- 1Molecular Modelling and Computer-Aided Drug Discovery Laboratory, Department of Bioinformatics, School of Earth, Biological and Environmental Sciences, Central University of South Bihar, Gaya, India
- 2Indian Council of Agricultural Research-Indian Institute of Wheat and Barley Research (ICAR-IIWBR), Karnal, India
- 3Department of Plant-Pathology, Faculty of Agricultural Sciences, Shree Guru Gobind Singh Tricentenary University, Gurgaon, India
Acetyl-CoA carboxylase (ACCase: EC 6.4.1.2) is one of the essential biotins containing enzymes required by plants for fatty acid synthesis and elongation. The unique enzyme is present in its homomeric form in all the Gramineae family, making it a suitable target for developing herbicides selectively against weeds of the Gramineae family. One such example is infestation of Phalaris minor in winter wheat crop fields, where aryloxyphenoxypropionates (FOP); cyclohexanediones (DIM) and phenyl pyrazoline (DEN) group of ACCase inhibiting herbicides are used. However, the increasing number of ACCase herbicide resistant weed populations has compelled agro-scientists to seek varied possibilities for weed control, through Integrated Weed Management (IWM) strategies. Developing new potential herbicides to regain sensitivity in weeds could be an approach to weed control. The current advancement in computational techniques could be of aid in developing new herbicide-like molecules by exploring the genomics, proteomics and structural details of catalytic sites of herbicide action in crops as well as weeds.
1 Introduction
Weeds are one of the most stubborn challenges for farmers, causing a reduction of ~35% in crop production (Oerke, 2006). There are broadly two ways to manage weed control: physical and chemical. Among the two, using chemical means (use of herbicides) is the more convenient and efficient method as it saves time and capital investment. Different herbicides have different targets, which have been classified accordingly into thirty-four groups (Heap, 2023) (https://www.hracglobal.com). Herbicides against six target sites: acetyl-CoA carboxylase (ACCase), acetolactate synthase (ALS), photosystem II (PSII), synthetic auxin, very long chain fatty acid (VLCFA) and protoporphyrinogen oxidase (PPO or Protox) are readily available and used. ACCase is the most studied among all the presently known herbicide targets. ACCase is a biotin-containing enzyme which is responsible for generating malonyl-CoA separately in plastids and cytoplasm of the plant cells, which is further used for fatty acid biosynthesis and elongation as well as secondary metabolites; respectively (Nikolau et al., 2003). Studies have revealed considerable variation in crop and weed grass ACCase protein, making it a suitable target to develop herbicides against them (Luo et al., 2012; Takano et al., 2020). Thus, ACCase-inhibiting herbicide has become a critical factor in controlling weeds (Naylor, 2008).
ACCase inhibitors, aka ‘Group A Herbicides’ precisely diclofop-methyl, were introduced in 1978 in the market (Neve and Powles, 2005; Kaundun, 2014). The newly introduced herbicides were selective against grass weeds, with zero to minimal harm on crop plants and lesser residual effect in the soil (Rendina and Felts, 1988; Chhokar and Sharma, 2008; Gherekhloo et al., 2021). Molecular studies have revealed the binding site of a protein and its interaction mechanism with herbicides. Herbicides of this group bind to the carboxylase transferase domain of the protein and block its functioning, thereby killing the plant (Zhang et al., 2004; Linda et al., 2010). The efforts of researchers have opened up the path to understanding the mechanism of resistance development in weeds due to misuse of herbicide doses. So far, numerous variants of ACCase have been reported in resistant biotypes of weeds (Gaines et al., 2020; Gherekhloo et al., 2021). The ACCase-resistant weeds has infested the crop fields of canola, chickpea, lentils, faba beans, peas, lupins, spring barley, flax and wheat causing a rapid decline in crop production (Heap, 2023). In a study conducted by the International Crop Research Institute for Semi-Arid Tropics (ICRISAT) out of the total annual loss of agricultural produce in India, weeds share almost 33% of the total losses.
Wheat grain production is drastically affected by a weed called ‘Little seed Canary grass’ i.e., Phalaris minor, which is an annual weed infesting winter season crops across many continents. Phalaris minor is a very competitive weed especially in wheat crops due to its similar morphology in the early growth stage. The problem of resistance is still increasing with the time swing which has led to devastation for wheat growers, causing a 15-50% loss in the total harvest (Rao et al., 2014; Gharde et al., 2018). Management of herbicide resistance usually depends on the understanding of the biology of the weed species, the reason for the development of resistance in them and the mechanism of interaction of herbicides at their respective target sites. By combining the knowledge of catalytic binding sites of herbicides and their mode of interaction, researchers can design and develop new herbicide-like molecules as well as incorporate a well-planned Integrated Weed Management (IWM) strategy to control weed infestation in crop fields.
2 Domain organization in ACCase protein
Biotin acts as an enzyme/biocatalyst to perform carboxylation, decarboxylation, and transcarboxylation in the central metabolism pathway. There are five different types of biotin-containing protein, among which four are biocatalysts, namely geranoyl-CoA carboxylase, 3-methylcrotonyl-CoA carboxylase, and the two structurally different acetyl-CoA carboxylases (ACCase) namely; heteromeric and homomeric ACCase (Wurtele and Nikolau, 1990). ACCase is the rate-limiting enzyme in fatty acid formations. It plays a key role as an intermediate between lipid and carbohydrate metabolism for fatty acid production (Luo et al., 2012). It is the first enzyme complex that catalyzes ATP-dependent carboxylation of acetyl-CoA to malonyl-CoA. For plants, ACCase directs the flow of carbon from photosynthesis to primary and secondary metabolites.
There are two distinct isoforms of ACCase in plants namely, the plastid ACCase (important in the biosynthesis of primary fatty acids) and the cytosolic ACCase (involved in the biosynthesis of long-chain fatty acids) (Yu et al., 2008). The heteromeric ACCase, which occurs in plastids of most of the plants of a non-Gramineae family, is composed of four independent polypeptides: Biotin Carboxylase- BC, Biotin Carboxyl Carrier protein- BCCP and two carboxyl transferase- α and β-CT, which is analogous to the organization of bacterial and archaeal ACCase (Sasaki and Nagano, 2004a; Zhang et al., 2004). Biochemical and molecular characterization has already been performed for heteromeric ACCase of various plant species such as Arabidopsis thaliana, Glycine max, Brassica napus, and Pisum sativum. Sequencing of ACCase protein has also been deduced, however 3D structural clarity of mature heteromeric sequence has not been figured out yet (Sun et al., 1997; Kaundun, 2014). Over the years researchers have studied and reported that the primary structure of each subunit of heteromeric ACCase has considerable conservation to other plant species and comparatively lower similarity to bacterial ACCase. Of the four heteromeric ACCase subunits, the 50-kDa BC subunit is the most highly conserved among plant species (Thelen et al., 2001; Cronan and Waldrop, 2002). Another isoform of the said biocatalyst is homomeric ACCase, a 500-kDa enzyme with two identical subunits. The enzyme is common in all the Gramineae plant families (grasses) (Incledon and Hall, 1997). Unlike heteromeric ACCase, where functional domains are distinctly classified as separate subunits. Homomeric ACCase has fused domains where functional domains (NH2-BC-BCCCT-COOH) are linearly arranged (Figure 1), thus providing a high degree of amino acid sequence conservation (Nikolau et al., 2003). Wheat crops lack heteromeric ACCase but have two isoforms of homomeric ACCase localized in the plastid and cytosol (Podkowinski et al., 2003).
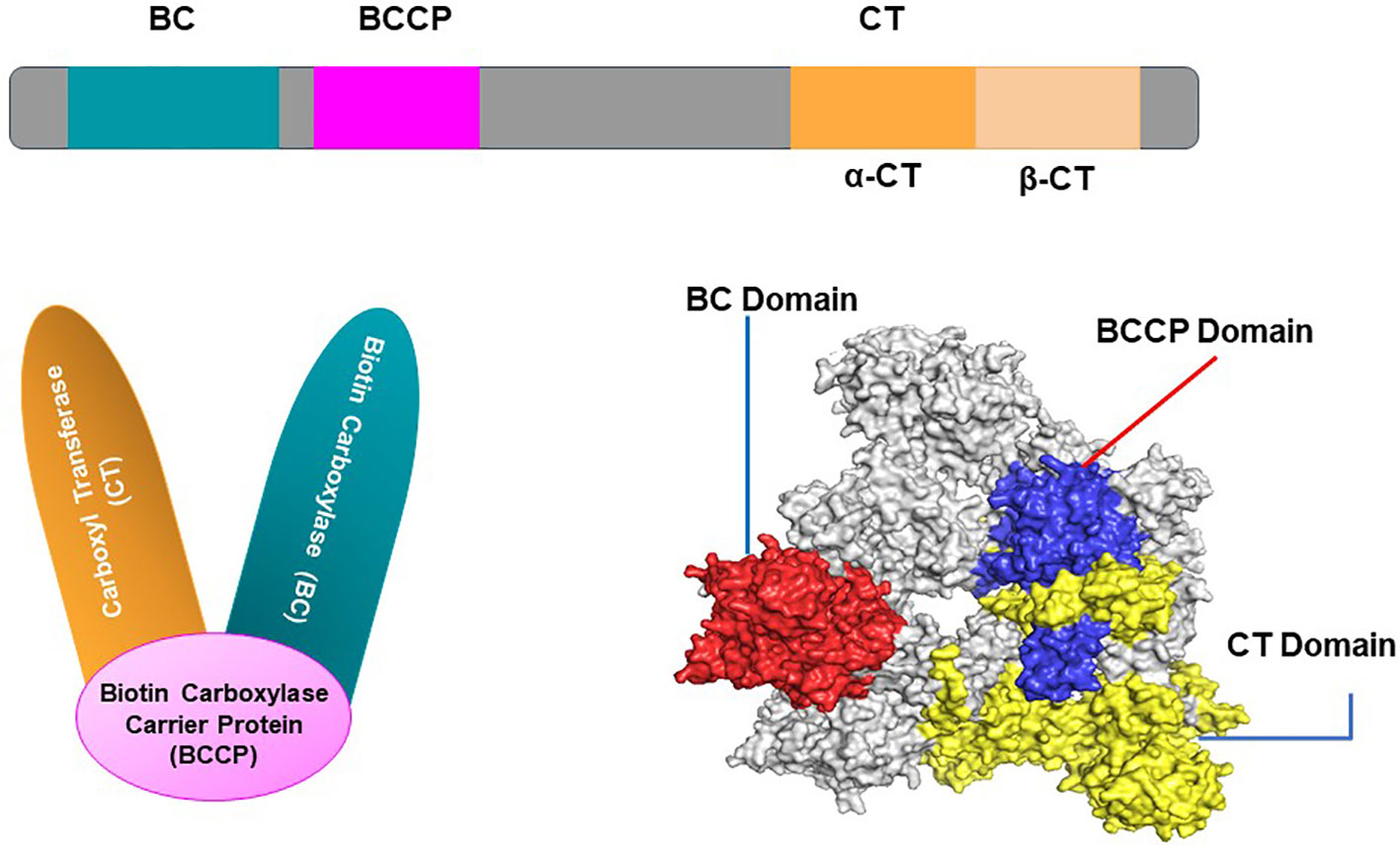
Figure 1 Domain organization of single unit of homomeric Acetyl-CoA Carboxylase (ACCase) protein, showing its three functioning regions namely, Biotin Carboxylase (BC), Biotin Carboxylase Carrier Protein (BCCP) and Catalytic Transferase (CT) domain.
Further studies have been conducted to identify the sequential differences among different homomeric ACCase of the Gramineae family, precisely among various infesting weeds and wheat. Multiple sequence alignments of Alopecurus (NCBI ID.- CAL63611.1), A. japonicus (NCBI ID.- AFD53915.1), and Beckmannia syzigachne (NCBI ID.- AGT45916.1) ACCase CT-domain amino acid sequences have been performed using Clustal Omega (https://www.ebi.ac.uk/Tools/msa/clustalo/) to evaluate evolutionary conserveness in protein profile. The alignment revealed that the major part of the CT-domain in protein sequence is conserved with hardly any variable regions that are not at the catalytic site of herbicides (Figure 2), which points towards the fact that there is little to no role of mutation in the selective activity of herbicides on weeds.
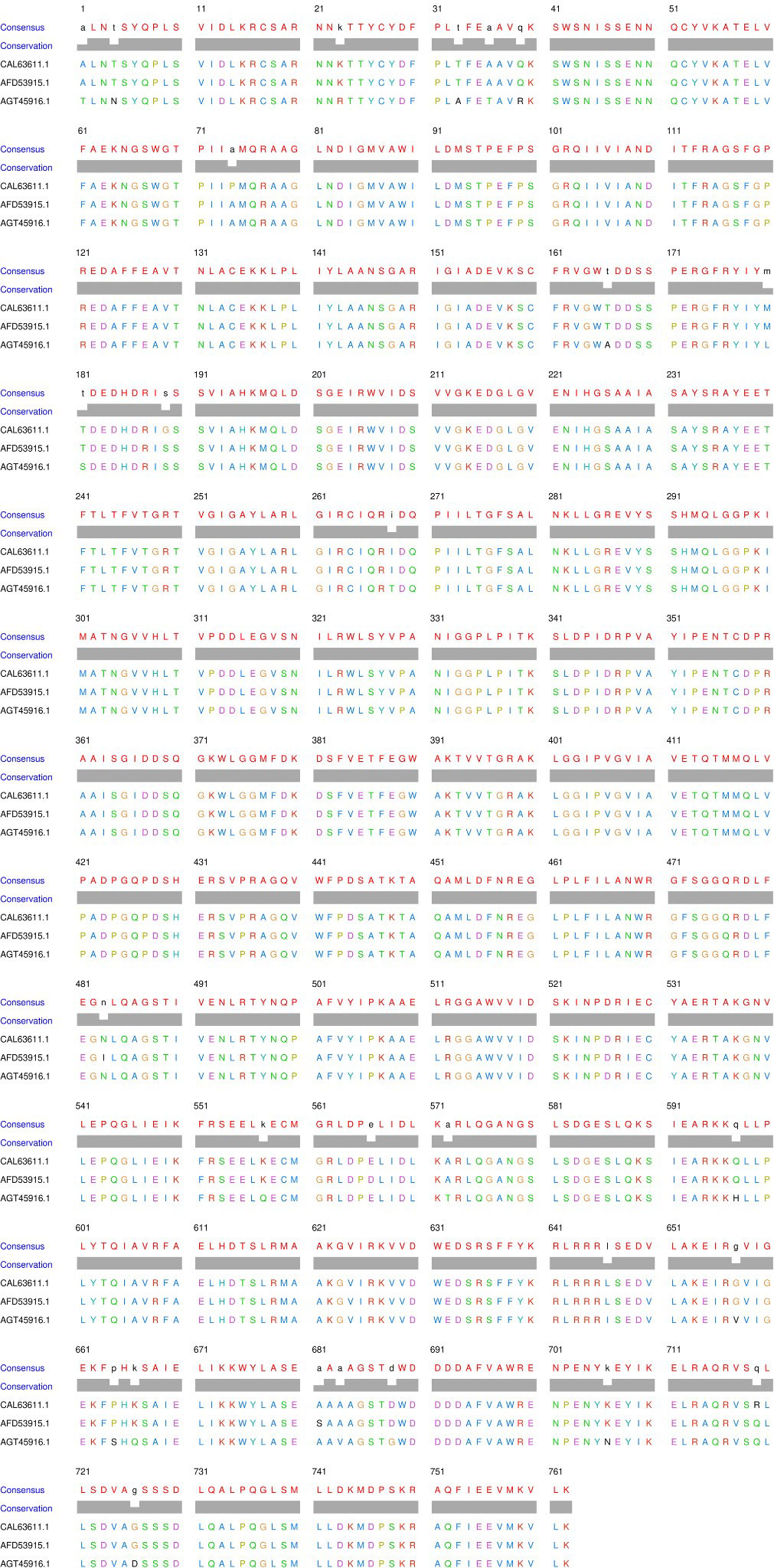
Figure 2 Multiple sequence alignment of the amino acid sequences of Alopecurus myosuroides, A. japonicus and Beckmannia syzigachne ACCase protein.
Studies have confirmed that resistance in crops including wheat from herbicides is accomplished majorly via the active metabolism of herbicides from crop plants by their detoxification with the help of cytochrome P450 (Cyt-P450) and glutathione S-transferase (GSTs) (De Prado et al., 2012). Active metabolism of chlorotoluron (Mougin et al., 1991), clodinafop-propargyl (Kreuz et al., 1991), and fenoxaprop-p-ethyl (Romano et al., 1993) in wheat mediated by Cyt-450 and GSTs, respectively, have been reported. Use of agrochemicals categorized as safeners (Davies and Caseley, 1999; Deng, 2022; Zhao et al., 2023) (chemical compounds used to diminish herbicide phytotoxicity in crops by the ease of physiological and molecular pathway alteration, without interfering in the activity of herbicides on weeds) in composition with herbicides have proved to increase expression of Cyt-P450 and GSTs selectively in grass crops, thereby enhancing detoxification in the crop from herbicides (Hatzios, 2003; Edwards et al., 2005)
3 Acetyl-CoA carboxylase: a biocatalyst
ACCase utilizes bicarbonate and ATP for carbon dioxide and energy sources (Konishi and Sasaki, 1994). The reaction pathway occurs in two steps: ATP-dependent transfer of CO2 from HCO3-to the nitrogen atom of the biotin prosthetic group of ACCase. The biotin is covalently bound to the ACCase enzyme at the carboxyl-terminal amino acid group of a lysine residue of the biotin side chain. The next step in the reaction is a transfer of active CO2 attached to biotin to acetyl-CoA. The acetyl-CoA carbon ion in the CT domain of the protein performs a nucleophilic attack on the carboxybiotin-enzyme. Finally, it transfers the active CO2 attached to biotin to acetyl-CoA. After this step, the biotin prosthetic group is again regenerated, and malonyl-CoA is formed (Sasaki and Nagano, 2004b; Takano et al., 2020).
(1) Biotin carboxyl carrier protein + HCO3 + Mg2+—ATP ⟶ Biotin carboxyl carrier protein—CO2 + Mg2+—ADP + Pi: Biotin carboxylase
(2) Biotin carboxyl carrier protein—CO2 + acetyl-CoA ⟶ Biotin carboxyl carrier protein + malonyl-CoA: carboxylase
After the reactions are complete, the protein reverts to its initial form without alteration in its structure and form. The reaction is the lead cause for the flux of carbon through the pathway (Figure 3).
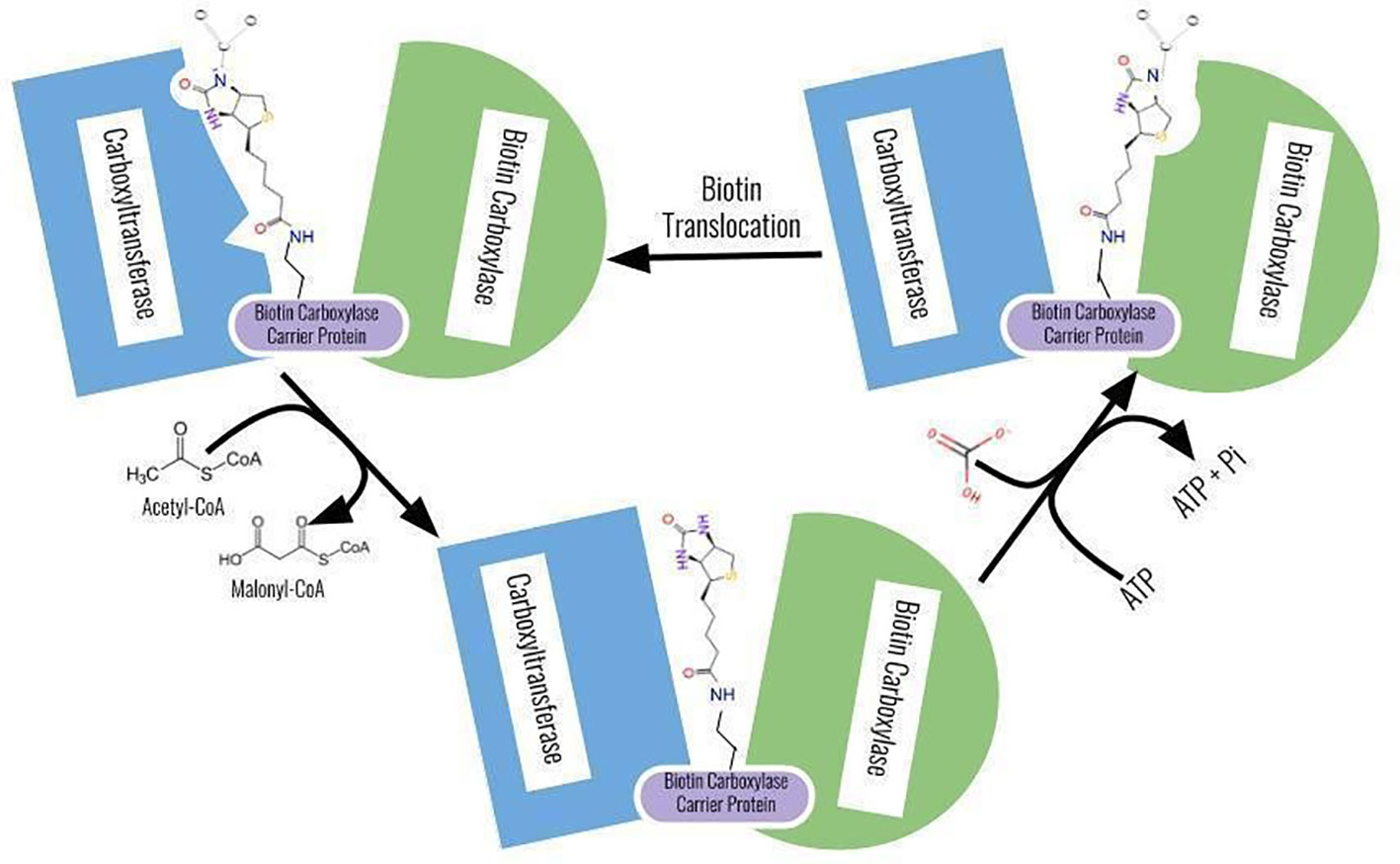
Figure 3 A schematic representation of the reaction of ACCase protein for its biological functioning. Source: Ohlrogge J, Browse J. Lipid biosynthesis. Plant Cell 1995.
Though there is considerable similarity in the pathway of ACCase biocatalytic action still, the plastid and cytoplasmic ACCase have a substantial difference in their structure, which enables herbicides to recognize and interact with plastid ACCase (Nikolskaya et al., 1999; Raghav et al., 2016; Takano et al., 2020). As discussed in the earlier section (Domain organization in ACCase protein), the linear homomeric ACCase is highly conserved with only a few changes between the sequence of different plant species. These small changes in amino acid sequence make it easy for researchers to develop selective herbicides against weeds that would not harm the crop (Green and Owen, 2011; Tehranchian et al., 2018).
4 ACCase: target for herbicide
ACCase is involved in various plants’ metabolic pathways, directly as well as indirectly, making it essential for their growth and survival. Since it is present in almost all plants and is vital for their survival, it has been used to develop several herbicides against weeds. The variation in the protein of crop’s amino acid sequence in comparison to weeds, encodes a structural variation in the binding site of herbicide. It allows the herbicide to interact with weed ACCase selectively (Umetsu and Shirai, 2020). However, before discovering structural differences, researchers kept on doing hits and trials to introduce herbicide molecules in the market, which also led to the discovery of non-selective herbicides (which acts upon all plants).
ACCase inhibiting herbicides (Group A, Table 1) are classified into three chemical families: aryloxyphenoxypropionate (APP or FOPs), cyclohexanedione (CHD or DIMs), and phenylpyrazole (DEN). The FOP and DIM group were introduced 45 years ago, while the DEN group herbicide that consists of pinoxaden was launched in the market in 2006 (Hofer et al., 2006; Dayan et al., 2019). These herbicides inhibit the ACCase enzyme activity and are typically used to control grass weeds during the cultivation of cereal crops or broadleaf crops. The molecules of these herbicidal groups consist of a similar carbon skeleton compared to a polar substitute. Still, at the same time, each one has its distinct features due to the presence of different functional groups in them (Délye, 2005). FOP groups are in the formulation of butyl, methyl, or ester, making them highly lipophilic, thus increasing the capacity to cross cell membranes (Shaner and Beckie, 2014). The residual activity of these herbicides has also been studied. It has been stated that they have limited residual activity in the soil, providing a higher value of solid-liquid partition and adsorption potential, i.e., herbicides become tightly bound to the soil. The bound molecules are absorbed by plant roots and then transform to their respective acid forms damaging the plants. However, such activity has been observed to last for only fourteen days (Lancaster et al., 2018).
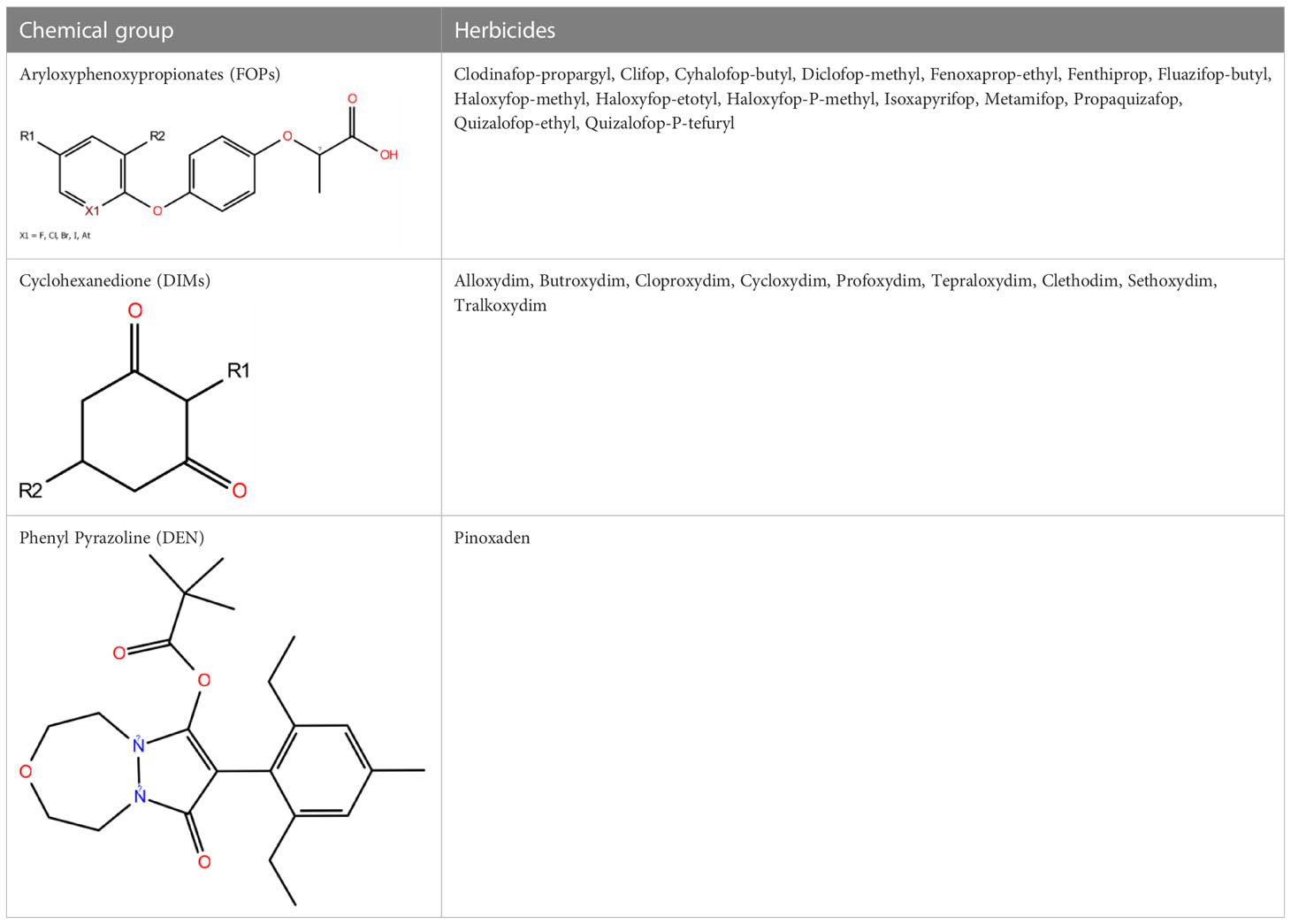
Table 1 ACCase inhibiting herbicides are classified into three groups based on their chemical scaffold (https://www.hracglobal.com).
5 ACCase interaction with herbicides
The activity of ACCase protein relies upon blocking fatty acid synthesis, which is a primary requirement for the regulation of plant growth hormones, lipids, and secondary metabolites (Heldt and Piechulla, 2011). The herbicide activity leads to a decrease in fatty acid synthesis, indirectly leading to the outflow of secondary metabolites and, thereby, plant disease (Délye, 2005; Kaundun, 2014). The herbicide translocation occurs in both the xylem and phloem tissue and travels to the meristematic region. Later, it is followed by the penetration of herbicides in the cell wall of weeds, leading to tissue damage and seepage of sap, which interrupts various metabolic pathways, leading to disruption in the growth of new leaves (Kukorelli et al., 2013). After one week of treatment, common symptoms in plants are evident, including initial greensickness followed by chlorosis in leaves (Dayan et al., 2019).
Biochemical and molecular studies have provided evidence that the catalytic binding site of FOPs, DIMs, and DEN group lies in the vicinity of the CT-domain of homomeric ACCase (Délye, 2005; Xia et al., 2016), though they bind slightly at different sites and interact with different amino acids, i.e., have different binding modes. After a comparative analysis of all the interacting residues of protein with FOP and DIM herbicide, it was divulged that they are conserved amino acid residues. Enzymatic studies of ACCase protein have proved that FOP and DIM group of herbicides are competitive inhibitors of ACCase substrate and non-competitive inhibitors HCO3-, Mg+2, and ATP, suggesting that they function by binding and blocking trans-carboxylation by CT-domain (Rendina et al., 1990; Burton et al., 1991; Devine, 2002). Crystal structure of Saccharomyces cerevisiae ACCase in complex with herbicides of different groups revealed that the molecules of other groups share common interacting amino acids (Ile1735 and Ala1627) as shown in Figure 4 and Table 2, giving ideas for a new class of herbicide (Xiang et al., 2009; Xia et al., 2016). Another interaction study of FOP and DIM group of herbicides with plastid ACCase protein of P. minor revealed that an essential interaction of FOP interacts with Ser133 amino acid residue for its binding at the CT-domain. While, the interaction of Ala56 and Ile160 amino acids is invariable for binding the DIM group to the CT domain (Rani et al., 2019)
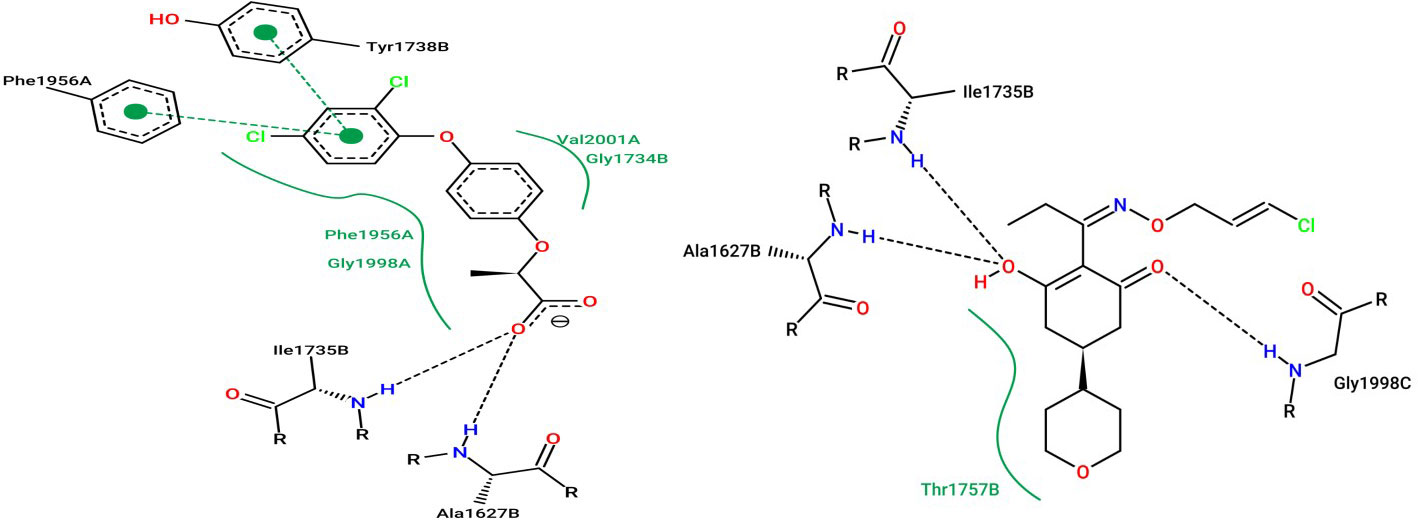
Figure 4 Interaction of (A) Diclofop: herbicide of aryloxyphenoxypropionates group, and (B) Tepraloxydim: herbicide of cyclohexanedione group, with the catalytic site residues of acetyl-CoA carboxylase protein.
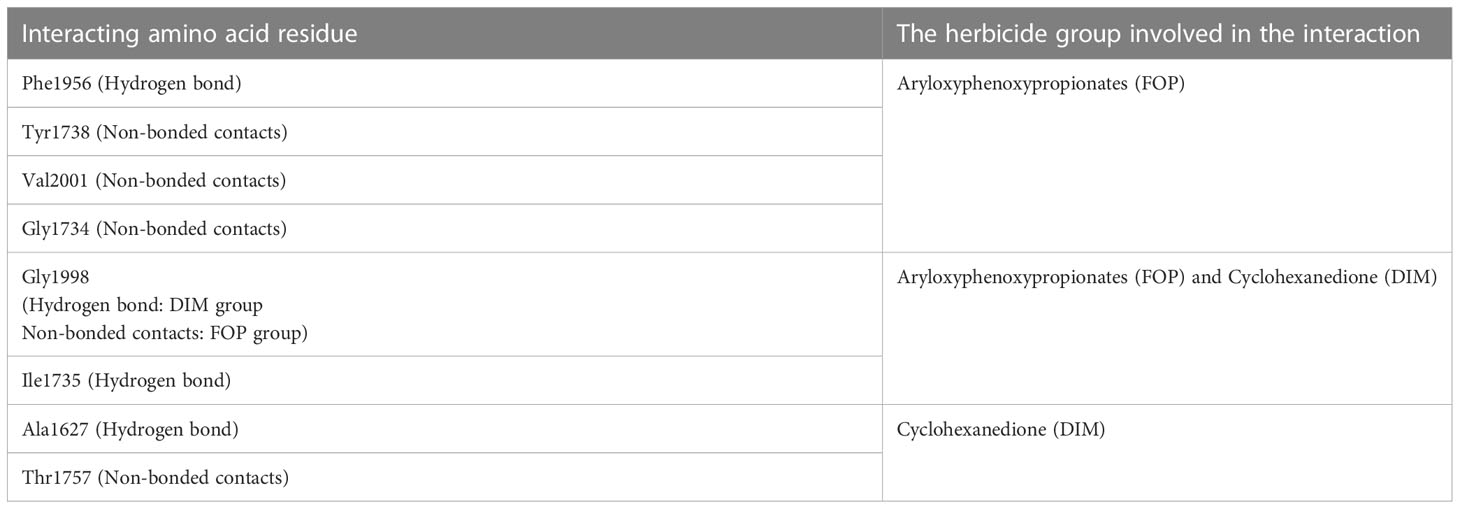
Table 2 Interacting amino acid residues of acetyl-CoA carboxylase protein with aryloxyphenoxypropionates (FOP) and cyclohexanedione (DIM) group of herbicide.
Understanding herbicide mode of action gave insight into the development of resistance in weeds against them. Studying mechanisms of action and classifying herbicides based upon their common mode of action instead of categorizing them based on the target site or their respective chemical families will help to ponder more deeply about the cause of resistance developed against them in plants (Singh et al., 2004). Interaction studies of herbicides have revealed that the most frequent cause of herbicide resistance development is a mutation at the target site by single or multiple amino acid changes at the CT-domain of homomeric ACCase protein. The mutation leads to a lower affinity for herbicides in resistant biotype plants without interpreting the normal biological function (Xiang et al., 2009; Zhu et al., 2009; Beckie and Tardif, 2012; Murphy and Tranel, 2019; Rani et al., 2019). However, mutations at non-target sites have also been noted that cause structural changes in protein, leading to alteration in binding sites.
6 Mutation in ACCase protein
Herbicides have been used to tackle the problem of weeds in crop fields ever since they were introduced into the market. Mismanagement and overdose of these herbicides have led to the issue of mutations in the target protein in weeds, making them less or non-sensitive against the herbicides, thereby enhancing the problem of weeds in crop fields leading to a loss in crop production. Homomeric ACCase enzyme of the Gramineae family is extremely sensitive toward aryloxyphenoxy propionates (APP) and cyclohexanedione (CHD) herbicide molecules (Burton et al., 1989; Sasaki and Nagano, 2004b; Délye et al., 2008; Vázquez-García et al., 2021), leading to mutations (Table 3).
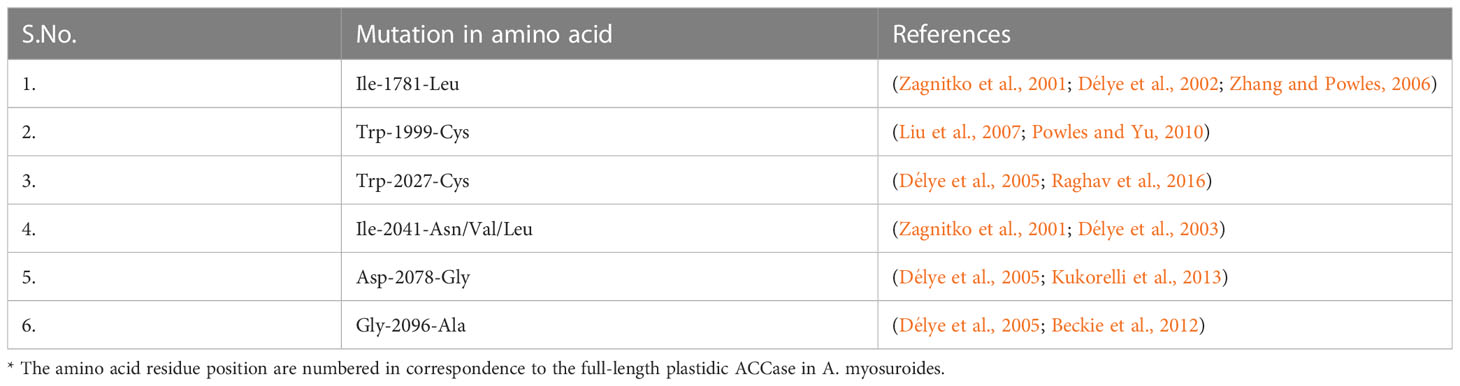
Table 3 Mutations in acetyl-CoA carboxylase protein, reported in the various species of the poaceae family.
Mutation in ACCase protein has been in several weed plants against various ACCase inhibiting herbicides. Point mutations (Trp-1999:Cys, Trp-2027:Cys, Cys-2088:Arg Asp-2078:Gly, Gly-2096:Ala, and Ile-2041:Asn) in ACCase of grasses have been reported, which lead to the development of herbicide-resistant weeds (Délye et al., 2008). Molecular studies have revealed that sensitive and resistant homomeric ACCase has the specific substitution of Ile to Lue in the CT domain, which changes the plastid ACCase from sensitive to the resistant enzyme (Zagnitko et al., 2001; Powles and Yu, 2010). Such mutations have been reported in P. minor, wheat, wild oats, and Setaria viridis L. Beauv, making the plants resistant to herbicides (Christoffers et al., 2002). There have also been reports of mutations that affect the sensitivity of more than one herbicide group towards the enzyme.
Several studies reported that Trp2027-Cys, Gly2096-Ala, and Ile2041-Asn mutation leads to resistance against APP herbicides while a mutation of Asp-2078-Gly caused resistance against APP as well as CHD herbicide group, which also includes clethodim (Beckie et al., 2000; Délye, 2005; Menchari et al., 2006; Liu et al., 2007; Yu et al., 2007; Kaundun, 2010). Some of the first reported mutations in P. minor’s ACCase protein in the Mexico population are Asp-2078:Gly and Ile-1781:Leu, which is the probable reason for the loss in herbicides’ affinity with the weed (Délye et al., 2008; Cruz-Hipolito et al., 2015; Raghav et al., 2016; Golmohammadzadeh et al., 2020).
These mutations causing resistance in weeds have become a challenge and difficult to control the weed population and regain the crop production to meet the world’s population needs. The production of wheat grain has been greatly reduced by the infestation of P. minor biotypes (Abbas et al., 2018; Gherekhloo et al., 2021; Rajak et al., 2023), which has become a world concern as wheat is the staple food in many parts of the world. Agricultural biologists have started a detailed study about the effect of these mutations, the interaction of herbicide at a molecular level with resistant and susceptible protein, insight into the catalytic site where herbicide binds in protein, etc., to develop effective herbicides to reclaim crop production.
7 Resistance management strategies
As formerly discussed in previous sections, herbicides did play a crucial role in increasing crop yields. But their repetitive application and overuse have led to the emergence of herbicide-resistant weed biotypes, posing a severe danger to the agricultural community by leading to deteriorated quality as well as quantity of crops. The reduction in crop production is not directly proportional to the increasing world population, but they are comparable (Food and Agriculture Organization of the United Nations, 2017; Calicioglu et al., 2019). Along with traditional farming cultural practices, different strategies are being used to control the weed infestation in the crop which include physical, biological and chemical methods as described below:
1. Physical method (mechanical and manual): It includes hand weeding and hoeing, digging, mowing and cutting, dredging and chaining, burning and flaming, soil solarization etc.
2. Cultural practices: Use of improved and certified seed varieties, planting at close row space with higher seed rate of the crop, sowing at the proper time, crop rotation, employing stale seed technique, intercultural, tillage, mulching etc. are some of the techniques employed as a cultural practice by farmers (Mohler et al., 2021).
3. It has been reported that the use of weed-contaminated wheat seeds increased the infestation of resistant P. minor at an alarming rate (Yadav et al., 2002; Travlos et al., 2020). As per general seed certification standards 2-5 P. minor seeds kg-1 is permissible in wheat.
4. Biological methods: To inhibit the infestation and growth of weeds herbivorous fishes, insects, and other animals could be used as biological agents. Competitive crop varieties are also being used to suppress the growth of weeds in crop fields. Competitive wheat varieties with unique genotypes (HD2787, WH542, PBW343) which have quick growth in initial stages are underused for weed management (Chauhan et al., 2001; Malik et al., 2002). Through this method, weed is not completely eradicated but its population is controlled. The use of crops population with resistant properties (non-transgenic) or genetic modification of crops (transgenic varieties) to develop herbicide-resistant properties has also greatly improved weed management strategies. Corn, canola, sorghum, corn, alfalfa, wheat, sugar beets, sunflower, rice etc. are some of the herbicide-resistant crop plants that are commercially available in the market (Duke, 2005).
5. Chemical control: Crude oil, rock salt, waste oil, arsenical ores and sulfuric acid have been in use for centuries to eradicate weeds (Bell, 2015), however, none of the chemicals had a selective effect on weeds. The breakthrough in selective chemical weed control was marked after the discovery of 2,4-D and MCAP in 1945 (Green and Castle, 2010).
The agricultural field is the reservoir of many weed seeds (seed banks), among which many may germinate upon irrigation or may remain in their dormant stage. Conventional weed control practices are only feasible to control the germinated seeds. In contrast, the remaining dormant seed in the seed bank reminisce unaffected in the soil and will become the cause of the infestation in the next cropping. It is important to integrate cultural practices, physical, chemical and mechanical methods to tackle the problem of resistant weed infestation (Kaur et al., 2022). This management scheme that incorporates multiple weed control techniques (physical, chemical and biological) in an integrated manner without emphasized reliance on a single technique to tackle weed infestation, and reduce their population below the economic threshold is termed Integrated Weed Management (IWM) (Friesen et al., 2000; Scavo and Mauromicale, 2020). IWM strategy is more efficient, as leftover weeds that remain in the field after one method can be eliminated with another weed management technique, which results in the reduction of the soil seed bank.
8 The rational approach to herbicide discovery
Using conventional methods to design, discover and develop new effective herbicide molecules is practically inconvenient due to enormous amounts of manual work and capital investment (Sparks and Lorsbach, 2017; Berestetskiy, 2023). In this era, agro-researchers are leaning more toward a computational and rational approach based on the genomic study (Gressel, 2011; Duke et al., 2019) predicted protein structure, predicted interaction study of molecules with protein (molecular docking and dynamics study), stability of bonds (interpreted through bond energies) etc. (Stewart et al., 2009; Horvath, 2010; Busi et al., 2013; Rajak et al., 2023). These studies are of utmost importance to unravelling the catalytic site of protein (susceptible and resistance type) and its interaction with molecules. It will give us an insight into the pharmacophoric features of the catalytic site and the minute difference in the catalytic site that changes the susceptible protein to resistance against herbicide. This knowledge would be helpful in the future to construct a potential compatible herbicide against the said protein.
A study has been carried out for sensitive and resistant ACCase protein of P. minor against the FOP and DIM group of herbicides, revealing the fact that there is overlap in the binding niche for both the group of herbicides which could be used to develop new molecules that can occupy whole binding site of both groups and make the new molecule more efficient (Singh et al., 2012). The same group has done in vitro testing on P. minor plantlets with several new molecules with herbicide activity and has discovered a molecule (6-ethoxy-4-N-(2-morpholin-4-ylethyl)-2-N-propan-2-yl-1,3,5-triazine-2,4-diamine) that is more efficient than the pre-existing herbicides (Singh and Rani, 2020) using a refined physiochemical parameter to determine molecules with a herbicide like properties (Rani et al., 2023). It would be safe to say that such studies could help to understand the interaction study of herbicide and protein with thousands and lakhs of possible molecules (Virtual High Throughput Screening) in real-time through computational means. It would save lots of time, labour, and capital investment, proving an excellent aid for agrochemical industries. Interaction study of known herbicides with the 3D structure of ACCase could also be performed computationally to decipher their mechanism of action and categorize them based on their mode of action, which might help in explaining weed resistance development.
9 Final remark
The importance of ACCase in the biological functioning of plants makes it a relevant herbicide target. Herbicides with a selective effect on weed ACCase have been introduced in past to control weed infestation in the field. However, due to the development and prevalence of resistant weed biotypes previously introduced herbicides are either no longer efficient or completely ineffective in weed infestation. One way to tackle the problem of ACCase-resistant weed populations is advancement in herbicide development strategies, which may include the computational approach to precisely design binding site-compatible herbicide-like molecules. Furtherance in technology has fueled the process by saving time and capital, and now it is possible to test hundreds of thousands of molecules for their potential within a few hours. Furthermore, integration of other weed management techniques (IWM) could be employed to achieve effective control over the monstrous problem of rapid weed infestations in crop fields.
Author contributions
BR and PR designed and contributed to the conception of the manuscript and drafted the manuscript. PM and NS contributed to the manuscript preparation and DS and RC supervised the manuscript and approved the final manuscript. All authors contributed to the article and approved the submitted version.
Funding
The authors are thankful to the Department of Science and Technology, India (File No. NASI/SoRFI/2014-15/19), Science and Engineering Research Board, India (File No. YSS/2015/001662), and the Department of Biotechnology, India (File No. BT/PR2487/AGIII/103/1179/2019), for their financial assistance.
Conflict of interest
The authors declare that the research was conducted in the absence of any commercial or financial relationships that could be construed as a potential conflict of interest.
Publisher’s note
All claims expressed in this article are solely those of the authors and do not necessarily represent those of their affiliated organizations, or those of the publisher, the editors and the reviewers. Any product that may be evaluated in this article, or claim that may be made by its manufacturer, is not guaranteed or endorsed by the publisher.
References
Abbas T., Nadeem M. A., Tanveer A., Ali H. H., Farooq N. (2018). Role of allelopathic crop mulches and reduced doses of tank-mixed herbicides in managing herbicide-resistant Phalaris minor in wheat. Crop Prot. 110, 245–250. doi: 10.1016/j.cropro.2017.06.012
Beckie H. J., Heap I. M., Smeda R. J., Hall L. M. (2000). Screening for herbicide resistance in Weeds1. Weed Technol. 14, 428–445. doi: 10.1614/0890-037X(2000)014[0428:SFHRIW]2.0.CO;2
Beckie H. J., Tardif F. J. (2012). Herbicide cross resistance in weeds. Crop Prot. 35, 15–28. doi: 10.1016/j.cropro.2011.12.018
Beckie H. J., Warwick S. I., Sauder C. A. (2012). Basis for herbicide resistance in Canadian populations of wild oat (Avena fatua). Weed Sci. 60, 10–18. doi: 10.1614/WS-D-11-00110.1
Bell C. (2015). A historical view of weed control technology. Univ. California Weed Science. Available at: http://ucanr.edu/blogs/blogcore/postdetail.cfm?postnum=17593 (Accessed October 2, 2022).
Berestetskiy A. (2023). Modern approaches for the development of new herbicides based on natural compounds. Plants 12, 234. doi: 10.3390/plants12020234
Burton J. D., Gronwald J. W., Keith R. A., Somers D. A., Gengenbach B. G., Wyse D. L. (1991). Kinetics of inhibition of acetyl-coenzyme a carboxylase by sethoxydim and haloxyfop. Pesticide Biochem. Physiol. 39, 100–109. doi: 10.1016/0048-3575(91)90130-E
Burton J. D., Gronwald J. W., Somers D. A., Gengenbach B. G., Wyse D. L. (1989). Inhibition of corn acetyl-CoA carboxylase by cyclohexanedione and aryloxyphenoxypropionate herbicides. Pesticide Biochem. Physiol. 34, 76–85. doi: 10.1016/0048-3575(89)90143-0
Busi R., Vila-Aiub M. M., Beckie H. J., Gaines T. A., Goggin D. E., Kaundun S. S., et al. (2013). Herbicide-resistant weeds: from research and knowledge to future needs. Evolutionary Appl. 6, 1218–1221. doi: 10.1111/eva.12098
Calicioglu O., Flammini A., Bracco S., Bellù L., Sims R. (2019). The future challenges of food and agriculture: an integrated analysis of trends and solutions. Sustainability 11, 222. doi: 10.3390/su11010222
Chauhan B. S., Yadav A., Malik R. K. (2001). Competitive wheat genotypes under zero tillage: an important tool to manage resistant Phalaris minor. Indian J. Weed Sci. 33, 75–76.
Chhokar R. S., Sharma R. K. (2008). Multiple herbicide resistance in littleseed canarygrass (Phalaris minor): a threat to wheat production in India. Weed Biol. Manage. 8, 112–123. doi: 10.1111/j.1445-6664.2008.00283.x
Christoffers M. J., Berg M. L., Messersmith C. G. (2002). An isoleucine to leucine mutation in acetyl-CoA carboxylase confers herbicide resistance in wild oat. Genome 45, 1049–1056. doi: 10.1139/g02-080
Cronan J. E. Jr., Waldrop G. L. (2002). Multi-subunit acetyl-CoA carboxylases. Prog. Lipid Res. 41, 407–435. doi: 10.1016/S0163-7827(02)00007-3
Cruz-Hipolito H., Fernandez P., Alcantara R., Gherekhloo J., Osuna M. D., De Prado R. (2015). Ile-1781-Leu and asp-2078-Gly mutations in ACCase gene, endow cross-resistance to APP, CHD, and PPZ in Phalaris minor from Mexico. Int. J. Mol. Sci. 16, 21363–21377. doi: 10.3390/ijms160921363
Davies J., Caseley J. C. (1999). Herbicide safeners: a review. Pesticide Sci. 55, 1043–1058. doi: 10.1002/(SICI)1096-9063(199911)55:11<1043::AID-PS60>3.0.CO;2-L
Dayan F. E., Barker A., Bough R., Ortiz M., Takano H., Duke S. O. (2019). Herbicide mechanisms of action and resistance. In Comprehensive Biotechnology, 3rd ed.; Grodzinski B., Ed. (Elsevier: Amsterdam, The Netherlands), 4. doi: 10.1016/B978-0-444-64046-8.00211-1
Délye C. (2005). Weed resistance to acetyl coenzyme a carboxylase inhibitors: an update. Weed Sci. 53, 728–746. doi: 10.1614/WS-04-203R.1
Délye C., Matéjicek A., Gasquez J. (2002). PCR-based detection of resistance to acetyl-CoA carboxylase-inhibiting herbicides in black-grass (Alopecurus mysosuroides huds) and ryegrass (Lolium rigidum gaud). Pest Manage. Sci. 58, 474–478. doi: 10.1002/ps.485
Délye C., Matéjicek A., Michel S. (2008). Cross-resistance patterns to ACCase-inhibiting herbicides conferred by mutant ACCase isoforms in Alopecurus mysosuroides huds.(black-grass), re-examined at the recommended herbicide field rate. Pest Manage. Science: formerly Pesticide Sci. 64, 1179–1186. doi: 10.1002/ps.1614
Délye C., Zhang X.-Q., Chalopin C., Michel S., Powles S. B. (2003). An isoleucine residue within the carboxyl-transferase domain of multidomain acetyl-coenzyme a carboxylase is a major determinant of sensitivity to aryloxyphenoxypropionate but not to cyclohexanedione inhibitors. Plant Physiol. 132, 1716–1723. doi: 10.1104/pp.103.021139
Délye C., Zhang X.-Q., Michel S., Matéjicek A., Powles S. B. (2005). Molecular bases for sensitivity to acetyl-coenzyme a carboxylase inhibitors in black-grass. Plant Physiol. 137, 794–806. doi: 10.1104/pp.104.046144
Deng X. (2022). Current advances in the action mechanisms of safeners. Agronomy 12, 2824. doi: 10.3390/agronomy12112824
De Prado R., Jorrín J., García-Torres L. (2012). Weed and crop resistance to herbicides (Springer Science & Business Media).
Devine M. D. (2002). “Acetyl-CoA Carboxylase Inhibitors ,” In: Böger, P., Wakabayashi, K., Hirai, K. (eds) in Herbicide Classes in Development, Berlin, Heidelberg:Springer. doi: 10.1007/978-3-642-59416-8_5
Duke S. O. (2005). Taking stock of herbicide-resistant crops ten years after introduction. Pest Manage. Science: formerly Pesticide Sci. 61, 211–218. doi: 10.1002/ps.1024
Duke S. O., Stidham M. A., Dayan F. E. (2019). A novel genomic approach to herbicide and herbicide mode of action discovery. Pest Manage. Sci. 75, 314–317. doi: 10.1002/ps.5228
Edwards R., Brazier-Hicks M., Dixon D. P., Cummins I. (2005). Chemical manipulation of antioxidant defences in plants. Adv. Botanical Res. 42, 1–32. doi: 10.1016/S0065-2296(05)42001-7
Food and Agriculture Organization of the United Nations (2017). The future of food and agriculture: trends and challenges (Rome: Food and Agriculture Organization of the United Nations).
Friesen L. S., Ferguson G. M., Hall J. C. (2000). Management strategies for attenuating herbicide resistance: untoward consequences of their promotion. Crop Prot. 19, 891–895. doi: 10.1016/S0261-2194(00)00116-2
Gaines T. A., Duke S. O., Morran S., Rigon C. A., Tranel P. J., Küpper A., et al. (2020). Mechanisms of evolved herbicide resistance. J. Biol. Chem. 295, 10307–10330. doi: 10.1074/jbc.REV120.013572
Gharde Y., Singh P. K., Dubey R. P., Gupta P. K. (2018). Assessment of yield and economic losses in agriculture due to weeds in India. Crop Prot. 107, 12–18. doi: 10.1016/j.cropro.2018.01.007
Gherekhloo J., Hassanpour-Bourkheili S., Hejazirad P., Golmohammadzadeh S., Vazquez-Garcia J. G., De Prado R. (2021). Herbicide resistance in Phalaris species: a review. Plants 10, 2248. doi: 10.3390/plants10112248
Golmohammadzadeh S., Rojano-Delgado A. M., Vázquez-García J. G., Romano Y., Osuna M. D., Gherekhloo J., et al. (2020). Cross-resistance mechanisms to ACCase-inhibiting herbicides in short-spike canarygrass (Phalaris brachystachys). Plant Physiol. Biochem. 151, 681–688. doi: 10.1016/j.plaphy.2020.03.037
Green J. M., Castle L. A. (2010). “Transitioning from single to multiple herbicide-resistant crops,” in Glyphosate resistance in crops and weeds: history, development, and management, 67–91.
Green J. M., Owen M. D. (2011). Herbicide-resistant crops: utilities and limitations for herbicide-resistant weed management. J. Agric. Food Chem. 59, 5819–5829. doi: 10.1021/jf101286h
Gressel J. (2011). Global advances in weed management. J. Agric. Sci. 149, 47–53. doi: 10.1017/S0021859610000924
Hatzios K. K. (2003). Herbicide safeners: effective inducers of plant defense gene-enzyme systems. Phytoparasitica 31, 3–7. doi: 10.1007/BF02979761
Heap I. (2023) The international herbicide-resistant weed database. Available at: http://www.weedscience.org/.
Heldt H. W., Piechulla B. (2011). “15-lipids are membrane constituents and function as carbon stores,” in Plant biochemistry, 4th ed. (San Diego: Academic Press), 359–398.
Hofer U., Muehlebach M., Hole S., Zoschke A. (2006). Pinoxaden-for broad spectrum grass weed management in cereal crops. Z. FUR PFLANZENKRANKHEITEN UND PFLANZENSCHUTZ-SONDERHEFT- 20, 989.
Horvath D. (2010). Genomics for weed science. Curr. Genomics 11, 47–51. doi: 10.2174/138920210790217972
Incledon B. J., Hall J. C. (1997). Acetyl-coenzyme a carboxylase: quaternary structure and inhibition by graminicidal herbicides. Pesticide Biochem. Physiol. 57, 255–271. doi: 10.1006/pest.1997.2279
Kaundun S. S. (2010). An aspartate to glycine change in the carboxyl transferase domain of acetyl-CoA carboxylase and non-target-site mechanism(s) confer resistance to ACCase inhibitor herbicides in a Lolium multiflorum population: resistance to ACCase herbicides in L. multiflorum. Pest. Manage. Sci. 66, 1249–1256. doi: 10.1002/ps.2003
Kaundun S. S. (2014). Resistance to acetyl-CoA carboxylase-inhibiting herbicides. Pest Manage. Sci. 70, 1405–1417. doi: 10.1002/ps.3790
Kaur S., Dhanda S., Yadav A., Sagwal P., Yadav D. B., Chauhan B. S. (2022). Current status of herbicide-resistant weeds and their management in the rice-wheat cropping system of south Asia. Adv. Agron. 172, 307–354. doi: 10.1016/bs.agron.2021.10.004
Konishi T., Sasaki Y. (1994). Compartmentalization of two forms of acetyl-CoA carboxylase in plants and the origin of their tolerance toward herbicides. Proc. Natl. Acad. Sci. 91, 3598–3601. doi: 10.1073/pnas.91.9.3598
Kreuz K., Gaudin J., Stingelin J., Ebert E. (1991). "Metabolism of the Aryloxyphenoxypropanoate Herbicide, CGA 184927, in Wheat, Barley and Maize: Differential Effects of the Safener, CGA 185072" Zeitschrift für Naturforschung C, vol. 46, no. 9-10, 1991, pp. 901–905. doi: 10.1515/znc-1991-9-1030
Kukorelli G., Reisinger P., Pinke G. (2013). ACCase inhibitor herbicides–selectivity, weed resistance and fitness cost: a review. Int. J. Pest Manage. 59, 165–173. doi: 10.1080/09670874.2013.821212
Lancaster Z. D., Norsworthy J. K., Scott R. C. (2018). Residual activity of ACCase-inhibiting herbicides on monocot crops and weeds. Weed Technol. 32, 364–370. doi: 10.1017/wet.2018.13
Linda P. C., Kim Y. S., Tong L. (2010). Mechanism for the inhibition of the carboxyltransferase domain of acetyl-coenzyme a carboxylase by pinoxaden. Proc. Natl. Acad. Sci. 107, 22072–22077. doi: 10.1073/pnas.1012039107
Liu W., Harrison D. K., Chalupska D., Gornicki P., O’Donnell C. C., Adkins S. W., et al. (2007). Single-site mutations in the carboxyltransferase domain of plastid acetyl-CoA carboxylase confer resistance to grass-specific herbicides. Proc. Natl. Acad. Sci. U. S. A. 104, 3627–3632. doi: 10.1073/pnas.0611572104
Luo D.-X., Tong D.-J., Rajput S., Wang C., Liao D.-F., Cao D., et al. (2012). Targeting acetyl-CoA carboxylases: small molecular inhibitors and their therapeutic potential. Recent Patents Anti-Cancer Drug Discov. 7, 168–184. doi: 10.2174/157489212799972918
Malik R. K., Yadav A., Singh S., Malik R. S., Balyan R. S., Banga R. S., et al. (2002). Herbicide resistance management and evolution of zero tillage-a success story. Res. Bull. 2002, 1–43.
Menchari Y., Camilleri C., Michel S., Brunel D., Dessaint F., Le Corre V., et al. (2006). Weed response to herbicides: regional-scale distribution of herbicide resistance alleles in the grass weed Alopecurus mysosuroides. New Phytol. 171, 861–874. doi: 10.1111/j.1469-8137.2006.01788.x
Mohler C. L., Teasdale J. R., DiTommaso A. (2021). Manage weeds on your farm. SARE Outreach 416. doi: 10.13016/xhlk-vt7c
Mougin C., Polge N., Scalla R., Cabanne F. (1991). Interactions of various agrochemicals with cytochrome p-450-dependent monooxygenases of wheat cells. Pesticide Biochem. Physiol. 40, 1–11. doi: 10.1016/0048-3575(91)90044-M
Murphy B. P., Tranel P. J. (2019). Target-site mutations conferring herbicide resistance. Plants 8, 382. doi: 10.3390/plants8100382
Neve P., Powles S. (2005). Recurrent selection with reduced herbicide rates results in the rapid evolution of herbicide resistance in Lolium rigidum. Theor. Appl. Genet. 110, 1154–1166. doi: 10.1007/s00122-005-1947-2
Nikolau B. J., Ohlrogge J. B., Wurtele E. S. (2003). Plant biotin-containing carboxylases. Arch. Biochem. biophysics 414, 211–222. doi: 10.1016/S0003-9861(03)00156-5
Nikolskaya T., Zagnitko O., Tevzadze G., Haselkorn R., Gornicki P. (1999). Herbicide sensitivity determinant of wheat plastid acetyl-CoA carboxylase is located in a 400-amino acid fragment of the carboxyltransferase domain. Proc. Natl. Acad. Sci. U.S.A. 96, 14647–14651. doi: 10.1073/pnas.96.25.14647
Podkowinski J., Jelenska J., Sirikhachornkit A., Zuther E., Haselkorn R., Gornicki P. (2003). Expression of cytosolic and plastid acetyl-coenzyme a carboxylase genes in young wheat plants. Plant Physiol. 131, 763–772. doi: 10.1104/pp.013169
Powles S. B., Yu Q. (2010). Evolution in action: plants resistant to herbicides. Annu. Rev. Plant Biol. 61, 317–347. doi: 10.1146/annurev-arplant-042809-112119
Raghav N., Singh R., Chhokar R. S., Sharma D., Kumar R. (2016). Mutations in the plastidic ACCase gene endowing resistance to ACCase-inhibiting herbicide in Phalaris minor populations from India. 3 Biotech. 6, 1–5. doi: 10.1007/s13205-015-0331-4
Rajak B. K., Rani P., Singh N., Singh D. V. (2023). Sequence and structural similarities of ACCase protein of Phalaris minor and wheat: an insight to explain herbicide selectivity. Front. Plant Sci. 13. doi: 10.3389/fpls.2022.1056474
Rani P., Kumari J., Agarwal S., Singh D. V. (2019). Binding mode of aryloxyphenoxypropionate (FOP) and cyclohexanedione (DIM) groups of herbicides at the carboxyl transferase (CT) domain of acetyl-CoA carboxylase of Phalaris minor. Network Modeling Anal. Health Inf. Bioinf. 8, 1–14. doi: 10.1007/s13721-019-0190-8
Rani P., Rajak B. K., Singh D. V. (2023). Physicochemical parameters for design and development of lead herbicide molecules: is ‘Lipinski’s rule of 5′ appropriate for herbicide discovery? Pest Manage. Sci. 79, 1931–1943. doi: 10.1002/ps.7367
Rao A. N., Wani S. P., Ladha J. K. (2014). Weed management research in India - an analysis of the past and outlook for future. In: DWR -Souvenir (1989–2014). DWR Publication No. 18. Directorate of Weed Research, Jabalpur, India, pp. 1–26. Available at: http://oar.icrisat.org/id/eprint/8592.
Ray B. (1975). Nutsedge- world's worst weed. Pesticides. 9, 15–17. Available at: http://oar.icrisat.org/id/eprint/8592
Rendina A. R., Craig-Kennard A. C., Beaudoin J. D., Breen M. K. (1990). Inhibition of acetyl-coenzyme a carboxylase by two classes of grass-selective herbicides. J. Agric. Food Chem. 38, 1282–1287. doi: 10.1021/jf00095a029
Rendina A. R., Felts J. M. (1988). Cyclohexanedione herbicides are selective and potent inhibitors of acetyl-CoA carboxylase from grasses. Plant Physiol. 86, 983–986. doi: 10.1104/pp.86.4.983
Romano M. L., Stephenson G. R., Tal A., Hall J. C. (1993). The effect of monooxygenase and glutathione s-transferase inhibitors on the metabolism of diclofop-methyl and fenoxaprop-ethyl in barley and wheat. Pesticide Biochem. Physiol. 46, 181–189. doi: 10.1006/pest.1993.1049
Sasaki Y., Nagano Y. (2004a). Cronan jr & waldrop 2002. Bioscience biotechnology Biochem. 68, 1175–1184. doi: 10.1271/bbb.68.1175
Sasaki Y., Nagano Y. (2004b). Plant acetyl-CoA carboxylase: structure, biosynthesis, regulation, and gene manipulation for plant breeding. Bioscience biotechnology Biochem. 68, 1175–1184. doi: 10.1271/bbb.68.1175
Scavo A., Mauromicale G. (2020). Integrated weed management in herbaceous field crops. Agronomy 10, 466. doi: 10.3390/agronomy10040466
Shaner D. L., Beckie H. J. (2014). Lancaster. Pest. Manage. Sci. 70, 1329–1339. doi: 10.1002/ps.3706
Singh D. V., Agarwal S., Kesharwani R. K., Misra K. (2012). Molecular modeling and computational simulation of the photosystem-II reaction center to address isoproturon resistance in Phalaris minor. J. Mol. Model. 18, 3903–3913. doi: 10.1007/s00894-012-1386-3
Singh D. V., Gaur A. K., Mishra D. P. (2004). Biochemical and molecular mechanisms of resistance against isoproturon in Phalaris minor: variations in protein and RAPD profiles of isoproturon resistant and sensitive Phalaris minor biotypes. Indian J. Weed Sci. 36, 256–259.
Singh D. V., Rani P. (2020) 6-ethoxy-4-n-(2-morpholin-4-ylethyl)-2-n-propan-2-yl-1,3,5-triazine-2,4-diamine endowed with herbicidal activity against susceptible and resistant biotypes of Phalaris minor. 30. Available at: http://ipindia.gov.in/writereaddata/Portal/IPOJournal/1_4931_1/Part-1.pdf.
Sparks T. C., Lorsbach B. A. (2017). Perspectives on the agrochemical industry and agrochemical discovery. Pest Manage. Sci. 73, 672–677. doi: 10.1002/ps.4457
Stewart C. N., Tranel P. J., Horvath D. P., Anderson J. V., Rieseberg L. H., Westwood J. H., et al. (2009). Evolution of weediness and invasiveness: charting the course for weed genomics. Weed Sci. 57, 451–462. doi: 10.1614/WS-09-011.1
Sun J., Ke J., Johnson J. L., Nikolau B. J., Wurtele E. S. (1997). Biochemical and molecular biological characterization of CAC2, the Arabidopsis thaliana gene coding for the biotin carboxylase subunit of the plastidic acetyl-coenzyme a carboxylase. Plant Physiol. 115, 1371–1383. doi: 10.1104/pp.115.4.1371
Takano H. K., Ovejero R. F. L., Belchior G. G., Maymone G. P. L., Dayan F. E. (2020). ACCase-inhibiting herbicides: mechanism of action, resistance evolution and stewardship. Scientia Agricola 78. doi: 10.1590/1678-992X-2019-0102
Tehranchian P., Nandula V., Jugulam M., Putta K., Jasieniuk M. (2018). Multiple resistance to glyphosate, paraquat and ACCase-inhibiting herbicides in Italian ryegrass populations from California: confirmation and mechanisms of resistance. Pest Manage. Sci. 74, 868–877. doi: 10.1002/ps.4774
Thelen J. J., Mekhedov S., Ohlrogge J. B. (2001). Brassicaceae express multiple isoforms of biotin carboxyl carrier protein in a tissue-specific manner. Plant Physiol. 125, 2016–2028. doi: 10.1104/pp.125.4.2016
Travlos I., Gazoulis I., Kanatas P., Tsekoura A., Zannopoulos S., Papastylianou P. (2020). Key factors affecting weed seeds’ germination, weed emergence, and their possible role for the efficacy of false seedbed technique as weed management practice. Front. Agron. 2. doi: 10.3389/fagro.2020.00001
Umetsu N., Shirai Y. (2020). Development of novel pesticides in the 21st century. J. Pesticide Sci. 45, 54–74. doi: 10.1584/jpestics.D20-201
Vázquez-García J. G., Torra J., Palma-Bautista C., Alcántara-de la Cruz R., Prado R. D. (2021). Point mutations and cytochrome P450 can contribute to resistance to ACCase-inhibiting herbicides in three Phalaris species. Plants 10, 1703. doi: 10.3390/plants10081703
Wurtele E. S., Nikolau B. J. (1990). Plants contain multiple biotin enzymes: discovery of 3-methylcrotonyl-CoA carboxylase, propionyl-CoA carboxylase and pyruvate carboxylase in the plant kingdom. Arch. Biochem. Biophys. 278, 179–186. doi: 10.1016/0003-9861(90)90246-u
Xia X., Tang W., He S., Kang J., Ma H., Li J. (2016). Mechanism of metamifop inhibition of the carboxyltransferase domain of acetyl-coenzyme a carboxylase in Echinochloa crus-galli. Sci. Rep. 6, 1–10. doi: 10.1038/srep34066
Xiang S., Callaghan M. M., Watson K. G., Tong L. (2009). A different mechanism for the inhibition of the carboxyltransferase domain of acetyl-coenzyme a carboxylase by tepraloxydim. Proc. Natl. Acad. Sci. 106, 20723–20727. doi: 10.1073/pnas.0908431106
Yadav A., Sirohi R. M., Chauhan B. S., Bellinder R., Malik R. K. (2002). Alarming contamination of wheat produce with resistant Phalaris minor. Pestology 26, 41–44.
Yu Q., Collavo A., Zheng M.-Q., Owen M., Sattin M., Powles S. B. (2007). Diversity of acetyl-coenzyme a carboxylase mutations in resistant Lolium populations: evaluation using clethodim. Plant Physiol. 145, 547–558. doi: 10.1104/pp.107.105262
Yu Q., Han H., Powles S. B. (2008). Mutations of the ALS gene endowing resistance to ALS-inhibiting herbicides in Lolium rigidum populations. Pest Manage. Science: formerly Pesticide Sci. 64, 1229–1236. doi: 10.1002/ps.1624
Zagnitko O., Jelenska J., Tevzadze G., Haselkorn R., Gornicki P. (2001). An isoleucine/leucine residue in the carboxyltransferase domain of acetyl-CoA carboxylase is critical for interaction with aryloxyphenoxypropionate and cyclohexanedione inhibitors. Proc. Natl. Acad. Sci. 98, 6617–6622. doi: 10.1073/pnas.121172798
Zhang X.-Q., Powles S. B. (2006). The molecular bases for resistance to acetyl co-enzyme a carboxylase (ACCase) inhibiting herbicides in two target-based resistant biotypes of annual ryegrass (Lolium rigidum). Planta 223, 550–557. doi: 10.1007/s00425-005-0095-x
Zhang H., Tweel B., Tong L. (2004). Molecular basis for the inhibition of the carboxyltransferase domain of acetyl-coenzyme-A carboxylase by haloxyfop and diclofop. Proc. Natl. Acad. Sci. 101, 5910–5915. doi: 10.1073/pnas.0400891101
Zhao Y., Ye F., Fu Y. (2023). Research progress on the action mechanism of herbicide safeners: a review. J. Agric. Food Chem. 71, 3639–3650. doi: 10.1021/acs.jafc.2c08815
Keywords: acetyl-CoA carboxylase (ACCase), carboxyl transferase (CT) domain, mutation, herbicide resistance, rational drug discovery, safeners, aryloxyphenoxypropionates, cyclohexanediones
Citation: Rajak BK, Rani P, Mandal P, Chhokar RS, Singh N and Singh DV (2023) Emerging possibilities in the advancement of herbicides to combat acetyl-CoA carboxylase inhibitor resistance. Front. Agron. 5:1218824. doi: 10.3389/fagro.2023.1218824
Received: 10 May 2023; Accepted: 27 June 2023;
Published: 19 July 2023.
Edited by:
Ricardo Alcántara-de la Cruz, Federal University of São Carlos, BrazilReviewed by:
Kassio Ferreira Mendes, Federal University of Viçosa, BrazilVeronica Hoyos, University of Magdalena, Colombia
Copyright © 2023 Rajak, Rani, Mandal, Chhokar, Singh and Singh. This is an open-access article distributed under the terms of the Creative Commons Attribution License (CC BY). The use, distribution or reproduction in other forums is permitted, provided the original author(s) and the copyright owner(s) are credited and that the original publication in this journal is cited, in accordance with accepted academic practice. No use, distribution or reproduction is permitted which does not comply with these terms.
*Correspondence: Durg Vijay Singh, ZHZiaW90ZWNoQGdtYWlsLmNvbQ==; ZHVyZ3ZpamF5c2luZ2hAY3ViLmFjLmlu