- Laboratorio de Entomología Experimental-Grupo de Investigación en Ecofisiología de Parasitoides y otros insectos (LEE-GIEP), Instituto de Ecología, Genética y Evolución (IEGEBA-CONICET/UBA), Departamento de Ecología, Genética y Evolución, Facultad de Ciencias Exactas y Naturales, Universidad de Buenos Aires, Buenos Aires, Argentina
The behavioral response of a parasitoid shows the effect on host parasitism patterns at a given host distribution. As a result, an increase or decrease in parasitism intensity according to local host densities is found. This relationship could be proportional, positive, or negative, as a consequence of host foraging. Mallophora ruficauda is a parasitoid fly that parasitizes Cyclocephala signaticollis scarab beetle larvae. Females search and place egg clusters overground in open grasslands. Larvae actively search hosts underground following chemical cues arising from the host itself. The parasitism patterns are a result of this complex host-searching strategy that is shared between both stages of the fly. In this work, we carried out a study at four spatial scales in apiaries located in the Pampas region of Argentina. We aimed to assess the potential of M. ruficauda as a control agent of white grubs. We found that parasitism has an inverse density-dependent in relation to low female activity and a direct density-dependent in relation to high female activity at the larger spatial scale. We found an inverse density-dependent pattern at the intermediate spatial scale. Parasitism is inversely density-dependent at both smaller spatial scales, associated with oviposition substrate availability and distance. Additionally, M. ruficauda does not select the oviposition substrates according to the abundance of C. signaticollis inhabiting underground. We determined that M. ruficauda could act as a natural biological controller of C. signaticollis. This work shows the importance of a proper scale for the analysis of factors that influence population dynamics of entomophagous insects and for evaluating their potential as biological control agents, and how environmental characteristics mold the parasitism patterns of this dipteran parasitoid.
1 Introduction
The term behavioral response of a parasitoid has been defined by Hassell (1966) as a behavioral response to the distribution of hosts that, being distributed unevenly, results in an increase or decrease of parasitism intensity according to local host densities. Hence, studying different dynamics of host–parasitoid systems has been the subject of great interest (Walde and Murdoch, 1988; Gunton and Pöyry, 2016). In particular, mechanisms leading to stability are useful not only from a theoretical view but also in applied science given the importance of parasitoids as biological control agents (Waage and Hassell, 1982; Bernstein, 1987; Fernández-arhex and Corley, 2003; Jervis, 2005). Spatial density dependence and host specificity are important factors for stability through their influence on the functional response of parasitoids and density dependence (Bernstein, 1987; Hassell, 2000).
Spatial density dependence in parasitism is the outcome of parasitoids’ response to differences in host density among patches leading to changes in the intensity of parasitism (Walde and Murdoch, 1988). Past work has shown that spatial density-dependent parasitism plays a role in population persistence and stability (May et al., 1981; Murdoch et al., 1984; Murdoch et al., 1985; Hassell and May, 1988; Murdoch and Stewart-Oaten, 1989; Murdoch et al., 1992; Godfray and Pacala, 1992; Murdoch and Briggs, 1996; Teder et al., 2000; Murdoch et al., 2005). Direct density dependence occurs when parasitoids aggregate as a response to high host densities, increasing the percentage of parasitism. On the contrary, if the parasitism percentage decreases with increasing host abundance, inverse density dependence occurs. Many studies show the relation between parasitism and host abundance and examples of direct, inverse, or independent density dependence have been found (Stiling, 1987; Walde and Murdoch, 1988; Gunton and Pöyry, 2016). In a leading paper, Heads and Lawton (1983) noted the importance of spatial scale in this process. Expected patterns of prey mortality imposed by a population of natural enemies aggregating in response to victim densities can vary from exponential curves, when the sample area is smaller than a “patch”, to an increasing relationship when the “patch” size is recognized by the natural enemy (Heads and Lawton, 1983).
In a more recent update, Gunton and Pöyry (2016) introduced the “scale-specific foraging hypothesis”, implying that the nature of observed correlations between local host densities and parasitism rates is the result of the methodological artifacts of the observational scales used. They propose that parasitoids discriminate among host patches according to their density at a “foraging grain size” that normally creates a positive density–parasitism relationship. However, this relationship will be detected as long as the study size units within which the densities are calculated are comparable to the foraging grain size.
Other important influential factors of spatial density dependence can be summarized in host characteristics. For instance, host distribution can exert a large influence on parasitoids’ response size where optimal strategies can vary for highly aggregated or randomly distributed hosts (Walde and Murdoch, 1988). Within hosts’ characteristics, exotic hosts and large-bodied parasitoids seem to be associated with negative parasitism responses (Gunton and Pöyry, 2016). Although there are many theoretical approaches and experimental studies trying to shed light on the effects and mechanisms of spatial density dependence on population dynamics, multi-scale studies are still needed. It is even more true if we consider that most of the studies (~85%) refer to hymenopteran parasitoids that differ greatly in the searching and oviposition strategies from dipteran parasitoids (Godfray, 1994; Feener and Brown, 1997; Gunton and Pöyry, 2016).
In this work, we analyze spatial density dependence parasitism in a dipteran parasitoid and its coleopteran host. Mallophora ruficauda Wiedemann 1828 (Diptera: Asilidae) is a pestiferous robber fly common in the open grasslands of the Pampas region of Argentina. Adults are predators of honey bees and other insects and larvae are solitary koinobiont ectoparasitoids of the second and third instar of scarab beetle larvae Cyclocephala signaticollis Burmeister 1847 (Coleoptera, Scarabaeidae) (Castelo and Capurro, 2000; Castelo and Corley, 2010; Crespo and Castelo, 2010). Mated M. ruficauda females deposit eggs in clusters that are placed at some distance from the host on elevated places, typically tall grasses, or artificial supports higher than 1.25 m, in areas close to beehives (Castelo and Corley, 2004; Castelo and Corley, 2010). Emerging robber fly larvae are wind dispersed, drop to the soil from the oviposition site, and bury themselves searching for hosts (Castelo and Lazzari, 2004; Castelo et al., 2006; Crespo and Castelo, 2008). It has been established that the selection of oviposition height by the M. ruficauda female contributes to larval dispersal and, as a result, the parasitism success is maximal when egg clusters are placed on substrates between 1.25 and 1.50 m in height (Castelo et al., 2006).
Hosts, commonly named soil white grubs, are phytophagous beetle larvae that live underground and produce damage to the roots of several plants (Potter, 1998; Carrasco et al., 2011). They complete most of their life cycle feeding in the soil. These larvae cause extensive damage to plant roots by the action of their mandibles, which is why they are considered important commercial crop pests and green recreation areas in different parts of the world (Ali Harivandi, 1987; Crutchfield and Potter, 1995; Smitley, 1996; Salvadori, 1997; Potter, 1998; Cranshaw, 1998). In Argentina, the most affected crops are cereals, forage plants (pastures), and vegetables (Remedi de Gavotto, 1964; Alvarado, 1980). These larvae are especially harmful to potato cultivars in the southeast part of the province of Buenos Aires (Remedi de Gavotto, 1964; Álvarez Castillo et al., 1993; Carmona et al., 1994; López et al., 1994). Nine species of white grubs have been detected in the province of Buenos Aires (Alvarado, 1980) in sympatry with M. ruficauda.
Regarding host location, this parasitoid has a split strategy in which both females and larvae are involved. Firstly, the female places its eggs on a tall substrate as mentioned before. After being wind dispersed, the first larval instar molts to the next instar and then active host searching occurs. In order to find its host, M. ruficauda larva orientates to its host through the detection of cues produced in the host’s posterior intestine (Castelo and Lazzari, 2004; Crespo and Castelo, 2008; Groba and Castelo, 2012). Larvae of M. ruficauda can discriminate among hosts from different instars, parasitism status, and species (Crespo and Castelo, 2008; Crespo et al., 2015; Martínez et al., 2017). Given the split strategy in this system involved in the host location, the second instar larva can be claimed to be the ecological equivalent of females in hymenopteran parasitoids. Hence, it is justified to ask if spatial density dependence of parasitism exists in this system.
Two previous analyses on this host–parasitoid system have shown contradictory results when analyzing spatial density dependence at a large spatial scale, but both were consistent in finding an inverse density dependence pattern at the smaller spatial scale (Castelo and Capurro, 2000; Castelo and Corley, 2010). One of them found evidence of direct density dependence at a spatial scale compatible with adult patches (Castelo and Capurro, 2000) while the other study, which included increased sample sites, found no relation at the larger spatial scale (Castelo and Corley, 2010). Both studies were performed, including all the potential host species that make up the community of rizophagous Scarabaeidae larvae in the study area, introducing a potential confounding factor because M. ruficauda has a marked preference for C. signaticollis larvae (Castelo and Crespo, 2012). This present work is motivated to clarify the results found in previous studies with, firstly, the addition of more sample sites and, secondly, much more information on host specificity in this species and other ecological features regarding oviposition height and distance to the hosts, substrate availability, and activity of females. Thus, the goal of this work is to show the results of our studies on the spatial density dependence of parasitism by M. ruficauda on its preferred host, C. signaticollis, at different spatial scales, and evaluate the M. ruficauda potential as a biological control agent of white grubs. We also show how the inclusion of information from habitat use by females and host use by larvae combine to properly determine the spatial density patterns at different spatial scales.
2 Materials and methods
2.1 Field sampling methods
Field studies of host abundance and parasitism were carried out in six geographical localities of the Pampas region of Argentina: General Rodríguez, Luján, Mercedes, Moreno, Pigüé, Pilar, and Victoria. Sampling was done from June to August between 1997 and 2006. These localities are within the major beekeeping region of Argentina, where adult robber flies feed mainly on honeybees (Figure 1).
The study was carried out in 24 apiaries where robber fly activity was registered in the previous summer. Some fields were sampled repeatedly in different years, so the combination apiary/year was defined as the scale “site” (see Table 1). In each site, three plots with different agricultural or cattle breeding management practices and vegetation (defined from now on as the “sub-site” scale) were sampled. Sites sampled in 2006 consisted only of one plot because it was not possible to sample three different plots in those locations. On each sub-site, a grid was placed next to the wire fence. Grids were made up of 10 lines of five samples parallel to the wire fence (“Line level”). Samples were taken every 2.5 m within each line. Lines were placed every 5 m, covering a total of 50 m. Each sample (small scale) consisted of the extraction of a soil block of 0.35 m wide by 0.30 m depth obtained with a shovel (volume: 36.8 L; surface area: 0.12 m2). In sum, samples were grouped in lines (five samples per line) with 10 lines per sub-site, obtaining 50 samples in total. The largest scale, “site”, consisted of three sub-sites, hence 150 samples, except for samples taken in 2006 that consisted of only one sub-site per site (Figure 2).

Table 1 Sites, apiaries, years, and localities where sites were found, during the fieldwork to determine the parasitism pattern of parasitoids.
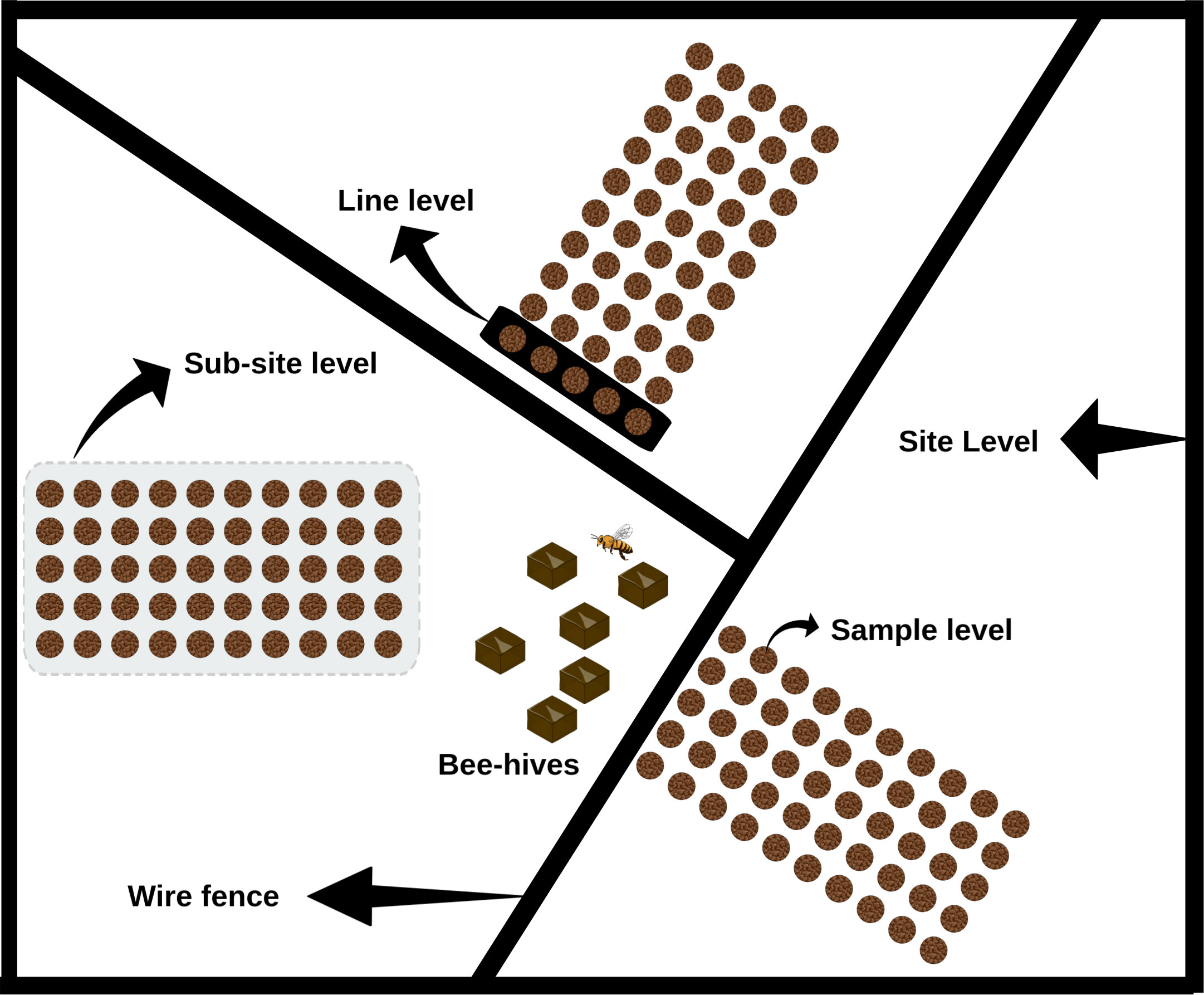
Figure 2 Schematics of hosts and parasitoid sampling showing the different scales considered in the analyses.
From each block of soil, all scarab beetle larvae were collected by manually breaking the soil and identified at the species level in the laboratory with a dichotomic key (Alvarado, 1980). A stereomicroscope was used to register the number of larvae of M. ruficauda attached to the host cuticle. Only C. signaticollis larvae were counted as host abundance since they are the preferred host for M. ruficauda (Crespo and Castelo, 2010; Barrantes and Castelo, 2014).
The different scales were chosen because we believe they represent the true complexity of this system. As mentioned before, M. ruficauda adults belong to a genus of robust dipterans that feed on other flying insects like honey bees (Bromley, 1930; Cole, 1964; Rabinovich and Corley, 1997). Asilids have an important flying capacity, nearly 1–2 km, easily covering a site area (Kanmiya, 2002; Londt, 2020). Once the female places its eggs on the substrate, larvae will be wind dispersed, so the sub-site together with the line levels capture mainly the influence of wind on larval dispersal and a possible effect of distance to the oviposition site but no further influence of females. Finally, the sample scale captures host-searching performed by the larva itself after dropping and burying into the soil.
2.2 Density dependence analysis
We considered in the analysis only sites where parasitized scarab beetle larvae were found (n = 24). We carried out sampling in different years because parasitoids move freely and frequently among localities as a consequence of the host population dynamics and food availability. C. signaticollis larvae abundance might be very variable over the years due to different causes (crop management, field conditions, and parasitism outcome itself), introducing variability in the presence of M. ruficauda and parasitism levels at a given site. Due to this scenario, it was necessary to redefine sampling places every year.
For each of the scales analyzed, (i) site (apiaries), (ii) sub-sites (field lots), (iii) lines (transects within field lots), and (iv) samples (unitary block of soil), the proportion of parasitized hosts was calculated as the ratio between the number of parasitized C. signaticollis and the total number of C. signaticollis found. To avoid overestimating the proportion of hosts bearing no parasitoids, those sites with 0% parasitism were excluded from analysis, assuming that parasitoid larvae may have not arrived in the soil in these places or adult parasitoids did not oviposit in those specific places the previous summer.
We analyzed the proportion of parasitized C. signaticollis through generalized linear models. For each scale, we generated a model that included different predictors informative of that scale (site, sub-site, line, and sample models). All models were generated with a binomial distribution and a logit link function. After modeling a full model, model selection was performed. For every model, the effect of dropping an interaction or a predictor variable (with drop1 function) was evaluated through the likelihood-ratio test and the AIC value. After obtaining the minimal model, significant terms were evaluated with the anova function. Finally, interaction plots of the estimated marginal means were done to explore the relation between the predictors and the response variable.
For the site model, host abundance and the number of egg clusters in place were used as predictor variables. Host abundance was included as a discrete predictor variable while the number of egg clusters was included as a categorical predictor variable with two levels (low and high). Egg clusters were included as a predictor variable since the abundance of M. ruficauda cannot be directly calculated.
The sub-site full model was constructed with host abundance as a discrete predictor variable and vegetation height as a categorical predictor variable (low or high). Vegetation height is an indicator of oviposition substrate availability for M. ruficauda (Castelo and Corley 2004). In fields with low vegetation height, only wire fences are available for oviposition while many other oviposition substrates (e.g., tall grasses, stems, and sticks) are also available in fields with high vegetation. If only wire fences are available for oviposition, egg-cluster aggregation could occur as a result of the availability of oviposition substrates. However, egg-cluster aggregation could still occur in vegetation if females are attracted to odors from damaged plants, hosts, or other egg clusters favoring oviposition in specific substrates. Attraction to damaged plants has already been discarded since oviposition on dry plants and wire fences is frequent (Castelo et al., 2006). In order to discard female attraction to host odors, we studied if female M. ruficauda places more egg clusters on plants and wire fences associated with hosts at a small scale. For this, we registered the position of between 28 and 35 plants or wire fences with egg clusters, in six apiaries with the proven presence of M. ruficauda in the previous summer. From each plant and wire fence, the total number of egg clusters placed by M. ruficauda females was registered. Wire fences were 2-m portions of longitudinal wire randomly chosen. Plants’ geographic positions were registered because the following step of the study was performed during autumn and many plants were gone by then. Hence, in autumn, soil samples were obtained using the same technique as previously described. Soil samples from previous plant positions were taken using the geographic position as the center. For samples from wire fences, soil samples were obtained from the midpoint of the wire longitude. Soil samples were analyzed to quantify the number of larvae of C. signaticollis present. With data from the number of egg clusters and the number of hosts, a model was constructed with the former as the response variable and the latter as a discrete numerical explanatory variable. The influence of the number of C. signaticollis hosts on the number of egg clusters was evaluated with a GLM with a Gamma distribution and log link function with the ID of each site as a random factor and the sub-site nested to the ID.
Next, the line model included the same predictor variables as the sub-site model (host abundance and vegetation height) and distance to the wire fence as another discrete predictor variable. This variable accounted for any distance effect that could be introduced in fields with low vegetation height. As random factors, we included the specific distance from where the sample was taken nested to the sub-site. In turn, the sub-site was nested to the ID as in the previous model.
Finally, the sample model included the same predictor variables as the line model (host abundance, vegetation height, and distance to the wire fence). We assumed that dependency on sites and sub-sites would not introduce a negative effect on the results since females are not able to place two egg clusters in a single day (M. Castelo, personal observation). Given the fact that at maximum only three sub-sites per site could be included as random effects, they were not included as random variables because at least five replicates are suggested to estimate variance. As random factors, we included the specific distance and line from where the sample was taken nested to the sub-site. In turn, the sub-site was nested to the ID as in the previous models.
All the statistical analyses were performed using R version 3.6.3 “Holding the Windsock” (R Core Team, 2020). Models were performed using the function glmmTMB from the glmmTMB package (Brooks et al., 2017). Interaction plots of the estimated margin means were performed using the emmip function of the emmeans library (Lenth et al., 2020). Plots were done using the ggplot2 library (Wickham et al., 2020).
3 Results
We observed C. signaticollis hosts parasitized by M. ruficauda in 24 out of 36 sampled sites. For the larger part of the soil samples, no hosts were found (n = 3,537). We collected 1,113 C. signaticollis, of which 192 were parasitized by M. ruficauda (~17.25% of parasitism).
The four models showed information that can be separated into three (site model, sub-site model, and line-sample models together). For every model, we found support for either direct or inverse density dependence.
For the site model, we found that the relationship between the proportion of parasitism and the number of hosts depended on the estimated abundance of M. ruficauda through the abundance of egg clusters found. In sites where the estimated abundance of M. ruficauda was high, we found direct density dependence. On the contrary, in sites where the estimated abundance of M. ruficauda was low, an inverse density dependence relation was found (Figure 3A, Table 2).
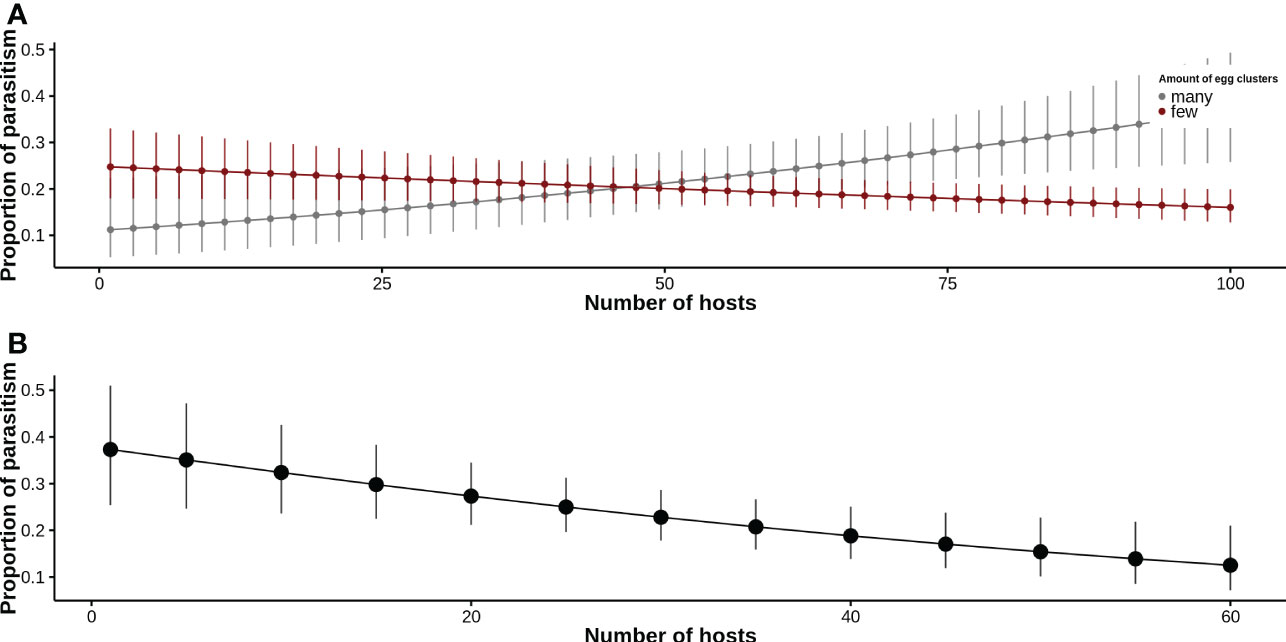
Figure 3 The expected proportion of parasitism related to the number of hosts. The fit was made with generalized linear models assuming a binomial distribution and a logit link function. (A) Site model. (B) Sub-site model.
For the sub-site model, we found an inverse density-dependence relationship between the proportion of parasitism and the number of C. signaticollis hosts (Figure 3B, Table 3). We also found no relation between the abundance of C. signaticollis hosts and the number of egg clusters in substrates, indicating that oviposition is not related to plants and wire fences associated with hosts at a small scale (Chisq3 = 1.936, p = 0.586).
For the line and sample models, we found an inverse density-dependence relationship between the proportion of parasitism and the number of C. signaticollis hosts but its intensity depended on the vegetation height and the distance to the wire fence. In both models, we found that the decrease in the proportion of parasitism was steeper in the closest 6–8 m to the wire fence in sites with low vegetation than those with high vegetation (Figures 4A–D, Tables 4, 5). For example, when there was only one host, the proportion of parasitism was higher closest to the wire fence than at 6 m, but this effect is lost as the number of hosts increases (Figures 4B, D).
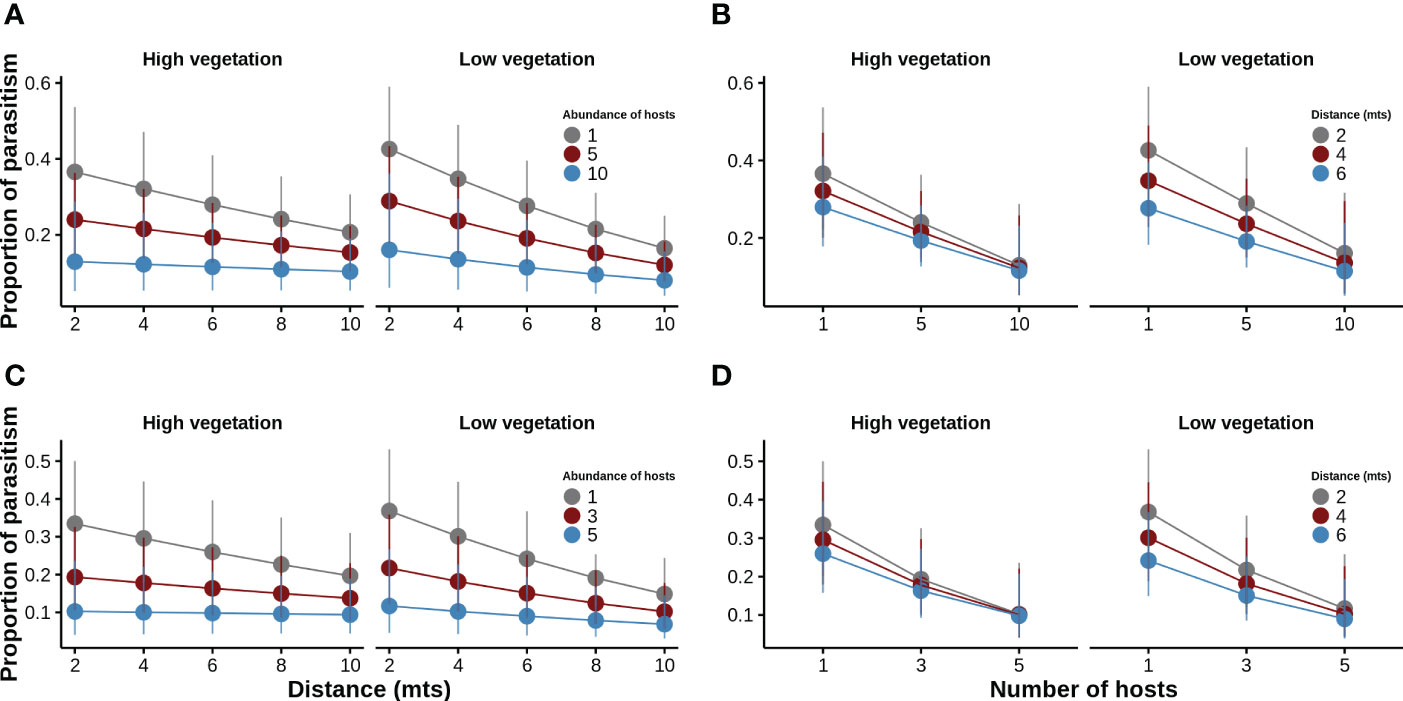
Figure 4 The expected proportion of parasitism generated with a generalized linear model assuming a binomial distribution and a logit link function. (A) line model, where the expected proportion of parasitism against distance for different host abundance is shown. (B) line model, showing the expected proportion of parasitism against the number of hosts at different distances. (C) sample model, where the expected proportion of parasitism against distance for different host abundance is shown. (D) sample model, showing the expected proportion of parasitism against the number of hosts at different distances.
4 Discussion
In this work, we show the results of our studies on spatial density-dependent parasitism by the robber fly M. ruficauda on C. signaticollis scarab beetle larvae at different spatial scales. We found two different scenarios. At large spatial scales, either direct density dependence or inverse density dependence was found. At smaller spatial scales, only inverse density dependence was found. The patterns we found in this system might be related to the two-step stages involved in host searching. In these kinds of parasitoids [dipteran, coleopteran, lepidopteran, neuropteran, strepsipteran, and trichopteran (Mills, 2009);] where the female cannot directly access the concealed host, females place their eggs close to hosts, and it is the larva that must locate the host (Godfray, 1994; Feener and Brown, 1997).
The spatial scale of analysis is central in determining parasitism patterns and natural enemy efficiency since the variations may be accounted for by different behavioral processes dominating each scale (Heads and Lawton, 1983; Walde and Murdoch, 1988). There are studies showing a switch in parasitism patterns as a result of changes in host searching behavior when parasitoids use different cues at different spatial scales (Jarošík and Lapchin, 2013), or as a result of demographic processes like the aggregation of parasitoids on natural vegetation near crops or the promotion of high female fecundities (Segoli, 2016; Morgan et al., 2017).
At large spatial scales, generalist adult parasitoids can produce a density-independent pattern of parasitism when parasitism rates vary among sites as a consequence of local variable abiotic factors. As host density can be variable, foraging parasitoids may not be able to distinguish infested areas with high host density. However, when the sample area is bigger than the effective patch, then density dependence patterns might be lost (Heads and Lawton, 1983). In M. ruficauda, we observed inverse density-dependent parasitism when the abundance of adults was low and a direct density dependence pattern was observed when the adult abundance was high. This result lends support to both previous studies that found contradictory results (Castelo and Capurro, 2000; Castelo and Corley, 2010). Although previous studies found support for opposed density-dependent patterns, both studies included, as potential hosts, larvae of other white grub species than C. signaticollis because it was thought that M. ruficauda could develop on several Scarabaeidae host species. However, it has been already shown that M. ruficauda can only develop to imago on C. signaticollis as its host albeit positive orientation toward other Cyclocephala species occurs (Barrantes and Castelo, 2014). Also, previous studies did not include information regarding environmental factors, such as vegetation or wire fences’ height, distance to the wire fences, or adult activity. This information, as shown through our analysis, proved relevant for M. ruficauda given its split host location strategy.
Another important difference that might influence the results found at the site scale could be related to the size of the site area that included fields with many different characteristics. The Pampas region where M. ruficauda is typically found has been highly modified for livestock and agricultural purposes. Site scale, therefore, includes fields with crops and livestock culture that introduces high variability. The abundance of hosts in fields with livestock culture or soybean crops is much lower than the abundance in cereal, potato crops, or sunflower cultivars. Another possible explanation for the difference found with previous studies at site scale might be that Asilidae adults are territorial (Onsager and Rees, 1985; Lavigne and Bullington, 2001; Weber and Lavigne, 2004; Haab et al., 2019).
At this large spatial scale, we found that when the number of hosts is relatively low (less than 50), the proportion of parasitism is lower when there are many egg clusters compared to sites with few egg clusters. This could be because a particular type of competition occurs in this species since oviposition often occurs on substrates where a previous egg cluster had been placed by another female (M. Castelo, personal observation). This particular behavior could introduce competition where not all larvae from egg clusters are able to reach a host or several larvae could reach the same host incurring an inefficient superparasitism. However, as more hosts become available, many more larvae can parasitize a host.
The results so far could indicate that female M. ruficauda could lay eggs in environments with high host density and may have some skill to qualify environments according to host abundance, supporting conclusions drawn in previous studies (Castelo and Capurro, 2000). However, Castelo and Corley’s (2010) study included a larger dataset and found density-independent parasitism at the site scale. In this work, where we included more relevant environmental factors and clarified which white grubs species are real hosts for M. ruficauda, we found that in sites with a high abundance of females, an important control on the host could occur. This highlights the importance of adequate sampling (replication and spatial scale) in detecting true density dependence and natural enemy efficiency to control pestiferous host species.
We then analyzed the sub-site scale that matches the surface of fields with a homogeneous type of land use. At this spatial scale, we found an inverse density pattern. This result is in line with what was found by Castelo and Corley (2010). This pattern could be a result of a limited detection threshold of larvae and a reduced number of parasitoids compared to hosts. M. ruficauda females select the higher available substrates maximizing larval dispersal and minimizing superparasitism (Castelo et al., 2006). Since larvae are dispersed by the wind, the distance traveled by the larvae will depend on environmental conditions like wind velocity and the height of the oviposition site. Once larvae land in the soil, they bury themselves and, after molting, start searching for their hosts orienting to host chemical cues (Castelo and Lazzari, 2004; Crespo and Castelo, 2008). This type of anemophilic dispersal imposes dispersion limits into this system, generating a top limit in the number of parasitoid larvae that can land in any specific spot. Given also that C. signaticollis hosts do not distribute randomly but tend to aggregate in space and are also attracted by the same chemical cues used by M. ruficauda. The resulting spatial pattern is inversely dependent. The spatial distribution of small particles that are wind dispersed in a heterogeneous habitat has been successfully used in modeling the spatial distribution of individuals from different species in forests (Shen et al., 2009). In this work, the authors showed that both dispersal limitation and habitat heterogeneity were important factors in determining the spatial distribution of propagules. Also, soil factors could affect the risk of parasitism because of differences in host accessibility, such as the depth to which hosts are buried underground in a patch (Okuyama, 2019). The idea that larval dispersion in M. ruficauda can be modeled as a seed dispersion phenomenon has already been explored with results that explained the oviposition strategy in this species (Castelo et al., 2006). Hence, an inverse density dependence at small spatial scales could indicate that dispersal limitation in a heterogeneous habitat is the driving process.
At smaller spatial scales, we found the same result as in previous studies, i.e., inverse density dependence. However, we found that the magnitude of the proportion of parasitism was different in plots with high or low vegetation and that differences tend to disappear as the distance to the wire fence increases. At both smaller spatial scales, the same pattern was found showing that habitat heterogeneity is important and can influence the proportion of parasitism. In fact, it is very frequent that plots where agriculture is practiced show low vegetation. Also, as the distance to the wire fence increases, the higher the chance to find spots treated with pesticides and herbicides.
An important conclusion of our work indicates that M. ruficauda could actually be considered of importance as a good agent to control C. signaticollis populations as opposed to suggestions previously made considering several species of Scarabaeidae larvae (Castelo and Corley, 2010). These results reinstate the discussion that actions against M. ruficauda to lessen their impact on beekeeping (e.g., egg cluster removal and adult mortality) may bring consequences for the population dynamics of C. signaticollis. The relative effect of M. ruficauda as a biological control agent against C. signaticollis should be dependent on the female oviposition site selection. Hence, it is important to understand if females place more egg clusters in substrates associated with more hosts underground that could be releasing attractive chemical cues. However, we found no relation between the number of egg clusters in a specific plant and the abundance of C. signaticollis at the plant scale. This result reinforces the idea that M. ruficauda does not use host-related cues associated with plants or wire fences. In fact, M. ruficauda females use any types of substrates, for example, alive plants, dead plants, or abiotic supports, mainly based on their height, which should minimize superparasitism and increase singly parasitism as has been already proposed (Castelo et al., 2006). However, we have frequently registered egg clusters clumped on the same plant in the field (M. Castelo, personal observation). It is unknown if egg-cluster aggregation poses some adaptive advantage for females during oviposition site seeking or if the females use some other characteristic of substrates indicative of good habitat quality. These characteristics could be other relevant factors related to their life cycles like host densities at different spatial scales, prey abundance, or even human-related activities like agricultural management.
Our main conclusion is that parasitoid–host systems with complex host searching strategies also show different parasitism patterns at different spatial scales. In fact, according to the “scale-specific foraging hypothesis” of Gunton and Pöyry (2016), the nature of the observed correlations is strongly affected by the observational spatial scale and supports the idea that parasitoids discriminate among host patches according to their density at a “foraging grain size”. However, when studying parasitism patterns and the influence of density dependence, it is important to analyze the potential effects of ecological factors such as dispersion limitation or habitat heterogeneity that have important roles in shaping complex host–parasitoid population dynamics and the subsequent implications of these processes on the efficiency of natural enemies as pest control agents.
Data availability statement
The datasets presented in this study can be found in online repositories. The names of the repository/repositories and accession number(s) can be found below: https://doi.org/10.7910/DVN/C7617E.
Author contributions
MC conceived and designed the experiments. MC and JC performed the experiments. JC analyzed the data. MC and JC wrote the manuscript. All authors contributed to the article and approved the submitted version
Funding
This work was supported by the Consejo Nacional de Investigaciones Científicas y Técnicas (grant number PIP 0368CO), Agencia Nacional de Promoción de la Investigación, el Desarrollo Tecnológico y la Innovación (grant number FONCYT-PICT-2019-00975), and Universidad de Buenos Aires (grant numbers UBACyT 0059BA, 0019BA).
Acknowledgments
The authors are indebted to Ángel Capurro and Juan Corley for their invaluable ideas and fruitful discussions.
Conflict of interest
The authors declare that the research was conducted in the absence of any commercial or financial relationships that could be construed as a potential conflict of interest.
Publisher’s note
All claims expressed in this article are solely those of the authors and do not necessarily represent those of their affiliated organizations, or those of the publisher, the editors and the reviewers. Any product that may be evaluated in this article, or claim that may be made by its manufacturer, is not guaranteed or endorsed by the publisher.
References
Ali Harivandi M. (1987). Tall fescue gaining popularity as a turfgrass (USA: California agriculture - California Agricultural Experiment Station).
Alvarado L. (1980). Sistematica bionomia coleopteros estados inmaduros viven suelo (Buenos Aires, Argentina: Universidad Nacional de la Plata).
Álvarez Castillo H. A., López A. N., Vincini A. M., Carmona D., Manetti P. L. (1993). Relevamiento de los insectos del suelo en cultivos de papa del sudeste bonaerense. Boletín. técnico) 118.
Barrantes M. E., Castelo M. K. (2014). Host specificity in the host-seeking larva of the dipteran parasitoid Mallophora ruficauda and the influence of age on parasitism decisions. Bull. Entomol. Res. 104 (3), 295–306. doi: 10.1017/S0007485314000029
Bernstein C. (1987). On assessing the role of spatially-heterogeneous density-independent host mortality on the stability of host-parasitoid systems. Oikos 49 (2), 236–239. doi: 10.2307/3566033
Brooks M. E., Kristensen K., van Benthem K. J., Magnusson A., Berg C. W., Nielsen A., et al. (2017). glmmTMB balances speed and flexibility among packages for zero-inflated generalized linear mixed modeling. R. J. 9 (2), 378–400. doi: 10.32614/RJ-2017-066
Carmona D., Vincini A. M., López A. N., Castillo H. A., Manetti P. L. (1994). Cambios estacionales en la comunidad de «insectos del suelo» en el cultivo de papa en el sudeste bonaerense Vol. 126 (Buenos Aires, Argentina: SAGP-INTA, CERBAS, EEA Balcarce, Informe Técnico), 15.
Carrasco N., Báez A., Belmonte M. L. (2011). Trigo. manual de campo (Buenos Aires, Argentina: INTA).
Castelo M. K., Capurro Á. (2000). Especificidad y denso-dependencia inversa en parasitoides con oviposición fuera del hospedador: el caso de Mallophora ruficauda (Diptera: Asilidae) en la pampa Argentina. Ecol. Aust. 10 (2), 89–101.
Castelo M.K., Corley J. C. (2004). Oviposition behavior in the robber fly Mallophora ruficauda (Diptera: Asilidae). aesa 97 (5), 1050–1054. doi: 10.1603/0013-8746(2004)097[1050:OBITRF]2.0.CO;2
Castelo M. K., Corley J. C. (2010). Spatial density-dependent parasitism and specificity in the robber fly Mallophora ruficauda (Diptera: Asilidae). Austral Ecol. 35 (1), 72–81. doi: 10.1111/j.1442-9993.2009.02013.x
Castelo M. K., Crespo J. E. (2012). Incidence of non-immunological defenses of soil white grubs on parasitism success of Mallophora ruficauda larva (Diptera: Asilidae). Insects 3 (3), 692–708. doi: 10.3390/insects3030692
Castelo M. K., Lazzari C. R. (2004). Host-seeking behavior in larvae of the robber fly Mallophora ruficauda (Diptera: Asilidae). J. Insect Physiol. 50 (4), 331–336. doi: 10.1016/j.jinsphys.2004.02.002
Castelo M. K., Ney-Nifle M., Corley J. C., Bernstein C. (2006). Oviposition height increases parasitism success by the robber fly Mallophora ruficauda (Diptera: Asilidae). Behav. Ecol. Sociobiol. 61 (2), 231–243. doi: 10.1007/s00265-006-0254-5
Cole F. R. (1964). The genus mallophora and related asilid genera in north America. (California, USA: California University Press)
Cranshaw W. (1998). Billbugs and white grubs: Control in home lawns – 5.516. (Colorado, USA: Colorado State University).
Crespo J. E., Castelo M. K. (2008). The ontogeny of host-seeking behaviour in a parasitoid dipteran. J. Insect Physiol. 54 (5), 842–847. doi: 10.1016/j.jinsphys.2008.03.002
Crespo J. E., Castelo M. K. (2010). Life-history traits in a parasitoid dipteran species with free-living and obligate parasitic immature stages. Physiol. Entomol. 35 (2), 160–167. doi: 10.1111/j.1365-3032.2010.00727.x
Crespo J. E., Martínez G. A., Castelo M. K. (2015). Exposure to competitors influences parasitism decisions in ectoparasitoid fly larvae. Anim. Behav. 100, 38–43. doi: 10.1016/j.anbehav.2014.11.005
Crutchfield B. A., Potter D. A. (1995). Damage relationships of Japanese beetle and southern masked chafer (Coleoptera: Scarabaeidae) grubs in cool-season turfgrasses. J. Econ. Entomol. 88 (4), 1049–1056. doi: 10.1093/jee/88.4.1049
Feener D. H. Jr., Brown B. V. (1997). Diptera as parasitoids. Annu. Rev. Entomol. 42 (1), 73–97. doi: 10.1146/annurev.ento.42.1.73
Fernández-arhex V., Corley J. C. (2003). The functional response of parasitoids and its implications for biological control. Biocontrol Sci. Technol. 13 (4), 403–413. doi: 10.1080/0958315031000104523
Godfray H. C. J. (1994). Parasitoids: Behavioral and evolutionary ecology (New Jersey, USA: Princeton University Press), 488.
Godfray H. C., Pacala S. W. (1992). Aggregation and the population dynamics of parasitoids and predators. Am. Nat. 140 (1), 30–40. doi: 10.1086/285401
Groba H. F., Castelo M. K. (2012). Chemical interaction between the larva of a dipteran parasitoid and its coleopteran host: A case of exploitation of the communication system during the searching behaviour? Bull. Entomol. Res. 102 (03), 315–323. doi: 10.1017/S0007485311000691
Gunton R. M., Pöyry J. (2016). Scale-specific spatial density dependence in parasitoids: a multi-factor meta-analysis. Funct. Ecol. 30 (9), 1501–1510. doi: 10.1111/1365-2435.12627
Haab K. A., McKnight T. A., McKnight K. B. (2019). Phenology and ethology of adult Lasiopogon slossonae Cole and Wilcox robber flies (Diptera: Asilidae) in a new York riparian habitat. Proceedings of the Entomological Society of Washington. 121, 4, 594–615. doi: 10.4289/0013-8797.121.4.594
Hassell M. P. (1966). Evaluation of parasite or predator responses. J. Anim. Ecol. 35 (1), 65–75. doi: 10.2307/2690
Hassell M. P. (2000). The spatial and temporal dynamics of host-parasitoid interactions. Edición: 1 (Oxford; New York: Oxford University Press), 208.
Hassell M. P., May R. M. (1988). Spatial heterogeneity and the dynamics of parasitoid-host systems. Annales. Zool. Fennici. 25 (1), 55–61.
Heads P. A., Lawton J. H. (1983). Studies on the natural enemy complex of the holly leaf-miner: The effects of scale on the detection of aggregative responses and the implications for biological control. Oikos 40 (2), 267–276. doi: 10.2307/3544591
Jarošík V., Lapchin L. (2013). An experimental investigation of patterns of parasitism at three spatial scales in an aphid-parasitoid system (Hymenoptera: Aphidiidae). EJE 98 (3), 295–299. doi: 10.14411/eje.2001.050
Jervis M. A. (Ed.) (2005). “Insects as natural enemies,” in A practical perspective (Netherlands: Springer).
Kanmiya K. (2002). Flight properties of orthorrhaphous brachycera flies in tethered flight performance (Insecta: Diptera). Med. Entomol. Zool. 53 (Supplement2), 109–120. doi: 10.7601/mez.53.109_2
Lavigne R., Bullington S. (2001). Evidence for territoriality by males of Laphria fernaldi (Diptera: Asilidae). Studia. Dipeterol. 8, 415–421.
Lenth R., Singmann H., Love J., Buerkner P., Herve M. (2020). Emmeans: Estimated marginal means, aka least-squares means.
Londt J. G. H. (2020). A survey of grassland asilidae (Diptera) at jacana eco estate, Hilton, south Africa. Afr. Invertebrates. 61 (1), 29–48. doi: 10.3897/AfrInvertebr.61.50895
López A. N., Alvarez Castillo H. A., Carmona D., Manetti P. L., Vincini A. M. (1994). Aspectos morfológicos y biológicos de cyclocephala signaticollis Burm.(Coleoptera: Scarabaeidae) (Buenos Aires, Argentina: Boletín Técnico).
Martínez G. A., Castelo M. K., Crespo J. E. (2017). Behavioural plasticity induced by intraspecific competition in host orientation in a parasitoid. Ecol. Entomol. 42 (4), 484–491. doi: 10.1111/een.12407
May R. M., Hassell M. P., Anderson R. M., Tonkyn D. W. (1981). Density dependence in host-parasitoid models. J. Anim. Ecol. 50 (3), 855–865. doi: 10.2307/4142
Mills N. (2009). “Chapter 190 - parasitoids,” in Encyclopedia of insects, 2nd ed.Eds. Resh V. H., Cardé R. T. (San Diego: Academic Press), 748–751.
Morgan W. H., Thébault E., Seymour C. L., van Veen F. J. F. (2017). Density dependence and environmental factors affect population stability of an agricultural pest and its specialist parasitoid. BioControl 62 (2), 175–184. doi: 10.1007/s10526-016-9777-5
Murdoch W. W., Briggs C. J. (1996). Theory for biological control: Recent developments. Ecology 77 (7), 2001–2013. doi: 10.2307/2265696
Murdoch W. W., Briggs C. J., Nisbet R. M., Stewart-Oaten A. (1992). Aggregation and stability in metapopulation models. Am. Nat. 140 (1), 41–58. doi: 10.1086/285402
Murdoch W., Briggs C. J., Swarbrick S. (2005). Host suppression and stability in a parasitoid-host system: Experimental demonstration. Science 309 (5734), 610–613. doi: 10.1126/science.111442
Murdoch W. W., Chesson J., Chesson P. L. (1985). Biological control in theory and practice. Am. Nat. 125 (3), 344–366. doi: 10.1086/284347
Murdoch W. W., Reeve J. D., Huffaker C. B., Kennett C. E. (1984). Biological control of olive scale and its relevance to ecological theory. Am. Nat. 123 (3), 371–392. doi: 10.1086/284210
Murdoch W. W., Stewart-Oaten A. (1989). Aggregation by parasitoids and predators: Effects on equilibrium and stability. Am. Nat. 134 (2), 288–310. doi: 10.1086/284981
Okuyama T. (2019). Density-dependent distribution of parasitism risk among underground hosts. Bull. Entomol. Res. 109 (4), 528–533. doi: 10.1017/S0007485318000871
Onsager J. A., Rees N. E. (1985). Longevity, survival rate, and size of territory of the robber fly, Efferia bicaudata (Diptera: Asilidae), estimated by a capture-recapture method. Environ. Entomol. 14 (4), 437–440. doi: 10.1093/ee/14.4.437
Potter D. A. (1998). Destructive turfgrass insects: Biology, diagnosis, and control. Edition: 1 (Chelsea, Mich: Wiley), 344.
Rabinovich M., Corley J. (1997). An important new predator of honey bees-the robber fly Mallophora ruficauda wiedemann (Diptera, asilidae) in Argentina. Am. Bee. J. 137 (4), 303–306.
R Core Team (2020) R: A language and environment for statistical computing. Available at: https://www.r-project.org/.
Remedi de Gavotto L. (1964). Ciclo biológico de Cyclocephala signaticollis burm. (Coleoptera, scarabaeidae) y caracteres específicos de su larva. Rev. Investigaciones. Agropecuarias. 1 (10), 151–161.
Segoli M. (2016). Effects of habitat type and spatial scale on density dependent parasitism in Anagrus parasitoids of leafhopper eggs. Biol. Control. 92, 139–144. doi: 10.1016/j.biocontrol.2015.10.011
Shen G., Yu M., Hu X. S., Mi X., Ren H., Sun I. F., et al. (2009). Species–area relationships explained by the joint effects of dispersal limitation and habitat heterogeneity. Ecology 90 (11), 3033–3041. doi: 10.1890/08-1646.1
Smitley D. R. (1996). Incidence of Papillia japonica (Coleoptera: Scarabaeidae) and other scarab larvae in nursery fields. J. Econ. Entomol. 89 (5), 1262–1266. doi: 10.1093/jee/89.5.1262
Stiling P. D. (1987). The frequency of density dependence in insect host-parasitoid systems. Ecology 68 (4), 844–856. doi: 10.2307/1938356
Teder T., Tanhuanpää M., Ruohomäki K., Kaitaniemi P., Henriksson J. (2000). Temporal and spatial variation of larval parasitism in non-outbreaking populations of a folivorous moth. Oecologia 123 (4), 516–524. doi: 10.1007/s004420000346
Waage J. K., Hassell M. P. (1982). Parasitoids as biological control agents – a fundamental approach. Parasitology 84 (4), 241–268. doi: 10.1017/S003118200005366X
Walde S. J., Murdoch W. W. (1988). Spatial density dependence in parasitoids. Annu. Rev. Entomol. 33 (1), 441–466. doi: 10.1146/annurev.en.33.010188.002301
Weber G., Lavigne R. (2004). Notes on the behaviour of Blepharotes coriarius (Wiedemann, 1830) (Diptera: Asilidae) with a description of the pupal case. Studia. Dipterol. 111, 13–21.
Keywords: parasitoid, scarab-beetle larvae, Scarabaeidae, spatial scale, parasitism
Citation: Castelo MK and Crespo JE (2023) Habitats and parasitoid abundance influence spatial density dependence patterns, rendering an asilid fly as a potential biological controller of white grubs. Front. Agron. 5:1029232. doi: 10.3389/fagro.2023.1029232
Received: 26 August 2022; Accepted: 12 January 2023;
Published: 27 January 2023.
Edited by:
María Gabriela Luna, Universidad Nacional de La Plata (UNLP), ArgentinaReviewed by:
Jozsef Kiss, Szent István University, HungaryOrlando Campolo, Mediterranea University of Reggio Calabria, Italy
Copyright © 2023 Castelo and Crespo. This is an open-access article distributed under the terms of the Creative Commons Attribution License (CC BY). The use, distribution or reproduction in other forums is permitted, provided the original author(s) and the copyright owner(s) are credited and that the original publication in this journal is cited, in accordance with accepted academic practice. No use, distribution or reproduction is permitted which does not comply with these terms.
*Correspondence: Marcela K. Castelo, bWNhc3RlbG9AZWdlLmZjZW4udWJhLmFy