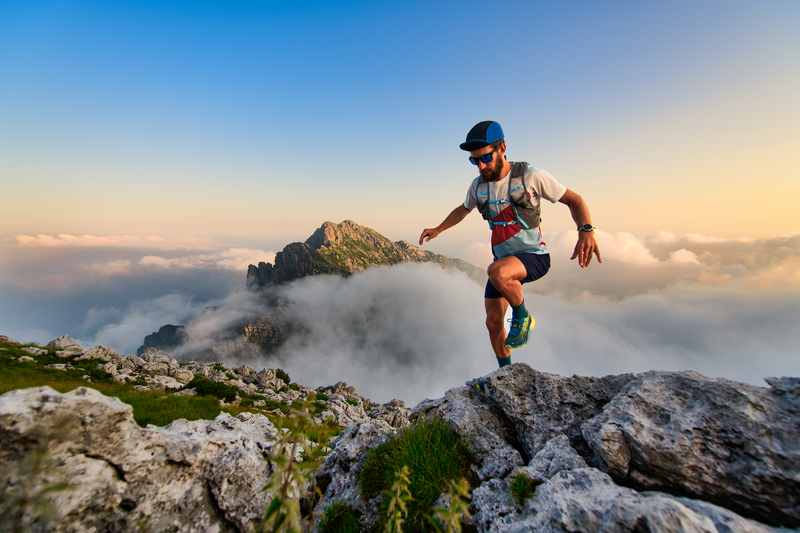
95% of researchers rate our articles as excellent or good
Learn more about the work of our research integrity team to safeguard the quality of each article we publish.
Find out more
REVIEW article
Front. Agron. , 03 October 2022
Sec. Disease Management
Volume 4 - 2022 | https://doi.org/10.3389/fagro.2022.932839
This article is part of the Research Topic Insights in Disease Management View all 6 articles
Biological control of plant pathogens has become increasingly possible with the use of fungi, which have a high reproductive rate (both sexually and asexually) and a short generation time and are very specific to their target. Trichoderma species are found in diverse habitats and experience various interactions with other organisms. They are used as bio-fungicides owing to their plant-protecting abilities, and they produce a large number of secondary metabolites (SMs) accompanied by enrichment in secondary metabolism-associated genes. This article aims to review and discuss the SMs produced by Trichoderma species, including their physiology, mode of action, mass production, and industrial and field applications for the control of plant diseases. We also discuss the evolutionary history, taxonomical gradient, classification, and ecology of Trichoderma species, as well as indirect and direct mechanisms used as plant protectors with gene improvement strategies. Aside from the bioactivity of SMs derived from Trichoderma species, compatibility with fungicides, mass formulation techniques, and industrial applications of Trichoderma species, the review focuses on its advent and progress as a global research pioneer.
The use of novel agricultural technologies has improved production, but some modern practices damage the environment. Increasing yields in an environment-friendly manner has become one of the recent challenges of advanced farming (Weller et al., 2014). There are a number of diseases that can be caused by bacteria, fungi, viruses, nematodes, and mycoplasmas in crops. Fungal pathogens are one of these plant pathogenic organisms that cause significant damage to agricultural crops around the world that reduce crop yields with an estimated loss of 15–17% during cropping and harvesting (Weller et al., 2014; Pandey et al., 2018). Mycotoxin production also results from fungal contamination of food commodities. Many approaches have been used to manage these pathogens, including cultural, mechanical, microbial biocontrol agents, and the use of resistant cultivars and chemical fungicides. The use of fungicides for the treatment of plant diseases may cause serious health and environmental effects (Suryanarayanan et al., 2016). Some of the negative effects of plant diseases on our everyday lives may go unnoticed. It is sometimes hard to get food if our crops do not succeed. Therefore, it is crucial for us to diversify our foods and develop eco-friendly agricultural technologies, so that we can grow healthier crops.
Synthetic fungicides are currently used to control plant diseases, but they play a major role in limiting the availability of nutritionally adequate and safe food (Russell, 2005). In order to ensure a sustainable production in the future, plant disease management strategies are needed (Russell, 2005; Weller et al., 2014). In contemporary agriculture systems, agrochemicals are important to reduce crop losses (Carvalho, 2006). In general, agrochemicals can be divided into fertilizers and pesticides. Nitrogen, phosphorus, and potassium are all elements found as chemical compounds in growth regulators and pesticides (Carvalho, 2006). The four main types of pesticides are insecticides, fungicides, nematicides, and herbicides. The majority of plant diseases are caused by fungi and oomycetes, and fungi can also cause chronic and acute health problems in human beings (Weller et al., 2014). For instance, in addition to causing Fusarium head blight, Fusarium graminearum also produces a mycotoxin, deoxynivalenol, that has a harmful effect to both animals and humans (Weller et al., 2014). Increasingly, widespread availability and greater efficacy of these fungicides have been attributed to increased crop productivity and combating fungal pathogens. Therefore, fungicides are essential for plant disease control (Reuveni and Reuveni, 1998).
It is believed that the first generation of fungicides was derived from the Bordeaux mixture, which was discovered in 1885, and powdery and downy mildew on grapes can be controlled with this chemical (Weller et al., 2014). Second-generation fungicides include organic chemicals, such as dithiocarbamates, and were first synthesized in 1934 (De Waard et al., 1993). These synthetic fungicides only affect plants on the surface and make no contact with the plant’s inner tissue. Organic fungicides of the third generation (1970–80) penetrated host tissues and controlled infections caused by fungal pathogens (De Waard et al., 1993). Fungicides of the fourth generation (from 1980 to the present) inhibit fungi from penetrating plant tissue, thus causing plant resistance (Russell, 2005). As a result of pesticide use, humans and ecosystems suffer adverse effects. Agrochemicals adversely affect the environment, food chain, and soil and disrupt the ecological balance in the environment (Anderson et al., 2004). In the long run, nitrogen fertilizers can contaminate ground water, and fertilizers based on ammonium can decrease pH levels and make soils more susceptible to Fusarium wilt (Carvalho, 2006). In particular, fungicides negatively affect saprobic fungi such as Penicillium species and Trichoderma species in soils (Suryanarayanan et al., 2016).
Due to frequent use of the fungicides, fungicide resistance develops, resulting in failures in controlling disease (Vinale et al., 2008; Burketova et al., 2015). Gray mold is caused by Botrytis cinerea on vegetables, fruits, and ornamental flowers. Botrytis cinerea developed resistance to benzimidazoles due to a mutation in β-tubulin, a protein-coding gene. Currently, QoI (quinol oxidation inhibitor) fungicides such as strobilurins are important fungicides and azoxystrobin is the world’s most popular fungicide, with extensive mitigation activity against many pathogens of food crops (Ishii, 2006). Nevertheless, QoI fungicides induce pathogen resistance. Melon and cucumber powdery mildew, as well as cucumber crops’ downy mildew, developed a fungicide resistance to QoI groups of fungicides in Japan (Ishii, 2006). In addition, Fraaije et al. (2005) found that Mycosphaerella graminicola, a wheat pathogen, has developed resistance to strobilurin, a Qol groups of fungicide. A range of fungicides called DMI (demethylation inhibitors; also known as sterol biosynthesis inhibitors) are deployed to combat diseases in vegetables, cereals, fruit crops, and other plantation crops (Ishii, 2006). Apple scab fungi such as Venturia inaequalis and V. nashicola have developed resistance to DMI fungicides (Ishii, 2006). The use of pesticides at higher concentrations is required to curb pesticide resistance (Tranier et al., 2014) along with other strategies such as alternation or mixing with other modes of action (Mikaberidze et al., 2014). Consequently, pesticide effectiveness decreased (Widawsky et al., 1998). Since the use of synthetic fungicides causes environmental contamination and pathogen resistance, therefore, alternative methods for battling pathogens have increasingly become important in recent years (Hasan et al., 2013). In achieving high-quality crops, non-chemical products are a critical component.
In order to achieve sustainable agriculture, fertilizers and pesticides must be reduced or eliminated (Pandey et al., 2021). Most countries have implemented regulatory measures that minimize disease control based on chemical fungicides and encourage alternative mitigation strategies (Widawsky et al., 1998). The Integrated Plant Disease Management system is an effective alternative crop management method. Sustainable agriculture is achieved through combining synthetic fungicides, organic fertilizers, biological control, better soil management, and water management (Carvalho, 2006; Suryanarayanan et al., 2016). One way to control pests and pathogens without using chemicals is to develop disease-resistant varieties of food crops (Widawsky et al., 1998). In addition to introducing disease-resistant cultivars, scientists are striving to increase yields by developing high-yielding varieties (Carvalho, 2006). Sustainable agriculture can also be achieved through organic farming. Natural bio-agrochemicals can be derived from organic debris such as phenolic compounds, flavonoids, terpenoids, alkaloids, and fatty acids (Chou, 2010). Using Trichoderma to manage crop diseases is not an exception. However, the findings of the research are scattered throughout the papers. Although, few researchers reviewed the use of Trichoderma for crop disease management. These reviews, however, were either crop specific (Olowe et al., 2022) or did not provide detailed information about the mode of action of Trichoderma in disease management (Al-Ani and Li, 2018; Meher et al., 2020) or its taxonomical and chemical characteristics (Asad, 2022). Thus, in this paper, we compiled a comprehensive review of Trichoderma species, their taxonomy, classification, and ecology, as well as indirect and direct mechanisms utilized as plant protective mechanisms. In addition, the review is focused on the compatibility, mass formulation techniques, and industrial applications of Trichoderma species more specifically on the advent and progress of Trichoderma research at a global level. The compiled reports will provide the scientific community with detailed information on the biology and multifaceted uses of the genus Trichoderma.
The genus Trichoderma is one of the most prevalent culturable fungi in the family Hypocreaceae and can be found in all types of ecological diversity. The genus is soil-dwelling, free-living, cosmopolitan, facultatively anaerobic, filamentous, and asexually reproducing and is widely distributed in root and soil ecosystems and plant debris. This fungus has long been recognized as a microbial biocontrol agent that can replace chemical fungicides against the large range of fungi that cause root rot, soilborne, and foliar disease (Harman et al., 2006) and for increasing root and shoot development, crop productivity, resistance to abiotic stresses, and nutrient uptake (Saba et al., 2012). By enhancing crop productivity, it also contributes to food security in a sustainable way without causing ecological imbalance. Since the first recognized application of Trichoderma species in early 1930, they have been widely applied for the management of many plant pathogens and associated diseases (Howell, 2003; Harman et al., 2006). Some of them are wilt disease, dry root rot, damping off, and collar rot caused by Fusarium spp., Rhizoctonia spp., Pythium spp., Phytophthora spp., and Sclerotium rolfsii, respectively (Yang et al., 2011). Trichoderma shows diverse versatility, high competence, and profuse root-colonizing nature and also exists as a virulent plant symbiont (Papavizas, 1985). Characteristically, the fungus is identified through rapid growth, bright green to yellow-colored conidia and branched conidiophores (Kumar et al., 2019). Due to its eco-friendly nature, the fungus has been recognized as a substitute to commercial synthetic fungicides against a broad range of fungal pathogens. It is also extensively utilized as a model organism to understand biological interaction among antagonistic fungi, mechanisms, host-defense response, and plethora of heterologous proteins affecting plant metabolism and physiology.
Trichoderma was first described 200 years ago by Persoon (1794) in Germany. In India, it was first isolated by Thakur and Norris (1928) from Madras. In the early 20th century during World War II, this fungus was identified as cellulolytic and identified as T. viride QM6a but later renamed T. reesei by Elwyn T. Reese due to its ability to decay wood (Simmons, 1977). In 1932, the first ever evidence of T. lingorum (Tode) Harz. (H. virens) as a mycoparasite having biocontrol potential against Rhizoctonia solani was established, followed by discovery of gliotoxin as the first antimicrobial compound from Trichoderma species in 1934 (Weindling, 1932; Weindling, 1934). Earlier studies by Gutter (1957) also highlighted the discovery of an effect of light on conidiation of T. reesei in 1957. However, the genus Trichoderma was classified for the first time in 1969 by Rifai (1969), leading to a concept for the identification of species belonging to the genus Trichoderma, and by 2006, more than 100 distinct species had been described (Druzhinina et al., 2006).
The first evidence of T. harzianum suppressing Sclerotium rolfsii in the field was reported in 1972 (Wells et al., 1972). Research on cloning studies on Trichoderma species dates back to 1983 reported cloning of first cellulase of T. reesei (Shoemaker et al., 1983) followed by cloning of the first mycoparasitism-related genes (prb1) and its induction by cell walls in 1993 (Geremia et al., 1993). In 1986, the ability of Trichoderma to support plant growth was discovered for the first time (Chang et al., 1986). Specifically, the genus boosts plant immunity by induced resistance in 1997 (Bigirimana et al., 1997) and internal colonization of root system by Trichoderma in 1999 (Yedidia et al., 1999). The first commercial formulation of Trichoderma, Binab T, for biological control of plant diseases was registered in 1989.
Trichoderma was historically described as a genus of anamorphic fungi found primarily in rotting plant material and soil (Persoon, 1794) with T. reesei as the first evidence for the existence of the genus. As early as 1865, Tulasne and Tulasne (1865) postulated a sexual relationship between Hypocrea (H. jecorina) and Trichoderma (T. reesei). Their hypothesis was confirmed 100 years later (Kuhls et al., 1996). As a consequence, Rossman et al. (1999) described the genera Hypocrea, Podostroma, and Sarawakus belonging to the Hypocreaceae family and class Ascomycetes as teleomorphs of Trichoderma. According to Bisby (1939), for many years, due to morphological similarity in the majority of Trichoderma species as rapid growth, bright green conidia with repetitive branched conidiophore, it was considered as a single species, T. viride. Earlier classification of the genus Trichoderma included consolidated taxonomical scheme proposed by Rifai (1969) that introduced the concept of “species aggregate” and identified nine species under the genus based on morphological characterization in a monograph. In later studies, Bissett (1991) attempted to classify Trichoderma by integrating similar forms within species concept based on morphology, that is, conidiophore branching system into five sections such as Pachybasium, Saturnisporum, Trichoderma, Longibrahiatum, and Hypocreanum. Table 1 presents morphological characteristics used for identification of important Trichoderma species. In the 20th century, several new DNA-based approaches, such as rDNA sequence analysis, random amplified polymorphic DNA (RAPDs) analysis and PCR fingerprinting methods were used in fungal systematics and taxonomical studies including identification and phylogenetic classification of various Trichoderma species. Several studies demonstrated great genetic diversity among Trichoderma species by identifying four distinct species within the T. harzianum aggregate as T. harzianum s. str., T. atroviride, T. longibrachiatum and T. asperellum(Hermosa et al., 2000). The biotypes within T. harzianum s. str. as T. harzianum Rifai and T. hamatum (Bon.) Bain were linked to biocontrol and mycoparasitic activity, whereas, T. aggressivum was associated with green mold of mushroom (Samuels et al., 2002). Phylogenetic studies based on 18S rDNA sequence analysis (Kulling-Gradiner et al., 2002), where, small mitochondrial rDNA subunit, ITS1, 5.8S rDNA, ITS2, 28S rDNA, translation elongation factor (TEF-1), and endochitinase 42 were used to construct a phylogenetic tree, suggested Trichoderma as a monophyletic branch under Hypocreaceae and identified 46 species under three sections, namely, Trichoderma, Pachybasium, and Longibrachiatum. In a recent study, Gu et al. (2020) identified four new species of Trichoderma in the Harzianum clade (Figure 1) based on ITS, RPB2, and TEF1-alpha sequence data set.
Figure 1 Phylogenetic tree of four new species of Trichoderma identified by Gu et al. (2020) based on Maximum Likelihood analysis of a combined ITS, RPB2, and TEF1α sequence data set.
As per Kirk’s classification, taxonomy based on molecular phylogeny in the Ainsworth and Bisby’s dictionary of fungi (10th edition), teleomorphic stage of the genus Trichoderma belongs to the domain Eukarya, kingdom Fungi, phylum Ascomycota, class Sordariomycetes, subclass Hypoceromycetidae, order Hypocreales, family Hypocreaceae, and genus Hypocrea species (Voigt and Kirk, 2011). A total of 75 Trichoderma species have been identified; the majority of which are considered as important microbial biological control agents. These include T. harzianum, T. hamatum, T. koningii Oud., T. polysporum (Link ex Pers.) Rifai, and T. virens (J. Miller, Giddens, and Foster) von Arx (Harman et al., 2004). Complete genome-sequencing analysis of the genus Trichoderma was assembled, annotated, and analyzed for the first time in the case of T. reesei as cellulase producer (Martinez et al., 2008) followed by T. virens, T. atroviride, T. harzianum, and T. asperellum (Kubicek et al., 2011) as microbial biocontrol species enabled studies on evolution in the context of ecological fitness. In recent years, identification and characterization of newly isolated Trichoderma species has been elucidated by development of phenotypic arrays investigating carbon utilization patterns (Kubicek et al., 2003), oligonucleotide barcode (TrichoOKEY), and similarity search tool (TrichoBLAST) (Kopchinskiy et al., 2005). At present, Index Fungorum Database listed 471 different names for Hypocrea species and 165 records for Trichoderma, whereas, International Sub commission on Trichoderma/Hypocrea listed 104 species (Internationally) and 13 species (From India) based on characterization at molecular level (Table 1).
Trichoderma species are ubiquitous, fast growing, cosmopolitan, and widely distributed as dominant microflora in soil including agricultural, orchard, forest, soil with organic matter (OM), pasture land, and desert soils from cool temperate to tropical climates (Domsch et al., 1980; Roiger et al., 1991). Saprophytic Trichoderma species were also recovered as mycelia from soil’s top horizon (F and H), humid litter of deciduous and coniferous forests as well as from extreme environments such as mangrove swamps, salt marshes, and estuarine sediments (Domsch et al., 1980; Widen and Abitbol, 1980). Knowledge on effects of ecological factors on Trichoderma species may lead to improve understanding of distribution, population dynamics, survival, and proliferation in soil and rhizosphere. Papavizas (1985) also found Trichoderma populations on plant root surfaces, decaying bark and on resting structure of soilborne fungi such as sclerotia or other fungal propagules.
However, soil colonization, composition, biomass, and biological activity of Trichoderma species are influenced by ecological parameters such as moisture and temperature of soil, atmosphere, pH, OM, nutrient content, and plant types (Domsch et al., 1980). Trichoderma species have been reported to grow in a wide range of soil temperatures varying from 0°C to as high as 40°C favoring T. viride and T. polysporum in cool temperature regions, whereas T. harzianum in warm tropical soils (Klein and Eveleigh, 1998). In an extensive study by researchers (Zehra et al., 2017a), variation in temperature attributed to the existence of Trichoderma species in particular niches by affecting growth, metabolic activity, enzyme production, and production of volatile antibiotics. The reduction of soil moisture or increase in soil temperature greatly hampered the establishment of Trichoderma colonies in soils by reducing the hyphal growth, germination, and spore production (Clarkson et al., 2004).
In the past, a few studies also concluded that T. pseudokoningii and T. hamatum are adapted to excessive soil moisture conditions, whereas T. koningii and T. hamatum are widely distributed in diverse climatic conditions. In general, the optimum growth and development of Trichoderma have been reported not only in pH condition ranging from 3.5 to 5.6 but also extended to extreme pH up to 2.1 in several studies (Ghazanfar et al., 2018). In addition, soil carbon dioxide (CO2) atmospheric content also affects growth of Trichoderma by affecting soil pH upon combining with water to form weak acid, that is, carbonic acid, which readily dissociates into H+ ions and , thus, decreasing soil pH (Killham, 1994). Dix and Webster (1995) also revealed that, under high soil CO2 concentration, basic substrates positively affect the growth of Trichoderma species, by influencing the availability of ions and nutrients in soil through salt solubilization in soil solution. Survivability of Trichoderma species in soil after application is basically mediated by hyphae, aggregate, or mycelial fragments, resting structure such as chlamydospores and conidia (Papavizas et al., 1984). Persistence of conidia lasted up to 110–113 days without any amendments or decreased initially, then stabilized up to 1/10th of original population in soil for 24 months (Papavizas and Lumsden, 1982; Abbas et al., 2022).
Trichoderma species operated through mixed mode of action involving more than one mechanism for antagonistic interaction and suppression of plant pathogens either through direct mechanisms viz., mycoparasitism, competition, and antibiosis or complex indirect interaction by stimulating induced systemic resistance (ISR), solubilization and sequestration of nutrients, nutrient uptake, and enhancement of plant growth (Figure 2). Trichoderma species have been known for their prolific production of extracellular enzymes, proteins, fungitoxic compounds, antibiotics, and defense-related substances in addition to their ability to enhance shoot and root growth (Dutta and Das, 1999a), nutrient uptake, resistance to abiotic stresses, and crop productivity (Howell, 2003).
Figure 2 Mycoparasitism of Trichoderma spp. within the soil community (adopted from Druzhinina et al., 2011).
Plant pathogenic fungi are sensitive to Trichoderma species through direct physical contact, and such biocontrol activity is called mycoparasitism (Figure 3; Dix and Webster, 1995; Druzhinina et al., 2011). However, the concept of mycoparasitism via mycoparasitism by Trichoderma dates back to demonstration of parasitism of Rhizoctonia solani by T. virens in mitigating citrus seedling disease by Weindling (1932). Earlier reports of direct parasitism of Pythium ultimum and Sclerotium rolfsii by Trichoderma species also provide evidence of its mycoparasitic ability (Papavizas, 1985). Dix and Webster (1995) suggested that mycoparasitism occurs as the direct mode of antagonism as a sequential process involving three steps, including chemotrophic growth, coiling and interaction of hyphae, and release of lytic enzymes. The mycoparasitic interaction is usually mediated by host-derived chemicals that are detected by Trichoderma species through specific signaling mechanisms mediating recognition via diffusible signals such as oligochitins, inducing enzyme secretion, namely, exochitinases, endochitinases, and 1,4-β-N-acetylglucosaminidases, extracellular β-(1,3)-glucanases, proteases, and lipases (Viterbo and Horwitz, 2010). Upon establishment of contact, Trichoderma attaches to the fungal cell through formation of papillae/appressoria-like structures, causing mycoparasitic coiling around the target fungus mediated by hydrophobin-like proteins and a lectin complex from the cell wall of Trichoderma and target pathogen (Figure 3), respectively (Howell, 2003). The secretion of particular lytic enzymes from the cell wall of Trichoderma viz., glucanases, chitinases, pectinases, and peptaibol antibiotics induced a cascade of physiological changes within the target fungus facilitating flow of nutrients to the mycoparasite and degeneration of target fungus (Howell, 2003). Mycoparasitic ability of Trichoderma species have been studied against various soilborne pathogens, such as Fusarium oxysporum, F. solani, R. solani, S. sclerotiorum, S. rolfsii, and Colletotrichum capsici in our earlier studies (Dutta and Das, 1999a; Dutta and Das, 2002; Dutta and Das, 2009; Dutta et al., 2018; Dutta et al., 2020).
Figure 3 Plant-pathogen-antagonist tri-trophic interaction, how Trichoderma species can modulate the molecular signaling in the challenge between the Fusarium and Alternaria and the host (tomato).
Weindling (1934) proposed the concept of “lethal principle” describing influence of certain lethal factors excreted by T. lingorum in soil inhibiting growth and development of R. solani and S. americana displayed a paradigm shift toward involvement of lethal factors apart from mycoparasitism in biocontrol activity. In 1941, the factor causing the “lethal principle” was identified as gliotoxin, secreted by Gliocladium virens (Now T. virens). Later, in 1983, Howell and Stipanovic (1983) reported another antibiotic, that is, gliovirin secreted from T. virens known for potential inhibitory effect against Phytophthora species and Pythium ultimum. The phenomenon of antibiosis, utilized by Trichoderma, produces low-molecular weight, diffusible, specific compounds, or an antibiotic possessing antifungal and antibacterial properties. Depending upon the biochemical nature, antibiotics act as metabolic inhibitors or block protein synthesis (translational pathways), penetrate host cells, inhibit cell wall synthesis, growth, uptake of nutrients, sporulation, and metabolite production by target pathogen.
Various species of Trichoderma are known for producing a diverse range of secondary metabolites (SMs) including polyketides, pyrones, oxygen heterocyclic compounds, polypeptides, terpenoids, and derivatives of fatty acids and amino acids (Table 2). The emission of coconut odor in case of few strains of T. viride and T. hamatum might be due to release of volatile 6-pentyl-α-pyrone, whereas, pigment-related compounds include anthroquinones such as chrysophanol (1,8-dihydroxy-3-methyl-9,10-anthroquinone), paschybasin (1,8-dihydroxy-3-methyl-9,10-anthroquinone), and emodin (1,6,8-trihydroxy-3-methyl-9,10-anthroquinone). Some metabolites attributed to mycotoxic properties of Trichoderma include trichothecenes (e.g., trichodermin, which impairs plant growth), cyclic peptides (e.g., suzukacillin, lipophilic alamethicin, trichopolyns, trichotoxins, and trichorianine, which attack the cell membrane of bacteria and eukaryotes promoting lysis), and isocyanide (e.g., trichoviridin). The volatile and non-volatile metabolites produced by various species of Trichoderma are described under separate section. In our recent study, cell-free culture filtrate of T. pseudokoningii showed efficacy against C. capsici, S. sclerotiorum, R. solani, and F. oxysporum due to release of extracellular SMs (Dutta et al., 2018).
Competition is one of the classical biocontrol mechanisms utilized by the genus Trichoderma, indirectly eliminating pathogens via reduction of food source and niche exclusion (Lorito et al., 1996; Elad et al., 2000). Corke and Hunter (1979) provided the first evidence of competition exerted by Trichoderma as a basis of biocontrol against Chrondrostereum purpureum, the silver leaf pathogen of plum trees. Sivan and Chet (1989) determined expression of antagonism by different Trichoderma species against F. oxysporum by exhibiting competition for carbon. Trichoderma species have been regarded as most aggressive competitors due to their ability to extensively proliferate in soil, competing for nutrients, space, water, or oxygen and capacity to mobilise soil nutrients as compared with other soil fungi. Such competitive ability enhanced by exerting resistance against a variety of toxins or antimicrobial compounds produced by other microorganisms due to the presence of ATP-binding cassettes transporters. Reports of T. harzianum CECT 2413 producing Gtt1 gene encoding for high-affinity glucose transporter expressed at very low glucose concentration and T. virens producing TvInv encoding for intracellular invertase for sucrose hydrolysis provided evidence for nutrient competition similar to the scenario of competence among microorganisms (Benitez et al., 2004).
The term “Rhizosphere” was coined for the first time for Trichoderma strains by Ahmad and Baker (1987) who attributed the capability of Trichoderma to colonize root surfaces to a depth greater than 2 cm (Chao et al., 1986), proliferate in developing rhizosphere to a concentration exceeding initial population on seed coat (Papavizas, 1982) and compete with other microorganisms for nutrients secreted by roots in rhizospheric soil. Seed treatment with T. harzianum rhizosphere competent strain T-95 of barley, cucumber, pea, radish, and tomato was implicated in reduced damping-off disease incidence caused by Pythium ultimum due to the absence of fungal units in up to 8 cm of root segment as compared with 3,000 CFU/g rhizosphere soil in case of untreated seeds (Ahmad and Baker, 1987). The colonization of roots by Trichoderma species is mediated by attachment of the fungus to roots via appressoria-like structures (class I hydrophobin encoded by gene TasHyd1), whereas penetration is achieved by release of protease and cellulolytic enzymes (Brotman et al., 2008). Recent study showed that rhizosphere competence by Trichoderma strains is governed by extensive communication via exchange and perception of signaling molecules, that is, deposition of fungal elicitors, auxin-like metabolites, and proteinaceous compounds released by Trichoderma are perceived by plants rhizosphere (Garnica-Vegara et al., 2015).
Studies by McLean et al. (2005) also determined that proliferation of T. atroviride C52 in onion rhizosphere and rhizoplane are dependent on the type of formulation used to introduce the fungus into the soil. Results indicated higher fungal concentration of 105 cfu/g soil was maintained through pellet formulation with reduced incidence of Sclerotium cepivorum as compared to solid-substrate and seed-coating formulations maintaining 104 and 101 CFU/g soil, respectively. Similarly, T. viride as cob-based formulation, when applied in the form of seed coat and soil treatment, imparted enhanced plant growth performance of mungbean, pea, and pigeon pea through better rhizosphere competence and reduced disease incidence of Fusarium in Cajanus sp. by 86.00% was also document by Pappu (2018).
Induction of local and systemic resistance as indirect mechanism by Trichoderma species have been reported for both monocots and dicots involving recognition of the fungus by plants through ISR and systemic acquired resistance (SAR) against many phytopathogens (Harman et al., 2004). The response is mediated by phytohormones viz., jasmonic acid (JA), and ethylene (ET) as closest analogue of induced resistance activated by rhizobacteria (Van loon, 2007) and induction of pathogenesis-related (PR) genes expression mediated by salicylic acid (SA), triggered by biotrophic and hemi-biotrophic pathogens. The first demonstration of induced resistance by Trichoderma was reported by Bigirimana et al. (1997) against Colletotrichum lindemuthianum and Botrytis cinerea, causing foliage diseases of beans.
The concept was further supported by Yedidia et al. (1999) who studied induced resistance by T. harzianum against cucumber seedling disease. Indirect evidence of plant ISR by Trichoderma was first described by (Calderon et al., 1993) through induction of hypersensitive response (HR) and phytoalexin synthesis by T. viride cellulase in grapevine cell cultures. Later, Chang et al. (1997) demonstrated the capability of heat-stable mycelial extracts of T. longibrachiatum to induce disease resistance against Phytophthora parasitica by induction of higher level of PR-1b and PR-5 in tobacco, Nicotiana tabacum (Chang et al., 1997). In addition, reports on soil inoculation with T. harzianumT39 imparted resistance to leaves of bean plants, that is, parts spatially separated from the site of inoculation against B. cinerea and C. lindemuthianum have also been documented (Bigirimana et al., 1997; De Meyer et al., 1998).
The ability of Trichoderma species to enhance plant growth and productivity was determined by utilization of indirect mechanism mediated by solubilization of mineral nutrients available in limited amounts for plants in soil, involving chelation and reduction (Harman et al., 2004). Earlier evidence on solubilization of various plant nutrients such as rock phosphate, Cu2+, Fe3+, Zn2+, and Mn4+ ions by T. harzianum T22 was documented by Altomare et al. (1999), possibly due to production of diffusible metabolites capable of reducing Fe (III) and Cu (II) due to the formation of Fe (II)-Na2-2,9-batho- and Cu(I)-Na2-2,9- dimethyl-4,7-diphenyl-1,10-phenanthrolinedisulfonic acid complexes. Brotman et al. (2012) described nitrogen use efficiency of T. asperelloides T203 through increased amino acid content in colonized plants by allocating, re-used nitrogen, and increased nitrogen uptake as major determinants of transported nitrogen in plants.
A reduction of soil pH, caused by biosynthesis and release of organic acids, such as gluconic, citric, and fumaric acids, facilitates Trichoderma’s mobilization of immobile nutrients, including phosphates, iron, magnesium, and manganese (Vinale et al., 2008). Jalal et al. (1986) identified Fe-chelating complex, that is, siderophores produced by T. virens are derivatives of hydroxymate nature classified under three families viz., fusarinines, coprogens, and ferrichrome, which play key role in binding insoluble iron (Fe3+), transforms it into a soluble form (Fe2+) making it available to plants. In addition, the formation of siderophore-iron complex by Trichoderma species participates in depletion of Fe sources from soil inhibiting growth of phytopathogenic fungi (Wallner et al., 2009). Siderophore production also played role in conidial germination of T. atroviride (Velazquez-Robledo et al., 2011), competitiveness of T. asperellum, and suppression of F. oxysporum f.sp. lycopersici (Segarra et al., 2010).
Fungal cell walls are composed of polysaccharides, lipids, proteins, β-glucans, and 90% of chitin. In contrast, cell walls of oomycetes consist of cellulose. Production of hydrolytic enzymes viz., chitinase, glucanase, N-acetylglucosaminidase, and protease by Trichoderma sp. causes the breakdown down of polysaccharides, chitin, and β-glucans, which are responsible for rigidity and integrity of fungal cells, and are attributed to successful mycoparasitic relationships. In recent literature, dual culture experiments between Trichoderma and R. solani Heflish et al. (2021) unravelled the presence of a diffusible molecule before direct contact, determined to activate transcription of cell wall degrading enzymes (CWDEs) encoding genes. However, under secretome analysis conducted for direct confrontation of T. harzianum EST 323 against R. solani through two-dimensional gels (2-DE) and liquid chromatography mass spectrometry (LC-MS/MS), seven CWDEs (viz., xylanase, chitinase, β-1,6-glucanase, β-1,3-glucanase, mannose, and protease) were identified (Tseng et al., 2008).
In similar studies, proteomic analysis confirmed a critical role of CWDEs produced by T. harzianum in antagonism by deactivating mycelia of B. cinerea, indicating cell walls as the primary target during mycoparasitism (Yang et al., 2009). Several studies identified virulent genes encoding for CWDEs viz., Eng18B a gene encoding for typical glycoside hydrolase family enzyme by T. atroviride, Nag1, and ech42 gene encoding for N-acetyl-glucosaminidase and endochitinase, respectively, by Trichoderma species (Kulling et al., 2000). Recently, the concept of enzyme biosynthesis merged with production with antibiotics unravelled a synergistic mechanism of biological control in T. harzianum through a combination endochitinase, gliotoxin, and peptabiols resulted in a detrimental effect on conidial germination and hyphal elongation of B. cinerea (Gu et al., 2020).
Trichoderma species are notable for having the ability to grow rapidly, exploit diverse substrates, and resist harmful chemicals. Among soilborne fungal communities, they are dominant. Trichoderma produce a broad range of biologically active compounds that are among the most fascinating and important properties of the organism. In particular, plant defense responses can be mediated by proteins, peptides, and low-molecular-weight compounds produced by Trichoderma species (Reino et al., 2008). Compounds with low molecular weights include aromatic compounds and polyketides such as butenolides and pyrones, isocyanate metabolites, and volatile terpenes. It produces volatile (such as ET, alcohols, hydrogen cyanide, ketones, and aldehydes) and non-volatile (such as peptides) compounds that inhibit microbial growth. Reino et al. (2008) have shown that Trichoderma species can produce a number of volatile (such as pyrones and sesquiterpenes) and non-volatile (such as peptaibols) metabolites. Here are a few examples of the volatile organic compounds (VOCs) and other metabolites released by different species of Trichoderma.
Trichoderma are well-known for their VOCs that make them of interest to the scientific community. Natural products, or SMs, are among these compounds. Often, these compounds do unknown or obscure things in the producing organism that are vital to humankind. Some of these VOCs are beneficial to society, such as ones for medical, industrial, and agricultural purposes. Numerous reports suggest that some VOCs possess antibacterial and immunosuppressive properties as well as phytotoxic and mycotoxin properties. VOCs are low-molecular-weight organic compounds with substantive vapor pressure under ambient conditions. They have diverse chemical structures such as alcohols, ketones, mono- and sesquiterpenes, esters, lactones, or C8 compounds (Korpi et al., 2009; Siddiquee et al., 2012). Chemical ecologists explain VOCs as semiochemicals that attract and deter insect pests and other invertebrates.
VOCs derived from fungi are used for biological control of plant pathogens in agriculture. Moreover, these VOC mixtures have been studied for their ability to promote plant growth. Food companies use the same biological control properties to reduce fungal spoilage of food commodities in postharvest, which is called “mycofumigation.” The potential role of fungal VOCs has recently been examined. The genus Trichoderma is well-known for its production of volatile compounds with potential biological activity. VOC is usually defined as normal saturated hydrocarbons (C7-C30), cyclopentane, cyclohexane, alcohol, fatty acid, sulfur-containing compounds, esters, simple and benzine derivatives, hydroxy, or amino compounds. A compound’s production differs based on (1) its specific molecular structure, (2) its strain and species, (3) its presence of microbes, and (4) the balance between its biosynthesis and biotransformation rates (Vinale et al., 2008, 2010). The important VOCs derived from Trichoderma are shown in Table 3.
Table 3 revealed that the major compounds produced by Trichoderma species include gliotoxin, gliovirin, glisoprenin, viridin, 6-pentyl-α-pyrone, hepteledic acid, koninginins, trichodermamides, peptaibols, anthraquinones, polypeptides, terpenoids, polyketides, trichodermaides, trichothecenes, harzialactones, compounds derived from alpha-amino acids, and azaphilones (Vey et al., 2001; Reino et al., 2008). Liu et al. (2009) reported that crysophanol, pachybasin, ω- hydroxypachybasin, emodin, 1, 7-dihydroxy-3-hydroxymethyl-9,10-anthraquinone, and 1,5-dihydroxy-3-hydroxymethyl-9,10-anthraquinone showed potential bioactivity against several plant pathogens. In addition, pchybasin and emodin play major roles in the biocontrol mechanism of Trichoderma mycoparasitic coils through cAMP signaling (Lin et al., 2012). A novel compound, cerinolactone, extracted from T. cerinum together with three known butenolides containing harzianolide, 3,4-dialkylfuran-2(5H)-one nucleus, T39butenolide, and dehydroharzianolide, both compounds exhibited activities against B. cinerea, R. solani, and P. ultimum (Vinale et al., 2012). T. harzianum ETS 323 exhibits a stimulatory effect and an antagonistic action on R. solani by a novel compound of l-amino oxidase (Th-LAAO). Considering these results, T. harzianum is a good biocontrol agent due to its ability to provide insight into the function of l-amino acid oxidase (Yang et al., 2011). Due to these beneficial effects, T. asperellum, T. atroviride, and T. harzianum strains have been used as plant protection agents in agriculture to control molds (Verma et al., 2007).
Mycoparasitism and interaction of Trichoderma with plants are mediated by VOCs (Vinale et al., 2008). Few research investigations addressed the effect of various culture media on the volatile types produced by Trichoderma (Wheatley et al., 1997) or the function properties of some of these volatiles (Nemčovič et al., 2008). There have been reports of multiple Trichoderma species producing VOCs as shown in Table 3 (Stoppacher et al., 2010). VOCs form intermediates and end products of diverse metabolic pathways and include ketones, alcohols, esters, lactones, mono- and sesquiterpenes, and some C8 compounds (Korpi et al., 2009; Siddiquee et al., 2012). These compounds are relatively nonpolar and have high vapor pressures. The compounds with high molecular weight are polar, such as peptaibols.
To determine whether these compounds are significant during the life cycles of their producing species properly, controlled studies are needed. Nevertheless, observing fungal ecology may lead to the development of strategies that have proven effective for the discovery of novel bioactive fungal compounds. The use of biocontrol tactics is one example. Historically, Trichoderma species have been used as biological control agents since the 1930s, and numerous field experiments have proven that applications of Trichoderma species promote plant growth while limiting pathogen growth. Thus, due to production of VOCs, Trichoderma species are effective biofungicides, as they degrade other pathogenic fungi enzymatically, produce antimicrobial compounds that kill pathogenic fungi, and compete with them for nutrients and space.
SMs produced by Trichoderma species have a variety of biological activities. There have been a number of reviews published about Trichoderma metabolites. These reviews focus on structure, biological activity, or fungal origin. An overview of some of the most important non-volatile compounds in Trichoderma has been provided in Table 3. Seventeen compounds were isolated from the endophytic fungus Trichoderma sp. Xy24: ergosterol, trichodimerol, cyclonerodiol, and trichoacorenol (Zhang, 2015); 10,11-dihydroxy-cyclonerodiol, trichocage B, harzianone, 14-hydroxy-trichoacorenol; ergokonin B, (9R,10R)-dihydro-harzianone, and methyl stearate (Zhang et al., 2014), trichoacorenol B, harzianelactone, cyclonerodiol B, and trichoacorenol C (Zhang et al., 2016). Trichoderma harzianum and T. longibrachiatum that contain tetracyclic scaffolds, harziandione, have been described as potential microbial biocontrol agents against C. lagenarium and F. oxysporum (Miao et al., 2012). There is a potential antagonistic action of T. saturnisporum owing to the presence of cerebroside A, sorbicillin B, bisvertinolone, and saturnispol A–D (Meng et al., 2017). The presence of 5-hydroxyvertinolide, bislongiquinolide (Andrade et al., 1997), and Ergokonin A (Vicente et al., 2001) in T. longibrachiatum also demonstrated antagonistic activity. Likewise, the antagonistic effect of T. harzianum was also attributed to non-volatile metabolites such as ergosterol, harzianolide, endoperoxide, and 3-indol acetic acid.
Inorganic pesticides (insecticides, fungicides, and herbicides) and fertilizers have played vital role in supplementing plant nutrients and curbing biotic stresses. The utilization of bioformulations as part of integrated plant disease management strategies involved combination of cultural, physical, chemical, and biological means. Dutta et al. (2017) studied compatibility of T. pseudokoningii with selective fertilizers, insecticides, fungicides, herbicides, and organic stickers. Researchers found that all the tested pesticides inhibited the growth of T. pseudokoningii, with the exception of thiamethonaus 25% WG at 0.125% and ritha at the highest test dose found compatible. Urea and MOP were found to be compatible, whereas SSP and CAN inhibited growth. These variations in inhibitory potential are attributed to inherent variations in chemical ingredients within the fungus’ cellular components. In another study, T. viride also showed compatibility with insecticide (imidacloprid), fungicides (mancozeb, tebuconazole, pencycuron, and propineb), and herbicides (imazathafir, 2, 4-D sodium salt, and oxyfluorfen) (Madhavi et al., 2011). In a recent study, Singh et al. (2019) tested compatibility of Trichoderma species with nematicides such as carbofuran, aldicarb, phorate, and thionazin and found compatibility with carbofuran and phorate for management of root knot nematode in rice. Trichoderma viride and T. harzianum were also found compatible with azoxystrobin and metalaxyl, respectively (Shashikumar et al., 2019).
In a view to improve the efficacy of T. harzianum application against phytopathogens and plant growth promotion, its compatibility was tested with biosynthesized (27.64 nm) and commercial grades (20 nm, Sigma-Aldrich, St. Louis, Missouri, United States) of silver (Ag) and chemically synthesized zinc oxide (ZnO, 20 nm) nanoparticles (NPs). In this context, Biswas and Dutta (2019) reported 100% compatibility of T. harzianum with commercial grade of AGNPs at 5,000 ppm and slightly lower of 98.94 and 90.00% in case of myco-AgNPs at 1,000 and 5,000 ppm, respectively. In contrast, inhibitory effect on growth of T. harzianum was observed under all the tested concentrations of ZnONPs. Recently, in a study by Upamanya et al. (2020), compatible reactions of T. harzianum and T. asperellum were also tested with fungal entomopathogens viz., Beauveria bassiana s.l., and Metarhizium anisopliae s.l. (recently named as M. robertsii) for development of microbial consortia. Under standard co-culture conditions, combination-I (B. bassiana s.l. + T. harzianum, M. anisopliae s.l. + T. harzianum, B. bassiana s.l. + M. anisopliae s.l. + T. harzianum) and combination-II (T. asperellum + B. bassiana s.l., T. asperellum + M. anisopliae s.l., T. asperellum + B. bassiana s.l. + M. anisopliae s.l.) gave compatible reaction. Mixed culture showed mutual growth and overlapping among test microbial biocontrol agents, due to lack of production of SMs by individual organism against another, while growing in the same media.
The development of potential Trichoderma species as successful microbial biocontrol agents and its effective commercial application depends on production of viable propagules, mass production, formulation strategies, and optimized delivery systems. Fungal spores of Trichoderma as active ingredient are formulated using different organic and inorganic carriers (diluents and surfactant), through solid or liquid state fermentation to improve physical characteristics, increase shelf life, and protect against adverse environmental conditions. Different kinds of Trichoderma propagules used in formulation includes hyphae, chlamydospores, and conidia, of which both conidia and chlamydospore are highly preferred means due to their ability to withstand adverse environmental conditions as compared with hyphae due to lack of resistance toward dehydration (Howell, 2003).
Under solid state formulation, Trichoderma species are commonly multiplied on boiled rice, sorghum seeds, rice saw dust, wheat bran-saw dust, and agro-waste products such as peels of potato, brinjal, papaya, banana, spinach, guava, used tea leaves, sugarcane, and pea husk used as solid substrate or food base. Solid formulation types viz., wet dust, dry pellets, granules, dry dust, and granules are adjuvated by using adhesive substances such as Arabic gum, carboxymethylcellulose (CMC), clays, compost, talc powder, and so forth. In a study by Dutta and Das (2009), the seed treatment of French bean (var. Contender) with talc-based formulation of T. harzianum in combination with methylcellulose and carbendazim was found significantly efficient and at par with seed treatment with carbendazim for the management of white mold rot of bean.
Liquid formulations were adopted for multiplication of fungal propagules in soluble materials viz., broth cultures of potato dextrose broth (PDB), and agricultural substrates such as rice water, vegetables juices, and boiled dal. Fully grown mycelial mat along with supernatant imposed with submerged conidia are grounded homogenously and amended with several adjuvants such as carboxymethyl cellulose (CMC), Tween-80, mannitol, peptone, and oil. Sprayable/liquid formulations include soluble liquids (SLs), soluble powders (SPs), soluble granules (SGs), emulsifiable concentrates, and liquid suspension dispersed in water, that is, suspension concentrates (SCs) and aqueous suspension (AS). Das et al. (2006) tested efficacy of osmoticant (mannitol) amended PDB liquid formulation yielded higher biomass, sporulation, cfu, and dry weight of biomass followed by modified Richard’s broth (MRB). In addition, talc-based formulation at 3:1 dose showed higher sporulation as compared with starch-based formulation at 1:1 dose with a shelf life of 60 and 30 days, respectively. However, seed treatment with bioformulation enriched with T. harzianum + talc + osmoticant assessed under field condition showed lowest stem rot disease index caused by R. solani with higher enhanced percent seed germination, plant vigour, and crop yield.
In North-Eastern region (NER) of India, several locally made liquid-based biopesticides from native strains of Trichoderma species were developed such as Org-Trichojal (T. harzianum) in Assam, UmTricho (T. harzianum), UmTriv (T. viride), and two Trichoderma-based microbial consortia, that is, UmTim (T. harzianum + Metarhizium anisopliae s.l.) and UmComb (T. harzianum + Beauveria bassiana s.l. + M. anisopliae s.l. + Akanthomyces (=Lecanicillium) lecanii + Pseudomonas fluorescens) in Meghalaya (Figure 4) and maintained by team workers at Central Agricultural University (Imphal), Umiam and Assam Agricultural University, Jorhat (Dutta et al., 2020; Dutta, 2020). The technology was used in preparation were standardized by Dutta et al. (2020) as mycelial mat centrifuged in PDB, amended with mannitol (osmoticant), sunflower oil (UV protector), Tween-80 (surfactant), CMC (cellulose-enrich), peptone (nitrogen supplier), and glycerol (preservative) with a shelf life of 180 days (Figure 4). Developed bioformulations have been locally accepted by farmers, KVKs, and institutes from the region as well as in different states of India and have been adopted in organic package of practices for cauliflower, cabbage, and spice crops of Assam. Seed treatment with T. harzianum–based bioformulation, that is, Org-Trichojal@ 5g/L of water + CMC @ 0.02% for 1h followed by shade dried for 2h prior to sowing has been recommended against soilborne disease such as damping off of cabbage and cauliflower. In spice crop cultivation, that is, bhoot jolokia, Capsicum chinense Jaqc, and seed treatment with T. harzianum–based bioformulation, Org-Trichojal was recommended at the rate of 5 ml/kg of seed against R. solani and Fusarium spp. Commercial formulations of Trichoderma species available worldwide and in India are listed in Table 4.
Figure 4 Biopesticides developed from native strains of Trichoderma spp. viz., (A) Org-Trichojal (T. harzianum) in Assam and (B) UmTriv (T. viride), (C) UmTricho (T. harzianum), and Trichoderma-based consortia, (D) UmComb and I (E) UmTim in Meghalaya of NER (Source: Dutta et al., 2020).
Genetic manipulation of Trichoderma species has been achieved by different techniques including protoplast-mediated transformation (PMT), electroporation, biolistic transformation, and Agrobacterium-mediated transformation (AMT) leading to alteration of fungal cell by insertion of genetic material into genome. Penttila et al. (1987) first successfully attempted introduction of DNA in prototrophic strain T. reesei along with argB gene as auxotrophic marker and smdS as dominant marker from Aspergillus nidulans by polyethylene glycol (PEG)/CaCl2–mediated protoplast transformation technique. Auxotrophic marker genes enable high transformation efficiency, whereas dominant marker genes confer properties viz., antibiotic resistance, nutrition utilization, for example, nitrogen or carbon, allowing transformed cells to thrive as compared with non-transformed cells. Several examples of dominant marker genes include acetamide (acrylamide) as nitrogen source encoded by amdS gene of A. nidulans, invertase sucAgene of A. niger using sucrose as carbon source, and pyrithiamine (ptrA)–resistant gene of A. oryzae have been expressed in T. reesei (Berges et al., 1993; Kubodera et al., 2002). Later, transfer of Trichoderma genes in plants was first successfully demonstrated by Lorito et al. (1998) in tobacco and potato plants expressing 42 kDaendochitinase gene chit42, conferred high resistance against A. alternata, A. solani, B. cinerea, and R. solani.
Chitinases genes elevated defense response by involving greater induction of ROS through expression of defense-related genes, PR enzymes, and terpenoid biosynthesis. Numerous studies demonstrated the expression of potential defense genes of Trichoderma sp. in plants through genetic transformation techniques have successfully conferred enhanced resistance to phytopathogenic fungi and bacteria (Table 5). Recently, marker-free transgenics of Trichoderma spp. were also generated via marker removal, recycling, and reusing for another transformation, through excision of marker genes mediated by native homologous recombination (HR) machinery or by heterologous site-specific recombinases. Sequential deletions using different cassettes comprising the excision of marker genes viz., direct-repeat-mediated HR for removal of pyr4 gene (Hartl and Seiboth, 2005) and site-specific Cre recombinase for removal of xyn1 promoter (Steiger et al., 2011) were reported in T. reesei. In addition, split-marker systems for successful gene deletions were also used in T. virens and T. atroviride (Trushina et al., 2013). Knockout strategies involving RNA interference (RNAi) gene silencing was also used to silence cel6a (cellobiohydrolase 2) gene expression in T. reesei (Brody and Maiyuram, 2009).
Table 5 Genetic manipulation using Trichoderma spp. in different crops conferred disease resistance.
The Trichoderma species, most commonly T. atroviride, T. harzianum, T. virens, T. hamatum, T. asperellum, and so forth, are progressively used as efficient microbial biocontrol agents due to their ability to activate local or systemic resistance in plants (Table 6). The concept of induced defense responses in plants by Trichoderma inoculation was first supported by the work of Yedidia et al. (1999), inoculated roots of 7-day-old cucumber seedlings with T. harzianum T-203 at 105 spores/ml. Roots and leaves of treated cucumber seedlings demonstrated initiation of plant defense, exerted increase in peroxidase activity, increase in chitinase, and deposition of callose-enriched appositions in inner surface of callose walls. Different strategies utilized by Trichoderma such as production of lytic enzymes, ABC transporter membrane pumps, diffusible or volatile and SMs compromising enzymatic and chemical weapons utilized by plant pathogens, make it efficient mycoparasite and antagonist. The defense mechanism of Trichoderma are triggered by regulatory mechanisms utilizing signal transduction pathways including heterotrimeric G-protein signaling, mitogen-activated protein kinase (MAPK) cascades, and cAMP pathway (Zeilinger and Omann, 2007).
Attempts made by Reithner et al. (2005) identified heterotrimeric G-protein signaling genes, that is, TGA of T. virens, GNA3 of T. reesei, and TGA1 and TGA3 of T. atroviride belonging to classes I and III of adenylate cyclase inhibiting G-alpha subunits, played an important role in the regulation of antifungal metabolites and coiling around host hyphae. MAP-kinase TVK1 characterized in T. asperellum, T. atroviride, and T. virens mediated the transfer of information from sensors, regulate signaling mechanisms, cellular responses in plant roots, and increased biocontrol effectively against R. solani (Mendoza-Mendoza et al., 2003). The perception of signals transmitted by Trichoderma in plants facilitated root colonization by swollenin and enhanced systemic resistance by ceratoplatanin family proteins, MAPK functions, indirectly leading to enhanced root proliferation, better growth and protection of plants. In a study, in model plant A. thaliana, root inoculation with T. virens and T. atroviride reported to increase the level of phytoalexin camalexin along with induction of PR-1a and LOX2 SA-responsive gene expression (Contreras-Cornejo et al., 2011; Contreras-Cornejo et al., 2016). Similarly, in another study, root inoculation of A. thaliana with T. asperelloidesT203 triggered rapid increase in the expression of transcription factors, that is, WRKY18, WRKY40, WRKy60, and WRKY33 exerted positive role in JA-mediated defense (Brotman et al., 2013; Abbas et al., 2022).
The pioneering work on Trichoderma species on their field application for disease management was initiated during 1970s, which reported success of several Trichoderma species viz., T. harzianum, T. hamatum, and T. viride against Pythium spp., F. oxysporum, R. solani, and Sclerotium rolfsii (Roy, 1977). Since then, many researchers from the region have worked on improving the efficacy of Trichoderma as potential antagonists against many soilborne and foliar plant pathogens and protectors of plants, as shown in Table 7.
Table 7 Application of Trichoderma species as fungal biocontrol agents against various crop diseases.
In 1976, the discovery of cellulase production efficiency of T. reesei QM6a by U.S. army during World War II (Reese, 1976) focused extensive research toward industrial application of enzymes, SMs, antibiotics and protein produced by Trichoderma spp. T. reesei, being potent cellulase producer were focused for improvement of enzyme cocktail efficiency resulting in the production of biofuel, that is, bioethanol from cellulosic waste material. Achievement of high level of cellulase and hemicellulase production on cellulose, xylan, plant polymers or lactose and high protein secretion capacity up to 100 g/L for 60.00% major cellulase Cel7a (CBHI) and 20.00% Cel6a (CBHII) attributed to agricultural or paper and pulp industry by-products (Buchert et al., 1998). Earlier evidences showed that the expression of heterologous protein by T. reesei was exploited for the production of calf chymosin followed by expression of immunologically active antibody fragments for production of several enzymes and proteins (Pentilla, 1998). Safe-scale industrial enzymes produced by Trichoderma species are used for brewing processes (β-glucanases), macerating enzymes in fruit juice production (hemicellulases, cellulases, and pectinases), feed additive for livestock farming (xylanases), baking, malting, grain alcohol production (cellulases), and food preservatives (Galante et al., 1998b).
In a study by Waiter et al. (2005), they reported that mutanase enzyme produced by T. harzianum can also be used in toothpaste for preventing accumulation of mutan in dental plaque. In wine industry, crude blend preparations of glycosidases and CWDEs produced by T. reesei are exploited in wine-making process for improving juice yield, flavor, clarification, filterability, facilitate liberation, and solubilization of phenolic compounds from seeds, skin, and flesh of grapes (Villanueva et al., 2000). Earlier, Perez-Gonzalez et al. (1993) also explored beneficial application of endo-β-1,4-glucanases and xylanases genes from T. longibrachiatum and T. reesei in wine making by developing recombinant yeast strains for improving free flow, different colors, intensity, stability while ageing, sensorial, and tasting capabilities in Pinot Noir and Ruby Cabernet. In addition, chemical such as 2,4,6-trichloroanisole released by T. longibrachiatum and T. viride have been involved in cork taint and musty-off odors. In addition, the application of Trichoderma sp. in beer industry attributed to exploitation of cellulolytic enzymes and recombinant yeast (Saccharomyces cerevisiae) constructed from egl1 gene from T. reesei for glucan hydrolysis, reduction of β-glucan content, enhanced filterability and beer flavor (Faulds et al., 2008).
Trichoderma species are effective biocontrol agents that can replace chemicals in agriculture. It is essential that microbial biocontrol agents succeed or fail as commercial products (Vurukonda et al., 2018). In order to be successful as a commercial product, it should fulfill farmer’s needs such as repeated positive results, realistic prices, easy usage, and long shelf life (Murphy et al., 2018). Nevertheless, a bioproduct with microbial biocontrol agents and/or SMs has the specific problem that its viability decreases during storage as well as its effectiveness for controlling pathogens and pests (Vurukonda et al., 2018). Lack of understanding of biocontrol techniques can result in a reduction in application and requirement of the product. Thus, understanding the practical deployment of Trichoderma as microbial biocontrol agents for disease management in agriculture is crucial.
Despite this, it is urgent that communication between researchers and the farmers be improved for efficient biocontrol methods. Therefore, it is crucial to ensure that farmers are aware of the correct use of products for a specific pathogen (Dutta and Das, 2009). There have been many studies investigating Trichoderma as efficient microbial biocontrol agents. Most of these research investigations, however, were conducted in lab environments, and their applicability was evaluated in the field. Alternatively, some Trichoderma species may be friendly with particular host plants in a narrow range of environmental conditions. The biological efficacy of Trichoderma can be affected by changes in agricultural conditions, including soil OM, pH, nutrients, and moisture content (Murphy et al., 2018). An in-depth analysis of field trials will help to develop strains of Trichoderma that are friendly with the crops and environment, as most isolates of Trichoderma are unique to their hosts and environments. Therefore, it is best to identify the field-related problems, follow up on continuous in vivo and in vitro laboratory experiments, and find a solution for that particular problem related to Trichoderma antagonists against plant pathogens and diseases. It is anticipated that these products will be in higher demand in the future (Vurukonda et al., 2018).
In terms of managing plant diseases, Trichoderma species could be a viable alternative to synthetic fungicides. Trichoderma species is already widely used against plant diseases as a microbial biocontrol agent. To develop successful commercial products of Trichoderma, in vitro tests under standardized conditions are routinely conducted to screen potential isolates of the fungus. However, field trials under different environmental conditions must also be conducted. Ecological and physical parameters of microbial biocontrol agents along with their environmental effects should be investigated in field experiments. For the commercialization of Trichoderma and their use by farmers in remote areas who do not know about them, further research is vital, as this would significantly reduce economic and environmental costs. We may be able to achieve this by using novel molecular technologies such as metagenomics and statistical advances, as well as environmental dynamics. Future work could integrate screening with antagonistic ability validation in greenhouse and field trials, as well as the production of biomass after the commercialization of Trichoderma species required to protect global food security. The following are an outline of the broad future outlook.
● Phylogenetic diversity of the genus Trichoderma needs to be explored by understanding sexual development; the genetic basis of chlamydospore production and the identification of niche-related genes through combined expression analysis and functional genomics can provide a blue print of Trichoderma species.
● As an opportunistic mycoparasite, investigation on induction and regulation of enzyme expression responsible for improvement of biocontrol abilities and development on potential commercial bio-fungicides need to be focused.
● Applications of high-throughput screening of peptaibols produced by Trichoderma provide extended scope of research in bio-medical applications beyond agriculture.
● Effort should also be made to identify plant receptors for Trichoderma elicitors and effectors triggering defense mechanisms in order to reprogramme host’s genetic machinery for understanding interaction of avirulent plant symbionts and host defense.
● Extensive studies regarding identification of diverse physiological traits to upgrade industrial application of Trichoderma for production of antibiotics, enzymes and biofuels as an alternative strategy.
PD: Original Draft Preparation review and edit, LD: review and edit, AP: Wrote existing crop management strategies, volatile and not volatile metabolites, challenges and future prospects, review and edit. All authors contributed to the article and approved the submitted version.
Authors are thankful to Department of Biotechnology, Government of India for providing grant through the sanction no. BT/KIS/123/SP45224/2022 and BT/NER/143/SP42744/2021.
The authors declare that the research was conducted in the absence of any commercial or financial relationships that could be construed as a potential conflict of interest.
All claims expressed in this article are solely those of the authors and do not necessarily represent those of their affiliated organizations, or those of the publisher, the editors and the reviewers. Any product that may be evaluated in this article, or claim that may be made by its manufacturer, is not guaranteed or endorsed by the publisher.
Abbas A., Mubeen M., Zheng H., Sohail M. A., Shakeel Q., Solanki M. K., et al. (2022). Trichoderma spp. genes involved in the biocontrol activity against Rhizoctonia solani. Front. Microbiol. 13. doi: 10.3389/fmicb.2022.884469
Abe N., Murata T., Hirota A. (1998a). Novel DPPH radical scavengers, bisorbicillinol and demethyltrichodimerol, from a fungus. Biosci. Biotechnol. Biochem. 62, 661–666. doi: 10.1271/bbb.62.661
Aboelmagd H. E. (2021). Efficacy of some bio-agents, chemical inducers and fungicides in controlling tomato root rot disease caused by Rhizoctonia solani. Ann. Agric. Sci. Moshtohor. 59 (2), 197–210. doi: 10.21608/assjm.2021.194261
Ahluwalia V., Kumar J., Rana V. S., Sati O. P., Walia S. (2015). Comparative evaluation of two Trichoderma harzianum strains for major secondary metabolite production and antifungal activity. Nat. Prod. Res. 29, 914–920. doi: 10.1080/14786419.2014.958739
Ahmad J. S., Baker R. (1987). Rhizosphere competence of Trichoderma harzianum. Ecol. Epidemiol. 77, 182–189.
Ahmed A. L., Katja F., Wright A. D. (2009). Trichopyrone and other constituents from the marine sponge-derived fungus Trichoderma sp. Zeitschrift für Naturforschung C 64, 186–192. doi: 10.1515/znc-2009-3-406
Ajiboye M. D., Sobowale A. A. (2022). Efficacy of native trichoderma asperellum in managing Alternaria solani causing early blight of Solanum lycopersicum mill. Arch. Phytopath. Plant Prot. 55, 1358–1378. doi: 10.1080/03235408.2022.2097001
Al-Ani L. K. T., Li W. J. (2018). “Trichoderma from extreme environments: Physiology, diversity, and antagonistic activity,” in Extremophiles in Eurasian ecosystems: Ecology, diversity, and applications. microorganisms for sustainability, Eds. Egamberdieva D., Birkeland N. K., Panosyan H. (Singapore: Springer) 8. doi: 10.1007/978-981-13-0329-6_14
Altomare C., Norvell W. A., Bjorkman T., Harman G. E. (1999). Solubilization of phosphates and micronutrients by the plant-growth-promoting and biocontrol fungus Trichoderma harzianum rifai. Appl. Environ. Microbiol. 65, 2926–2933. doi: 10.1128/AEM.65.7.2926-2933.1999
Anderson P. K., Cunningham A. A., Patel N. G., Morales F. J., Epstein P. R., Daszak P. (2004). Emerging infectious diseases of plants: pathogen pollution, climate change and agrotechnology drivers. Trends Ecol. Evol. 19, 535e544. doi: 10.1016/j.tree.2004.07.021
Andrade R., Ayer W. A., Trifonov L. S. (1997). The metabolites of Trichoderma longibrachiatum. III. two new tetronic acids: 5-hydroxyvertinolideand bislongiquinolide. Aust. J. Chem. 50, 255–257. doi: 10.1071/C96103
Asad S. A. (2022). Mechanisms of action and biocontrol potential of Trichoderma against fungal plant diseases - a review. Ecol. Complexcity 49, 100978. doi: 10.1016/j.ecocom.2021.100978
Baldwin J. E., O’Neil I. A., Russell A. T. (1991). Isonitrin a: revision of the structure and total synthesis in racemic form. Synlett 8, 551–552. doi: 10.1055/s-1991-20792
Benitez M. T., Ana M., Rincon M., Carmen L. A., Codon C. (2004). Biocontrol mechanisms of Trichoderma strains. Int. J. Microbol. 7, 249–260.
Benoni H., Taraz K., Korth H., Pulverer G. (1990). Characterization of 6-pentyl-a-pyrone from the soil fungus Trichoderma koningii. Naturwissen 77, 539–540. doi: 10.1007/BF01139267
Berges T., Barreau C., Peberdy J. F., Boddy L. M. (1993). Cloning of an Aspergillus niger invertase gene by expression in Trichoderma reesei. Curr. Genet. 24, 53–59. doi: 10.1007/BF00324665
Biam M., Majumder D. (2019). Biocontrol efficacy of Trichoderma isolates against tomato damping off caused by Pythium spp. and Rhizoctonia solani (Kuhn.). Intr. J. Chem. Stud. 7 (3), 81–89.
Bigirimana J., De Mayer G., Poppe J., Elad Y., Hofte M. (1997). Induction of systemic resistance on bean (Phaseolus vulgaris) by Trichoderma harzianum. Med. Fac. Landbouww Univ. Gent. 62, 1001–1007.
Bisby G. R. (1939). Trichoderma viride pers. ex fries, and notes on Hypocrea. Trans. Br. Mycol. Soc. 23, 149–168. doi: 10.1016/S0007-1536(39)80020-1
Bissett J. (1991). A revision of the genus Trichoderma II. intragenic classification. Can. J. Bot. 60, 2357–2372. doi: 10.1139/b91-297
Biswas D., Dutta P. (2019). Silver and zinc oxide nanoparticles are compatible with fungal biocontrol agents. Int. J. Chem. Stud. 7 5), 2159–2162.
Bora M. (2017). Aggrieved Trichoderma harzianum in management of sclerotinia rot of carrot in organically amended soil. Ph.D. thesis (Jorhat, Assam: Assam Agric. University).
Brody H., Maiyuram S. (2009). RNAi-mediated genes silencing of highly expressed genes in the industrial fungi Trichoderma reesei and Aspergillus niger. ind. Biotechnol. 5. 53–60. doi: 10.1089/ind.2009.5.53
Brotman Y., Briff E., Viterbo A., Chet I. (2008). Role of swollenin, an expansion-like protein form Trichoderma, in plant root colonization. Plant Physiol. 147, 779–789. doi: 10.1104/pp.108.116293
Brotman Y., Landau U., Cuadros-Inostroza A., Takayuki T., Fernie A. R., Chet I., et al. (2013). Trichoderma-plant root colonization: escaping early plant defense responses and activation of the antioxidant machinery for saline stress tolerance. PloS Pathog. 9, e1003221. doi: 10.1371/journal.ppat.1003221
Brotman Y., Lisec J., Meret M., Chet I., Willmitzer L., Viterbo A.. (2012). Transcript and metabolite analysis of the Trichoderma induced systemic resistance response to Pseudomonas syringae in Arabidopsis thaliana. Microbiol. 158, 139–146. doi: 10.1099/mic.0.052621-0
Buchert J., Oksanen T., Pere J., Siika-Aho M., Suurnakki A., Viikari L. (1998). “Application of Trichoderma reesei enzymes in the pulp and paper industry,” in Trichoderma and Gliocladium. Eds. Harman G. E., Kubeick C. P. (London: Taylor and Francis) 343–363.
Burketova L., Trda L., Ott P. G., Valentova O. (2015). Bio-based resistance inducers for sustainable plant protection against pathogens. Biotechnol. Adv. 33 (6), 994–1004. doi: 10.1016/j.biotechadv.2015.01.004
Caledron A. A., Zapata J. M., Munoz R., Pedreno M. A., Barcelo A. R. (1993). Resveratrol production as a part of the hypersensitive-like response of grapevine cells to an elicitor from Trichoderma viride. New Phytol. 124, 455–463. doi: 10.1111/j.1469-8137.1993.tb03836.x
Camacho-Luna V., Flores-Moctezuma H. E., Rodríguez-Monroy M., Montes-Belmont R., Sepúlveda-Jiménez G. (2021). Induction of the defense response of onion plants in interaction with Trichoderma asperellum and Alternaria porri. Rev. Mexicana Cienc. Agrícolas 12. doi: 10.29312/remexca.v12i4.2683
Carvalho F. P. (2006). Agriculture, pesticides, food security and food safety. Environ. Sci. Pol. 9, 685–692. doi: 10.1016/j.envsci.2006.08.002
Chang Y.-C., Chang Y.-C., Baker R., Kleifeld O., Chet I. (1986). Increased growth of plants in the presence of the biological control agent Trichoderma harzianum. Plant Dis. 70, 145–148. doi: 10.1094/PD-70-145
Chang P. F. L., Xu Y., Barsimhan M. L., Cheah K. T., Durzo M. P., Damsz B., et al. (1997). Induction of pathogen resistance and pathogenesis-related genes in tobacco by a heat-stable Trichoderma mycelial extract and plant signal messengers. Physiol. Plant 100, 341–352. doi: 10.1111/j.1399-3054.1997.tb04792.x
Chantrapromma S., Jeerapong C., Phupong W., Quah C. K., Fun H. K. (2014). Trichodermaerin: A diterpene lactone from Trichoderma asperellum. Acta Crystallogr. 70, 408–409. doi: 10.1107/S1600536814004632
Chao W. L., Nelson E. B., Harman G. E., Hoch H. C. (1986). Colonization of the rhizosphere by biological control agents applied to seeds. Phytopathol 76, 60–65. doi: 10.1094/Phyto-76-60
Chavez J. R., Raja H. A., Graf T. N., Gallagher J. M., Metri P., Xue D., et al. (2017). Prealamethicin F50 and related peptaibols from Trichoderma arundinaceum: Validation of their authenticity via in situ chemical analysis. RSC Adv. 7, 45733–45741. doi: 10.1039/C7RA09602J
Chen J. L., Liu K., Miao C. P., Guan H. L., Zhao L. X., Sun S. Z. (2015). Chemical constituents with siderophores activities from Trichoderma koningiopsis YIM PH30002. Nat. Prod. Res. Dev. 27, 1878–1883.
Chen L., Zhong P., Pan J. R., Zhou K. J., Huang K., Fang Z. X., et al. (2013). Asperelines G and h, two new peptaibbols from the marine-deriver fungus Trichoderma asperellum. Heterocycles 87, 645–655. doi: 10.3987/COM-12-12644
Chou C. H. (2010). Role of allelopathy in sustainable agriculture: use of allelochemicals as naturally occurring bio-agrochemicals. Allelopathy J. 25, 3–16.
Clarkson J. P., Mead A., Payne T., Whipps J. M. (2004). Effect of environmental factors and Sclerotium cepivorum isolates on sclerotial degradation and biological control of white rot by Trichoderma. Plant Pathol. 53, 353–362. doi: 10.1111/j.0032-0862.2004.01013.x
Contreras-Cornejo H. A., Macias-Rodriguez L., Beltran-Pena E., et al. (2011). Trichoderma-induced plant immunity likely involves both hormonal and camalexin dependent mechanisms in A. thaliana and confers resistance against necrotrophic fungi Botrytis cinerea. Plant Signal Behav. 6, 1554–1563. doi: 10.4161/psb.6.10.17443
Contreras-Cornejo H. A., Macías-Rodríguez L., Del-Val E., Larsen J (2016). Ecological functions of Trichoderma spp. and their secondary metabolites in the rhizosphere: interactions with plants. FEMS Microbiol. Ecol. 92, fiw036
Corke A. T. K., Hunter T. (1979). Biocontrol of Nectaria galligena infections of pruning wounds of apple shoots. J. Horticul. Sci. 54, 47. doi: 10.1080/00221589.1979.11514847
Da Costa A. C., Miranda R. F., Costa F. A., Ulhoa C. J. (2021). Potential of Trichoderma piluliferum as a biocontrol agent of Colletotrichum musae in banana fruits. Biocatalysts Agric. Biotechnol. 34, 102028. doi: 10.1016/j.bcab.2021.102028
Das B. C., Das B. K., Dutta P., Sarmah D. K. (2006). Bioformulation of Trichoderma harzainum rifai for management of soybean stem rot caused by Rhizoctonia solani Kuhn. J. Biol. Cont. 20 (1), 57–64.
Das B. C., Hazarika D. K. (2000). Biological management of sheath blight of rice. Ind. Phytopathol. 53, 433–435.
Das B. C., Khairuzzaman A. S. M., Islam, Dutta P. (1997). “Biological seed treatment for management od sheath blight of rice,” in proceedings of International Symposium on Rainfed Rice Production Strategy for Twenty First Century, Assam Agricultural University, Jorhat, 25-27 315–319.
De Meyer G., Bigirimana J., Elad Y., Hofte M. (1998). Induced systemic resistance in Trichoderma harzianum T39 biocontrol of Botrytis cinerea. Eur. J. Plant Pathol. 104, 279–286. doi: 10.1023/A:1008628806616
De Waard M. A., Georgopoulos S. G., Hollomon D. W., Ishii H., Leroux P., Ragsdale N. N., et al. (1993). Chemical control of plant diseases: Problems and prospects. Annu. Rev. Phytopathol. 31, 403e421. doi: 10.1146/annurev.py.31.090193.002155
Ding G., Wang H. L., Li L., Chen A. J., Chen L., Chen H., et al. (2012). Trichoderones a and b: Two pentacyclic cytochalasans from the plant endophytic fungus Trichoderma gamsii. Eur. J. Org. Chem. 2012, 2516–2519. doi: 10.1002/ejoc.201200053
Domsch K. H., Gams W., Anderson T. H. (1980). Compendium of soil fungi (London, New York: Academic Press).
Druzhinina I. S., Kopchinskiy A. G., Kubicek C. P. (2006). The first 100 Trichoderma species characterized by molecular data. Mycol-science 46, 55–64. doi: 10.1007/S10267-006-0279-7
Druzhinina I., Seidl-Seiboth V., Herrera-Estrella A., Horwitz B. A., Kenerley C. M., Monte E., et al. (2011). Trichoderma: the genomics of opportunistic success. Nat. Rev. Microbiol. 9, 749–759. doi: 10.1038/nrmicro2637
Dubey S. C., Tripathi A., Tak R., Devi S. I. (2020). Evaluation of bio-formulations of fungal and bacterial biological control agents in combination with fungicide in different mode of application for integrated management of tomato wilt. Indian Phytopath. 73 (3), 425–432. doi: 10.1007/s42360-020-00255-6
Dunne C., Delany I., Gara F. O. (1996). Mechanism involved in biocontrol by microbial inoculants. Agronomie 16, 721–729. doi: 10.1051/agro:19961017
Dutta P., Bhowmick P., Boruah S. (2018). Effect of extracellular metabolites of Trichoderma pseudokoningii on radial growth of Fusarium oxysporum, Colletotrichum capsici, Rhizoctonia solani and Sclerotinia sclerotiorum. Int. J. Curr. Micrbiol. App. Sci. 7, 874–879. doi: 10.20546/ijcmas.2018.701.106
Dutta A., Borah A., Mahanta B., Dutta P. (2020). Integration of biocontrol agents and chemical for the management of meloidogyne incognita and Sclerotinia sclerotiorum complex on French bean. J. Entomol. Zool. Stud. 8, 1546–1551.
Dutta P., Das B. C. (1999a). Effect of seed pelleting and soil application of Trichoderma harzianum in the management of stem rot of soybean. J. Mycol. Pl. Path. 29 (2), 317–322.
Dutta P., Das B. C. (1999b). Control of Rhizoctonia solani in soybean (Glycine max) by farm yard manure culture of Trichoderma harzianum. Ind. J. Agril. Sci. 69 (8), 317–322.
Dutta P., Das B. C. (2002). Management of collar rot of tomato by Trichoderma spp. and chemicals. Ind. Phytopath. 55 (2), 235–237.
Dutta P., Das B. C. (2009). Efficacy of talc-based formulation of Trichoderma harzianum for management of white mold of French bean (Phaseolus vulgaris). J. Mycol. Pl. Pathol. 39 2), 278–282.
Dutta P., Das B. C., Hazarika D. K. (2000). Integrated management of stem rot of soybean. J. Biol. Contr. 14, 67–69.
Dutta P., Deb L., Gogoi J., Mahanta M., Kumari A., Yasin A., et al. (2021). “UmTricho” a, liquid bioformulation of indigenous strains of Trichoderma harzianum effectively managed the tikka disease (Cercospora spp.) of groundnut, Arachis hypogea L. Biol. Forum 13 (2), 529–535.
Dutta P., Deb L., Tombisana Devi R. K., Pathak M., Patidar R. K., Chauhan J. K. (2020). Bioformulations of indigenous biocontrol agents- a potential alternative for pests and disease management in Meghalaya. Just Agric. 1 (2), 34–42.
Dutta P., Das B. C., Islam M. (2008). Eco-friendly management of sclerotinia rot of French bean. J. Biol. Control. 22 (2), 405–410.
Dutta P., Kakati N., Das A., Kaushik H., Boruah S., Bhowmick P., et al. (2017). Trichoderma pseudokoningii showed compatibility with certain commonly used inorganic pesticides, fertilizers and stickers cum spreaders. Int. J. Curr. Microbiol. Appl. Sci. 6 (2), 140–146. doi: 10.20546/ijcmas.2017.602.020
Dutta P., Kaushik H., Puzari K. C., Bhuyan R. P. (2016). “Eco-friendly management of tea diseases in current scenario of climate change,” in Dynamic in crop protection and climate change (New Delhi, India: Studera Press), 326–340.
Elad Y., Freeman S., Monte E. (2000). Biocontrol agents: mode of action and interactions with other means of control. IOBC Sevilla Espana, 24.
El-Benawy N. M., Abdel-Fattah G. M., Ghoneem K. M., Shabana Y. M. (2020). Antimicrobial activities of Trichoderma atroviride against common bean seed-borne Macrophomina phaseolina and Rhizoctonia solani. Egyptian J. Basic Appl. Sci. 7, 267–280. doi: 10.1080/2314808X.2020.1809849
Elshahawy I. E., El-Mohamedy R. S. (2019). Biological control of Pythium damping-off and root-rot diseases of tomato using Trichoderma isolates employed alone or in combination. J. Plant Pathol. 101 (3), 597–608. doi: 10.1007/s42161-019-00248-z
Evidente A., Cabras A., Maddau L., Marras F., Andolfi A., Melck D., et al. (2006). Viridenepoxydiol, a new pentasubstituted oxiranyldecene produced by Trichoderma viride. J. Agric. Food Chem. 54, 6588–6592. doi: 10.1021/jf060713m
Evidente A., Cabras A., Maddau L., Serra S., Andolfi A., Motta A. (2003). Viridepyronone, a new antifungal 6-substituted 2H-pyran-2-one produced by Trichoderma viride. J. Agric. Food Chem. 51, 6957–6960. doi: 10.1021/jf034708j
Evidente A., Ricciardiello G., Andolfi A., Sabatini M. A., Ganassi S., Altomare C., et al. (2008). Citrantifidiene and citrantifidiol: bioactive metabolites produced by Trichoderma citrinoviride with potential antifeedant activity toward aphids. J. Agric. Food Chem. 56, 3569–3573. doi: 10.1021/jf073541h
Faulds C. B., Robertson J. A., Waldron K. W. (2008). Effect of pH on the solubilization of brewer’s spent grain by microbial carbohydrases and proteases. J. Agric. Food Chem. 27, 7038–7043. doi: 10.1021/jf800433c
Fraaije B. A., Cools H. J., Fountaine J., Lovell D. J., Motteram J., West J. S, et al. (2005). Role of ascospores in further spread of QoI-resistant cytochrome b alleles (G143A) in field populations of Mycosphaerella graminicola. Phytopathol 95, 933–941. doi: 10.1094/PHYTO-95-0933
Fujita T., Takaishi Y., Takeda Y., Fujiyama T., Nishi T. (1984). Fungal metabolites. II. structural elucidation of minor metabolites, valinotricin, cyclonerodiol oxide, and epicyclonerodiol oxide, from Trichoderma polysporum. Chem. Pharm. Bull. 32, 4419–4425. doi: 10.1248/cpb.32.4419
Fujta T., Wada S. I., Iida A., Nishimura T., Kanai M., Toyama N. (1994). Fungal metabolites. XIII. isolation and structural elucidation of new peptaibols, trichodecenins-I and II, from Trichoderma viride. Chem. Pharm. Bull. 42, 489–494. doi: 10.1248/cpb.42.489
Galante Y. M., De Conti A., Monteverdi R. (1998b). “Application of trichoderma enzymes in the food and feed industries,” in Trichoderma and Gliocladium. Eds. Harman G. E., Kubeick C. P. (London: Taylor and Francis) 327–342.
Garnica-Vegara A., Barrera-Ortiz S., Munoz-Parra E., Raya-González J., Méndez-Bravo A., Macías-Rodríguez L, et al. (2015). The volatile 6-pentyl-2H-pyran-2-one from Trichoderma atroviride regulates Arabidopsis thaliana root morphogenesis via auxin signalling and ETHYLENE INSENSITIVE 2 functioning. New Phytopathol. 209, 1496–1512. doi: 10.1111/nph.13725
Garo E., Starks C. M., Jensen P. R., Fenical W., Lobkovsky E., Clardy J. (2003). Trichodermamides a and b, cytotoxic modified dipeptides from the marine-derived fungus Trichoderma virens. J. Nat. Prod. 66, 423–426. doi: 10.1021/np0204390
Geremia R. A., Golgman G. H., Jacobs D., Ardiles W., Vila S. B., Montagu M. V.. (1993). Molecular characterization of the proteinase-encoding gene, prb1, related to mycoparasitism by Trichoderma harzianum. Mol. Mcrobiol. 8, 603–613. doi: 10.1111/j.1365-2958.1993.tb01604.x
Ghazanfar M. U., Raza M., Raza W. (2018). Effect of physiological parameters on mass production of Trichoderma species. Pakistan J. Phytopathol. 30. doi: 10.33866/phytopathol.030.01.0447
Gogoi R., Saikia M., Helim R., Ullah Z. (2007). Management of potato diseases using Trichoderma viride formulations. J. Mycol. Plant Pathol. 37, 227–230.
Gutter Y. (1957). Effect of light on sporulation of Trichoderma viride pers. Ex. Fries. Bull. Res. Counc. Israel Sect. D. Bot. 5, 273–286.
Gu X., Wang R., Sun Q., Wu B., Sun J. Z. (2020). Four new species of Trichoderma in the harzianum clade from northern china. MycoKeys 73, 109–132. doi: 10.3897/mycokeys.73.51424
Haggag W. M., Abo-Sedera S. A. (2005). Characteristics of three Trichoderma species in peanut haulms compost involved in biocontrol of cumin wilt disease. Int. J. Agric. Biol. 7, 222–229.
Harman G. E., Howell C. R., Viterbo A., Chet I., Na Lorito M. (2004). Trichoderma species-opportunistic, avirulent plant symbionts. Nat. Rev. Microbiol. 2, 43–56. doi: 10.1038/nrmicro797
Hartl L., Seiboth B. (2005). Sequential gene deletions in Hyprocrea jecorina using a single blaster cassette. Curr. Genet. 48, 204–211. doi: 10.1007/s00294-005-0011-8
Hasan S., Gupta G., Anand S., Chaturvedi A., Kaur H. (2013). Biopotential of microbial antagonists against soilborne fungal plant pathogens. Int. J. Agric. Food Sci. Technol. 4, 37–39.
Hermosa M. R., Grondona I., Iturriaga E. A., Diaz-Minguez J. M., Castro C., Monte E., et al. (2000). Molecular characterization and identification of biocontrol isolates of trichoderma spp. Appl. Environ. Microbiol. 66, 1890–1898. doi: 10.1128/AEM.66.5.1890-1898.2000
Heflish A. A., Abdelkhalek A., Al-Askar A. A., Behiry S. I. (2000). Protective and curative effects of Trichoderma asperelloides Ta41 on tomato root rot caused by Rhizoctonia solani Rs33. Agronomy 11, 1162
Howell C. R. (2003). Mechanisms employed by Trichoderma species in the biological control of plant diseases: the history and evolution of current concepts. Plant Dis. 87, 4–10. doi: 10.1094/PDIS.2003.87.1.4
Howell C. R., Stipanovic E. R. D. (1983). Glioviridin, a new antibiotic from Gliocladium virens and its role in the biological control of Pythium ultimum. Can. J. Microbiol. 29, 321–324. doi: 10.1139/m83-053
Huang Q., Tezuka Y., Hatanaka Y., Kikuchi T., Nishi A., Tubaki K. (1995). Studies on metabolites of mycoparasitic fungi. III. new sesquiterpene alcohol from Trichoderma koningii. Chem. Pharm. Bull. 43, 1035–1038. doi: 10.1248/cpb.43.1035
Hu X., Gong M. W., Zhang W. W., Zheng Q. H., Liu Q. Y., Chen L., et al. (2014). Novel cytotoxic metabolites from the marine-derived fungus Trichoderma citrinoviride. Heterocycles 89, 189–196. doi: 10.3987/COM-13-12874
Ishii H. (2006). Impact of fungicide resistance in plant pathogens on crop disease control and agricultural environment. Jpn. Agric. Res. Q. 40, 205e211. doi: 10.6090/jarq.40.205
Jalal M. A., Love S. K., van der Helm D. (1986). Siderophore mediated iron (III) uptake in Gliocladium virens. properties of cis-fusarinine, transfusisinine, dimeric acid, and their ferric complexes. J. Inorg. Biochem. 28, 417–430. doi: 10.1016/0162-0134(86)80027-7
Jamil A. (2021). Antifungal and plant growth promoting activity of trichoderma spp. against Fusarium oxysporum f. sp. lycopersici colonizing tomato 61. J. Plant Prot. Res. 61, 243–253.
Ji Z. L., Ma D. S., Miao F. P. (2014). Chemical constituents from Trichoderma longibrachiatum, an endophytic fungus derived from marine green alga codium fragile. J. Shenyang Univ. 26, 277–280.
Kamala T., Devi S. I. (2012). Biocontrol properties of indigenous Trichoderma isolates from north-east India against Fusarium oxysporum and Rhizoctonia solani. Afr. J. Biotechnol. 11 (34), 8491–8499. doi: 10.5897/AJB11.1938
Kamala T., Indira S. (2011). Evaluation of indigenous Trichoderma isolates from manipur as biocontrol agent against Pythium aphanidermatum on common beans. 3 Biotech. 1, 217–225. doi: 10.1007/s13205-011-0027-3
Kandasamy S., Suresh M., Ramachandran C., Elango J., Ravi Shankar B. Y., Deepthi M., et al. (2018). Novel metabolites from Trichoderma atroviride against human prostate cancer cells and their inhibitory effect on Helicobacter pylori and Shigella toxin producing Escherichia coli. Microb. Pathogen. 126, 19–26.
Kangjam B., Rajesh T., Majumder D., Devi K. J. (2017). Evaluation of plant extracts, biocontrol agents and fungicides agents against the growth of turmeric leaf spot pathogen, Colletotrichum capsici under in vitro condition. Environ. Ecol. 35 (2B), 1173–1178.
Kareem F. H., Matloob A. A. (2019). Efficiency of some biological control agents and plant extracts against Fusarium solani causing agent of damping off disease on tomato. Plant Arch. 19 (2), 937–942.
Khan P., Bora L. C., Bora P., Taludar K., Kataky L. (2018). Efficacy of microbial consortia against bacterial wilt caused by Ralstonia solanacearum in hydroponically grown lettuce plant. Int. J. Curr. Microbiol. App. Sci. 7 (6), 3046–3055. doi: 10.20546/ijcmas.2018.706.358
Khatso K., Tiameren Ao N. (2013). Biocontrol of rhizome rot disease of ginger (Zingiber officinale rosc.). Int. J. Bio-res. Str. Mang. 4 (2), 317–321.
Kithan C., Daiho L. (2014). In vitro evaluation of botanicals, bio-agents and fungicides against leaf blight of Etlingera linguiformis caused by Curvularia lunata var. aeria. J. Plant Pathol. Microbiol. 5, 3. doi: 10.4172/2157-7471.1000232
Klein D., Eveleigh E. (1998). “Ecology of Trichoderma,” in Trichoderma and Gliocladium. Eds. Kubeick C. P., Harman G. E. (London, Bristol, PA: Taylor and Francis) 57–69.
Koijam K., Sinha B. (2018). Antagonistic potential and molecular characterization of trichoderma spp. against Rhizoctonia solani infecting ghost pepper in manipur. Int. J. Curr. Microbiol. App. Sci. 7 (2), 2085–2093.
Kopchinskiy A., Komon M., Kubeick C. P., Druzhinina I. S. (2005). TrichoBLAST: a multilocus database for Trichoderma and Hypocrea identifications. Mycol. Res. 109, 658–660. doi: 10.1017/S0953756205233397
Korpi A., Järnberg J., Pasanen A. L. (2009). Microbial volatile organic compounds. Crit. Rev. Toxicol. 39, 139–193. doi: 10.1080/10408440802291497
Kubicek C. P., Bissett J., Druzhinina I., Kulling-Gradinger C., Szakas G. (2003). Genetic and metabolic diversity of Trichoderma: a case study on south-East Asian isolates. Fungal Genet. Biol. 38, 310–319. doi: 10.1016/S1087-1845(02)00583-2
Kubicek C. P., Herra-Estrella A., Seidl-Seiboth V., Martinez D. A., Druzhinina I. S., Thon M, et al. (2011). Comparative genome sequence analysis underscores mycoparasitism as the ancestral life style of Trichoderma. Genome Biol. 12, R40. doi: 10.1186/gb-2011-12-4-r40
Kubodera T., Yamashita N., Nishimura A. (2002). Transformation of aspergillus sp. and Trichoderma reesei using the pyrithiamine resistance gene (ptrA) of Aspergillus oryzae. Biosci. Biotechnol. Biochem. 66, 404–406. doi: 10.1271/bbb.66.404
Kuhls K., Lieckfeldt E., Samuels G. J., Kovacs W., Meyer W., Petrini O., et al. (1996). Molecular evidence that the asexual industrial fungus Trichoderma reesei is a clonal derivative of the ascomycete Hypocrea jecorina. Proc. Natl. Acad. Sci. U.S.A. 93, 7755–7760. doi: 10.1073/pnas.93.15.7755
Kulling-Gradiner C. M., Szakacs G., Kubicek C. P. (2002). Phylogeny and evolution of the genus Trichoderma: multigene approach. Mycol. Res. 106, 757–767. doi: 10.1017/S0953756202006172
Kulling C., March R., Lorito M., Kubicek C. P. (2000). Enzyme diffusion from Trichoderma atriviride (T. harzianum P1) to Rhizoctonia solani is a prerequisite for triggering of Trichoderma ech42 gene expression before mycoparasitic contact. Appl. Environ. Microbiol. 66, 2232–2234. doi: 10.1128/AEM.66.5.2232-2234.2000
Kumar V., Verma D. K., Pandey A. K., Srivastava S. (2019). “Trichoderma spp.: Identification and characterization for pathogenic control and its potential application,” in Microbiology for sustainable agriculture, soil health and environment protection (USA: Apple Academic Press), 223–258.
Kumhar K. C., Kumar K., Arora I., Bhatia A. K., Batra V. K., Raj H. (2022). Management of tomato damping-off disease in the nursery using of Trichoderma asperellum. Int. J. Econom. Plants. 9, 145–151. doi: 10.23910/2/2022.0427c
Lalngaihawmi, Bhattacharya A. (2019). Study on different action of potential Trichoderma spp. from banana rhizosphere against Fusarium oxysporum f.sp. cubense. Int. J. Curr. Microbiol. App. Sci. 8 (1), 1028–1040.
Lee S. H., Hensens O. D., Helms G. L., Liesch J. M., Zink D. L., Giacobbe R. A., et al. (1995a). L-735,334, a novel sesquiterpenoid potassium channel-agonist from Trichoderma virens. J. Nat. Prod. 58, 1822–1828. doi: 10.1021/np50126a004
Liang X. R. (2016). Secondary metabolites from three marine-derived Trichoderma fungi and their bioactivities (Yantai, China: Yantai Institute of Coastal Zone Research, Chinese Academy of Sciences).
Lida A., Uesato S., Shingu T., Nagaoka Y., Kuroda Y., Fujita T. (1993). Fungal metabolites. part 7. solution structure of an antibiotic peptide trichosporin b-V, from Trichoderma polysporum. J. Chem. Soc Perkin Trans. 24, 375–379.
Lin Y. R., Lo C. T., Li S. Y., Peng K. C. (2012). Involvement of pachybasin and emodin in self-regulation of Trichoderma harzianum mycoparasitic coiling. J. Agric. Food Chem. 60, 2123–2128. doi: 10.1021/jf202773y
Liu S. Y., Lo C. T., Shibu M. A., Leu Y. L., Jen B. Y., Peng K. C. (2009). Study on the anthrqrquinones separated from the cultivation of Trichoderma harzianum strain Th-R16 and their biological activity. J. Agric. Food Chem. 57, 7288–7292. doi: 10.1021/jf901405c
Lorito M., Woo S. L., D’Ambrosio M., Harman G. E., Hayes C. K., Kubeick C. P., et al. (1996). Synergistic interaction between cell wall degrading enzymes affecting compounds. Mol. Plant Microbe Interact. 9, 206–213. doi: 10.1094/MPMI-9-0206
Lorito M., Woo S. L., Fernandez I. G., Collucci G., Harman G. E., Pintor-Toros J. A., et al. (1998). Genes from mycoparasitic fungi as source for improving plant resistance to fungal pathogens. Proc. Natl. Acad. Sci. U.S.A. 95, 7860–7865. doi: 10.1073/pnas.95.14.7860
Lu X., Tian L., Chen G., Xu Y., Wang H. F., Li Z. Q., et al. (2012). Three new compounds from the marine-derived fungus Trichoderma atroviride G20-12. J. Asian. Nat. Prod. Res. 14, 647–651. doi: 10.1080/10286020.2012.682256
Macias F. A., Varela R. M., Simonet A. M., Cutler H. G., Cutler S. J., Eden M. A., et al. (2000). Bioactive carotanes from Trichoderma virens. J. Nat. Prod. 63, 1197–1200. doi: 10.1021/np000121c
Madhavi G. B., Bhattiprolu S. L., Reddy V. B. (2011). Compatibility of biocontrol agent Trichoderma viride with various pesticides. J. Hortl. Sci. 6 (1), 71–73.
Malmierca M. G., Cardoza R. E., Alexander N. J., McCormick S. P., Hermosa R., Monte E., et al. (2012). Involvement of Trichoderma trichothecenes in the biocontrol activity and induction of plant defense-related genes. Appl. Environ. Microbiol. 78 (14), 4856–4868. doi: 10.1128/AEM.00385-12
Martinez D., Berka R. M., Henrissat B., Saloheimo M., Arvas M., Baker S. E, et al. (2008). Genome sequencing and analysis of the biomass-degrading fungus Trichoderma reesei (syn. Hypocrea jecorina). Nat. Biotechnol. 26, 553–560. doi: 10.1038/nbt1403
McLean K. L., Swaminathan J., Frampton C. M., Hunt J. S., Ridgway H. J., Stewart A. (2005). Effect of formulation on the rhizosphere competence and biocontrol ability of Trichoderma atroviride C52. Plant Pathol. 54, 212–218. doi: 10.1111/j.1365-3059.2005.01158.x
McMullin D. R., Renaud J. B., Barasubiye T., Sumarah M. W., David M. J. (2017). Metabolites of Trichoderma species isolated from damp building materials. Can. J. Microbiol. 63, 621–632. doi: 10.1139/cjm-2017-0083
Mech S. (2004). UV-Radiated mutant of Trichoderma harzianum Rifai and its carrier-based formulation for management of white mold of French bean (Jorhat, Assam: Assam Agric. University).
Meher J., Rajput R. S., Bajpai R., Teli B., Sarma B. K. (2020). “Trichoderma: A globally dominant commercial biofungicide,” in Trichoderma: Agricultural applications and beyond, vol. 61 . Eds. Manoharachary C., Singh H. B., Varma A. (Switzerland:Springer, Cham) pp. 195–208. doi: 10.1007/978-3-030-54758-5_9
Mendoza-Mendoza A., Pozo M. J., Grzegorski D., Martinez P., Garcia J. M., Olmedo-Monfil V., et al. (2003). Enhanced biocontrol activity of Trichoderma through inactivation of a mitogen-activated protein kinase. Proc. Natl. Acad. Sci. U.S.A. 100, 15965–15970. doi: 10.1073/pnas.2136716100
Meng J. J., Wang B., Cheng W. (2017). Study on the secondary metabolites of Trichoderma saturnisporum. chin. J. Mar. Drugs 36, 27–31.
Metz N., Hausladen H. (2022). Trichoderma spp. as potential biological control agent against Alternaria solani in potato. Biol. Contr. 166, 104820.
Miao F.-P., Liang X.-R., Yin X.-L., Wang G., Ji N.-Y. (2012). Absolute configurations of unique harziane diterpenes from Trichoderma species. Org. Lett. 14 (15), 3815–3817. doi: 10.1021/ol3014717
Mikaberidze A., McDonald B. A., Bonhoeffer S. (2014). Can high-risk fungicides be used in mixtures without selecting for fungicide resistance? Anal. Theor. Plant Pathol. 104 (4), 324–331.
Murphy B. R., Doohan F. M., Hodkinson T. R. (2018). From concept to commerce: Developing a successful fungal endophyte inoculant for agricultural crops. J. Fungi 4 (1), 24e35. doi: 10.3390/jof4010024
Naglot A., Gosawami S., Rahman I., Shrimali D. D., Yadav K., Gupta V. K., et al. (2015). Antagonistic potential of native Trichoderma viride strain against potent tea fungal pathogens in north east India. Plant Pathol. J. 31 (3), 278–289. doi: 10.5423/PPJ.OA.01.2015.0004
Nemčovič M., Jakubíková L., Víden I., Vladimír F. (2008). Induction of conidiation by endogenous volatile compounds in Trichoderma spp. FEMS Microbiol. Lett. 284, 231–236. doi: 10.1111/j.1574-6968.2008.01202.x
Nielsen K. F., Grafenhan T., Zafari D., Thrane U. (2005). Trichothecene production by Trichoderma brevicompactum. J. Agric. Food Chem. 53, 8190–8196. doi: 10.1021/jf051279b
Olmedo-Monfil V., Casas-Flores S (2014). “Molecular mechanisms of biocontrol in Trichoderma spp. and their applications in agriculture,” in. Biotechnology and Biology of Trichoderma edsGupta. V., Schmoll M., Herrera-Estrella A., Upadhyay R., Druzhinina I., Tuohy M., et al (Amsterdam:Elservier), 429–453.
Olowe O. M., Nicola L., Asemoloye M. D., Akanmu O., Babalola O. (2022). Trichoderma: Potential bio-resource for the management of tomato root rot diseases in Africa. Microbiol. Res. 257, 126978. doi: 10.1016/j.micres.2022.126978
Omomowa O. P., Fadiji A. E., Omomowo I. (2018). Assessment of bio-efficacy of Glomus versiforme and Trichoderma harzianum in inhibiting powdery mildew disease and enhancing the growth of cowpea. Ann. Agric. Sci. 63, 9–17. doi: 10.1016/j.aoas.2018.03.001
Pandey A. K., Burlakoti R. R., Kenyon L., Nair R. (2018). Perspectives and challenges for sustainable management of fungal diseases of mungbean (Vigna radiata (L.) R. Wilczek var. radiata): A Review. Front. Environ. Sci 6, 53.
Pandey A. K., Deka B., Varshney R., Cheramgoi E. C., Babu A. (2021). Do the beneficial fungi manage phytosanitary problems in the tea agro-ecosystem? Biocontrol 66, 445–462. doi: 10.1007/s10526-021-10084-9
Papavizas G. C. (1982). Survival of Trichoderma harzianum in soil and in pea and bean rhizosphere. Phytopathol 72, 121–125. doi: 10.1094/Phyto-72-121
Papavizas G. C. (1985). Trichoderma and Gliocladium: Biology, ecology and potential for biocontrol. Ann. Rev. Phytopathol. 23, 23–54. doi: 10.1146/annurev.py.23.090185.000323
Papavizas G. C., Dunn M. T., Lewis J. A., Beagle-Ristaino J. (1984). Liquid fermentation technology for experimental production of biocontrol fungi. Phytopathol 74, 1171–1175. doi: 10.1094/Phyto-74-1171
Papavizas G. C., Lumsden R. D. (1982). Improved medium for isolation of Trichoderma spp. from soil. Plant Dis. 66, 1019–1020. doi: 10.1094/PD-66-1019
Pappu L. (2018). Enhanced rhizosphere competence of Trihoderma viride in solid state fermentation on corn cob residue. Arch. Phytopathol. Plant Protec 51, 505–529. doi: 10.1080/03235408.2018.1490236
Pentilla M. (1998). “Heterologous protein production in trichoderma,” in Trichoderma andGliocladium. Eds. Harman G. E., Kubeick C. P. (London: Taylor and Francis), 365–382.
Penttila M., Nevalainen H., Ratto M., Salminen E., Knowles J. (1987). A versatile transformation system for the cellulolytic filamentous fungus Trichoderma reesei. Gene 61, 155–164. doi: 10.1016/0378-1119(87)90110-7
Perez-Gonzalez J. A., Gonzalez R., Querol A., Sendra J., Ramon D. (1993). Construction of a recombinant wine yeast strain expressing β-(1,4)-endoglucanase and its use in micro vinification processes. Appl. Environ. Microbiol. 59, 2801–2806. doi: 10.1128/aem.59.9.2801-2806.1993
Phuwapraisirisan P., Rangsan J., Siripong P., Tip-Pyang S. (2006). 9-epi-Viridiol, a novel cytotoxic furanosteroid from soil fungus Trichoderma virens. Nat. Prod. Res. 20 (14), 1321–1325. doi: 10.1080/14786410601101969
Polizzi V., Adams A., Picco A. M., Adriaens E., Lenoir J., Van Peteghem C., et al. (2011). Influence of environmental conditions on production of volatiles by Trichoderma atroviride in relation with the sick building syndrome. Build. Environ. 46 (4), 945–954. doi: 10.1016/j.buildenv.2010.10.024
Poveda J. (2021). Biological control of Fusarium oxysporum f. sp. ciceri and Ascochyta rabiei infecting protected geographical indication fuentesaúco-chickpea by Trichoderma species. Eur. J. Plant Pathol. 160, 825–840. doi: 10.1007/s10658-021-02286-9
Promwee A., Intana W. (2022). Trichoderma asperellum (NST-009): A potential native antagonistic fungus to control cercospora leaf spot and promote the growth of ‘Green oak’ lettuce (Lactuca sativa L.) cultivated in the commercial NFT hydroponic system. Plant Protect. Sci. 58, 139–149. doi: 10.17221/69/2021-PPS
Puyam A. (2016). Advent of Trichoderma as a biocontrol agent- a review. J. Appl. Nat. Sci. 8 (2), 1100–1109.
Reese E. T. (1976). History of the cellulase program at the U.S. army natick development center. Biotechnol. Bioeng. Symp. 6, 9–20.
Reichenbach H., Forche E., Gerth K., Irschik H., Kunze B., Sasse F., et al. (1990). Fungicidal steroids from Trichoderma. Ger. Offen DE Patent 3823068 11.
Reino J. L., Guerrero R. F., Hernandez-Galan R., Collado I. G. (2008). Secondary metabolites from species of the biocontrol agent Trichoderma. Phytochem. Rev. 7, 89–123. doi: 10.1007/s11101-006-9032-2
Reithner B., Brunner K., Schuhmacher R., Peissel I., Seidl V., Krska R., et al. (2005). The G-protein a-subunit Tga1 of t. atroviride is involved in chitinase formation and differential production of antifungal metabolites. Fungal Genet. Biol. 42, 749–760. doi: 10.1016/j.fgb.2005.04.009
Reuveni R., Reuveni M. (1998). Foliar-fertilizer therapy – a concept in integrated pest management. Crop Protect. 17, 111e118. doi: 10.1016/S0261-2194(97)00108-7
Roiger D. J., Jeffers S. N., Caldwell R. W. (1991). Occurrence of Trichoderma species in apple orchard and woodland soils. Soil Biol. Biochem. 23, 353–359. doi: 10.1016/0038-0717(91)90191-L
Roy A. K. (1977). Parasitic activity of Trichoderma viride on the sheath blight of rice (Cortium sasaki). J. Plant Dis. Prot. 84, 675–683.
Russell P. E. (2005). A century of fungicide evolution. J. Agric. Sci. 143, 11e25. doi: 10.1017/S0021859605004971
Saba H., Vibhash D., Manisha M., Prashant K. S., Farman H., Tauseed A. (2012). Trichoderma–a promising plant growth stimulator and biocontrol agent. Mycosphere 3, 524–531. doi: 10.5943/mycosphere/3/4/14
Sallam N. M. A., Eraky A. M. I., Sallam A. (2019). Effect of Trichoderma spp. on fusarium wilt disease of tomato. Mol. Biol. Rep. 46, 4463–4470. doi: 10.1007/s11033-019-04901-9
Samuels G. J., Dodd S. L., Gams W., Castlebury L. A., Petrini O. (2002). Trichoderma species associated with the green mold epidemic of commercially grown Agaricus bisporus. Mycologia 94, 146–170. doi: 10.1080/15572536.2003.11833257
Sarhan E. A. D., Abd-Elsyed M. H. F., Ebrahiem A. M. Y. (2020). Biological control of cucumber powdery mildew (Podosphaera xanthii) (Castagne) under greenhouse conditions. Egypt. J. Biol. Pest Contr. 30, 65. doi: 10.1186/s41938-020-00267-4
Sawant S. N., Deshmukh S. K., Ganguli B. N. (1996). On an unstable antifungal metabolite from Trichoderma koningii-isolation and structure elucidation of a new cyclopentenone derivative (3-Dimethylamino-5- hydroxy-5-vinyl-2-cyclopenten-1-one). J. Antibiot. 49, 220–221.
Schuster A., Schmoll M. (2010). Biology and biotechnology of Trichoderma. Appl. Microbiol. Biotechnol. 87, 787–799. doi: 10.1007/s00253-010-2632-1
Segarra G., Cassanova E., Aviles M., Trillas I. (2010). Trichoderma asperellum strain T34 control Fusarium wilt disease in tomato plants in soil less culture through competition for iron. Fungal Microbiol. 59, 141–149.
Shashikumar H. M., Koulagi S., Navyashree S. E. (2019). Compatibility of Trichoderma viride and Trichoderma harzianum with fungicides against soil borne diseases of tomato and cabbage. Int. J. Curr. Microbiol. Appl. Sci. 8 (4), 1920–1928. doi: 10.20546/ijcmas.2019.804.225
Shi Z. Z. (2018). Chemical structures and biological activities of secondary metabolites from five marine-Alga-Epiphytic fungi (Yantai, China: Yantai Institute of Coastal Zone Research, Chinese Academy of Sciences).
Shoemaker S., Schweikart V., Ladner M., Gelfand D., Kwok S., Myambo K., Innis M., et al. (1983). Molecular cloning of exocellubiohydralase I derived from Trichoderma reesei strain L27. Nat. Biotechnol. 1, 691–696. doi: 10.1038/nbt1083-691
Siddiquee S., Cheong B. E., Taslima K., Hossain K., Hasan M. M. (2012). Separation and identification of volatile compounds from liquid cultures of Trichoderma harzianum by GC-MS using three different capillary columns. J. Chromatogr. Sci. 50, 358–367. doi: 10.1093/chromsci/bms012
Silva H. F., Santos A. M. G., do Amaral A. C. T., Bezerra J. L., Martins E. D., Luz N., et al. (2020). Bioprospection of Trichoderma spp. originating from a cerrado-caatinga ecotone on Colletotrichum truncatum, in soybean. Rev. Bras. Ciências Agrárias Agron. 15, 7680.
Simmons E. G. (1977). “Classification of some cellulase-producing trichoderma species,” in International mycolohical congress, 2nd. Eds. Bigelow H. E., Simmons E. G. (Tampa: University of South Florida), 618.
Singh H. B., Daiho L., Upadhyay D. N. (1988). “Biological control of Sclerotium rot of knol-khol by Trichoderma harzianum,” in Proc. Of the international Conf. on res. In Pl. and its relevance to future. 92 (Delhi Univ).
Singh S., Dureja P., Tanwar R. S., Singh A. (2005). Production and antifungal activity of secondary metabolites of Trichoderma virens. pestic. Res. J. 17, 26–29.
Singh J., Khilari K., Singh R., Mishra P., Kumar P., Singh R. (2019). Studies on the compatibility of Trichoderma spp. with nematicides under in vitro conditions. J. Pharmacog. Phytochem. SP3, 08–11.
Sivan A., Chet I. (1989). The possible role of competition between T. harzianum and F. oxysporum on rhizosphere colonization. Phytopathol 79, 198–203. doi: 10.1094/Phyto-79-198
Song F., Dai H., Tong Y., Ren B., Chen C., Sun N., et al. (2010). Trichodermaketones a-d and 7-o-methylkoninginin d from the marine fungus Trichoderma koningii. J. Nat. Prod. 73, 806–810. doi: 10.1021/np900642p
Song Y. P., Miao F. P., Fang S. T., Yin X. L., Ji N. Y. (2018). Halogenated and nonhalogenated metabolites from the marine-alga-endophytic fungus Trichoderma asperellum cf44-2. Mar. Drugs 16, 266. doi: 10.3390/md16080266
Sonowal B., Mahanta B., Borah A., Dutta P. (2020). Efficacy of glomus fasciculatum, org-trichojal, vermicompost and carbofuran -G in the management of Meloidogyne incognita in ivy gourd. Int. J. Curr. Microbiol. Appl. Sc. 9, 936–943. doi: 10.20546/ijcmas.2020.905.103
Steiger M. G., Vitikainen M., Uskonen P., Brunner K., Adam G., Pakula T., et al. (2011). Transformation system for hypocrea jecorina (T. reesei) that favours homologous integration and employs reusable bidirectionally selectable markers. Appl. Environ. Microbiol. 77, 114–121. doi: 10.1128/AEM.02100-10
Stoppacher N., Kluger B., Zeilinger S., Krska R., Schuhmacher R. (2010). Identification and profiling of volatile metabolites of the biocontrol fungus Trichoderma atroviride by HS-SPME-GC-MS. J. Microbiol. Methods 81, 187–193. doi: 10.1016/j.mimet.2010.03.011
Sun Y., Lv A. L., Tian L., Wei L., Pei Y. H. (2007). Secondary metabolism product of fungus Trichoderma reesei. J. Shenyang Pharm. Univ. 24, 546–548.
Suryanarayanan T. S., Rajulu G., Vidal S. (2016). Biological control through fungal endophytes: gaps in knowledge hindering success. Curr. Biotechnol. 5, 1–13.
Swehla A., Pandey A. K., Nair R. M. (2020). Bioactivity of Trichoderma harzianum isolates against fungal root rot pathogens with special reference to Macrophomina phaseolina causing dry root rot of mungbean. Indian Phytopath. 73, 787–792. doi: 10.1007/s42360-020-00288-x
Tarus P. K., Langat-Thoruwa C. C., Wanyonyi A. W., Chhabra S. C. (2003). Bioactive metabolites from Trichoderma harzianum and Trichoderma longibrachiatum. bull. Chem. Soc Ethiop. 17, 185–190.
Thakur A. K., Norris R. V. (1928). A biochemical study of some soil fungi with special reference to ammonia production. J. Indian Inst. Sci. XI A. 12, 141–160.
Thoudam R., Dutta B. (2012). Control of black rot disease of tea (Camellia sinensis i (L.) O kuntze) with the mycoflora isolated from tea environment and phyllosphere. J. Mycopathol. Res. 50, 205–210.
Tranier M. S., Pognant-Gros J., Quiroz R. D. C., Gonzalez C. N. A., Mateille T., Roussos S. (2014). Commercial biological control agents targeted against plant-parasitic root-knot nematodes. Braz. Arch. Biol. Technol. 57 (6), 831–841. doi: 10.1590/S1516-8913201402540
Trushina N., Levin M., Mukherjee P. K., Horwitz B. A. (2013). PaC and pH-dependent transcriptome of the mycotrophic fungus Trichoderma virens. BMC Genet. 14, 138. doi: 10.1186/1471-2164-14-138
Tseng S. C., Liu S. Y., Yang H. H., Lo C. T., Peng K. C. (2008). Proteomic study of bicontrol mechanisms of Trichoderma harzianum EST 323 in response to Rhizoctonia solani. J. Agric. Food Chem. 56, 6914–6922. doi: 10.1021/jf703626j
Upamanya G. K., Bhattacharya A., Dutta P. (2020). Consortia of entomopathogenic fungi and biocontrol agents improve the agro ecological conditions for brinjal cultivation of Assam. 3 Biotech. 10, 450. doi: 10.1007/s13205-020-02439-3
Van loon L. C. (2007). Plant responses to plant growth-promoting rhizobacteria. Eur. J. Plant Pathol. 119, 243–254. doi: 10.1007/s10658-007-9165-1
Velazquez-Robeldo R., Contreras-Cornejo H., Macia-rodriguez L. I., Hernández-Morales A., Aguirre J., Casas-Flores S, et al. (2011). Role of the 4-phosphopanthenyl transferase of Trichoderma virens ins secondary metabolism and induction of plant defense responses. Mol. Plant Microb. Int. 24, 1459–1471. doi: 10.1094/MPMI-02-11-0045
Verma M., Brar S. K., Tyagi R. D., Surampalli R. Y., Valéro J. R. (2007). Antagonistic fungi, Trichoderma spp. panoply of biological control. Biochem. Eng. J. 37, 1–20. doi: 10.1016/j.bej.2007.05.012
Vey A., Hoag I. R. E., Butt T. M. (2001). “Toxic metabolites of fungal biocontrol agents,” in Fungi as biocontrol agents: Progress, problems and potential. Eds. Butt T. M., Jackson C., Magan N. (Bristol, USA: CAB International Publishing), 311.
Vicente M. F., Cabello A., Platas G., Basilio A., Dier M. T., Dreikorn S., et al. (2001). Antimicrobial activity of ergokonin a from Trichoderma longibrachiatum. J. Appl. Microbiol. 91, 806–813. doi: 10.1046/j.1365-2672.2001.01447.x
Villanueva A., Ramon D., Valles S., Lluch M. A., MacCabe A. P. (2000). Heterologous expression in Aspergillus nidulans of a Trichoderma longibrachiatum endoglucanase of ecological relevance. J. Agric. Food Chem. 48, 951–957. doi: 10.1021/jf990606a
Vinale F., Girona I. A., Nigro M., Mazzei P., Piccolo A., Ruocco M., et al. (2012). Cerinolactone, a hydroxy-lactone derivative from Trichoderma cerinum. J. Nat. Prod. 75, 103–106. doi: 10.1021/np200577t
Vinale F., Sivasithamparam K., Ghisalberti E. L., Marra R., Barbetti M. J., Li H., et al. (2008). A novel role for Trichoderma secondary metabolites in the interactions with plants. Physiol. Mol. Plant P. 72, 80–86. doi: 10.1016/j.pmpp.2008.05.005
Viterbo A., Horwitz B. A. (2010). “Mycoparasitism,” in Cellular and molecular biology of filamentous fungi. Eds. Borkovich K., Ebbole D. J. (Washington D.D: ASM Press), 676–693.
Voigt K., Kirk P. M. (2011). Recent developments in the taxonomic affiliation and phylogenetic positioning of fungi: Impact in applied microbiology and environmental biotechnology. Appl. Microbiol. Biotechnol 90, 41–57.
Vurukonda S. S. K. P., Giovanardi D., Stefani E. (2018). Plant growth promoting and biocontrol activity of streptomyces spp. as endophytes. Int. J. Mol. Sci. 19 (4), 952–978. doi: 10.3390/ijms19040952
Wada S. I., Iida A., Akimoto N., Kanai M., Toyama N., Fujta T. (1995). Fungal metabolites. XIX. structural elucidation of channel-forming peptides, trichorovins-I-XIV, from the fungus Trichoderma viride. Chem. Pharm. Bull. 43, 910–915. doi: 10.1248/cpb.43.910
Waiter A., Szczodrak J., Pleszczynska M. (2005). Optimization of conditions for the efficient production of mutan in streptococcal cultures and post-culture liquids. Acta Biol. Hung. 56, 137–150. doi: 10.1556/ABiol.56.2005.1-2.14
Wallner A., Blatzer M., Schrettl M., Sarg B., Lindner H., Haas H. (2009). Ferrocrocin, a siderophore involved in intra- and transcellular iron distribution in Aspergillus fumigatus. Appl. Envrion. Microbiol. 75, 4194–4196. doi: 10.1128/AEM.00479-09
Weindling R. (1932). Trichoderma lingorum as a parasite of other soil fungi. Phytopathol 22, 837–845.
Weindling R. (1934). Studies on a lethal principle effective in the parasitic action of Trichoderma lingorum on Rhizoctonia solani and other soil fungi. Phytopathol 24, 1153–1179.
Weller S. C., Culbreath A. K., Gianessi L., Godfrey L. D. (2014). The contributions of pesticides to pest management in meeting the global need for food production by 2050 (Ames, Iowa: Council for Agricultural Science and Technology (CAST).
Wells H., Bell D., Jaworski A. (1972). Efficacy of Trichoderma harzianum as a biological control for Sclerotium rolfsii. Phytopathology 62, 442–447. doi: 10.1094/Phyto-62-442
Wheatley R., Hackett C., Bruce A., Kundzewicz A. (1997). Effect of substrate composition on production of volatile organic compounds from Trichoderma spp. inhibitory to wood decay fungi. Int. Biodeterior. Biodegrad. 39, 199–205. doi: 10.1016/S0964-8305(97)00015-2
Widawsky D., Rozelle S., Jin S., Huang J. (1998). Pesticide productivity, host-plant resistance and productivity in China. Agric. Econ. 19, 203e217.
Widen P., Abitbol J. J. (1980). Seasonality of Trichoderma species in a spruce-forest soil. Mycologia 72, 775–784. doi: 10.1080/00275514.1980.12021246
Wipf P., Kerekes A. D. (2003). Structure reassignment of the fungal metabolite TAEMC161 as the phytotoxin viridiol. J. Nat. Prod. 66, 716–871. doi: 10.1021/np0300277
Yang C. A., Cheng C. H., Lo C. T., Liu S. Y., Lee J. W., Peng K. C. (2011). A novel l-amino acid oxidase from Trichoderma harzianum ETS 323 associated with antagonism of Rhizoctonia solani. J. Agric. Food Chem. 59, 4519–4526. doi: 10.1021/jf104603w
Yang H. H., Yang S. L., Peng K. C., Lo C. T., Liu S. Y. (2009). Induced proteome of Trichoderma harzianum by Botrytis cinerea. Mycol. Res. 113, 924–932. doi: 10.1016/j.mycres.2009.04.004
Yang P., Yang Q., Xu Q. (2014). Study on metabolites related to biocontrol from Trichoderma asperellum. J. Harbin Univ. Commun. 30, 36–40.
Yedidia I., Benhamou N., Chet I. (1999). Induction of defense responses in cucumber plants (Cucumis sativus l.) by the biocontrol agent Trichoderma harzianum. Appl. Environ. Microbiol. 65, 1061–1070. doi: 10.1128/AEM.65.3.1061-1070.1999
Zehra A., Dubey M. K., Meena M., Upadhyay R. S. (2017a). Effect of different environmental conditions on growth and sporulation of some Trichoderma species. J. Environ. Biol. 38, 197–203. doi: 10.22438/jeb/38/2/MS-251
Zehra A., Meena M., Dubey M. K., Aamir M., Upadhyay R. S. (2017b). Activation of defense response in tomato against fusarium wilt disease triggered by Trichoderma harzianum supplemented with exogenous chemical inducers (SA and MeJA). Braz. J. Bot. 40, 651–664. doi: 10.1007/s40415-017-0382-3
Zeilinger S., Omann M. (2007). Trichoderma biocontrol: signal transduction pathways involved in host sensing and mycoparasitism. Gene Regul. Syst. Biuol. 1, 227–234. doi: 10.4137/GRSB.S397
Zhang M. (2015). Mangrove endophytic fungus trichoderma sp. Xy24 steroid metabolites and microbial transformation of harzianone (Beijing, China: Beijing University of Chinese Medicine).
Zhang M., Li N., Chen R. D., Zou J. H., Wang C. M., Dai J. G. (2014). Two terpenoids and a polyketide from the endophytic fungus trichoderma sp. Xy24 isolated from mangrove plant Xylocarpus granatum. J. Chin. Pharm. Sci. 23, 421–424. doi: 10.5246/jcps.2014.06.056
Keywords: microbial biocontrol agents, ecology, mode of action, disease control, industrial application
Citation: Dutta P, Deb L and Pandey AK (2022) Trichoderma- from lab bench to field application: Looking back over 50 years. Front. Agron. 4:932839. doi: 10.3389/fagro.2022.932839
Received: 30 April 2022; Accepted: 08 September 2022;
Published: 03 October 2022.
Edited by:
Jonathan Spencer West, Rothamsted Research, United KingdomReviewed by:
Pedro Laborda, Nantong University, ChinaCopyright © 2022 Dutta, Deb and Pandey. This is an open-access article distributed under the terms of the Creative Commons Attribution License (CC BY). The use, distribution or reproduction in other forums is permitted, provided the original author(s) and the copyright owner(s) are credited and that the original publication in this journal is cited, in accordance with accepted academic practice. No use, distribution or reproduction is permitted which does not comply with these terms.
*Correspondence: Abhay K. Pandey, YWJoYXlrdW1hcnBhbmRleS5rdUBnbWFpbC5jb20=; Pranab Dutta, cHJhbmFiZHV0dGE3NEBnbWFpbC5jb20=
Disclaimer: All claims expressed in this article are solely those of the authors and do not necessarily represent those of their affiliated organizations, or those of the publisher, the editors and the reviewers. Any product that may be evaluated in this article or claim that may be made by its manufacturer is not guaranteed or endorsed by the publisher.
Research integrity at Frontiers
Learn more about the work of our research integrity team to safeguard the quality of each article we publish.