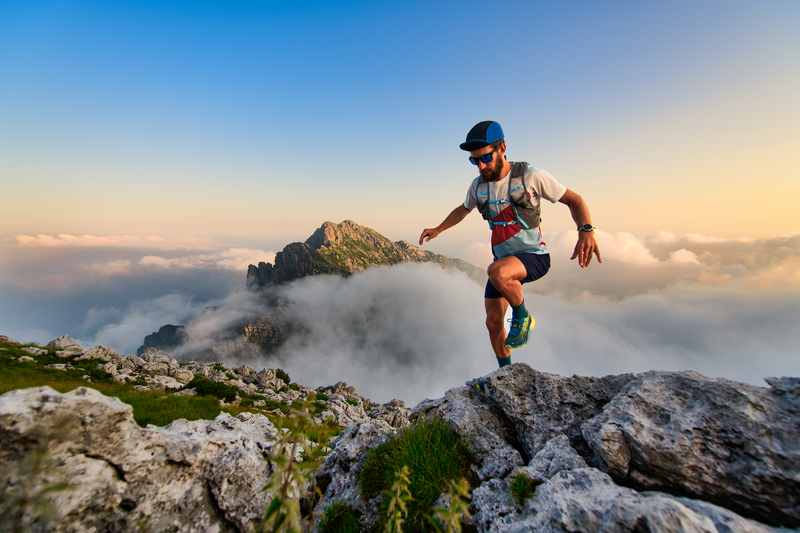
95% of researchers rate our articles as excellent or good
Learn more about the work of our research integrity team to safeguard the quality of each article we publish.
Find out more
ORIGINAL RESEARCH article
Front. Agron. , 05 September 2023
Sec. Disease Management
Volume 5 - 2023 | https://doi.org/10.3389/fagro.2023.1229717
Maize or corn (Zea mays L.) is the third most important cereal crop in the economy of agriculture. Banded leaf and sheath blight (BLSB) caused by Rhizoctonia solani (= R. solani f. sp. sasakii) is one of the highly devastating soil-borne diseases of maize in South and Southeast Asia. Although the use of resistant varieties is preferred as an eco-friendly and cheapest approach to disease management, unfortunately, no true genetic sources of BLSB resistance are available in maize. Hence, chemically induced resistance in the host plant is considered an alternative strategy against many crop diseases. The present study investigated the basis of BLSB resistance in maize hybrid variety Vivek QPM-9 by seed priming with two plant defense inducers, viz., salicylic acid (SA) and jasmonic acid (JA). Higher concentrations (100 ppm) of SA and JA were significantly more effective against R. solani than the lower concentrations (75 and 50 ppm) in vitro. The study found that the application of SA and JA as exogenous pretreatment resulted in improved seed germination, increased seedling weight, and enhanced overall plant growth. During the Kharif season (June–October) in both 2020 and 2021, under in vivo conditions in a net house, the application of SA at 100 and 75 ppm and JA at 100 ppm resulted in a significant decrease in the percent disease index (PDI) of 46.79%, 47.05%, and 48.85%, respectively. Both plant defense inducers elevated the activity of the enzymes superoxide dismutase (SOD), catalase (CAT), and phenylalanine ammonia-lyase (PAL) in maize at higher concentrations of 100 ppm. Seed priming with a high concentration of the inducers was more effective in suppressing the disease and increasing grain yield under the controlled condition of the net house. The study shows the scope of using need-based fungicides with a reduced amount in the management of fungal diseases of maize by adopting a plant defense inducer-mediated host resistance approach.
Maize or corn (Zea mays L.) is considered an internationally important commodity driving world agriculture. In India, the annual production of maize is 27.82 million metric tons with average national productivity of 3.02 tons per hectare (FAOSTAT, 2018). It is grown during Kharif (June–October), Rabi (October to March), and spring (March–June) seasons; however, major production is achieved during the rainy season (Yadav et al., 2015). The production of maize is influenced by numerous biotic stresses, with one of the primary challenges being its susceptibility to various diseases. This susceptibility to diseases poses a significant obstacle to achieving high grain yields in maize cultivation. Banded leaf and sheath blight (BLSB) is a major disease and most prevalent in tropical regions of the world, in south and southeast Asian countries, and specifically in India during the Kharif season. The disease causes losses of up to a hundred percent under favorable conditions (Anonymous, 2017; Devi and Thakur, 2018). BLSB is caused by the soil-borne pathogen Rhizoctonia solani [R. solani f. sp. sasakii (Kuhn)], which is generally identified by the characters of mycelia and sclerotia. The pathogen is recognized as one of the most prevalent, destructive, and adaptable fungi found across many regions worldwide. It has the ability to infect plants from a wide range of taxonomic groups, affecting 32 families and 188 genera of crops such as cereals, millets, pulses, and oilseeds, as well as grasses and weeds (Devi and Thakur, 2018). Cereals are infected by the pathogen belonging to the AG 1-1A anastomosis group of R. solani (Wang et al., 2013). In maize, the disease starts from the first leaf sheath and extends up to the ears to cause maximum damage. High relative humidity and rainfall significantly favor the development and rapid spread of this disease.
The disease is manageable to some extent using single or combinations of different management strategies like cultural, chemical, and biological. Thus, for identifying climate-resilient components, immediate attention is required and, due to the present changing climate, integrated disease management (IDM) is considered the best module for the management of BLSB. Resistance of host plants plays a significant role in the IDM approach. Therefore, identifying resistance genes to this aggressive disease and combining them with high grain yield potentiality is a priority of the Indian maize breeding program. However, BLSB-resistant cultivars are scarce. Limited variation for resistance to BLSB is a major bottleneck for an effective resistance breeding program. Identifying genotypes with genetic resistance, i.e., the capacity to restrict blighted lesion expansion, is quite difficult among maize germplasm as experienced in rice against the same fungus. However, national programs in India, China, Indonesia, and the Philippines are making efforts toward screening genotypes for BLSB resistance. In the All India Coordinated Research Project (AICRP) on maize, both inbred and hybrids have been evaluated for their reaction against BLSB, and some lines with a moderate level of resistance were identified (Hooda et al., 2015). Under such circumstances, chemically induced resistance in the host plant is considered a new potential strategy against many fungal diseases.
Salicylic acid (SA) is a plant growth regulator which plays a significant role in signaling pathways induced by various biotic and abiotic stresses (Saleem et al., 2021). It achieves this by regulating various aspects, including the antioxidant defense system, transpiration rates, stomatal movement, and photosynthetic rate (Nazar et al., 2015). Exogenously applied SA has been observed to induce both local and systemic acquired resistance in diverse plant species against a range of pathogens, such as Fusarium oxysporum (Jendoubi et al., 2015), Penicillium digitatum/Alternaria alternata (Allahverdi Beyk et al., 2021), Magnaporthe grisea (Yang et al., 2019a), Colletotrichum gloeosporioides (He et al., 2017), Xanthomonas spp. (Le Thanh et al., 2017), viruses (Ong and Cruz, 2016; Zhao et al., 2019), and phytoplasma (Ahmed et al., 2022). For instance, in China, foliar application of different concentrations of SA ranging from 0.1 to 5.0 mM resulted in direct and systemic effects on chemical defense responses with an increase in content of total phenol, DIMBOA (2,4-dihydroxy-7-methoxy-2H,1,4-benzoxazin-3(4H)-one), and a significant increase in activity of superoxide dismutase (SOD), polyphenol oxidase, peroxidase, and catalase (Feng et al., 2022). Recently, in Thailand, an SA formulation called Zacha was tested and exogenous application of the same could significantly inhibit the growth of soil-borne fungi Fusarium solani and significantly reduced the disease severity of Fusarium root rot in cassava at 500 ppm (Saengchan et al., 2022). A remarkable resistance against Curvularia lunata was induced in maize when treated with different chemical compounds, including SA. SA, in particular, showed significant effectiveness, resulting in a disease reduction of 49.6% (Chengbo and Huazhi, 2005). In addition to priming the plant for disease resistance, SA has also been reported to ameliorate abiotic stress. In Iran, SA as seed treatment at a concentration of 0.5 mM resulted in the alleviation of lead toxicity by modulating glyoxalase I activity and thereafter reducing methylglyoxal accumulation (Zanganeh et al., 2020). Another study in Saudi Arabia demonstrated that at a concentration of 0.05 mM, SA improved the salinity stress tolerance in maize by regulating phytochromes as well as various organic and inorganic osmolytes (Elhakem, 2020). The role of jasmonic acid (JA) in plants has received extensive research attention due to its crucial roles in mediating plant responses and defenses against biotic and abiotic stresses when applied exogenously in small amounts (Wang et al., 2021). Reports also suggest that plants can defend themselves against necrotrophic pathogens and insects through JA signaling (Liu et al., 2017). Feng et al. (2012) demonstrated that the application of JA had a systemic effect on the quantities of defense chemicals in both the aboveground and belowground parts when either of them was continuously treated with JA. Additionally, it was observed that the phenolic and DIMBOA contents significantly increased after 2–4 weeks of application. Girault et al. (2008) observed that, compared with the control group (18.65%), the exogenous application of the lipid transfer protein–jasmonic acid complex resulted in a remarkable resistance of 80.3% against Botrytis cinerea. Induction of systemic resistance with increased catalase, polyphenol oxidase, and peroxidase activity in cucumber and excellent efficacy of JA against Pythium aphanidermatum was documented by Sabbagh et al. (2018). Like SA, JA has also been shown to alleviate lead stress by improving the physiological processes and enhancing the ability of maize plants to overcome oxidative damage by inducing the activity of antioxidant enzymes such as SOD, peroxidase, catalase, and glutathione and also phenol, ascorbic acid, and total soluble sugar (Sofy et al., 2020). Similarly, salinity tolerance was found to be improved by the exogenous application of JA (Ali et al., 2020). Thus, disease resistance in host plants induced by the exogenous application of SA and JA can be a boon in cost-effective disease management without any ecological consequences. Furthermore, this type of resistance is governed by the accumulation of PR proteins that are known to be associated with systemic acquired resistance (SAR) in plants (Ali et al., 2018; Jain and Khurana, 2018). Despite these observations, the exact mechanism exerted by these inducers through different modes and timings of application remains vague. Further extensive studies are required to elucidate this mechanism. Nevertheless, it has been noted that these inducers interact in a complex manner with the antioxidative metabolism, leading to the modulation of cellular redox homeostasis and ultimately resulting in the activation of defense genes (Hernández et al., 2017; Jabnoun-Khiareddine et al., 2015).
Based on the aforementioned background, the objective of the present investigation was to gather information regarding the management of BLSB disease through the application of plant defense inducers, specifically SA and JA. Additionally, the study aimed to assess the positive effects of these inducers on maize germination and biomass, as well as their in vitro antifungal effect against R. solani f. sp. sasakii. Furthermore, the study aimed to gain insights into the underlying mechanisms of chemically induced disease resistance in maize.
Leaf and sheath samples showing typical symptoms of BLSB were collected from the maize field of ICAR-IARI, Pusa campus, New Delhi, during the Kharif season. The pathogen was isolated in potato dextrose agar (PDA) by following the standard technique of Tutte (1969). Small pieces of leaf sample of around 5 mm with symptoms along with a green healthy portion were cut and washed with 1% sodium hypochlorite followed by subsequent washing with sterilized distilled water three times. Then, with the help of forceps, the cut pieces were dried using sterilized tissue paper and placed on solid media. The pure cultures were maintained in PDA slants kept at 4°C ± 1°C for further use in the study. The morpho-molecular identification was conducted, and the isolate was submitted to the Indian Type Culture Collection at ICAR-IARI, New Delhi. The assigned ITCC accession number for the submission is 9244, and the NCBI GenBank accession number is OR251925. The molecular identification was conducted using ITS1 and ITS4 primers. The mass culture of R. solani was prepared in barley grains by the method described by Ahuja and Payak (1978). Barley grains were soaked in distilled water overnight. Soaked seeds were half-filled in an Erlenmeyer flask, plugged with a non-adsorbent cotton plug, and autoclaved two times at 121°C, 15 pounds per square inch (psi) for two consecutive days. The fungus was allowed to grow for 10 days by incubating at 26°C ± 2°C with intermittent shaking for uniform coverage.
Two defense inducers salicylic acid (SA, SISCO Research Laboratories, Mumbai, India, product code: 11453) and jasmonic acid (JA, Biosphere Corporation, Dnipro, Ukraine) at three concentrations, namely, 50, 75, and 100 ppm, were used for seed priming. A susceptible variety of maize called Vivek QPM-9 was procured from ICAR-Vivekananda Parvatiya Krishi Anusandhan Sansthan, Almora, Uttarakhand, India. Before sowing, seeds were soaked overnight in the suspensions of plant defense inducers (SA and JA) at concentrations of 50, 75, and 100 ppm. The treated seeds were dried with the help of tissue paper and then sown. Seeds soaked only in water served as a check (untreated). There were 10 seeds sown in each pot and placed in a containment facility. The pot (10-inch-diameter) was filled with soil collected from the field mixed with vermiculite in a 1:1 ratio. Four pots were maintained for each treatment, and each pot was considered as one replication. The effect of the treatments on seed germination was recorded from pots as well as from directly sown seeds under net house conditions described later in Section 2.5.
Three seedlings 15 days old were carefully uprooted from each pot, washed, and air dried for 1 h. Treatment-wise pre-labeled seedlings were weighed using an electronic balance (Wensar, Chennai, India) for fresh weight (FW). The seedlings were dried in direct sunlight for 5 days, and the sun-dried seedlings were further dried overnight in an oven at 50°C. Then, the dry weight of the seedlings was recorded.
The experiments were conducted in vitro by following the poisoned food technique (Nene and Thapliyal, 1979) to check the effect of the plant defense inducers SA and JA on R. solani. Stock solutions of SA and JA were prepared by dissolving desired quantity in a measured volume of sterilized distilled water. A measured volume from the stock solution was added to the sterilized PDA medium before solidification. Three concentrations (25, 50, and 100 ppm) of both SA and JA with three replications were maintained in potato dextrose agar (PDA) and potato dextrose broth (PDB) culture media using a micropipette. Mycelial discs of actively grown R. solani were placed onto the media under aseptic conditions and then incubated at 27°C ± 1°C in a biological oxygen demand (BOD) incubator. Percent inhibition of the mycelial growth over control was calculated in each treatment by applying the formula described by Vincent (1947).
where, I = percent inhibition (%), C = colony diameter in control (mm), and T = colony diameter in treatment (mm).
The experiments to evaluate the efficacy of SA and JA on BLSB disease of maize and grain yield were conducted during the Kharif season (June–October), 2020, and 2021 in a net house located at the Division of Plant Pathology, ICAR-IARI, New Delhi (latitude: 28.6410° N; longitude: 77.1716° E). Maize seeds of a susceptible variety Vivek QPM-9 were primed with the solutions of SA and JA as described earlier, sown, and raised by following standard agronomic practices in the soil of a net house to protect the crop from squirrels and other large pests and to facilitate precise monitoring and assessment of the impact of inducers on disease progression and maize grain yield. The soil of the net house was sandy loam type, and prior to land preparation, farm yard manure was incorporated at the rate of 10 t per hectare. Irrigation was given as per the recommendation, through the flood irrigation method. Sprinkler irrigation was also used to maintain humidity. The experiment was laid out in a randomized block design (RBD). The plot size for one treatment was 4.5 m2 with three rows (length: 3 m, R × R: 75 cm), and in each treatment, 13 to 15 plants per row were maintained. The treatments (including control, i.e., without priming) were replicated thrice.
Inoculation was done on 35-day-old maize plants (stage 5 of maize growth stages, Payak and Sharma, 1983) with 15-day-old mass culture of R. solani by placing two to three infected barley grains between the stem and sheaths on each plant (Ahuja and Payak, 1978). The inoculated plants were monitored regularly for the appearance of symptoms and disease incidence. The parameters like lesion length (cm), disease score (scales 1–5), and percent disease index (PDI) were recorded 30 DAI (days after inoculation) in every treatment, as suggested by Ahuja and Payak (1983). As per the rating scale, PDI was calculated using the formula given by McKinney (1923).
For grain yield (kg ha−1), the total weight (kg) of the fully matured harvested cobs (90 DAI) was recorded treatment-wise. Initial shelling of the grains was done, and the moisture content of the grains was recorded by using a moisture meter (AgraTronix MT-PRO, USA). Grain yield was calculated by applying the following three formulas (Chikkappa, 2005).
Healthy and young leaves of 35-day-old maize plants were collected on 05/07/2020 and 2021 from the trial conducted in the net house on the day of inoculation (0 day) followed by a daily collection for up to 5 days. The upper leaves were collected during early morning hours using a scissor, immediately transferred into plastic pre-labeled bags put into ice boxes, and stored at −80°C for further use. Three samples were collected from three different maize plants for each treatment for 5 consecutive days. Three enzymes, namely, SOD, CAT, and phenylalanine ammonia-lyase (PAL), were assayed from the maize plants that emerged from the seeds primed with SA and JA at three concentrations (50, 75, and 100 ppm).
The activity of SOD was assayed followed by the method of Dhindsa and Baker (1981). One gram of maize leaf tissue was cut using a sterilized scissor, weighed using an electronic balance (Wensar, Chennai, India), and ground in 5 ml grinding media (0.1 M phosphate buffer pH 7.5, containing 0.5 mM EDTA). The homogenate was filtered using Whatman filter paper, and the filtrate was centrifuged for 20 min at 15,000 rpm. The supernatant was used as an enzyme. All the steps in the preparation of the enzyme extract were carried out at 0°C–4°C. The activity of SOD was assayed by a 3-ml reaction mixture containing 50 mM phosphate buffer (pH 7.8), 13.33 mM L-methionine, 75 µM NBT, 2 µM riboflavin, 0.1 mM EDTA, and 100 µl enzyme extract. The reaction was started by adding 0.1 ml of 2 µM riboflavin and placing the tubes under two 15-W fluorescent lamps for 15 min. A complete reaction mixture without enzyme which gave the maximum color served as control. A non-irradiated complete reaction mixture served as a blank. The absorbance was recorded using an OD 560-nm UV-visible spectrophotometer (Evolution 220 UV-Visible Spectrophotometer, Thermo Scientific, USA). Calculations was carried out using the following formula.
CAT assay was performed as per the method described by Aebi (1984) with slight modifications. One gram of leaf tissue was ground in liquid nitrogen, to which 1 ml of 0.1 M phosphate buffer pH 7.0 was added and well homogenized. After homogenization, the mixture was passed through Whatman filter paper and the resulting filtrate was subjected to centrifugation at 15,000 rpm for 20 min at 4°C and the supernatant was used as an enzyme. Protein estimation described by Bradford (1976) was performed by adding 2 ml of Bradford solution, 990 µl of distilled water, and 10 µl of tissue extract followed by incubation for 10 min at room temperature in the dark. Finally, OD was taken at 595 nm and the protein concentration calculated by using the BSA standard curve (Lowry et al., 1951).
The activity of CAT was assayed by preparing a 3-ml reaction mix [50 mM potassium phosphate buffer 1.5 ml (pH 7.0), 0.5 ml of 75 mM hydrogen peroxide (H2O2), 100 µl enzyme extract, water for making up the volume to 3 ml], then OD was taken at 240 nm with a 1-min interval for 3 min. The CAT activity, expressed as µM min−1g−1 FW, was calculated by using the extinction coefficient of € = 39.4 mM−1 cm−1 to determine the reduction in hydrogen peroxide concentration.
Initial reading – final reading = quantity of hydrogen peroxide reduced per min per g fresh weight.
The activity of PAL was assayed by following the standard method (Zuker, 1965). One gram of plant sample was homogenized in a pre-cooled mortar and pestle using liquid nitrogen (N2), to which 20% of polyvinyl pyrrolidone (PVP) and 2 ml of borate buffer (pH 8.5) was immediately added and subjected to centrifugation at 14,000 rpm for 20 min at 4°C, and the supernatant was used as an enzyme. The activity of PAL was assayed by a 3-ml reaction mixture [1 ml of 4 mM L-phenylalanine, 1.5 ml of 0.05 mM borate buffer (pH 8.8), 0.1 ml of enzyme extract; its volume was made up of distilled water]. The reaction was started by incubating test tubes without shaking in a 38°C hot water bath for 1 h. The reaction was stopped by adding 0.1 ml of 5 M HCL. Absorbance was recorded at 290 nm to determine the amount of trans-cinnamic acid. A reaction mixture without phenylalanine was used as a control. The activity of PAL was calculated by using a standard curve of trans-cinnamic acid, and protein was estimated by the Lowry method (Lowry et al., 1951). PAL activity was expressed in terms of µmol h−1 g−1 fresh weight.
The experimental data were analyzed using the statistical procedure of SAS 9.4 (SAS Institute, 2003, Cary, NC). To ensure the normality of distribution, the data falling in the range of 0%–30% and 70%–100% were transformed by the square root transformation, and data that were not within the specified range were subjected to the angular transformation method prior to analysis (Gomez and Gomez, 1984). After the transformation, the data were found to be symmetrically distributed around the mean, as most of the values were close to the mean with fewer values in the tails. Complete randomized design (CRD) was used in vitro with three replications and pot experiment with four replications. Randomized block design (RBD) was followed for in vivo (net house) experiment with three replications. The experiments were repeated twice in vitro and in vivo. The significant difference between the treatment’s mean was determined by the Tukey HSD test (p ≤ 0.05) after analysis of variance (ANOVA).
The effect of SA and JA at 50, 75, and 100 ppm on seed germination and weight of the maize seedlings was determined in potted soil under controlled conditions and by direct seed sowing in the soil of a net house. Under controlled conditions, no effect of the plant defense inducers was observed on the germination of maize seeds. Under the net house, maximum seed germination was observed with SA at 100 ppm and JA at 100 ppm and the lowest seed germination at 50 ppm of both chemicals (Table 1; Supplementary Table 1 and Figure 1). Overall germination was 100% under containment facility, whereas under the net house, SA at 50, 75, and 100 ppm rendered germination percentages of 86.67%, 88.33%, and 96.67%, respectively, and JA at 50, 75, and 100 ppm rendered germination percentages of 95.00%, 95.00%, and 96.67%, respectively. Except for SA at 50 and 75 ppm, the germination percentage of maize treated with other treatments was significantly higher as compared with the control (90.84%) (p ≤ 0.05). However, there was no significant difference between the treatments of JA.
Table 1 Effect of salicylic acid (SA) and jasmonic acid (JA) on germination of maize seeds (Vivek QPM-9) and weight of maize seedlings.
Figure 1 Germination of maize seeds (Vivek QPM-9) primed with salicylic acid (SA) and jasmonic acid (JA) at 50-, 75-, and 100-ppm concentrations. (A) Maize seeds directly sown in the ground of the net house and (B) maize seeds sown in the potted soil and placed in a containment facility room.
The fresh weight of the maize seedlings was highest with SA at 50 ppm (5.05 g) with an increase of 22.06% followed by SA at 75 ppm (4.87 g) and at 100 ppm (4.57 g) with an increase of 17.42% and 10.39%, respectively, as compared with the control (4.14 g). In the case of JA, an increase of 9.11%, 8.09%, and 8.37% was observed in maize seedlings treated with 50, 75, and 100 ppm of JA, respectively, as compared with the control (4.14 g). Similarly, the highest dry weight was recorded in SA at 50 ppm for treated seedlings, an increase of 27.03%, followed by SA at 75 ppm and SA at 100 ppm with an increase of 24.32% and 11.66%, respectively, as compared with the control. Comparatively, the increase in weight due to JA treatment was less, i.e., 9.62%, 4.97%, and 5.53% at 50, 75, and 100 ppm of JA, respectively. The SA treatment resulted in higher biomass of the seedlings as compared with the JA treatment. However, the increase in biomass of the maize seedlings recorded in SA and JA was not significant as compared with the check; also, the difference between the treatments was insignificant (Table 1; Supplementary Table 2).
Plant defense inducers were evaluated in vitro to determine their fungicidal efficacy, against the test pathogen. In the PDA medium, maximum significant inhibition of radial growth of R. solani was recorded with SA at 100 ppm (30.37%) followed by SA at 50 ppm (20.93%) and SA at 25 ppm (14.81%) as compared with control (p ≤ 0.05) (Table 2; Figure 2). In none of the concentrations of JA, any inhibition in radial growth was recorded (Table 2; Supplementary Table 3; Figure 2). Moreover, the effect of different concentrations of SA was statistically significant from each other (p ≤ 0.05).
Table 2 Effect of salicylic acid (SA) and jasmonic acid (JA) on growth and weight of Rhizoctonia solani on PDA and PDB.
Figure 2 Effect of salicylic acid (SA) and jasmonic acid (JA) on radial growth of Rhizoctonia solani in vitro (on PDA). (A) 25 ppm SA, (B) 50 ppm SA, (C) 100 ppm SA, (D) 25 ppm JA, (E) 50 ppm JA, (F) 100 ppm JA, and (G) check (untreated).
In the PDB medium, the maximum reduction of 51.12% in mycelial mass of R. solani was recorded when treated with the highest concentration of SA at 100 ppm followed by JA at 100 ppm (30.07%), which was significantly less as compared with the control (p ≤ 0.05). The lowest reduction in mycelial mass of the pathogen was observed in JA at 25 ppm (10.89%) followed by JA at 50 ppm (15.50%) and SA at 25 ppm (17.30%); nevertheless, the reduction was significant as compared with the control (Table 2; Supplementary Table 4; Figure 3).
Figure 3 Effect of salicylic acid (SA) and jasmonic acid (JA) on the mycelial mass of Rhizoctonia solani in vitro (on PDB). (A) 25 ppm SA, (B) 50 ppm SA, (C) 100 ppm SA, (D) 25 ppm JA, (E) 50 ppm JA, (F) 100 ppm JA, and (G) check (untreated).
Maximum lesion length among the treatments except for check was observed in the plants treated with JA at 50 ppm (37.19 cm) followed by maize plants treated with SA at 50 ppm (36.93 cm), JA at 75 ppm (36.75 cm), and SA at 75 ppm (35.94 cm), whereas a statistically significant reduction in lesion length was observed in maize plant treated with SA at 100 ppm (33.82 cm) and JA at 100 ppm (33.87 cm) as compared with control (p ≤ 0.05) (Table 3).
Table 3 Efficacy of plant defense inducers on banded leaf and sheath blight (BLSB) disease and grain yield of maize (variety Vivek QPM-9) under net house condition.
The percent disease index (PDI) was significantly lower in treated maize plants as compared with the control (69.09%) (Table 3). The least PDI of 46.79% was recorded in maize plants treated with SA at 100 ppm followed by SA at 75 ppm with a PDI of 47.05%. JA treatment also led to a significant reduction of PDI as compared with the control (p ≤ 0.05), with the least PDI of 48.85% at 100 ppm of JA. However, SA at 75 ppm, SA at 100 ppm, and JA at 100 ppm were insignificant to each other when compared.
The highest grain yield was observed in the maize plants treated (seed primed) with SA at 100 ppm, an increase of 52.31%, followed by its 75- and 50-ppm concentrations, with an increase of 44.24% and 24.00%, respectively, as compared with control (Table 3). In the case of JA at 50 and 75 ppm, the increase in yield was insignificant as compared with the control (p ≤ 0.05); nonetheless, JA at 100 ppm could offer a maximum yield of 38.17 × 100 kg ha−1 which is an increase by 11.53%. The yield subsequently decreased with the lowering of the concentration of JA. The yield was significantly high in both seasons when treated with three different concentrations of SA. In the case of JA, in the first season, the yield increase was insignificant compared with the check; nevertheless, in the second season, JA at 100 ppm produced a statistically significant yield compared with the check. When compared between the treatments of SA and JA, SA-treated plants rendered a significantly higher yield than the JA-treated plants.
The activity of SOD was assayed in the maize plants germinated from SA- and JA-treated seeds after inoculation with R. solani and is presented in Figure 4. The SOD activity was recorded highest on the fourth day in a maize plant treated with JA at 100 ppm (5.81 U mg−1 protein). In the case of SA, the highest activity was observed with SA at 100 ppm on the fifth DAI (5.55 U mg−1 protein). Overall, the activity of SOD was found highest in JA 100 ppm with an increase of 52.72% followed by SA 100 ppm, JA 75 ppm, and SA 75 ppm with an average increase of 39.86%, 16.27%, and 13.80%, respectively, as compared with the check. The least activity was observed in the check (3.66 U mg−1 protein) and the lowest concentration of both SA and JA (50 ppm). The activity of SOD in the treatments showed fluctuation with time; however, in the case of a check, the activity increased up to 4 DAI and decreased thereafter.
Figure 4 Superoxide dismutase (SOD) activity in maize (Vivek QPM-9) primed with SA and JA at 50-, 75-, and 100-ppm concentrations on different days after inoculation (DAI) of Rhizoctonia solani. The error bars correspond to the standard error of the mean. Different letters in each column indicate a significant difference (Tukey HSD, p ≤ 0.05).
Maize plants treated with SA at 100 ppm showed the highest CAT activity on the fourth day (2.32 U min−1 mg−1 protein) followed by JA at 100 ppm (2.27 U min−1 mg−1 protein) and SA at 75 ppm (2.10 U min−1 mg−1 protein) on the fifth day (Figure 5). Overall, the highest average percentage increase of 31.00% was observed in maize plant treated with SA at 100 ppm, followed by JA at 100 ppm and SA at 75 ppm with an average increase of 18.35% and 15.96%, respectively. The lowest activity of 1.63, 1.77, 1.55, and 1.69 U min−1 mg−1 protein was observed in the check, SA at 50 ppm, JA at 50 ppm, and JA at 75 ppm, respectively. The activity of CAT gradually increased up to the third day and sharply decreased thereafter in most of the concentrations of SA and JA. In check, peak activity was recorded on the third day.
Figure 5 Catalase (CAT) activity in maize (Vivek QPM-9) primed with SA and JA at 50-, 75-, and 100-ppm concentrations on different days after inoculation (DAI) of Rhizoctonia solani. The error bars correspond to the standard error of the mean. Different letters in each column indicate a significant difference (Tukey HSD, p ≤ 0.05).
The activity of PAL was highest in maize plants treated with SA at 100 ppm treatment on the fourth day (6.28 U mg−1 protein) followed by JA at 100 ppm, SA at 75 ppm, and SA at 50 ppm (Figure 6). Overall, SA at 100 ppm, JA at 100 ppm, and SA at 75 ppm led to an increase in activity of 46.31%, 26.74%, and 25.64%, respectively, as compared with the check. The lowest activity was observed in the check. The activity of PAL in maize plants treated with JA 50 ppm and 100 ppm decreased gradually with time. The change in activity did not follow a definite pattern with different concentrations of defense inducers.
Figure 6 Phenylalanine ammonia-lyase (PAL) activity in maize (Vivek QPM-9) primed with SA and JA at 50-, 75-, and 100-ppm concentrations on different days after inoculation (DAI) of Rhizoctonia solani. The error bars correspond to the standard error of the mean. Different letters in each column indicate a significant difference (Tukey HSD, p ≤ 0.05).
The present study generated information on the management of BLSB disease utilizing plant defense inducers and elucidated the biochemical mechanisms of disease resistance in maize. Chemically induced disease resistance could serve as a viable alternative to true resistance in maize varieties lacking resistance to BLSB. Thus, two plant defense inducers, viz., salicylic acid (SA) and jasmonic acid (JA), were tested in vitro against R. solani and in vivo for restriction of the BLSB disease of maize.
SA being an endogenous growth regulator of phenolic nature can positively influence a range of diverse processes in plants, including percent seed germination, vigor index, mean germination time, plant height, number and area of green leaves (check leaf chlorophyll degradation), stem diameter, and dry weight of the whole plant of cereal crops (Anwar et al., 2013; Alamri et al., 2018; Zhu et al., 2021). Similarly, JA, known as lipid-derived phytohormones, can also regulate overall plant growth under both abiotic and biotic stresses (Siddiqi and Husen, 2019).
Our study demonstrates a positive impact of SA and JA on germination with the increase in the concentration of SA and JA. In the case of the net house condition, a significantly better germination percentage was observed at higher concentrations of JA and SA (100 ppm). The improved germination observed with SA treatment can be attributed to the augmented uptake of oxygen and enhanced α-amylase activity, consequently leading to efficient mobilization of nutrients from cotyledons to the embryonic axis, resulting in increased levels of soluble sugars, amino acids, and proteins (Fardus et al., 2018). A similar positive impact on germination was reported by Ilyas et al. (2017), where they observed the application of SA and JA acid on wheat under drought-ameliorated stress with the increase of germination by 21% and 27%, respectively, which under stress was reduced by 26%. Moreover, an increase in the shoot length by 20% and 23%, respectively, was recorded. However, in contradiction to the present study, a plant (Lavandula angustifolia Mill.) grown in SA-free media could produce healthier and more shoots than the plant grown in SA-treated media. However, plants grown with amended elicitor media were found to have higher polyphenolic and chlorophyll content than the controls, proving a positive impact on the secretion of secondary metabolites (Miclea et al., 2020). In harmony with our findings, Fardus et al. (2018) recorded improved germination and various growth parameters with application of SA exogenously.
In our study, we observed an increase in biomass in maize seedlings treated with SA and JA. However, this increase was found to be statistically insignificant. The increase in biomass can be primarily attributed to changes in hormone functioning or an enhancement in photosynthesis and stomatal conductance, and these factors collectively contribute to the overall growth (Ibrahim et al., 2017). Another possible reason for the increase in biomass could be the induction of meristematic cell division (Vanacker et al., 2001). Furthermore, the increase in biomass could also be attributed to the improvement in the photosynthetic activity of the plant treated with SA or JA at an optimum concentration, which in turn enhances the plant’s ability to produce more glucose, resulting in the accumulation of biomass. (Bukhat et al., 2020; Noor et al., 2022; Li et al., 2023). They improve plant growth through their regulation of various processes. It influences stomatal movements, ensuring optimal gas exchange and water balance. It also plays a role in regulating Rubisco biosynthesis, a key enzyme involved in photosynthesis. Additionally, they enhance the uptake of essential nutrients such as phosphorus and nitrogen, promoting healthy plant growth. Furthermore, it facilitates the efficient transport of carbohydrates within plants, ensuring an adequate supply of energy for growth and development (Fu et al., 2017: Wasternack and Hause, 2013). In concurrence with the current findings, the promotion of dry leaf mass and dry root mass of fennel (Foeniculum vulgare Miller) at a concentration of 0.50 and 0.25 mM of SA, respectively, was documented (Gorni et al., 2017). Similarly, when studying Brassica juncea plants treated with 0.01 mM SA, significant increases in dry root mass (26%) and shoot growth (51%) were reported (Parashar et al., 2014). Further supporting our findings, Bukhat et al. (2020) also observed an increase in root and shoot biomass of radish when treated with 2 mM of SA by 2.89- and 2.80-fold, respectively. In a recent study, it was observed that the exogenous application of JA (120 mM) during salt stress significantly increased the fresh weight, dry weight, root length, and photosynthetic pigments of two soybean varieties, namely, parachinar-local soybean and swat-84 (Noor et al., 2022). In line with our findings, Awan et al. (2021) also demonstrated an increase in fresh and dry weight, shoot, and root length in pearl millet upon treatment with 100 µM of JA. Under in vivo conditions, the PDI of BLSB was significantly reduced to 46.79%, 47.05%, and 48.85% with SA at 100 ppm, SA at 75 ppm, and JA at 100 ppm, respectively (Table 3), compared with the control (69.09%). Moreover, the yield was also significantly increased with different concentrations of inducers, highest at SA@100 ppm with 52.13 × 100 kg ha−1 of grain yield. The decrease in PDI is mainly because of the induction of several defense biochemical pathways. Also, SA and JA cause early defense-related gene induction and late defense-related gene induction in plants, respectively, protecting them from pathogens and insects (Yang et al., 2019b). Application of SA to plants renders the expression of pathogenesis-related genes and other defensive compounds at local and systemic levels conferring immunity (Sivakumar et al., 2014; Wani et al., 2017). Possibly, the exogenous application of SA leads to the accumulation of endogenous SA, which plays a crucial role in conferring disease resistance through the activation of the SA signaling pathway, primarily associated with systemic acquired resistance (SAR). Exogenous treatment with SA to rice has been found to reduce disease severity of blast disease caused Magnaporthe oryzae by inducing the expression of defense-related genes such as PAL, PR1a, PR5, and HSP90 (Yang et al., 2019a). Intriguingly, an SA-based abiotic elicitor called S-Ricemate at 100 ppm or more was demonstrated to reduce the disease severity of leaf blight caused by Xanthomonas oryzae pv. oryzae by 17.71%–12.50% as compared with control (Thepbandit et al., 2021). Earlier results indicated that the jasmonate response reduces damage caused by a wide range of pathogens from different lifestyles in tomatoes (Thaler et al., 2004). In agreement with our findings, Meyer et al. (2006) also reported that SA (2.5 mM) sprayed 20 days before disease inoculation significantly reduced Rhizoctonia foliar blight infection and observed that resistance activators performed better on Rhizoctonia foliar blight control when applied together with fungicides. In concurrence with our results, Ziasmin Islam et al. (2017) observed the dose-dependent effect of SA as a foliar spray on morphological and yield-contributing characters of wheat such as tiller number, effective tiller numbers/plant, spike length, number of spikelets/spike, number of effective spikelets/spike, and number of grains/spike that accredited higher grain yield. Similarly, seed priming with SA could significantly influence the wheat grain filling rate and duration, grain yield, grain protein percent, and total weight of dry seed (Khamseh et al., 2013). In addition, Elgamaal and Maswada (2013) recorded significant enhancement in total chlorophyll, flag leaf area, relative water content, and grain yield with the increase in the concentration of SA in three yellow maize hybrids. Furthermore, exogenous application of SA not only alleviated the inhibitory effect of drought stress but also had a stimulatory effect on physiological traits and grain yield of tested maize hybrids, and hence they opined that foliar application of SA at 0.5 and 1.0 mM is beneficial for boosting the productivity of maize plants apart from higher tolerance to drought stress. It has been also observed that the pretreatment or exogenous application of JA exhibited multi-stress resilience under changing environments, biotic stress conditions (Siddiqi and Husen, 2019) as well as abiotic stress like herbicide (imidacloprid) toxicity in plants (Sharma et al., 2018). Based on previous reports and current findings, it is possible to postulate that an increase in yield may be attributed to the enhancement of various physiological characteristics of plants like source–sink relationship, which directly or indirectly contribute to yield improvement.
In the study, defense inducers exhibited fungicidal properties, and comparatively, SA performed better than JA. SA at 100 ppm could reduce radial growth and weight by 30.37% and 51.99%, respectively. Hence, utilization of SA may contribute dual benefits, viz., acting against the growth of the pathogen and induction of host defense mechanism to contain disease spread. Contrary to our findings, Meyer et al. (2006) reported no mycelial growth inhibition of R. solani causing Rhizoctonia foliar blight of soybean when the concentration of SA was below 10 mg L−1. In another in vitro test, SA did not exert a direct antifungal effect on the mycelial growth of Fusarium oxysporum f. sp. lycopersici. These results indicated the role of SA in the plant to activate the SA pathway leading to induction of antimicrobial peptides such as PR-1 which directly affect the invading fungal pathogen (Mandal et al., 2009). However, in a study conducted by da Rocha Neto et al. (2015), 90% inhibition of fungal germination after 30 min of exposure to the SA was observed with apparent damage to the plasma membrane of conidia, resulting in protein leakage. The antifungal activity of SA was also demonstrated by its ability to induce certain compounds such as phenol-2,4-bis-(1,1-dimethylethyl) against Aspergillus sp. Penicillium cinnamomi in avocado (Rangel-Sánchez et al., 2014). The antimicrobial activity of SA as observed by the reduction of radial growth and weight of mycelia in our study could be attributed to its ability to directly penetrate and initiate multiple interactions with the plasma membrane, which results in the disruption of the lipid bilayer and potential damage to the proteins involved in maintaining cellular permeability. These effects ultimately contribute to an increase in the concentration of reactive oxygen species. In addition, cellular respiration can also be disrupted by redirecting the electron flow away from the cytochrome pathway toward an alternative pathway associated with cyanide (Kapulnik et al., 1992).
The activity of the host defense induction indicative enzymes SOD, CAT, and PAL was assayed in maize (Vivek QPM-9) inoculated with R. solani after treatment (seed treatment) with the plant defense inducers, viz., SA and JA. SOD, which is a widespread metalloenzyme (Jackson et al., 1978), forms the primary defense against reactive oxygen species (ROS) and serves as a highly efficient component of the antioxidant defense system in plant cells, countering ROS toxicity. It plays an important role in the development as well as stress resistance in plants (Cannon et al., 1987). SOD genes catalyze the superoxide radicals () into H2O2, to protect plant cells against oxidative stress (Dos Santos and Rey, 2006; Hossain et al., 2009). On the other hand, CAT catalyzes hydrogen peroxide conversion into water and oxygen without requiring cellular energy consumption, and it is a major H2O2 scavenging enzyme in all aerobic organisms (Ghasemi et al., 2013; Sharma and Ahmad, 2014). PAL is an inducible enzyme that responds to various biotic and abiotic stresses, including pathogenic attacks (MacDonald and D’Cunha, 2007). Its induction is triggered by these stresses, highlighting its role in plant defense mechanisms. PAL is involved in the phenylpropanoid pathway and facilitates the synthesis of cinnamic acid from phenylalanine (Vogt, 2010). Additionally, PAL is actively involved in the biosynthesis of salicylic acid (SA), which serves as a vital signal in plant systemic resistance (Chaman et al., 2003). We observed the activities of all three enzymes, viz., SOD, CAT, and PAL, highest at a concentration of 100 ppm of SA and JA as compared with the control. SA as an endogenous signal molecule playing an important role in the development of systemic resistance in plants is well established (Dempsey et al., 1999). Moreover, SA confers an anti-stress role by stimulating the expression of genes involved in the biosynthesis of antioxidants, thereby enhancing the antioxidant potential of plants further contributing to the plants’ ability to mitigate the harmful effects of stress (Najafabadi and Ehsanzadeh, 2017; Wang et al., 2019). JA regulates plant responses to abiotic and biotic stresses as well as plant growth (Wang et al., 2021). It has an important role in response to the wounding of plants and SAR. The Dgl gene is responsible for maintaining levels of JA during usual conditions in Zea mays as well as the preliminary release of JA immediately after being fed by insects (Galis et al., 2009).
In support of our findings, recently, Khalvandi et al. (2021) demonstrated a significant increase in the activity of enzymatic antioxidants in wheat under drought conditions, viz., PAL, SOD, and CAT by 18.18%, 42.16%, 56.6%, respectively, when SA (0.5 mM) was exogenously applied. Another study also documented an increase in SOD and CAT activity after 7 days with exogenous JA treatment by 48.6% and 36.7% (Awan et al., 2021). In concurrence with our results, Jini and Joseph (2017) observed an increase in SOD and CAT activity in rice varieties ASD16 and BR26 treated with SA (1 mM L−1). Similarly, SOD enzyme activity was found significantly raised in the leaves, skin, and flesh of grapes (Vitis vinifera L.) by the SA treatment at the 1 mM dose (Nazari et al., 2022). As observed in the present study, enhancement of PAL activity was reported in response to R. solani inoculation in cowpea pretreated with SA (Chandra et al., 2007). Similarly, SA spraying on Ya Li pear plants increased PAL activity greatly and contributed to the protection of pear fruits against postharvest diseases (Cao et al., 2006). In addition, in support of our findings, the PAL activity was found to increase to a greater extent in SA-treated tomato plants compared with non-SA-treated plants, which probably enhanced resistance in tomatoes to Fusarium oxysporum f. sp. lycopersici (Mandal et al., 2009). The results of the present study indicate that SA and JA serve as effective inducers of disease resistance in host plants, functioning as potential triggers for systemic resistance. This induction is supported by the activation of essential defense-related enzymatic antioxidants. As a result, the application of SA and JA significantly reduced the incidence of BLSB disease in maize caused by the soil-borne pathogen R. solani. In regard to R. solani, it is presumed that ROS favors the pathogen by rendering cell death as, being a necrotroph, it requires dead cells for its nutrition. Antioxidant enzymes scavenge free radical and hence keeps the ROS concentration under check, therefore possibly indirectly preventing the ingression of the pathogen.
The findings of this study have demonstrated the reduction of disease severity/PDI of BLSB due to the induction of resistance in maize upon exogenous pre-treatment with SA and JA, as evidenced by the activation of defense-related enzymes, namely, SOD, CAT, and PAL. In addition, a significant increase in the yield of maize was also discerned. The abiotic defense inducers SA and JA also testified to suppress the necrotrophic soil-borne phytopathogen R. solani under in vitro conditions. Furthermore, seed priming with the inducers contributed to promotion of germination and biomass of the maize indicating a positive role in the growth and development of maize plants, opening the scope for quality fodder production as well. Maize being an exhaustive crop requires high inputs in crop culture and pest management. Under such circumstances, our study is able to project light on the scope of reducing large quantities of fungicides used for the management of fungal diseases of maize, adopting a plant defense inducer-mediated host resistance approach, upholding a safe environment as well.
The original contributions presented in the study are included in the article/Supplementary Material. Further inquiries can be directed to the corresponding authors.
AK supervision and provided lab facilities. AS supervised biochemical aspects and provided lab facilities. RG conceptualized and designed experiments, supervised, provided resources, and revised and finalized the manuscript. RY supervised field experiments. SH conducted experiments, generated data, and wrote the first draft of the manuscript. LD revised and edited the manuscript. All authors have read and approved the manuscript.
The senior author is thankful to the PG School, ICAR-Indian Agricultural Research Institute, New Delhi, and the Head Division of Plant Pathology, IARI, for providing working facilities during his M. Sc. research program. His sincere acknowledgment is due to the Ministry of Higher Education (MoHE), and Ministry of Borders and Tribal Affairs (MoBTA), the Government of the Islamic Republic of Afghanistan (GoIRA), for introduction to pursue an M. Sc. program abroad, and the Indian Council for Cultural Relations (ICCR), Government of India (GoI), for providing the scholarship program.
The authors declare that the research was conducted in the absence of any commercial or financial relationships that could be construed as a potential conflict of interest.
All claims expressed in this article are solely those of the authors and do not necessarily represent those of their affiliated organizations, or those of the publisher, the editors and the reviewers. Any product that may be evaluated in this article, or claim that may be made by its manufacturer, is not guaranteed or endorsed by the publisher.
The Supplementary Material for this article can be found online at: https://www.frontiersin.org/articles/10.3389/fagro.2023.1229717/full#supplementary-material
Ahmed E. A., Shoala T., Abdelkhalik A., El-Garhy H. A., Ismail I. A., Farrag A. A. (2022). Nanoinhibitory impacts of salicylic acid, glycyrrhizic acid ammonium salt, and boric acid nanoparticles against phytoplasma associated with faba bean. Molecules 27 (5), 1467. doi: 10.3390/molecules27051467
Ahuja S. C., Payak M. M. (1978). A field inoculation technique for evaluating maize germplasm to banded leaf and sheath blight. Indian Phytopath. 31, 517–520.
Ahuja S. C., Payak M. M. (1983). A rating scale for banded leaf and sheath blight of maize. Indian Phytopath. 36, 338–340.
Alamri S. A., Siddiqui M. H., Al-Khaishani M. Y., Ali M. H. (2018). Response of salicylic acid on seed germination and physio-biochemical changes of wheat under salt stress. Acta Sci. Agri 2 (5), 36–42.
Ali S., Ganai B. A., Kamili A. N., Bhat A. A., Mir Z. A., Bhat J. A., et al. (2018). Pathogenesis-related proteins and peptides as promising tools for engineering plants with multiple stress tolerance. Microbiol. Res. 212, 29–37. doi: 10.1016/j.micres.2018.04.008
Ali A. Y. A., Ibrahim M. E. H., Zhou G., Nimir N. E. A., Jiao X., Zhu G., et al. (2020). Exogenous jasmonic acid and humic acid increased salinity tolerance of sorghum. Agron. J. 112 (2), 871–884. doi: 10.1002/agj2.20072
Allahverdi Beyk P., Atghia O., Fallahi M., Mohammadi N., Mirzadi Gohari A. (2021). Salicylic acid impact on Penicillium digitatum and Alternaria alternata in vitro and post-harvest lemon diseases. Mycol. Iran. 8 (1), 25–34. doi: 10.22043/MI.2021.355575.1197
Anonymous (2017). The biology of Zea mays L. ssp mays (maize or corn). Office Gene Technol. Regulator.
Anwar S., Iqbal M., Raza S. H., Iqbal N. (2013). Efficacy of seed preconditioning with salicylic and ascorbic acid in increasing vigor of rice (Oryza sativa L.) seedling. Pak. J. Bot. 45 (1), 157–162.
Awan S. A., Khan I., Rizwan M., Zhang X., Brestic M., Khan A., et al. (2021). Exogenous abscisic acid and jasmonic acid restrain polyethylene glycol-induced drought by improving the growth and antioxidative enzyme activities in pearl millet. Physiol. Plant 172 (2), 809–819. doi: 10.1111/ppl.13247
Bradford M. M. (1976). A rapid and sensitive method for the quantitation of microgram quantities of protein utilizing the principle of protein-dye binding. Analytic Bio-chem. 72, 248–254. doi: 10.1006/abio.1976.9999
Bukhat S., Manzoor H., Athar H. U. R., Zafar Z. U., Azeem F., Rasul S. (2020). Salicylic acid induced photosynthetic adaptability of Raphanus sativus to salt stress is associated with antioxidant capacity. J. Plant Growth Regul. 39, 809–822. doi: 10.1007/s00344-019-10024-z
Cannon R. E., White J. A., Scandalios J. G. (1987). Cloning of cDNA for maize superoxide dismutase 2 (SOD2). Proc. Natl. Acad. Sci. 84 (1), 179–183. doi: 10.1073/2Fpnas.84.1.179
Cao J., Zeng K., Jiang W. (2006). Enhancement of postharvest disease resistance in Ya Li pear (Pyrus bretschneideri) fruit by salicylic acid sprays on the trees during fruit growth. Eur. J. Plant Pathol. 114 (4), 363–370. doi: 10.1007/s10658-005-5401-8
Chaman M. E., Copaja S. V., Argandona V. H. (2003). Relationships between salicylic acid content, phenylalanine ammonia-lyase (PAL) activity, and resistance of barley to aphid infestation. J. Agric. Food Chem. 51, 2227–2231. doi: 10.1021/jf020953b
Chandra A., Saxena R., Dubey A., Saxena P. (2007). Change in phenylalanine ammonia lyase activity and isozyme patterns of polyphenol oxidase and peroxidase by salicylic acid leading to enhance resistance in cowpea against Rhizoctonia solani. Acta Physiol. Plant 29 (4), 361–367. doi: 10.1007/s11738-007-0045-2
Chengbo Y., Huazhi Y. (2005). Induced resistance of maize against {\sl Curvularia lunata} with exogenous chemical compounds. Plant Prot. 31 (5), 31–35.
Chikkappa G. K. (2005). Genetic and molecular characterization of early maize (Zea mays L.) parental lines and their hybrids. M. Sc. Thesis (New Delhi, India: Division of Genetics, Indian Agricultural Research Institute), 22–23.
da Rocha Neto A. C., Maraschin M., Di Piero R. M. (2015). Antifungal activity of salicylic acid against Penicillium expansum and its possible mechanisms of action. Int. J. Food Microbiol. 215, 64–70. doi: 10.1016/j.ijfoodmicro.2015.08.018
Dempsey D. M. A., Shah J., Klessig D. F. (1999). Salicylic acid and disease resistance in plants. Crit. Rev. Plant Sci. 18 (4), 547–575. doi: 10.1080/07352689991309397
Devi B., Thakur B. R. (2018). Integrated management of banded leaf and sheath blight of maize caused by Rhizoctonia solani f. sp. sasakii. Pro. Natl. Acad. Sci. India Sec. B: Biol. Sci. 88 (2), 769–777. doi: 10.1007/s40011-016-0814-z
Dhindsa C., Baker K. F. (1983). The nature and practice of biological control of plant pathogens. Am. Phytopathol. Soc, 539.
Dos Santos C. V., Rey P. (2006). Plant thioredoxins are key actors in the oxidative stress response. Trends Plant Sci. 11 (7), 329–334. doi: 10.1016/j.tplants.2006.05.005
Elgamaal A. A., Maswada H. F. (2013). Response of three yellow maize hybrids to exogenous salicylic acid under two irrigation intervals. Asian J. Crop Sci. 5, 264–274. doi: 10.3923/ajcs.2013.264.274
Elhakem A. (2020). Salicylic acid ameliorates salinity tolerance in maize by regulation of phytohormones and osmolytes. Plant Soil Environ. 66 (10), 533–541. doi: 10.17221/441/2020-PSE
FAOSTAT (2018)Production, crops. In: Food and agricultural organization of the united nations (Accessed April 5, 2020).
Fardus J., Matin M. A., Hasanuzzaman M., Hossain M. A. (2018). Salicylic acid-induced improvement in germination and growth parameters of wheat under salinity stress. J. Anim. Plant Sci. 28 (1), 219–232. doi: 10.1111/j.1469-8137.2010.03378.x
Feng Y., Wang X., Du T., Shu Y., Tan F., Wang J. (2022). Effects of salicylic acid concentration and post-treatment time on the direct and systemic chemical defense responses in maize (Zea mays L.) following exogenous foliar application. Molecules 27 (20), 6917. doi: 10.3390/molecules27206917
Feng Y., Wang J., Luo S., Fan H., Jin Q. (2012). Costs of jasmonic acid induced defense in aboveground and belowground parts of corn (Zea mays l.). J. Chem. Ecol. 38, 984–991. doi: 10.1007/s10886-012-0155-1
Fu J., Wu H., Ma S., Xiang D., Liu R., Xiong L. (20172108). OsJAZ1 attenuates drought resistance by regulating JA and ABA signaling in rice. Front. Plant Sci. 8. doi: 10.3389/fpls.2017.02108
Galis I., Gaquerel E., Pandey S. P., Baldwin I. T. (2009). Molecular mechanisms underlying plant memory in JA mediated defence responses. Plant Cell Environ. 32 (6), 617–627. doi: 10.1111/j.1365-3040.2008.01862.x
Ghasemi F., Heidari R., Jameii R., Purakbar L. (2013). Responses of growth and anti-oxidative enzymes to various concentrations of nickel in Zea mays leaves and roots. Rom. J. Plant Biol. 58 (1), 37–49.
Girault T., François J., Rogniaux H., Pascal S., Delrot S., Coutos-Thévenot P., et al. (2008). Exogenous application of a lipid transfer protein-jasmonic acid complex induces protection of grapevine towards infection by Botrytis cinerea. Plant Physiol. Biochem. 46 (2), 140–149. doi: 10.1016/j.plaphy.2007.10.005
Gomez K. A., Gomez A. A. (1984). Statistical procedures for agricultural research. Wiley publication. Pp, 680.
Gorni P. H., Brozulato M. D. O., Lourenção R. D. S., Konrad E. C. G. (2017). Increased biomass and salicylic acid elicitor activity in fennel (Foeniculum vulgare Miller). Braz. J. Food Technol. 20, e2016172. doi: 10.1590/1981-6723.17216
He J., Ren Y., Chen C., Liu J., Liu H., Pei Y. (2017). Defense responses of salicylic acid in mango fruit against postharvest anthracnose, caused by Colletotrichum gloeosporioides and its possible mechanism. J. Food Saf. 37 (1), e12294. doi: 10.1111/jfs.12294
Hernández J. A., Diaz-Vivancos P., Barba-Espín G., Clemente-Moreno M. J. (2017). “On the role of salicylic acid in plant responses to environmental stresses” in Salicylic acid: a multifaceted hormone, ed. Nazar R., et al. (Springer Nature Singapore Pte Ltd. 2017), 17–34. doi: 10.1007/978-981-10-6068-7_2
Hooda K. S., Khokhar M. K., Parmar H., Gogoi R., Joshi D., Sharma S. S., et al. (2015). Banded leaf and sheath blight of maize: historical perspectives, current status and future directions. Proc. Natl. Acad. Sci. India. Sec. B. Biol. Sci. 87 (4), 1–12. doi: 10.1007/s40011-015-0688-5
Hossain Z., López-Climent M. F., Arbona V., Pérez-Clemente R. M., Gómez-Cadenas A. (2009). Modulation of the antioxidant system in citrus under waterlogging and subsequent drainage. J. Plant Physiol. 166 (13), 1391–1404. doi: 10.1016/j.jplph.2009.02.012
Ibrahim M. H., Omar H., Zain N. A. M. (2017). Salicylic acid enhanced photosynthesis, secondary metabolites, antioxidant, and lipoxygenase inhibitory activity (LOX) in Centella asiatica. Annu. Res. Rev. Biol. 17, 1–14. doi: 10.9734/ARRB/2017/36153
Ilyas N., Gull R., Mazhar R., Saeed M., Kanwal S., Shabir S., et al. (2017). Influence of salicylic acid and jasmonic acid on wheat under drought stress, Com. Soil Sci. Plant Anal. 48 (22), 2715–2723. doi: 10.4172/2157-7471.1000325
Jabnoun-Khiareddine H., El-Mohamedy R. S. R., Abdel-Kareem F., Abdallah R. A. B., Gueddes-Chahed M., Daami-Remadi M. (2015). Variation in chitosan and salicylic acid efficacy towards soil-borne and air-borne fungi and their suppressive effect of tomato wilt severity. J. Plant Pathol. Microbiol. 6 (325), 2. doi: 10.4172/2157-7471.1000325
Jackson C. A., Moore A. L., Halliwell B., Foyer C. H., Hall D. O. (1978). Subcellular localization and identification of superoxide dismutase in the leaves of higher plants. Eur. J. Biochem. 91, 339–344. doi: 10.1111/j.1432-1033.1978.tb12685.x
Jain D., Khurana J. P. (2018). “Role of pathogenesis-related (PR) proteins in plant defense mechanism” in Molecular aspects of plant-pathogen interaction, ed. Singh A., Singh I. K. (Springer Nature Singapore Pte Ltd. 2018) , 265–281. doi: 10.1007/978-981-10-7371-7_12
Jendoubi W., Harbaoui K., Hamada W. (2015). Salicylic acid-induced resistance against Fusarium oxysporumf. s. pradicis lycopercisi in hydroponic grown tomato plants. J. New Sci. 21 (5), 21.
Jini D., Joseph B. (2017). Physiological mechanism of salicylic acid for alleviation of salt stress in rice. Rice Sci. 24 (2), 97–108. doi: 10.1016/j.rsci.2016.07.007
Kapulnik Y., Yalpani N., Raskin I. (1992). Salicylic acid induces cyanide-resistant respiration in tobacco cell-suspension cultures. Plant Physiol. 100 (4), 1921–1926. doi: 10.1104/pp.100.4.1921
Khalvandi M., Siosemardeh A., Roohi E., Keramati S. (2021). Salicylic acid alleviated the effect of drought stress on photosynthetic characteristics and leaf protein pattern in winter wheat. Heliyon 7 (1). doi: 10.1016/j.heliyon.2021.e05908
Khamseh S. R., Sekari F., Saba J., Zangani E. (2013). Effects of priming with salicylic acid on grain growth of three wheat cultivars under rainfed conditions. Int. J. Agro. Plant Prod. 4 (8), 2061–2068.
Le Thanh T., Thumanu K., Wongkaew S., Boonkerd N., Teaumroong N., Phansak P., et al. (2017). Salicylic acid-induced accumulation of biochemical components associated with resistance against Xanthomonas oryzae pv. oryzae in rice. J. Plant Interact. 12 (1), 108–120. doi: 10.1080/17429145.2017.1291859
Li Y., Han X., Ren H., Zhao B., Zhang J., Ren B., et al. (2023). Exogenous SA or 6-BA maintains photosynthetic activity in maize leaves under high temperature stress. Crop J. 11 (2), 605–617. doi: 10.1016/j.cj.2022.08.006
Liu H., Carvalhais L. C., Schenk P. M., Dennis P. G. (2017). Effects of jasmonic acid signaling on the wheat microbiome differ between body sites. Sci. Rep. 7 (41766), 1–8. doi: 10.1038/srep41766
Lowry O. H., Rosebrough N. J., Farr A. L., Randall R. J. (1951). Protein measurement with the folin phenol reagent. J. Biol. Chem. 193 (1), 265–275. doi: 10.1016/s0021-9258(19)52451-6
MacDonald M. J., D’Cunha G. B. (2007). A modern view of phenylalanine ammonia-lyase. Biochem. Cell Biol. 85, 273–282. 10.1016/j.plaphy.2009.03.001
Mandal S., Mallick N., Mitra A. (2009). Salicylic acid-induced resistance to fusarium oxysporum f. sp. lycopersici in tomato. Plant Physiol. Biochem. 47 (7), 642–649. doi: 10.1016/j.plaphy.2009.03.001
Meyer M. C., Bueno C. J., De Souza N. L., Yorinori J. T. (2006). Effect of doses of fungicides and plant resistance activators on the control of Rhizoctonia foliar blight of soybean, and on Rhizoctonia solani AG1-IA in vitro development. Crop Prot. 25 (8), 848–854. doi: 10.1016/j.cropro.2005.11.008
Miclea I., Suhani A., Zahan M., Bunea A. (2020). Effect of jasmonic acid and salicylic acid on growth and biochemical composition of in-vitro-propagated Lavandula angustifolia Mill. Agronomy 10 (11), 1722. doi: 10.3390/agronomy10111722
Najafabadi M. Y., Ehsanzadeh P. (2017). Photosynthetic and antioxidative upregulation in drought-stressed sesame (Sesamum indicum L.) subjected to foliar-applied salicylic acid. Photosynthetica 55 (4), 611–622. doi: 10.1007/s11099-017-0673-8
Nazar R., Umar S., Khan N. A., Sareer O. (2015). Salicylic acid supplementation improves photosynthesis and growth in mustard through changes in proline accumulation and ethylene formation under drought stress. South Afr. J. Bot. 98, 84–94. doi: 10.1016/j.sajb.2015.02.005
Nazari F., Maleki M., Rasouli M. (2022). Effect of Salicylic Acid on Changes in Superoxide Dismutase Enzyme Activity, Protein, Proline, and Some Photosynthetic Pigments in Grape (Vitis vinifera L.) Bidane Ghermez and Bidane Sefid Cultivars at Two Growth Stages. Erwerbs-Obstbau 64 (Suppl 1), 37–45. doi: 10.1007/s10341-022-00683-w
Nene Y. L., Thapliyal P. N. (1979). Fungicides in plant disease control. (New Delhi, Bombay, Calcutta: Oxford and IBH Publishing Co.), 406–425.
Noor J., Ullah A., Saleem M. H., Tariq A., Ullah S., Waheed A., et al. (2022). Effect of jasmonic acid foliar spray on the morpho-physiological mechanism of salt stress tolerance in two soybean varieties (Glycine max L.). Plants 11 (5), 651. doi: 10.3390/plants11050651
Ong S., Cruz F. C. S. (2016). Effect of exogenous application of salicylic acid on the severity of tomato leaf curl disease. J. Int. Soc Southeast Asian Agric. Sci. 22 (1), 137–145.
Parashar A., Yusuf M., Fariduddin Q., Ahmad A. (2014). Salicylic acid enhances antioxidant system in Brassica juncea grown under different levels of manganese. Int. J. Biol. Macromol. 70, 551–558. doi: 10.1016/j.ijbiomac.2014.07.014
Payak M. M., Sharma R. C. (1983). Disease Rating Scales of Maize in India. “Techniques of scoring for resistance to important diseases of maize“. AICMIP. IARI New Delhi pp, 1–97.
Rangel-Sánchez G., Castro-Mercado E., García-Pineda E. (2014). Avocado roots treated with salicylic acid produce phenol-2, 4-bis (1, 1-dimethylethyl), a compound with antifungal activity. J. Plant Physiol. 171 (3-4), 189–198. doi: 10.1016/j.jplph.2013.07.004
Sabbagh E., Sabbagh S. K., Panjehkeh N., Bolok-Yazdi H. R. (2018). Jasmonic acid induced systemic resistance in infected cucumber by. Pythium aphanidermatum. J. Agric. Sci. 24 (1), 143–152. doi: 10.15832/ankutbd.446416
Saengchan C., Phansak P., Thumanu K., Siriwong S., Le Thanh T., Sangpueak R., et al. (2022). Resistance Induction by Salicylic Acid Formulation in Cassava Plant against Fusarium solani. Plant Pathol. J. 38 (3), 212. doi: 10.5423/2FPPJ.OA.02.2022.0019
Saleem M., Fariduddin Q., Janda T. (2021). Multifaceted role of salicylic acid in combating cold stress in plants: A review. J. Plant Growth Regul. 40, 464–485. doi: 10.1007/s00344-020-10152-x
Sharma I., Ahmad P. (2014). “Catalase: a versatile antioxidant in plants”, in Oxidative damage to plants, ed. Ahmad P. (Elsevier Inc., Academic Press), 131–148.
Sharma A., Kumar V., Yuan H., Kanwar M. K., Bhardwaj R., Thukral A. K., et al. (20181609). Jasmonic acid seed treatment stimulates insecticide detoxification in brassica juncea L. Front. Plant Sci. 9. doi: 10.3389/fpls.2018.01609
Siddiqi K. S., Husen A. (2019). Plant response to jasmonates: current developments and their role in changing environment. Bul. Nat. Res. Cen. 43 (153), 1–11. doi: 10.1186/s42269-019-0195-6
Sivakumar S., Khatiwada C. P., Sivasubramanian J. (2014). Studies the alterations of biochemical and mineral contents in bone tissue of mus musculus due to aluminum toxicity and the protective action of desferrioxamine and deferiprone by FTIR, ICP-OES, SEM and XRD techniques. Spectrochim. Acta A Mol.Biomol. Spectrosc. 126, 59–67. doi: 10.1016/j.saa.2014.01.136
Sofy M. R., Seleiman M. F., Alhammad B. A., Alharbi B. M., Mohamed H. I. (2020). Minimizing adverse effects of pb on maize plants by combined treatment with jasmonic, salicylic acids and proline. Agronomy 10 (5), 699. doi: 10.3390/agronomy10050699
Thaler J. S., Owen B., Higgins V. J. (2004). The role of the jasmonate response in plant susceptibility to diverse pathogens with a range of lifestyles. Plant Physiol. 135 (1), 530–538. doi: 10.1104/2Fpp.104.041566
Thepbandit W., Papathoti N. K., Daddam J. R., Thumanu K., Siriwong S., Thanh T. L., et al. (2021). Identification of salicylic acid mechanism against leaf blight disease in Oryza sativa by SR-FTIR microspectroscopic and docking studies. Pathogens 10 (6), 652. doi: 10.3390/2Fpathogens10060652
Tutte J. (1969). Plant pathological method fungi and bacteria Vol. 229 (USA: Burgess Publishing Company).
Vanacker H., Lu H., Rate D. N., Greenber J. T. (2001). A role for salicylic acid and NPR1 in regulating cell growth in Arabidopsis. Plant J. 28, 209–216. doi: 10.1046/j.1365-313x.2001.01158.x
Vincent J. M. (1947). Distortions of fungal hyphae in the presence of certain inhibitors. Nature 50, pp. doi: 10.1038/159850b0
Wang L., Liu L. M., Wang Z. G., Huang S. W. (2013). Genetic structure and aggressiveness of rhizoctonia solani AG 1-IA, the cause of sheath blight of rice in southern China. J. Phytopathol. 161 (11-12), 753–762. doi: 10.1111/jph.12127
Wang Y., Mostafa S., Zeng W., Jin B. (2021). Function and mechanism of jasmonic acid in plant responses to abiotic and biotic stresses. Int. J. Mol. Sci. 22 (16), 8568. doi: 10.3390/2Fijms22168568
Wang Y. Y., Wang Y., Li G. Z., Hao L. (2019). Salicylic acid-altering Arabidopsis plant response to cadmium exposure: underlying mechanisms affecting antioxidation andphotosynthesis-related processes. Ecotoxicol. Environ. Saf. 169, 645–653. doi: 10.1016/j.ecoenv.2018.11.062
Wani A. B., Chadar H., Wani A. H., Singh S., Upadhyay N. (2017). Salicylic acid to decrease plant stress. Environ. Chem. Lett. 15, 101–123. doi: 10.1007/s10311-016-0584-0
Wasternack C., Hause B. (2013). Jasmonates: biosynthesis, perception, signal transduction and action in plant stress response, growth and development. An update to the 2007 review. Ann. Bot. 111 (6), 1021–1058. doi: 10.1093/aob/mcm079
Yadav O. P., Hossain F., Karjagi C. G., Kumar B., Zaidi P. H., Jat S. L., et al. (2015). Genetic improvement of maize in India: Retrospect and prospects. Agric. Res. 4 (4), 325–338. doi: 10.1007/s40003-015-0180-8
Yang J., Duan G., Li C., Liu L., Han G., Zhang Y., et al. (2019a). The crosstalks between jasmonic acid and other plant hormone signaling highlight the involvement of jasmonic acid as a core component in plant response to biotic and abiotic stresses. Front. Plant Sci. 10. doi: 10.3389/fpls.2019.01349
Yang J., Wang Y., Liu L., Liu L., Wang C., Wang C., et al. (2019b). Effects of exogenous salicylic acid and pH on pathogenicity of biotrophy-associated secreted protein 1 (BAS1)-overexpressing strain. Magnaporthe oryzae. Environ. Sci. pollut. Res. 26, 13725–13737. doi: 10.1007/2Fs11356-018-2532-y
Zanganeh R., Jamei R., Rahmani F. (2020). Pre-sowing seed treatment with salicylic acid and sodium hydrosulfide confers Pb toxicity tolerance in maize (Zea mays L.). Ecotoxicol. Environ. Saf. 206, 111392. doi: 10.1016/j.ecoenv.2020.111392
Zhao L., Chen L., Gu P., Zhan X., Zhang Y., Hou C., et al. (2019). Exogenous application of melatonin improves plant resistance to virus infection. Plant Pathol. 68 (7), 1287–1295. doi: 10.1111/ppa.13057
Zhu Z. H., Sami A., Xu Q. Q., Wu L. L., Zheng W. Y., Chen Z. P., et al. (2021). Effects of seed priming treatments on the germination and development of two rapeseed (Brassica napus L.) varieties under the co-influence of low temperature and drought. PloS One 16 (9), e0257236. doi: 10.1371/journal.pone.0257236
Ziasmin Islam M. M., Ahmed K. U., Islam S., Parvin S. (2017). Response of wheat to salicylic acid. Int. J. Sci. Res. Pub. 7 (12), 738–742.
Keywords: maize, BLSB, Rhizoctonia solani, salicylic acid, jasmonic acid, host defense activation management
Citation: Hamidi SM, Gogoi R, Kumar A, Singh A, Yadav R and Dorjee L (2023) Tackling banded leaf and sheath blight disease of maize through activation of host defense. Front. Agron. 5:1229717. doi: 10.3389/fagro.2023.1229717
Received: 10 June 2023; Accepted: 08 August 2023;
Published: 05 September 2023.
Edited by:
Giovanni Bubici, National Research Council (CNR), ItalyReviewed by:
Jiban Shrestha, Nepal Agricultural Research Council, NepalCopyright © 2023 Hamidi, Gogoi, Kumar, Singh, Yadav and Dorjee. This is an open-access article distributed under the terms of the Creative Commons Attribution License (CC BY). The use, distribution or reproduction in other forums is permitted, provided the original author(s) and the copyright owner(s) are credited and that the original publication in this journal is cited, in accordance with accepted academic practice. No use, distribution or reproduction is permitted which does not comply with these terms.
*Correspondence: Lham Dorjee, bGFtZG9yZzEyQGdtYWlsLmNvbQ==; Robin Gogoi, ci5nb2dvaWlhcmlAZ21haWwuY29t
Disclaimer: All claims expressed in this article are solely those of the authors and do not necessarily represent those of their affiliated organizations, or those of the publisher, the editors and the reviewers. Any product that may be evaluated in this article or claim that may be made by its manufacturer is not guaranteed or endorsed by the publisher.
Research integrity at Frontiers
Learn more about the work of our research integrity team to safeguard the quality of each article we publish.