown in soil conditioned by different cropping systems. Bulk soil (BS) collected from smallholder farms with different cropping systems; MBB, maize black bean; MPP, maize pigeon pea; MCB, maize common bean; MGG, maize green gram intercropping; MMC, maize-monocultures; S, sterilized soil; S soil inoculated with 5% soil conditioned by different cropping systems (5% IBS). Different letters above the bars in each week (W1, W2, W3) for each soil conditioned type (BS and 5% IBS) indicates a significant difference (P < 0.05) between the treatment. N = 384 plants.
3.3.3 Number of maize leaves
There were significant differences in the number of maize leaves of plants grown in BS and 5% IBS soils during W1, W2 and W3 after germination (ANOVA; F5,18 = 33.77, P < 0.001), (ANOVA: F5,18 = 21.37, P < 0.001) and (ANOVA: F5,18 = 33.77, P < 0.001), respectively (Figure 6). During W1 of BS soils, the number of leaves per plant was significantly higher for plants grown in MCB and MBB soils compared to the other soil types (P < 0.001). A similar trend was observed among the BS soils in W2 after germination where the number of leaves was significantly higher in MPP, MBB and MCB soil types. During W3, the number of leaves of maize plants grown in MPP soils was significantly different from MGG, MMC, MCB and S soils (P < 0.001). We observed similar trends with experiment with soils inoculated with 5% IBS, where the number of leaves was significantly higher in sterile soil inoculated with 5% different soil types (MCB, MBB, and MPP) in W1, W2 and W3 respectively after germination (P < 0.001). However, the number of leaves was lowest in plants grown in S soil inoculated with 5% of MMC, MGG and S soils, regardless of the weeks after germination (Figure 6).
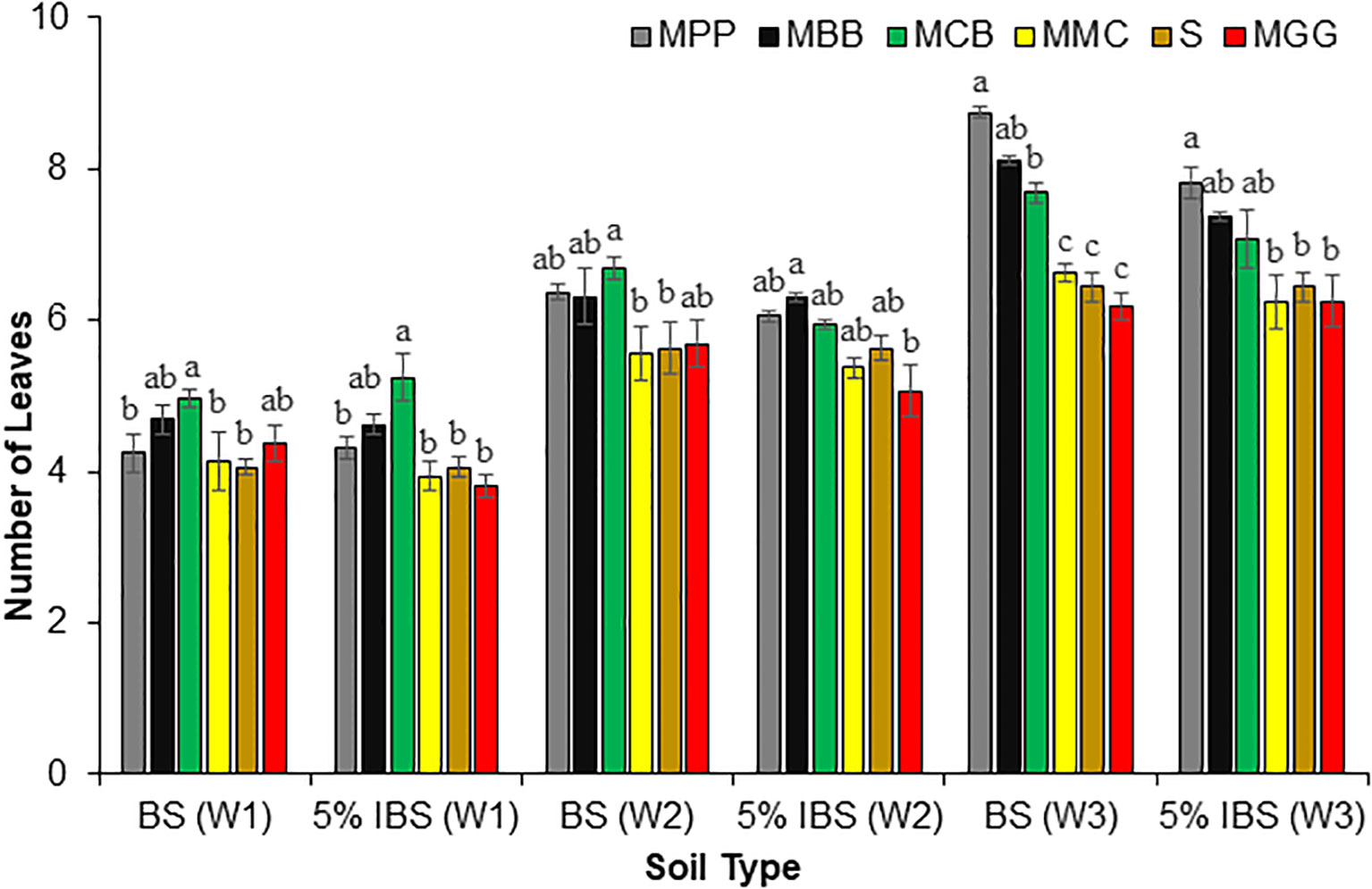
Figure 6 Number of maize plant leaves (Mean ± SE) in first (W1), second (W2), and third (W3) weeks for plants grown in soil conditioned by different cropping systems. Bulk soil (BS) collected from smallholder farms with different cropping systems; MBB, maize black bean; MPP, maize pigeon pea; MCB, maize common bean; MGG, maize green gram intercropping; MMC, maize-monocultures; S, sterilized soil; S soil inoculated with 5% soil conditioned by different cropping systems (5% IBS). Different letters above the bars in each week (W1, W2, W3) for each soil conditioned type (BS and 5% IBS) indicate a significant difference (P < 0.05) between the treatments. N = 384 plants.
3.3.4 Leaf chlorophyll content
The leaf chlorophyll content of maize plants grown in BS and 5% IBS soils were significantly different in W1, W2 and W3 after germination (ANOVA: F5,18 = 27.05, P < 0.001), (ANOVA: F5,18 = 19.64, P < 0.001) and (ANOVA: F5,18 = 47.55, P < 0.001), respectively (Figure 7). Among soil conditioned by BS, the chlorophyll content throughout the experimental period was higher on the leaves of maize plants grown in MBB, MPP and MCB soil types (P < 0.001). However, chlorophyll content on the leaves from MGG, MMC and S soil types, were the lowest, during all the weeks after germination. Similar trend was observed for a plant grown in 5% IBS soils, where chlorophyll content was higher in sterile 5% soil (MBB, MPP, MCB and MGG soils) during the three weeks of the experiment. Indeed, chlorophyll content was lowest in MMC and S soil regardless of soil type (BS and 5% IBS) or duration of the plant after germination (W1, W2, and W3) (Figure 7).
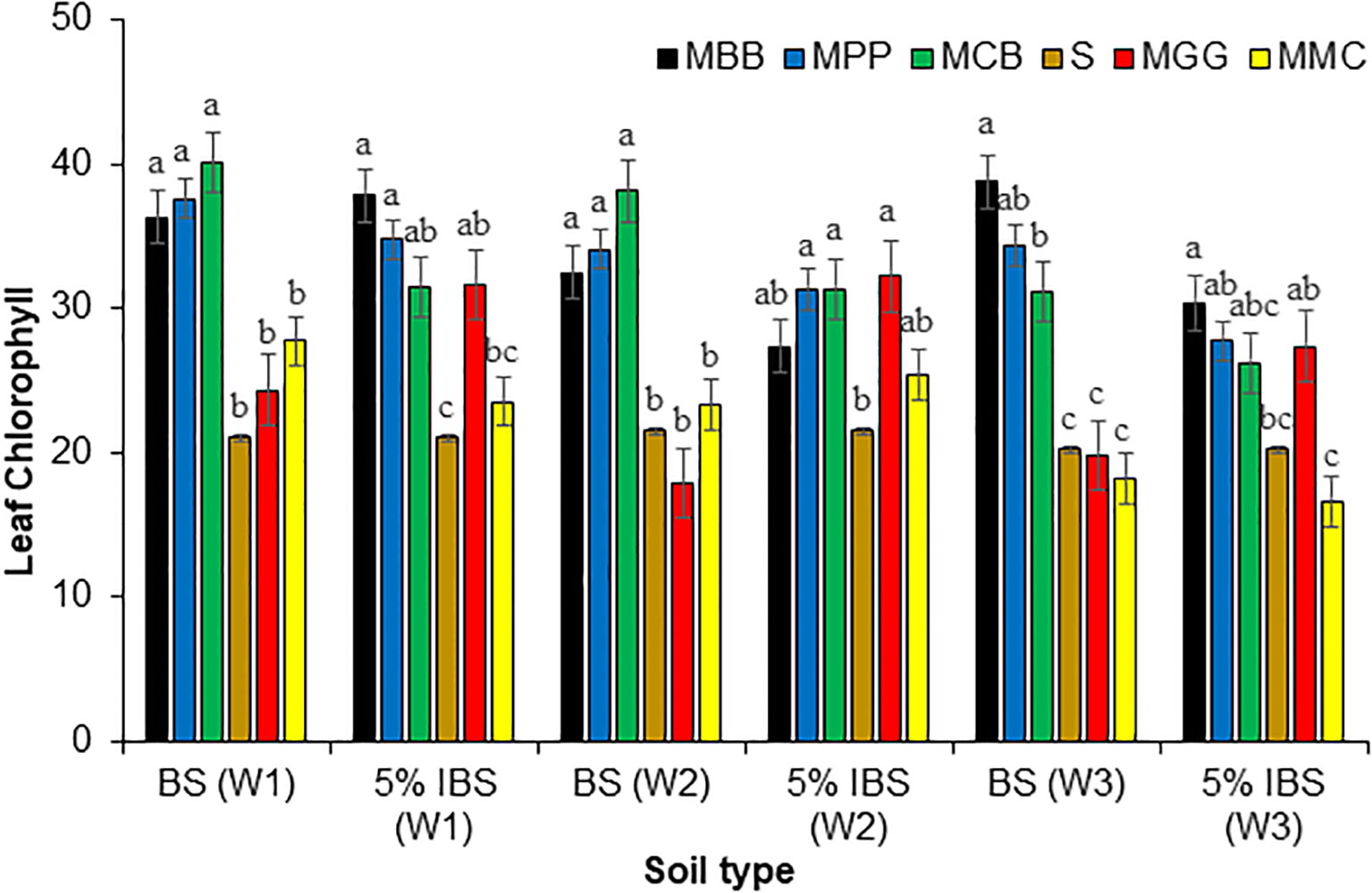
Figure 7 Leaf chlorophyll (Mean ± SE) content of maize plant in first (W1), second (W2), and third (W3) weeks for plants grown in soil conditioned by different cropping systems. Bulk soils (BS) collected from smallholder farms with different cropping systems; MBB, maize black bean; MPP, maize pigeon pea; MCB, maize common bean; MGG, maize green gram intercropping; MMC, maize-monocultures; S, sterilized soil; S soil inoculated with 5% soil conditioned by different cropping systems (5% IBS). Different letters above the bars in each week (W1, W2, W3) for each soil conditioned type (BS and 5% IBS) indicate a significant difference (P < 0.05) between the treatments. N = 384 plants.
3.3.5 Plant biomass
Shoot fresh (SFW) and dry weight (SDW) of maize plants grown in BS and sterilized soils were significantly different (ANOVA: F5,18 = 33.77, P < 0.001) and (ANOVA: F5,18 = 21.37, P < 0.001), respectively (Table 3). Plants grown in MPP and MCB soils recorded the highest SFW and SDW when compared to MBB, MGG, MMC and S soils. Similarly, the highest root fresh (RFW) and dry weight (RDW) was recorded for plants grown in MPP and MCB soils, compared to other soil types (ANOVA: F5,18 = 12.92, P < 0.001) and (ANOVA: F5,18 = 28.64, P < 0.001). We observed similar trends when the above experiment was conducted in 5% IBS (MBB, MPP, MCB, MGG and MMC soils, Table 3).
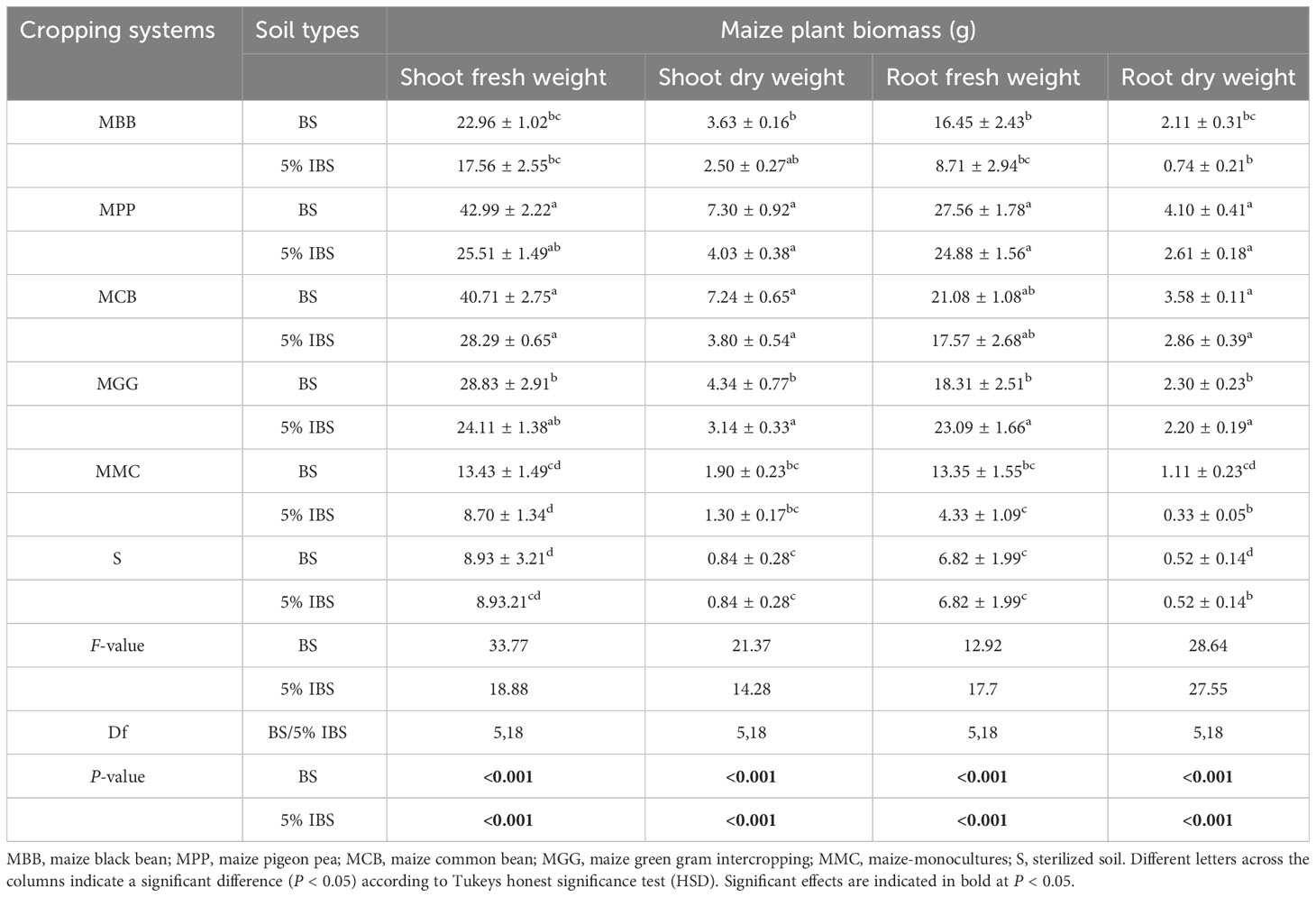
Table 3 Maize plant biomass after three weeks of germination for plants grown in soil conditioned by different maize-edible legume intercropping, maize-monoculture cropping systems and sterilized soil.
3.3.6 Correlation between maize plant biomass and soil physico-chemical parameters
We found a negative correlation between pH in BS and plant biomass (SFW, RFD, SDW and RDW). This correlation was strong with a significant correlation coefficient (r) ranging between -0.65 and -0.77 for SFW, SDW and RDW (P < 0.05, Table 4). The SFW, SDW, RFW and RDW were significantly and positively correlated with EA in BS soils, with r ranging between 0.62 and 0.69. However, the correlation between plant biomass and P, K, Mg, N, OC, Fe and Zn in BS was weak and not significant. For maize grown in 5% IBS soils, a similar trend as above was observed where soil pH negatively correlated with plant biomass (Table 4). In addition, Na and Ca in 5% IBS soil had a moderate negative correlation with plant biomass, but the correlation was not significant (Table 4).
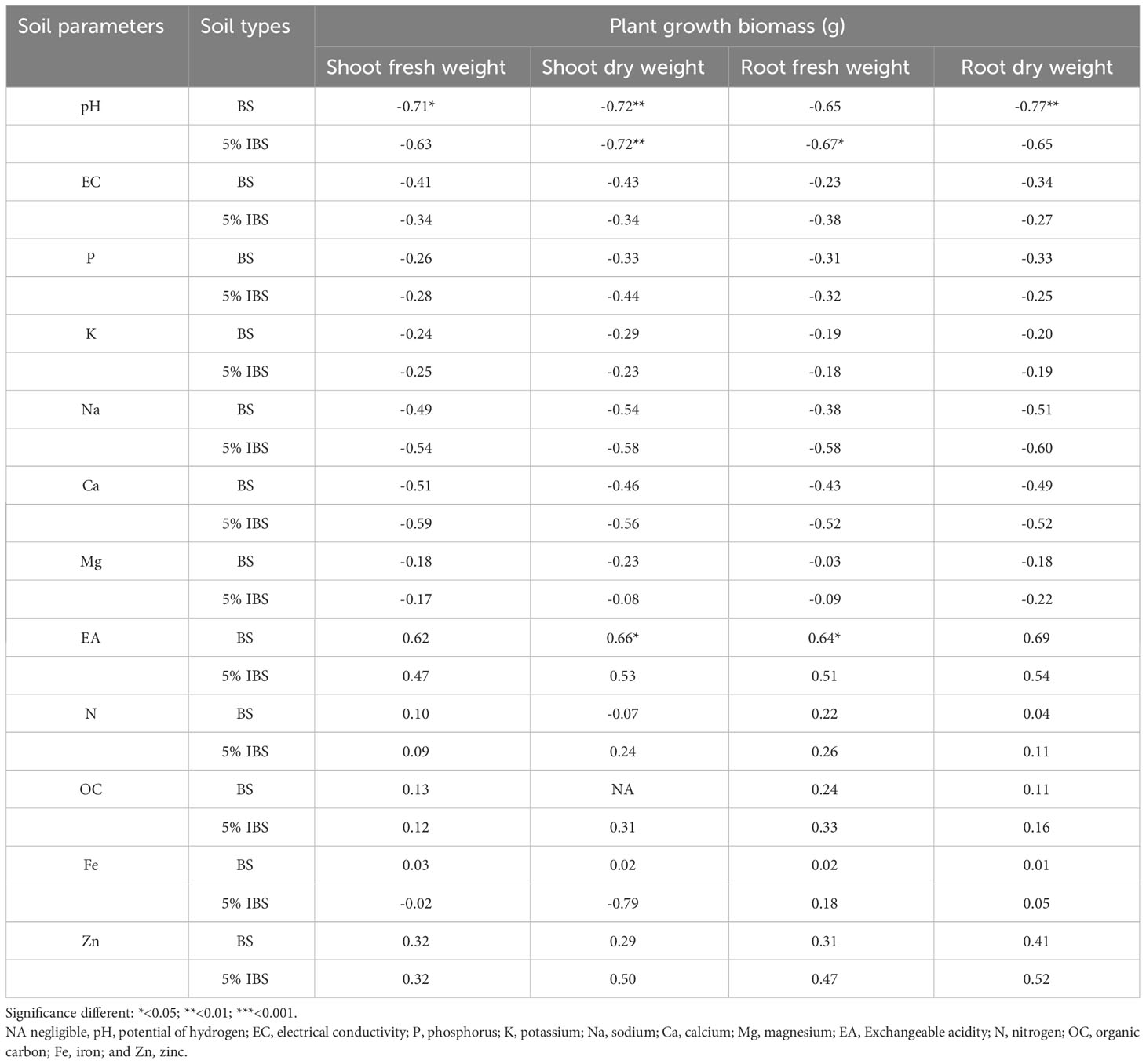
Table 4 Correlation between maize plant biomass (plant grown in bulk conditioned soil) (in gram) and soil physico-chemical properties.
3.3.7 Correlation between maize plant growth and soil physico-chemical parameters
The pH in BS soils had a strong negative correlation with leaf number (NL), leaf chlorophyll content (CC), plant diameter (PD) and plant height (PH) (P < 0.05) (Table 5). However, other parameters of BS had a weak correlation with plant growth parameters except, EA which had a moderate positive and negative significant correlation with NL. Similar correlation trends were observed when maize plants grown in 5% IBS (Table 5). However, all correlation coefficients between plant growth parameters and 5% IBS soil parameters were not significant (Table 5).
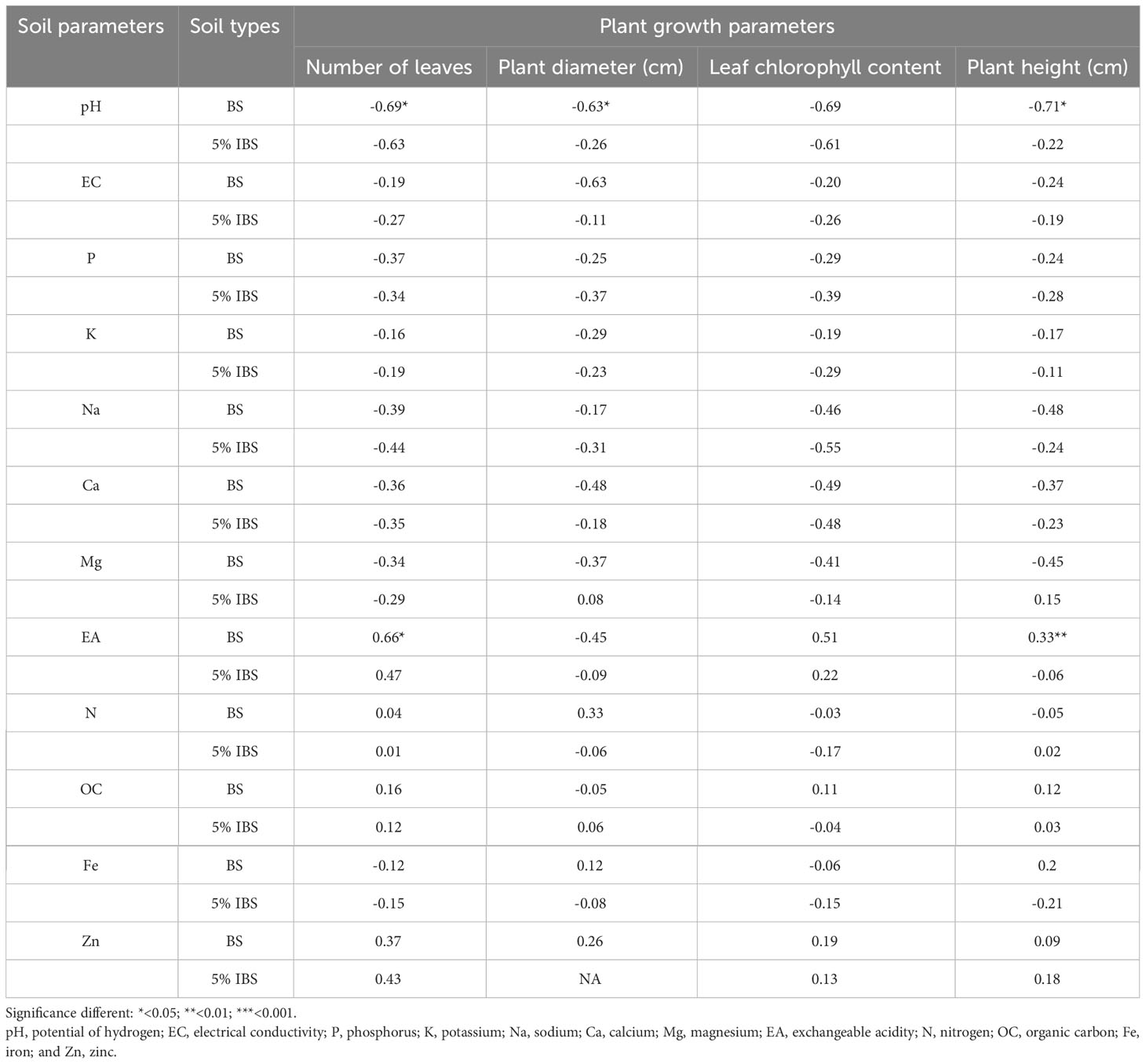
Table 5 Correlation between maize plant growth parameters in bulk soils conditioned and soil physico-chemical properties.
3.4 Spodoptera frugiperda larval feeding
A comparison of leaf discs consumed area between different soil types (MMC, S, MBB, MPP, MCB and MGG) is illustrated in Figure 8. A significant difference was detected in leaf consumed area of constitutive and induced leaf disc plants grown in BS soils (F5,18 = 19.58, P < 0.001); (F5,18 = 2 3.8, P < 0.001, respectively) (Figure 8). For plants grown in BS soils, there was increased consumption in leaf disc of plant grown in MMC and S soil in both constitutive and induced assay. Similar results were obtained when the experiment was conducted with a plant grown in 5% IBS. Consumption by FAW neonates was higher in leaf discs of plants grown in 5% IBS conditioned by MMC soil type (Figure 8).
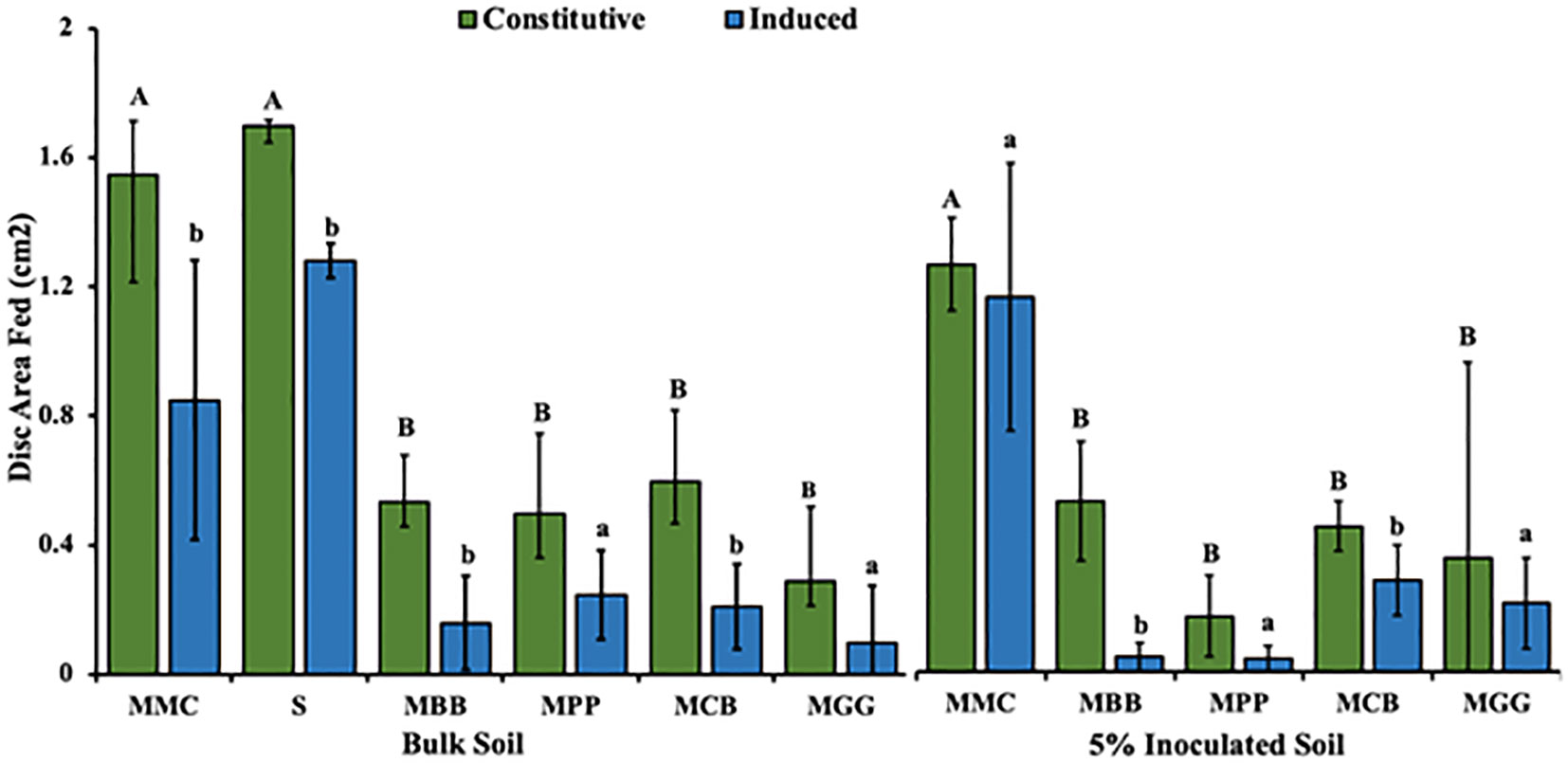
Figure 8 Mean ( ± SE) of maize leaf disc fed by Spodoptera frugiperda neonates. (BS) Bulk soil, soil conditioned by different cropping systems collected from smallholder farms; MBB, maize black bean; MPP, maize pigeon pea; MCB, maize common bean; MGG, maize green gram intercropping; MMC, maize-monocultures; S, sterilized soil; S soil inoculated with 5% soil conditioned by different cropping systems (5% IBS). Different capital letter above the error bars indicates significantly different across the different cropping systems soil conditioned types (P < 0.05).
Comparison between constitutive and induced feeding assay for each different cropping system in each soil type is presented in Figure 9. Overall, the disc consumed area by FAW neonates was significantly higher in constitutive feeding assay, compared to induced feeding assay irrespective of soils types (both BS and 5% IBS soils).
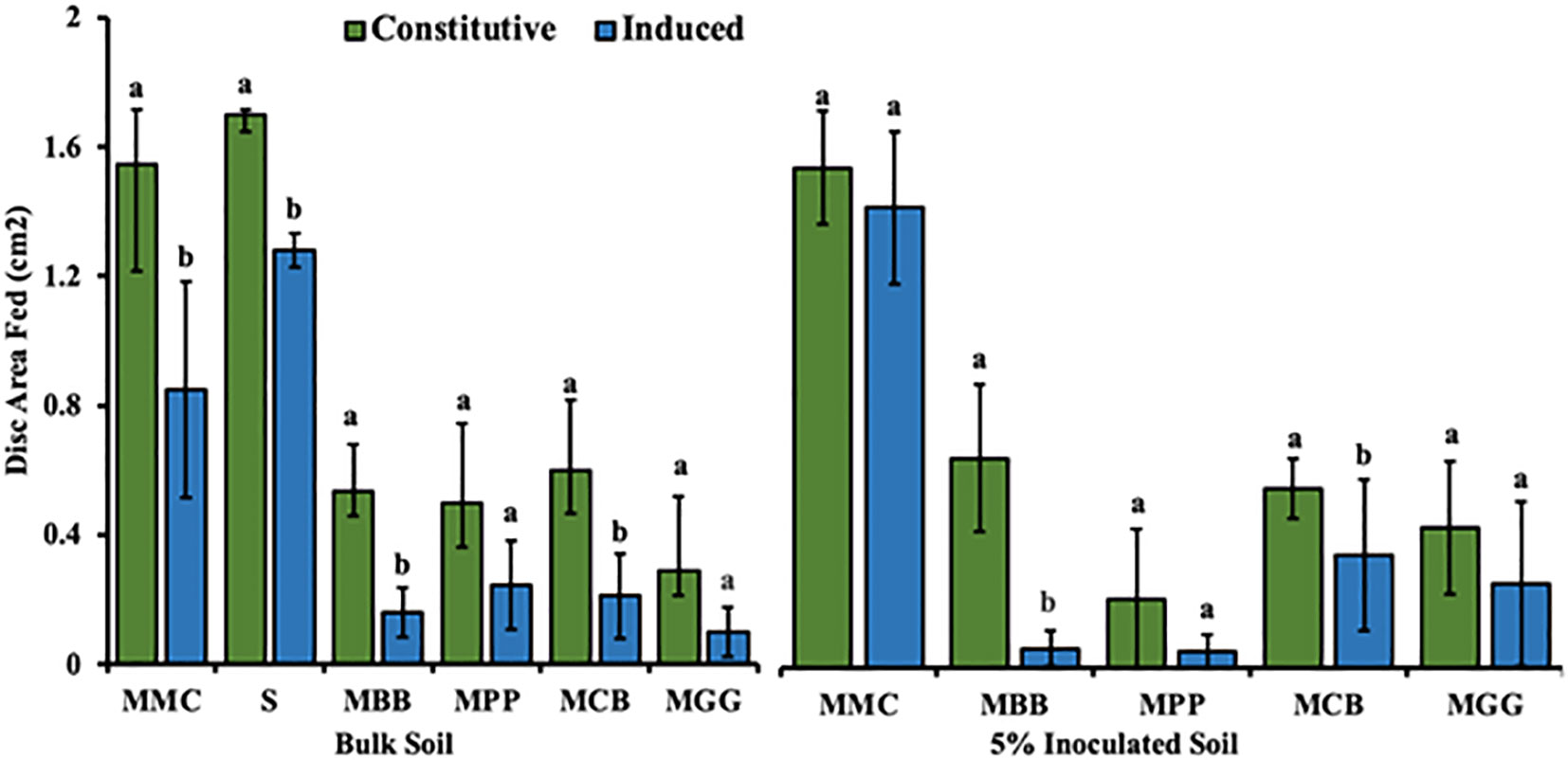
Figure 9 Comparison of leaf disc (Students T-test (Mean ± SE)) fed by Spodoptera frugiperda neonates through constitutive against induced feeding. Bulk soil, soils conditioned by different cropping systems from smallholder farms; MBB, maize black bean; MPP, maize pigeon pea; MCB, maize common bean; MGG, maize green gram intercropping; MMC, maize-monocultures; S, sterilized soil; S soil inoculated with 5% soil conditioned by different cropping systems (5% IBS). Different capital letter above the error bars indicates significantly different across the different cropping systems soil conditioned types (P < 0.05).
3.5 Spodoptera frugiperda larval growth and development
There were significant differences in FAW larval weight (FLW) when neonates were exposed for 15 days in the greenhouse on maize plants grown in BS soil types (F5,18 = 14.58, P < 0.001) (Table 6). Fall armyworm larval weight of MCB, MPP and MBB soil was less compared to those grown in MMC, S and MGG soils (P < 0.001). FAW larval lengths (FLL) were also significantly different across BS soils (F5,18 = 16.12, P < 0.001). The smallest FAW larvae length (FLL) was observed in MBB and MCB soils. Moreover, there were significant differences in FAW larval instar (FLI) across BS soils (F5,18 = 27.36, P < 0.001). The survival rates of FAW larvae number (FLN) on maize plants grown in BS soil types were significantly higher in S, MGG and MMC cropping systems (F5,18 = 33.53 P < 0.001) (Table 6). Similar results as above were obtained when the experiment was conducted in 5% IBS (Table 6).
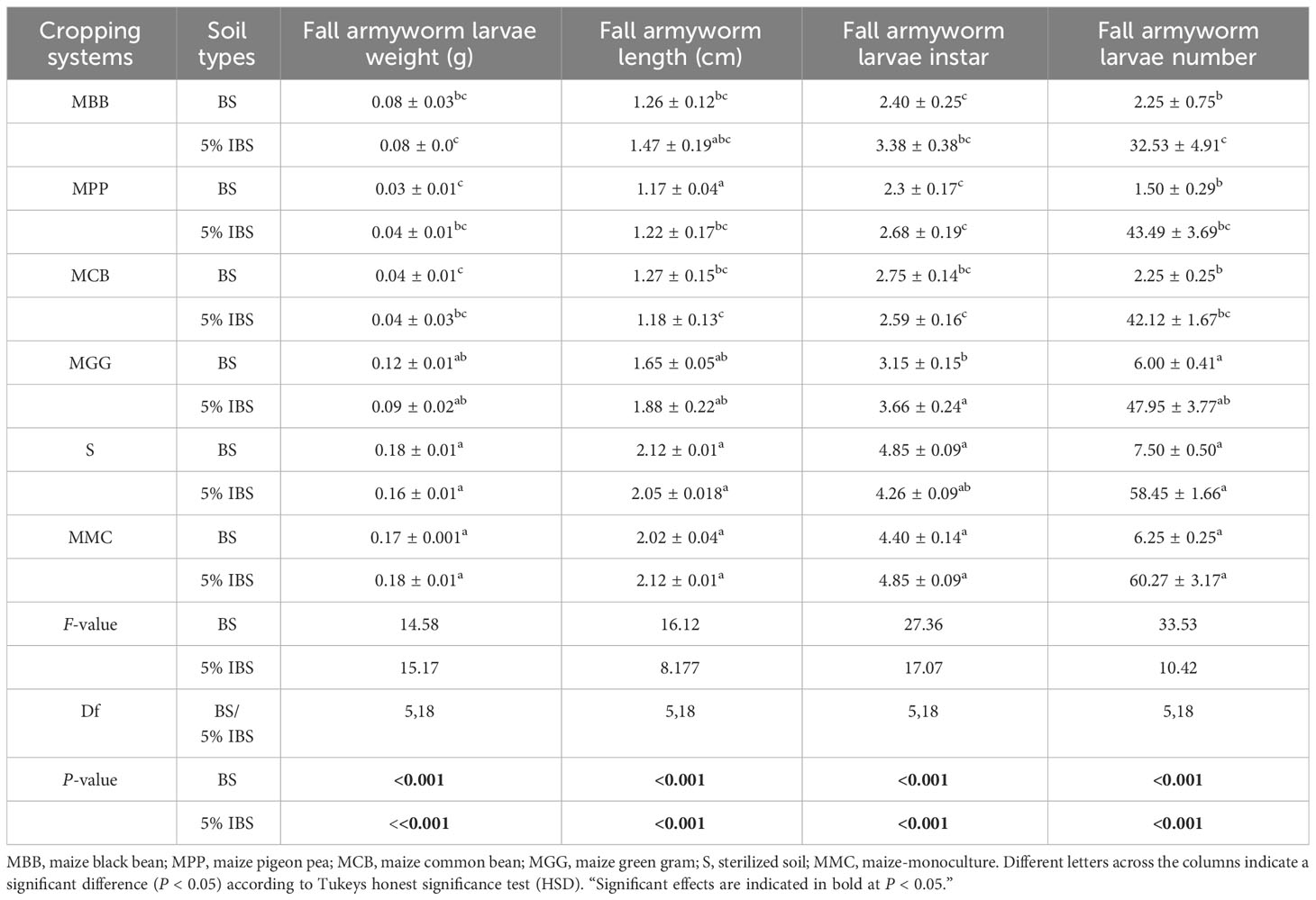
Table 6 Effects of soil conditioned by different cropping systems on Spodoptera frugiperda larval growth and development on bulk soil.
4 Discussion
We showed here that maize-legume intercropping system’s soil legacies positively impact seed germination, maize plant growth, soil physico-chemical properties, health, and FAW resistance by reducing larval feeding on maize plants constitutively and when feeding is induced. Thus, our findings bring to fore an additional pest-regulating effect of MLI systems on the widespread maize pest FAW which is novel to SSA. Notably, in this case, plant-soil feedback effects on maize-plant performance not only reduce FAW feeding but also mediate the enhancement of soil fertility, thus improving maize plant growth. Through this different larval feeding, MLI systems affect insect pest pressure. The findings here suggest a significant mechanism that involves feedback of maize-legume intercropping system-mediated pest resistance that is facilitated by soil conditioning.
There is a body of literature which indicates that prior vegetation contributes significantly to soil properties and communities, with a cascading effect on successive crops (Kaplan et al., 2018). The physico-chemical properties of soil can enhance plant growth by providing significant micronutrients (Agegnehu et al., 2016; Van der Putten et al., 2016; Pervaiz et al., 2020). We observed that the increased levels of certain soil micronutrients (pH, N, OC, P and Zn) can significantly improve maize growth and biomass. Soil OC levels serve as a reliable indicator for soil, plant health and yield (Li et al., 2020; Ndayisaba et al., 2022). Our study found that soil conditioned by MLI systems had higher OC levels than soil conditioned by MMC and S systems. Furthermore, most rhizosphere samples from conditioned soil by MLI systems exhibited high levels of pH, N, K, and P, while soil conditioned by MMC and S systems had a pH below 5.5. Studies have shown that a pH below 5.5 can be detrimental to agroecosystems because it negatively affects plant growth and environment interactions (Wahome et al., 2023), indicating that the soil pH in MMC and S poses a significant threat to farming systems (Delgado and Gómez, 2016). This finding corresponds with the effect of multiple cropping systems on soil characteristics such as pH, OC, P, and N compared to monoculture (Chen et al., 2021; Chen et al., 2022). Other intercropping systems, like push-pull technology and various soil-preserving methods, have been proven to enhance soil function and fertility by increasing soil OC levels and enhancing the availability of N and P (Ndayisaba et al., 2020; Drinkwater et al., 2021; Ndayisaba et al., 2022). Our findings support the general prediction that crop diversification can have a profound impact on many soil properties (Ndayisaba et al., 2020; Chen et al., 2021; Ndayisaba et al., 2022).
Eyheraguibel et al., 2008, reported that maize seeds germinate between four to ten days approximately. Hence, this germination period boosts maize plant growth and production (Wimalasekera, 2015). One of the best strategies to promote seed germination is to soak or imbibe the seeds in water for hours before planting (Ashraf & Foolad, 2005; Dezfuli et al., 2008; Ahmed et al., 2018). In our study we observed that the germination rate and time of SC Duma 43 maize seeds planted in soil conditioned by MLI systems germinated faster than those planted in soil conditioned by MMC and S soils.
Generally, there is a common consensus that intercropping systems are essential for maintaining optimum ecosystem functioning, thereby strengthening agricultural practices in environmental dynamics (Picasso et al., 2008; Harrison and Bardgett, 2010; Ndayisaba et al., 2022). However, disentangling the cropping system significantly affects biomass accumulation, and plant quality remains superficial. We expected that maize plants’ above-ground and below-ground biomasses were higher in soil conditioned by MLI systems compared to MMC and S measured in this current study. Different cropping systems positively impact soil fertility, increasing plant biomass accumulation and crop production (Wardle et al., 2003; Picasso et al., 2008). This study examined four plant parameters, including plant height, diameter, number of leaves, and leaf chlorophyll concentration, as growth parameters altered by soil conditioned by different cropping systems. These growth parameters in soil conditioned by MLI systems were higher compared to soil conditioned by MMC and S. These phenomena were partly linked with improved soil fertility of soil conditioned by different cropping systems allowing plants to grow faster and healthier compared to MMC and S conditioned soil. These findings concurred with recent studies where cropping systems, such as push-pull technology, mixed grassland, and forage, improved plant growth (Loreau et al., 2001; Sanderson et al., 2004; Harrison and Bardgett, 2010; Mutyambai et al., 2019). Therefore, farmers are encouraged to practice different intercropping systems to improve soil fertility, plant quality, and subsequent crop production. Interestingly, the enhanced growth of maize plants in soil conditioned by MLI systems is linked to a substantial rise in their expression of direct and indirect resistance by reducing larval feeding on maize plants.
Unravelling key mechanisms driving interactions between farming practices and anti-herbivory has remained challenging, as most studies focused on the effects of soil properties on plants’ physical attributes (Harrison and Bardgett, 2010; Wang et al., 2021; Davidson-Lowe et al., 2021). Here, in addition to the impact of cropping systems on the physical properties of plants, we investigated how MLI systems play a role in deterring herbivory. Generally, plant growth and insect resistance are often negatively correlated (Stamp, 2003) with growth matching plant quality and metabolism, thus defense and growth trade-offs. Given that plants can protect themselves against herbivory through induce and constitutive defense mechanisms (Chuang et al., 2014; Acevedo et al., 2019), plants are unpalatable for herbivore feeding (Karban, 2011). Larval feeding assays in this study demonstrated significant differences in the consumed area by FAW neonates in soil conditioned by MLI systems compared to MMC and S systems. It showed that FAW consumed less leaf disc tissue from soil conditioned by MLI systems while consuming more in the MMC system and S soil plant. Feeding by FAW adversely affects subsequent herbivory (Mutua et al., 2022), thus the differences in larval feeding observed here could be attributed to the levels of defense in soil conditioned by MLI systems. However, defense genes and secondary metabolites in different cropping systems can be constitutively or induced by herbivory, and such mechanisms can provide a clear explanation of the observed phenomenon (Makgoba et al., 2021).
Infestation of FAW neonates on maize plants for 15 days revealed that their larval growth and development, instars, and survival differed significantly across the different soil conditions by MLI systems. In soil conditioned by MLI systems, the FAW larvae attained reduced growth indices in FAW larvae weight (FLL), FAW larvae length (FLL), FAW larvae instar; (FLN), and FAW larvae number (FLN) compared to MMC and S systems. Although the underlying mechanisms of these observed behavioural responses were beyond the scope of this study, previous studies have shown changes in defense metabolite profiles and reduced larval feeding in maize plants grown in soil conditioned by maize-Desmodium intercropping (Mutyambai et al., 2019). Similar mechanisms could be the behind the observed responses and are currently under investigation in a separate study. Reduction in survival and growth indices are indicators of poor insect performance and the negative effects of plant defense on insect herbivores (Karban, 2011; Gordy et al., 2015; Kaplan et al., 2018). Our findings demonstrated that the MLI systems negatively affected herbivory by affecting behavior and population dynamics throughout the colonization, establishment, and population development phases of infestation. It has been shown that changing cropping systems and diversifying agricultural practices can decrease the prevalence and harm caused by insect pests (Midega et al., 2018; De Groote et al., 2020; Guera et al., 2021; Mutyambai et al., 2022). This study has demonstrated that there is a reduction in FAW feeding in maize plants grown in soil conditioned by different MLI systems compared to soil conditioned by MMC and S, thereby reducing the damage and increasing maize plant biomass. We postulate that changes in the soil physico-chemical properties could have affected the morphological and chemistry of the maize plant resulting to the observed responses. Indeed, maize has been shown to exhibit changes in metabolism in favour of known defense metabolites like phenolics and benzoxazinoids when grown in soil conditioned by different cropping systems (Mutyambai et al., 2019). These findings, establish the resilience of MLI systems in its functionality against the invasive FAW pest. Therefore, it paves the way for integrating intercropping systems in IPM strategies to manage FAW infestation. As such, farmers need to avoid planting the same crops in successive years and adopt the use of intercropping systems and crop rotation, as shown in this study to reduce insect-pest build-up and improve soil health. Plant-soil feedback can govern both positive and negative feedback since they are intimately linked to agricultural systems. Utilizing the potential for plant resistance in the soil left over from previous farming practices might be crucial for long-term integrated insect pest control and production enhancement (Kaplan et al., 2018; Davidson-Lowe et al., 2021).
5 Conclusions
Our findings showed that maize-edible legume intercropping systems remarkably improved soil health by altering physico-chemical characteristics. Besides, we found significant differences in the impacts of various MLI systems on plant biomass. Maize-edible legume soil legacies enhanced maize’s direct resistance to FAW damage. Soils from MLI systems significantly reduced FAW larval feeding and development on maize plants, therefore serving as controlling mechanisms. Though beyond the scope of the current study, underpinning mechanisms behind the observed responses in herbivore pest mainly plant changes in structure and chemistry should be the focus of the follow up study. These findings encourage the identification of optimal maize-legume combinations with overall positive interaction effects. Adopting such sustainable FAW control options through a farming system approach and taking into account potential trade-offs requires an understanding of current smallholder agronomic management strategies and livelihood factors, as well as how these factors are likely to interact for optimal performance. There is need for future studies to examine the combined effects of intercropping, soil resources, secondary metabolites and genes, and soil microbes on fall armyworm feeding behaviour. Lastly, we need to encourage and train small-scale farmers to adopt these intercropping practices.
Data availability statement
The raw data supporting the conclusions of this article will be made available by the authors, without undue reservation.
Ethics statement
The manuscript presents research on animals that do not require ethical approval for their study.
Author contributions
AJ: Data curation, Formal Analysis, Investigation, Methodology, Writing – original draft, Writing – review & editing. AY: Formal Analysis, Methodology, Writing – review & editing. FK: Data curation, Investigation, Methodology, Writing – review & editing. SS: Conceptualization, Investigation, Supervision, Writing – review & editing. DM: Conceptualization, Data curation, Formal Analysis, Investigation, Methodology, Writing – original draft, Writing – review & editing.
Funding
The author(s) declare financial support was received for the research, authorship, and/or publication of this article. The authors gratefully acknowledge the financial support for this research by the following organizations and agencies: Integrated pest management strategy to counter the threat of invasive FAW to food security in eastern Africa (FAW-IPM) (Grant No. DCI-FOOD/2017/) financed by European Union, IKEA Foundation under grant RG-2204-02144. The first Author (AJ) was sponsored by the German Academic Exchange Service (DAAD)-In-Region Postgraduate Scholarship through the African Regional Postgraduate Programme in Insect Science (ARPPIS). We are grateful to the Swiss Agency for Development and Cooperation (SDC); Swedish International Development Cooperation Agency (Sida); Federal Democratic Republic of Ethiopia; the Government of the Republic of Kenya for core support to icipe.
Acknowledgments
We are grateful to the Arthropod Pathology Unit (APU) of the icipe and the local smallholder farmers in Embu, Tharaka Nithi, Kitui and Meru Counties for allowing us to use their farms to collect our soil samples and field surveys. Many thanks go to Johnstone Mutiso Mutua, Catherine Awuoche, Faith Ndunge, and Kentos Gutu Ouma for insect rearing and laboratory assistance, and Amos Mwangangi, Moses Ouma, Collins Otieno and Basilio Njiru for their assistance in greenhouse operations, Abdelmutalab Gesmalla for assistance in statistical analysis.
Conflict of interest
The authors declare that the research was conducted in the absence of any commercial or financial relationships that could be construed as a potential conflict of interest.
Publisher’s note
All claims expressed in this article are solely those of the authors and do not necessarily represent those of their affiliated organizations, or those of the publisher, the editors and the reviewers. Any product that may be evaluated in this article, or claim that may be made by its manufacturer, is not guaranteed or endorsed by the publisher.
References
Abro Z., Kimathi E., De Groote H., Tefera T., Sevgan S., Niassy S., et al. (2021). Socioeconomic and health impacts of fall armyworm in Ethiopia. PloS One 16 (11), e0257736. doi: 10.1371/journal.pone.0257736
Acevedo F. E., Smith P., Peiffer M., Helms A., Tooker J., Felton G. W. (2019). Phytohormones in fall armyworm saliva modulate defense responses in plants. J. Chem. Ecol. 45, 598–609. doi: 10.1007/s10886-019-01079-z
Agegnehu G., Nelson P. N., Bird M. I. (2016). Crop yield, plant nutrient uptake and soil physico-chemical properties under organic soil amendments and nitrogen fertilization on Nitisols. Soil Tillage Res. 160, 1–13. doi: 10.1016/j.still.2016.02.003
Ahmed S., Raza M. A., Zhou T., Hussain S., Khalid M. H. B., Feng L., et al. (2018). Responses of soybean dry matter production, phosphorus accumulation, and seed yield to sowing time under relay intercropping with maize. Agronomy 8 (12), 282. doi: 10.3390/agronomy8120282
Ashraf M., Foolad M. R. (2005). Pre-sowing seed treatment-A shotgun approach to improve germination, plant growth, and crop yield under saline and non-saline conditions. Adv. Agron. 88, 223–271. doi: 10.1016/S0065-2113(05)88006-X
Beretta A. N., Silbermann A. V., Paladino L., Torres D., Kassahun D., Musselli R., et al. (2014). Soil texture analyses using a hydrometer: modification of the Bouyoucos method. Ciencia e investigación agraria: Rev. latinoamericana Cienc. la agricultura 41 (2), 263–271. doi: 10.4067/S0718-16202014000200013
Chen J., Manevski K., Lærke P. E., Jørgensen U. (2022). Biomass yield, yield stability and soil carbon and nitrogen content under cropping systems destined for biorefineries. Soil Tillage Res. 221, 105397. doi: 10.1016/j.still.2022.105397
Chen S., Cade-Menun B. J., Bainard L. D., Luce M. S., Hu Y., Chen Q. (2021). The influence of long-term N and P fertilization on soil P forms and cycling in a wheat/fallow cropping system. Geoderma 404, 115274. doi: 10.1016/j.geoderma.2021.115274
Chiriboga M. X., Tamiru A., Sobhy I. S., Bruce T. J., Midega C. A., Khan Z. (2021). Evaluation of African maize cultivars for resistance to fall armyworm Spodoptera frugiperda (JE Smith) (Lepidoptera: Noctuidae) larvae. Plants 10 (2), 392. doi: 10.3390/plants10020392
Chuang W. P., Herde M., Ray S., Castano-Duque L., Howe G. A., Luthe D. S. (2014). Caterpillar attack triggers accumulation of the toxic maize protein RIP 2. New Phytol. 201 (3), 928–939. doi: 10.1111/nph.12581
Davidson-Lowe E., Ray S., Murrell E., Kaye J., Ali J. G. (2021). Cover crop soil legacies alter phytochemistry and resistance to fall armyworm (Lepidoptera: Noctuidae) in maize. Environ. Entomology 50 (4), 958–967. doi: 10.1093/ee/nvab047
De Groote H., Kimenju S. C., Munyua B., Palmas S., Kassie M., Bruce A. (2020). Spread and impact of fall armyworm (Spodoptera frugiperda J.E. Smith) in maize production areas of Kenya. Agriculture Ecosyst. Environ. 292, 106804. doi: 10.1016/j.agee.2019.106804
De Lange E. S., Laplanche D., Guo H., Xu W., Vlimant M., Erb M., et al. (2020). Spodoptera frugiperda caterpillars suppress herbivore-induced volatile emissions in maize. J. Chem. Ecol. 46, 344–360. doi: 10.1007/s10886-020-01153-x
Delgado A., Gómez J. A. (2016). “The soil physical, chemical and biological properties,” in Principles of agronomy for sustainable agriculture. Eds. Villalobos F., Fereres E. (Cham, Switzerland: Springer). doi: 10.1007/978-3-319-46116-8_2
Dezfuli P. M., Sharif-Zadeh F., Jan mohammadi M. (2008). Influence of priming techniques on seed germination behavior of maize inbred lines (Zea mays L.). ARPN J. Agric. Biol. Sci. 3 (3), 22–25.
Drinkwater L. E., Midega C. A., Awuor R., Nyagol D., Khan Z. R. (2021). Perennial legume intercrops provide multiple belowground ecosystem services in smallholder farming systems. Agriculture Ecosyst. Environ. 320, 107566. doi: 10.1016/j.agee.2021.107566
Ewel J. J., Mazzarino M. J., Berish C. W. (1991). Tropical soil fertility changes under monocultures and successional communities of different structure. Ecol. Appl. 1 (3), 289–302. doi: 10.2307/1941758
Eyheraguibel B., Silvestre J., Morard P. (2008). Effects of humic substances derived from organic waste enhancement on the growth and mineral nutrition of maize. Bioresource Technol. 99 (10), 4206–4212. doi: 10.1016/j.biortech.2007.08.082
FAO (2018) Integrated management of the fall armyworm on maize: A guide for farmer field schools in africa. Available at: https://www.fao.org/documents/card/en/c/I8741EN/.
Gordy J. W., Leonard B. R., Blouin D., Davis J. A., Stout M. J. (2015). Comparative effectiveness of potential elicitors of plant resistance against Spodoptera frugiperda (J. E. Smith) (Lepidoptera: Noctuidae) in four crop plants. PloS One 10 (9), 1–14. doi: 10.1371/journal.pone.0136689
Guera O. G. M., Castrejón-Ayala F., Robledo N., Jiménez-Pérez A., Sánchez-Rivera G., Salazar-Marcial L., et al. (2021). Effectiveness of push–pull systems to fall armyworm (Spodoptera frugiperda) management in maize crops in Morelos, Mexico. Insects 12 (4), 298. doi: 10.3390/insects12040298
Hailu G., Niassy S., Zeyaur K. R., Ochatum N., Subramanian S. (2018). Maize–legume intercropping and push–pull for management of fall armyworm, stemborers, and striga in Uganda. Agron. J. 110 (6), 2513–2522. doi: 10.2134/agronj2018.02.0110
Harrison K. A., Bardgett R. D. (2010). Influence of plant species and soil conditions on plant-soil feedback in mixed grassland communities. J. Ecol. 98 (2), 384–395. doi: 10.1111/j.1365-2745.2009.01614.x
Jalli M., Huusela E., Jalli H., Kauppi K., Niemi M., Himanen S., et al. (2021). Effects of crop rotation on spring wheat yield and pest occurrence in different tillage systems: a multi-year experiment in Finnish growing conditions. Front. Sustain. Food Syst. 5. doi: 10.3389/fsufs.2021.647335
Kansiime M. K., Mugambi I., Rwomushana I., Nunda W., Lamontagne-Godwin J., Rware H., et al. (2019). Farmer perception of fall armyworm (Spodoptera frugiderda J.E. Smith) and farm-level management practices in Zambia. Pest Manage. Sci. 75 (10), 2840–2850. doi: 10.1002/ps.5504
Kaplan I., Pineda A., Bezemer M. (2018). Application and theory of plant–soil feedbacks on aboveground herbivores. In: Ohgushi T., Wurst S., Johnson S. N., eds. Aboveground-belowground community ecology. Cham, Switzerland: Springer International, 319–343. doi: 10.1007/978-3-319-91614-9_14
Karban R. (2011). The ecology and evolution of induced resistance against herbivores. Funct. Ecol. 25 (2), 339–347. doi: 10.1111/j.1365-2435.2010.01789.x
Kassie M., Wossen T., De Groote H., Tefera T., Sevgan S., Balew S. (2020). Economic impacts of fall armyworm and its management strategies: evidence from southern Ethiopia. Eur. Rev. Agric. Economics 47 (4), 1473–1501. doi: 10.1093/erae/jbz048
Khan Z. R., Midega C. A., Pittchar J., Bruce T. J., Pickett J. A. (2012). ‘Push–pull’revisited: the process of successful deployment of a chemical ecology based pest management tool. Biodiversity Insect pests: key Issues Sustain. Manage., 259–275. doi: 10.1002/9781118231838.ch16
Koffi D., Agboka K., Adenka D. K., Osae M., Tounou A. K., Anani Adjevi M. K., et al. (2020). Maize infestation of fall armyworm (Lepidoptera: Noctuidae) within agro-ecological zones of togo and ghana in west africa 3 yr after its invasion. Environ. Entomol. 49 (3), 645–650. doi: 10.1093/ee/nvaa048
Kumela T., Simiyu J., Sisay B., Likhayo P., Mendesil E., Gohole L., et al. (2019). Farmers' knowledge, perceptions, and management practices of the new invasive pest, fall armyworm (Spodoptera frugiperda) in Ethiopia and Kenya. Int. J. Pest Manag. 65 (1), 1–9. doi: 10.1080/09670874.2017.1423129
Li J., Nie M., Powell J. R., Bissett A., Pendall E. (2020). Soil physico-chemical properties are critical for predicting carbon storage and nutrient availability across Australia. Environ. Res. Lett. 15 (9), 094088. doi: 10.1088/1748-9326/ab9f7e
Librán-Embid F., Olagoke A., Martin E. A. (2023). Combining milpa and push-pull technology for sustainable food production in smallholder agriculture. A review. Agron. Sustain. Dev. 43 (4), 45. doi: 10.1007/s13593-023-00896-7
Loreau M., Naeem S., Inchausti P., Bengtsson J., Grime J. P., Hector A., et al. (2001). Ecology: Biodiversity and ecosystem functioning: Current knowledge and future challenges. Science 294 (5543), 804–808. doi: 10.1126/science.1064088
Makgoba M. C., Tshikhudo P. P., Nnzeru L. R., Makhado R. A. (2021). Impact of fall armyworm (Spodoptera frugiperda) (J.E. Smith) on small-scale maize farmers and its control strategies in the Limpopo province, South Africa. Jàmbá:. J. Disaster Risk Stud. 13 (1), 1016. doi: 10.4102/jamba.v13i1.1016
Midega C. A. O., Pittchar J. O., Pickett J. A., Hailu G. W., Khan Z. R. (2018). A climate-adapted push-pull system effectively controls fall armyworm, Spodoptera frugiperda (J E Smith), in maize in East Africa. Crop Prot. 105, 10–15. doi: 10.1016/j.cropro.2017.11.003
Montezano D. G., Sosa-Gómez D. R., Specht A., Roque-Specht V. F., Sousa-Silva J. C., Paula-Moraes S. D., et al. (2018). Host plants of Spodoptera frugiperda (Lepidoptera: Noctuidae) in the Americas. Afr. entomology 26 (2), 286–300. doi: 10.4001/003.026.0286
Mutua J. M., Mutyambai D. M., Asudi G. O., Khamis F., Niassy S., Jalloh A. A., et al. (2022). Competitive plant-mediated and intraguild predation interactions of the invasive Spodoptera frugiperda and resident stemborers Busseola fusca and Chilo partellus in maize cropping systems in Kenya. Insects 13 (9), 790. doi: 10.3390/insects13090790
Mutyambai D. M., Bass E., Luttermoser T., Poveda K., Midega C. A. O., Khan Z. R., et al. (2019). More than “push” and “pull”? plant-soil feedbacks of maize companion cropping increase chemical plant defenses against herbivores. Front. Ecol. Evol. 7 (217). doi: 10.3389/fevo.2019.00217
Mutyambai D. M., Niassy S., Calatayud P.-A., Subramanian S. (2022). Agronomic factors influencing fall armyworm (Spodoptera frugiperda) infestation and damage and its co-occurrence with stemborers in maize cropping systems in Kenya. Insects 13 (3), 266. doi: 10.3390/insects13030266
Ndayisaba P. C., Kuyah S., Midega C. A. O., Mwangi P. N., Khan Z. R. (2020). Intercropping desmodium and maize improves nitrogen and phosphorus availability and performance of maize in Kenya. Field Crops Res. 263, 108067. doi: 10.1016/j.fcr.2021.108067
Ndayisaba P. C., Kuyah S., Midega C. A. O., Mwangi P. N., Khan Z. R. (2022). Push-pull technology improves carbon stocks in rainfed smallholder agriculture in Western Kenya. Carbon Manage. 13 (1), 127–141. doi: 10.1080/17583004.2022.2035823
Okalebo J. R., Gathua K. W., Woomer P. L. (2002). Laboratory methods of soil and plant analysis: a working manual second edition. Sacred Africa Nairobi 21, 25–26.
Onyango F. O., Ochieng’-Odero J. P. R. (1994). Continuous rearing of the maize stem borer Busseola fusca on an artificial diet. Entomologia Experimentalis Applicata 73 (2), 139–144. doi: 10.1111/j.1570-7458.1994.tb01848.x
Overton K., Maino J. L., Day R., Umina P. A., Bett B., Carnovale D., et al. (2021). Global crop impacts, yield losses and action thresholds for fall armyworm (Spodoptera frugiperda): A review. Crop Prot. 145, 105641. doi: 10.1016/j.cropro.2021.105641
Pervaiz Z. H., Iqbal J., Zhang Q., Chen D., Wei H., Saleem M. (2020). Continuous cropping alters multiple biotic and abiotic indicators of soil health. Soil Syst. 4 (4), 59. doi: 10.3390/soilsystems4040059
Peter E., Tamiru A., Sevgan S., Dubois T., Kelemu S., Kruger K., et al. (2023). Companion crops alter olfactory responses of the fall armyworm (Spodoptera frugiperda) and its larval endoparasitoid (Cotesia icipe). Chem. Biol. Technol. Agriculture. 10, 61. doi: 10.1186/s40538-023-00415-6
Picasso V. D., Brummer E. C., Liebman M., Dixon P. M., Wilsey B. J. (2008). Crop species diversity affects productivity and weed suppression in perennial polycultures under two management strategies. Crop Sci. 48 (1), 331–342. doi: 10.2135/cropsci2007.04.0225
R Core Team (2018) R: A Language and Environment for Statistical Computing. Available at: https://www.R-project.org/ (Accessed 4 January 2023).
Sanderson M. A., Skinner R. H., Barker D. J., Edwards G. R., Tracy B. F., Wedin D. A. (2004). Grazing land ecosystems. Crop Sci. 44 (4), 1132–1144. doi: 10.2135/cropsci2004.1132
Schneider C. A., Rasband W. S., Eliceiri K. W. (2012). NIH image to imageJ: 25 years of image analysis. Nat. Methods 9 (7), 671–675. doi: 10.1038/nmeth.2089
Sharma S. B. (2022). Trend setting impacts of organic matter on soil physico-chemical properties in traditional vis-a-vis chemical-based amendment practices. PloS Sustainability Transformation 1 (3), e0000007. doi: 10.1371/journal.pstr.0000007
Sokame B. M., Subramanian S., Kilalo D. C., Juma G., Calatayud P. A. (2020). Larval dispersal of the invasive fall armyworm, Spodoptera frugiperda, the exotic stemborer Chilo partellus, and indigenous maize stemborers in Africa. Entomologia Experimentalis Applicata 168 (4), 322–331. doi: 10.1111/eea.12899
Sparks D. L., Page A. L., Helmke P. A., Loeppert R. H. (1996). Methods of soil analysis, part 3, chemical methods. (Madison, WI, USA: Soil Science Society of America Inc).
Stamp N. (2003). Out of the quagmire of plant defense hypotheses. Q. Rev. Biol. 78 (1), 23–55. doi: 10.1086/367580
Stokstad E. (2017). New crop pest takes Africa at lightning speed. Science 356 (6337), 473–474. doi: 10.1126/science.356.6337.473
Tambo J. A., Kansiime M. K., Mugambi I., Rwomushana I., Kenis M., Day R. K., et al. (2020). Understanding smallholders' responses to fall armyworm (Spodoptera frugiperda) invasion: evidence from five African countries. Sci. Total Environ. 740, 140015. doi: 10.1016/j.scitotenv.2020.140015
Van der Putten W. H., Bradford M. A., Pernilla Brinkman E., van de Voorde T. F., Veen G. F. (2016). Where, when and how plant–soil feedback matters in a changing world. Funct. Ecol. 30 (7), 1109–1121. doi: 10.1111/1365-2435.12657
Van Dijk M., Morley T., Rau M. L., Saghai Y. (2021). A meta-analysis of projected global food demand and population at risk of hunger for the period 2010–2050. Nat. Food 2 (7), 494–501. doi: 10.1038/s43016-021-00322-9
Wahome C. N., Maingi J. M., Ombori O., Njeru E. M., Muthini M., Kimiti J. M. (2023). Diversity and abundance of bacterial and fungal communities in rhizospheric soil from smallholder banana producing agroecosystems in Kenya. Front. Horticulture 2, 1061456. doi: 10.3389/fhort.2023.1061456
Wang G., Bei S., Li J., Bao X., Zhang J., Schultz P. A., et al. (2021). Soil microbial legacy drives crop diversity advantage: Linking ecological plant–soil feedback with agricultural intercropping. J. Appl. Ecol. 58 (3), 496–506. doi: 10.1111/1365-2664.13802
Wardle D. A., Yeates G. W., Williamson W., Bonner K. I. (2003). The response of a three trophic level soil food web to the identity and diversity of plant species and functional groups. Oikos 102 (1), 45–56. doi: 10.1034/j.1600-0706.2003.12481.x
Wimalasekera R. (2015). Role of seed quality in improving crop yields. In Crop production and global environmental issues. Cham, Switzerland: Springer 2015, 153–168. doi: 10.1007/978-3-319-23162-4_6
Keywords: crop mixtures, fall armyworm, soil health, seed germination, plant growth
Citation: Jalloh AA, Yusuf AA, Khamis F, Subramanian S and Mutyambai DM (2023) Soil legacies in maize-edible legume intercropping alter maize growth and reduce Spodoptera frugiperda larval feeding. Front. Agron. 5:1300545. doi: 10.3389/fagro.2023.1300545
Received: 23 September 2023; Accepted: 20 November 2023;
Published: 12 December 2023.
Edited by:
Alpha Kamara, International Institute of Tropical Agriculture (IITA), NigeriaReviewed by:
Christopher Ngosong, University of Buea, CameroonSanjay Singh Rathore, Indian Agricultural Research Institute (ICAR), India
Copyright © 2023 Jalloh, Yusuf, Khamis, Subramanian and Mutyambai. This is an open-access article distributed under the terms of the Creative Commons Attribution License (CC BY). The use, distribution or reproduction in other forums is permitted, provided the original author(s) and the copyright owner(s) are credited and that the original publication in this journal is cited, in accordance with accepted academic practice. No use, distribution or reproduction is permitted which does not comply with these terms.
*Correspondence: Daniel Munyao Mutyambai, ZG11dHlhbWJhaUBpY2lwZS5vcmc=