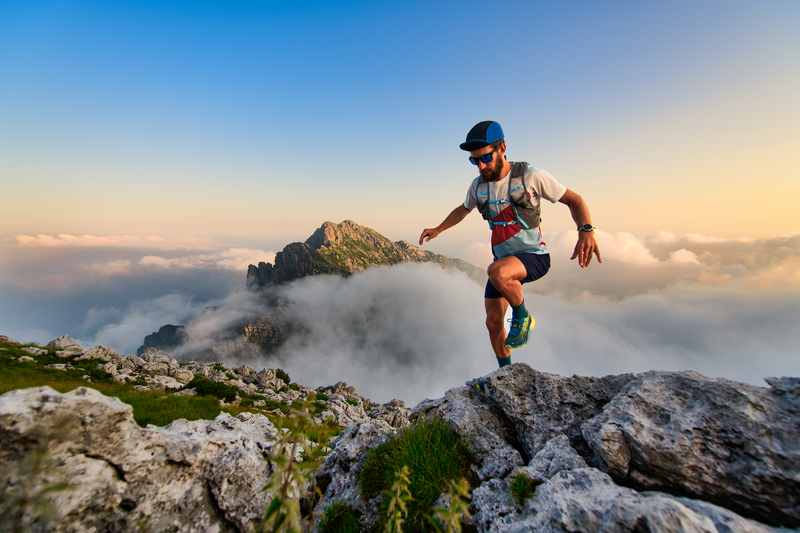
95% of researchers rate our articles as excellent or good
Learn more about the work of our research integrity team to safeguard the quality of each article we publish.
Find out more
REVIEW article
Front. Agron. , 21 April 2022
Sec. Plant-Soil Interactions
Volume 4 - 2022 | https://doi.org/10.3389/fagro.2022.844166
This article is part of the Research Topic Belowground Interactions and Implications for Nutrient Use Eco-Efficiency in Cropping Systems View all 6 articles
Hot and dry Mediterranean ecoregions are characterized by low soil organic carbon content and large potential to become carbon sink when appropriately managed. Soil carbon sequestration may also play an important role in improving the resilience of these vulnerable agroecosystems to increasingly drastic impacts of global climate change. One agricultural practice that aims to increase soil organic carbon stocks, among other beneficial outcomes, is the use of cover crops. Although cover crops can increase soil organic carbon content, recent studies have observed that cover crops may lead to lower soil carbon stocks when considering co-management strategies, especially at greater soil depths. In this review, we outline the current paradigm of soil organic carbon dynamics and aim to apply our current understanding of soil carbon sequestration processes to cover crop management. We review how cover crop practices such as cover crop species selection, growth duration, and termination methodologies may impact soil organic matter sequestration and stabilization processes and provide insights to direct future research and inform cover crop management for C sequestration in Mediterranean agroecosystems.
The world's attention is gaining focus on possible ways for agriculture to offset the impacts of global climate change (Lal, 2016). Soils have the unique capacity to sequester large amounts of carbon (C). Soils contain ~75% of the terrestrial carbon pool, which corresponds to two to three times the amount of C in both the atmosphere and vegetation (Fontaine et al., 2007; Schmidt et al., 2011; Scharlemann et al., 2014). Thus, with about half of habitable land-use being allocated to agriculture (Ellis et al., 2010), agricultural soils play a key role in maintaining a balanced global carbon cycle and provide mitigation and adaptation strategies to address global climate change (Gross and Harrison, 2019; Tautges et al., 2019; Lavallee et al., 2020).
Occupying nearly 15% of the global land area, soils forming in Mediterranean climates have the potential to be significant carbon sinks (Safriel et al., 2005). Having cool moist winters and warm dry growing seasons (Aguilera et al., 2013), these soils are typically low in soil organic carbon (SOC) due to limited primary productivity and conditions that favor rapid microbial decomposition of plant residue (Van-Camp et al., 2004; Chiti et al., 2012; Wiesmeier et al., 2013). However, agricultural practices remove some of these limitations by increasing biomass inputs and therefore C storage potential if managed adequately.
Soils of Mediterranean environments are also highly biodiverse (Underwood et al., 2009) and provide natural resources to support some of the most productive agroecosystems in the world (Guan et al., 2009) in regions of California, central Chile, southwest Australia, southwest South Africa, and land around the Mediterranean Sea (Aschmann, 1984; Aguilera et al., 2013). In fact, the state of California supports the production of more than half of the US fruits, nuts, and vegetables (Johnston and Carter, 2000). Adoption of irrigated agriculture in these designed landscapes impact many of the processes regulating carbon deposition and cycling, such as enhancing biomass inputs into the soil ecosystem. However, intensification, simplified rotations, and soil disturbances have hampered this potential despite the recognized benefits of conservation practices for SOC accumulation (Lal, 2020). Given the C sequestration potential of Mediterranean soils and the potential for widespread soil degradation in these agroecosystems, there is an urgent need to identify effective management approaches that optimize soil organic carbon sequestration and stabilization in these unique agroecosystems.
Cover cropping has been recognized as an effective practice to promote soil conservation with co-benefits for sustainability and resilience of agriculture (Duval et al., 2016; Novara et al., 2021) through erosion control, and nutrient and water conservation (Shroder, 2020). Cover crops can be grown as grazed or exported forage, nutrient catch crops, ground cover to reduce losses, and green manure when used as a source of nutrients (Shackelford et al., 2019). As such, cover crops are widely promoted through EU Common Agricultural Policy (Shackelford et al., 2019) and federal and state incentive programs in the US. Cover cropping is also increasingly recognized as a practice that increases SOC (Gristina et al., 2020). However, uncertainties and high variability in the impacts of cover crops on SOC stocks remain. While various meta-analyses have indicated that cover crops can increase SOC content (Aguilera et al., 2013; Poeplau and Don, 2015), other studies show no effects (Kaspar et al., 2006; Celette et al., 2009; Steele et al., 2012; Oliveira et al., 2016) and even loss of soil organic carbon in the deep soil horizons of some agroecosystems (Tautges et al., 2019; Camarotto et al., 2020). The effectiveness of cover cropping is therefore site-specific and varies with soil properties, climate, and management practices (García-Orenes et al., 2010; Peregrina et al., 2010; Ramos et al., 2010; Gómez, 2017). Recent understanding of carbon and nutrient flows in soil ecosystems also point to the importance of cycling through microbial communities for long term carbon sequestration (Lehmann and Kleber, 2015; Kallenbach et al., 2016). Thus, the emerging complexities of SOC cycling and stabilization and destabilization mechanism must inform cover crop management to maximize C sequestration (Harden et al., 2018) and more accurately predict cover crop management outcome (Dijkstra et al., 2021).
The goal of this review is to apply our current understanding of SOC pools and fluxes to guide cover crop management strategies that prioritize SOC sequestration. To contextualize this discussion, we constrained this review to studies of cover cropping in systems with Mediterranean-type climates. These agroecosystems are complex and include a wide range of commodities (perennial and annual crops) and large variability in agricultural practices that affect cover crop management and SOC dynamics. Cover crop management goals are also diverse in these settings—including a source of crop nutrients, to control erosion, improve soil health, promote biodiversity, and offset greenhouse gas emissions—with each objective influencing how cover crops are selected and managed. We first review a set of grounding principles to appraise SOC stabilization and destabilization mechanisms in these systems based on three influencing factors: (1) SOC cycling; and influence of (2) soil depth; and (3) plant residue quality. We then discuss how considering SOC pools and storage mechanisms across depths and for various biomass inputs can provide insight into cover crop management (i.e., species selection, growth duration, and termination methodology) for increased SOC sequestration.
Understanding SOC dynamics in response to land-use changes, soil management practices, and global climate change will help identify opportunities to optimize SOC sequestration and ensure its long-term persistence. Scientific research has well-established the drawbacks associated with considering soil organic matter (SOM) as a single uniform entity (Parton et al., 1988; Jenkinson, 1990; Trumbore, 2009). More recent conceptual frameworks have developed upon our understanding of SOM dynamics, specifically the physicochemical complexity of SOC and its continuum as pools (storage) and fluxes (inflow and outflow rate) components across a spectrum of decomposition stages, each with contrasting properties (Six et al., 2002; Cotrufo et al., 2013; Lehmann and Kleber, 2015; Basile-Doelsch et al., 2020; Lavallee et al., 2020; Zhu et al., 2020). Nevertheless, our understanding of the specific determinants underlying the long-term persistence of SOC are currently under contention. Recent conceptual frameworks are broadly moving away from models based on chemical recalcitrance mechanisms, such as humic substances with degradative resistance, toward models based on chemical stabilization and physical protection mechanisms of SOM via mineral sorption and aggregate occlusion (Figure 1; Six et al., 2002; Lehmann and Kleber, 2015; Basile-Doelsch et al., 2020; Witzgall et al., 2021). Ultimately, the most significant challenge remains in categorizing and measuring this dynamic SOC continuum; especially when considering that the formation of stable and long-term sequestered SOC requires continual flux and turnover (Six et al., 2002; Janzen, 2006; Kallenbach et al., 2016; Dynarski et al., 2020).
Figure 1. Summary of the main mechanisms of soil organic carbon stabilization/destabilization. (1) Physical protection, (2) Physicochemical protection, (3) DOC deep percolation.
Recently developed SOC cycling theories propose that, upon deposition into the soil ecosystem, plant and animal-derived organic material of diverse sizes, quality, and molecular complexity are continuously processed by the soil decomposer community, through fragmentation and depolymerization, eventually resulting in the lower molecular-weight and labile substrates that are utilized during microbial assimilation (Cotrufo et al., 2013; Lehmann and Kleber, 2015; Liang et al., 2017; Basile-Doelsch et al., 2020).
Compared to larger organic fragments, these simple and labile biopolymers and monomers have both a higher oxidation state and increased solubility in water, thus increasing their transport and reactivity within the soil solution. In turn, this dissolved organic matter (DOM) is generally more spatially accessible (Erktan et al., 2020) with a higher capacity for assimilation into microbial biomass due to its smaller size and lower molecular weight (Weiss et al., 1991; Kallenbach et al., 2015). Simultaneously, the solubility and heightened oxidation state of these labile carbon substrates are thought to facilitate more sorption interactions with mineral surfaces, increasing their protection and persistence through stabilization within the soil matrix and/or incorporation within aggregates (Cotrufo et al., 2013; Robertson et al., 2019). While this microbial metabolic processing of substrates results in some loss of SOC through respiration, it also facilitates the flux of C through the soil ecosystem via production of microbially-derived substrates and turnover of microbial necromass—a concept known as the microbial carbon pump (MCP; Liang et al., 2017; Liang, 2020; Zhu et al., 2020). Thus, under this concept, C inputs have several possible pathways: (1) continual flux through the MCP; (2) escape from the MCP through microbial respiration; or (3) escape from the MCP via physicochemical protection of microbial necromass within the soil matrix and/or occlusion within aggregates.
Importantly, organic substrates exist along a thermodynamic gradient whereby the transformation of large molecular weight, recalcitrant, and energy-rich compounds into smaller molecular weight, labile, and energy-poor compounds release energy and reduce the activation energy required to depolymerize subsequent stages (Malik et al., 2016). This has functional relevance since the enzymatic investment requirements of the soil microbial community to process soluble, labile substrates is much less than for processing recalcitrant, high-energy compounds such as cellulose and lignin. As such, alterations in the quality of C inputs into the soil ecosystem may cause shifts in the microbial community's energy investment strategies (Schimel et al., 2007; Kallenbach et al., 2015; Kravchenko et al., 2019; Malik et al., 2020), as well as their Carbon Use Efficiency (CUE) and thus the fate of C inputs (Manzoni et al., 2012; Cotrufo et al., 2013; Kirkby et al., 2013; Kallenbach et al., 2015). Extracellular enzyme production and increased metabolic costs associated with breakdown of complex biopolymers have been shown to reduce CUE when compared to labile DOM compounds, which may be assimilated into microbial biomass with minimal depolymerization (Lekkerkerk et al., 1990; Malik et al., 2016). The higher nitrogen (N) content of the DOM pool may also facilitate more efficient utilization, requiring less microbial investment in nutrient acquisition (Berg and Meentemeyer, 2002; Hessen et al., 2004; Liang et al., 2007, 2019). As such, it has been hypothesized that labile C substrates are the dominant source of microbial products, while also having a more direct and efficient pathway toward mineral stabilization (Cotrufo et al., 2013; Kallenbach et al., 2015). In general, cover cropping is understood to increase the proportion of labile carbon inputs into soil (Duval et al., 2016; White et al., 2020). However, less attention has been paid to the specific ways by which cover crop management strategies may increase the relative proportion of plant photosynthates allocated toward labile dissolved carbon inputs, such as through enhanced rhizodeposition, and how this may therefore result in improved SOC accumulation.
With respect to the monitoring of SOC persistence, a framework, proposed in Lavallee et al. (2020), suggests a simple and ecologically relevant method for measuring SOC storage that separates SOM into two physically defined pool fractions, particulate organic matter (POM) and mineral-associated organic matter (MAOM). There is potential for cover crop selection and management to influence the size of these fractions. Also called the light fraction, POM is formed during litter decomposition through fragmentation and depolymerization. It contains lightweight fragments of plant and fungal material, composed predominantly of complex structural compounds such as celluloses, lignin, and chitin, that are relatively undecomposed and generally low in nutrient content (Six et al., 2001; Kögel-Knabner et al., 2008; Sanderman et al., 2014; Cotrufo et al., 2015). POM exists within a spectrum of size and density categories and may either be physically accessible for decomposition (free) or protected from degradation through occlusion within aggregates (Jastrow and Miller, 1996; Lavallee et al., 2020). Significant research has concluded that POM is essential in the genesis of large microaggregates and macroaggregates, as a nucleus for the assemblage of smaller microaggregates together (Cambardella and Elliott, 1993; Jastrow and Miller, 1996; Angers et al., 1997; Six et al., 2000; Bongiovanni and Lobartini, 2006; Jastrow et al., 2007; Witzgall et al., 2021). As such, the role of POM in the formation of soil structure and SOC physical occlusion is significant. The physical transport pathways of POM are notably more limited than that of DOM substrates, and so its incorporation within the soil profile occurs more slowly in lieu of cultivation and bioturbation (Rabbi et al., 2014; Cotrufo et al., 2015).
On the other hand, MAOM contains low molecular weight compounds derived mainly from microbial decomposition (Kögel-Knabner et al., 2008; Cotrufo et al., 2013; Sanderman et al., 2014; Castellano et al., 2015; Kleber et al., 2015). The extent of MAOM accumulation in soils is dependent on the balance between production of microbial biomass and decomposition to necromass (Six et al., 2006). This balance is influenced by the microbial CUE, which is defined as the proportion of organic C allocated toward the accumulation of biomass (anabolism) relative to the organic C allocated toward respiration (catabolism) (Spohn et al., 2016). Thus, higher CUE leads to higher microbial biomass, and therefore, higher SOC cycling and storage from necromass, while lower CUE leads to more carbon respiration and gas losses (Manzoni et al., 2012).
The microbial biomass (necromass) produced is then stabilized into the MAOM pool mainly through physicochemical interactions with soil minerals (Figure 1; Six et al., 2002; von Lützow et al., 2007; Cotrufo et al., 2013; Kallenbach et al., 2016). Hence, the capacity of a soil to store SOC as MAOM is conditioned by the inherent soil physicochemical characteristics, such as the relative ratio of clay and silt content, clay mineralogy (i.e., 1:1 or 2:1 phyllosilicates), pH, short-range ordered minerals, and the presence of crystalline and poorly crystalline Fe/Al oxides that combine to determine mineral surface area and sorption and desorption dynamics (Six et al., 2002; Jastrow et al., 2007; Bailey et al., 2019). Thus, soils have different SOC mineral saturation potentials, defined as the theoretical maximum of SOC storage within the MAOM under optimum conditions (i.e., soil moisture, temperature, continuous carbon inputs, and soil mineralogy; Castellano et al., 2015).
This differing SOC saturation potential was highlighted in a recent comparative study that aimed to quantify the potential of SOC sequestration of a wide range of soil functional groups in the World Reference Base (i.e., calcaric, calcic, fluvic, and vertic) as a result of cover cropping. All the soils studied exhibited different C sequestration potential, where soils with fluvic diagnostic materials (marine and lacustrine parent materials) showed the highest SOC storage potential (34.4 t/ha; Gristina et al., 2020). The concept of saturation limit predominately concerns the MAOM pool, with some studies indicating no saturation limitations for POM (Six et al., 2002; Stewart et al., 2009; Lajtha et al., 2014). Given that at least half of the total SOC in arable land consists of the MAOM pool (Kirchmann et al., 2004; Miltner et al., 2012; Plaza et al., 2013; Wiesmeier et al., 2013), it may be most impactful to target soil C increases within the MAOM. However, several studies suggest that once the MAOM fraction is saturated, the SOC accrual increase in the POM fraction becomes larger (Six et al., 2002; Castellano et al., 2015; Cotrufo et al., 2019).
Most notably, POM and MAOM have highly variable mean residence times. While the POM fraction generally resides from years to decades, MAOM has longer residence time potentials of decades to centuries (Kögel-Knabner et al., 2008; Kleber et al., 2015). Though distinct in their chemical composition (Cotrufo et al., 2015), the residence times of POM and MAOM are thought to be related more to their physical protection mechanisms in soil, and thus, when physically protected through macroaggregate occlusion, POM may persist on timescales of decades to centuries (von Lützow et al., 2007). However, macroaggregates are less persistent than smaller microaggregates and are thought of as highly susceptible to breakdown upon soil disturbance. Given the context of our need to monitor SOC sequestration and the challenge of identifying meaningful and accurate indicators of SOC change over time, POM (both free and occluded) and MAOM are understood to be distinct and relevant measurements for evaluating the responses of SOC to contrasting agricultural management practices. They are also relevant in considering physical stabilization mechanisms, with approachable measurability and broad applications for landscape modeling of cover crops and SOC sequestration rates across semi-arid regions (Cambardella and Elliott, 1993; Collins et al., 1999; Duval et al., 2013; Rocci et al., 2021).
With further relevance to semi-arid cropland, having regionalized knowledge of SOC sequestration potentials (saturation deficit) for each pedoclimatic condition would help effectively assess the capacity of soils to store more C and inform cover crop management for C sequestration (Castellano et al., 2015; Cotrufo et al., 2019; Gristina et al., 2020; Devine et al., 2021). In fact, soils can be saturated even when SOC is low, due to limitations of SOC stabilization imposed by management and climate (Stewart et al., 2007). As a result, an increase in C inputs would not necessarily increase the total and/or physico-chemically stabilized SOC stocks (Castellano et al., 2015). In fact, sometimes soils can exhibit a negative balance of SOC due to the occurrence of some destabilization mechanisms such as desorption of the previously adsorbed SOC (Figure 1; Schmidt et al., 2011). Once SOC is detached from soil minerals, it becomes more available for decomposition, mineralization, microbial assimilation and eventually release to the atmosphere as CO2 (Figure 1; Novara et al., 2016).
Recent studies have demonstrated that small amounts of organic C in deep soil layers equate to large SOC stocks when integrated across the entire soil profile (Richter and Markewitz, 1995; Rumpel and Kögel-Knabner, 2011; Harper and Tibbett, 2013; Moreland et al., 2021), with more than half of the total SOC stored in deep soil layers beyond the top 20 cm (Jobbágy and Jackson, 2000; Harrison et al., 2011; Schmidt et al., 2011). The SOC storage dynamics in deep soil layers are regulated by the lack of consistent fresh C inputs, and therefore a greater degree of energy limitation, a smaller microbial population, and physical isolation between microbial decomposers and organic matter, contributing to slower turnover times with increasing depth (Fontaine et al., 2007; Schmidt et al., 2011; Hicks Pries et al., 2018). Pedogenic processes and features of subsoils also contribute to C sequestration potentials, such as: (1) clay accumulation, a key characteristic of many subsoils, which promotes both higher MAOM saturation thresholds and stable microaggregate formation with the capacity to occlude POM (Six et al., 2000); (2) transformations of primary minerals and associated weathering products into secondary minerals within the subsoil, contributing to greater amounts of substrates to facilitate MAOM stabilization compared to topsoil (Rasmussen et al., 2006); and (3) presence of large structural units, dense or cemented horizons typical of subsoils that can exclude roots, water, and air, limiting microbial activity and influencing physical transport mechanisms that further isolate SOC from bulk soil microbial communities (Schmidt et al., 2011).
Traditionally, deep SOC has been considered more stable (Harrison et al., 2011; Schmidt et al., 2011) and is also generally of older age (Liebmann et al., 2020). For instance, deep SOC storage at a 6-m depth was recently found to persist for over 20,000 years (Moreland et al., 2021). However, while subsurface SOC stabilization and storage appears to occur relatively slowly (Liebmann et al., 2020), some lines of evidence suggest that deep SOC, mainly MAOM, may be proportionally more susceptible to rapid destabilization and decomposition than SOC in topsoil. This is due to changes in environmental conditions, such as soil properties and climate, as well as soil management practices (Harrison et al., 2011; Keiluweit et al., 2015; Shahzad et al., 2019). These conditions may affect the mineral surface charge and the mineral solubility, which will eventually lead to more soil organic carbon destabilization within the MAOM pool (Bailey et al., 2019).
For instance, soil pH and redox directly influence MAOM destabilization (Rasmussen et al., 2018; Bailey et al., 2019). A change in soil pH (increase or decrease) may induce a change in the mineral surface charge (positive or negative; Bailey et al., 2019), and therefore its reactivity vis a vis the adsorption of certain SOC compounds (Bailey et al., 2019). Poorly crystalline iron oxides are among the soil minerals that are highly correlated with SOC content (Huang et al., 2016), and these colloids are susceptible to changes in redox potential (Bailey et al., 2019).
The significant input of fresh C is another factor that appears to accelerate destabilization of older SOM at greater depths via microbial priming (Jobbágy and Jackson, 2000; Fontaine et al., 2007), especially when introduced infrequently as a large quantity pulse input (Sokol et al., 2019). This may result during the mixing of large quantities of fresh POM or during a burst DOM flux from surface to sub-surface soil layers (e.g., caused by termination and/or incorporation of CC residues; Nieminen, 2004; Evans et al., 2007; Fontaine et al., 2007; Butman et al., 2015). This may also occur due to the growth of deep rooting plant species, resulting from the production of several types of rhizodeposits (e.g., oxalic acid) that are shown to accelerate rhizosphere priming, and also the subsequent turnover of those roots during senescence (Keiluweit et al., 2015; Poirier et al., 2018). To this point, a recent experiment found that mixing living root inputs (rhizodeposits) with root litter inputs induced a loss of about 30% of MAOM through the rhizosphere priming effect (Sokol et al., 2019). Though temperature fluctuations are greatest near the soil surface, increasing temperatures are considered an additional exacerbating factor for increasing microbial priming rates of both surface and sub-surface SOC. Despite the relatively long turnover periods of deep SOM, a recent soil warming experiment indicated that SOC from the deeper profile could currently account for 25% of soil respiration (Hicks Pries et al., 2018), with substantial losses of soil C predicted under climate warming (Soong et al., 2021).
Alternatively, dissolved organic matter is also a potential source of stabilized SOC in deeper soil horizons (Kalbitz and Kaiser, 2008; Rumpel and Kögel-Knabner, 2011). As DOM percolates vertically into subsoils, through soil pores and preferential flow paths (e.g., soil cracks, earthworms cast, and root channels), certain SOC compounds become preferentially sorbed to mineral surfaces (Gross and Harrison, 2019). The vast majority of sub-surface MAOM content is microbially-derived, and thus, plant-derived DOM substances will generally undergo multiple sequences of transformation, sorption, and desorption during the process of percolating down the soil profile (Liebmann et al., 2020). The availability of N plays a central role in the formation of microbially-derived SOM products, especially the persistent SOC found with MAOM (Cotrufo et al., 2019). The stronger affinity of soil minerals to smaller, highly-oxidized, hydrophobic, and nitrogenous organic compounds is likely a dominant reason behind the preferential sorption of proteinaceous microbial residues versus less proteinaceous plant residue (Jardine et al., 1989; Kaiser and Zech, 1997, 2000; Gleixner et al., 2002; Guggenberger and Kaiser, 2003; Kiem and Kögel-Knabner, 2003; Knicker, 2004; Kleber et al., 2007; Kaiser and Kalbitz, 2012; Stockmann et al., 2013; Lehmann and Kleber, 2015). This highlights the key importance of SOC quality (i.e., composition) in long-term MAOM storage (Barré et al., 2018), which is particularly relevant at depths where rhizodeposits are a dominant source of microbial energy (Baumert et al., 2018) and pedogenic transformations are the greatest (Kramer et al., 2017).
Carbon input frequency (infrequent “pulse” vs. frequent “drip”) and spatial distribution are particularly important considerations for SOM stabilization in subsoil (Sokol and Bradford, 2019). Continuous, low-volume inputs of living root rhizodeposits, deeper in the soil profile, may facilitate steady microbial processing and stabilization pathways. On the other hand, large and infrequent pulses of DOM (via precipitation or irrigation) and POM (via tillage and incorporation) may facilitate “boom and bust” cycles for the microbial community—the formation of a temporarily enlarged microbial population, without subsequent energy inputs, that results in significant metabolic priming of resident SOC pools.
Mechanisms that promote POM destabilization are generally linked to aggregate disruption factors associated with increased soil disturbance, which tend to be more frequent near the soil surface (Six et al., 2000). While a potential increase in soil cultivation with the adoption of cover cropping is notable, it is not the only considerable disturbance factor affecting POM destabilization. For instance, the alternation of freeze and thaw cycles may induce a break-up of soil aggregate through the expansion of water (Bailey et al., 2019). Wetting and drying cycles may further lead to shrinking and swelling for some types of clay (2:1 clays), which can reshape soil aggregates and induce a loss of the POM (Hu et al., 2015; Rahman et al., 2018). Aggregate disruption may also occur through SOC cycling by soil fauna or during a process known as bioturbation—the physical displacement of materials by soil fauna throughout the soil profile—which can induce either SOC destabilization or stabilization depending on the bioturbation agents and mode of action (Bailey et al., 2019). For instance, earthworms may cause destabilization through consumption and decomposition of SOC (Bailey et al., 2019). Yet, they may also act as stabilization agents through the mucilage they excrete, which favors the formation of microaggregates (Six et al., 2004; Bottinelli et al., 2015) and therefore increases SOC stabilization via physical occlusion within soil aggregates.
Historically, the quantity and quality of the aboveground plant biomass fraction was considered as the overwhelmingly dominant driver determining SOC stocks (Larson et al., 1972; Rasmussen et al., 1980). Litter quality remains widely accepted as a major factor affecting SOM stabilization (Castellano et al., 2015), particularly at depth (Kramer et al., 2017). In order to link litter quality and SOC stabilization, Cotrufo et al. (2013) developed the Microbial Efficiency-Matrix Stabilization (MEMS) framework where plant litter that produces more microbial residues will likely result in more MAOM (Castellano et al., 2015). However, the ability to render a positive effect of new residue inputs within the MAOM pool is influenced by SOC saturation status (Castellano et al., 2015). In this model, improved litter quality will likely increase MAOM and result in a low saturation deficit (potential C sequestration; Castellano et al., 2015). After saturation, litter quality wouldn't affect the storage of carbon within MAOM as much, the transfer of POM into MAOM would decrease (Castellano et al., 2015), and MAOM would be more prone to destabilization through SOC desorption mechanisms (Figure 1; Bailey et al., 2019).
Recent studies provide more fine grained and robust evidence suggesting that the belowground plant biomass fraction contributes more efficiently to stable SOC than aboveground carbon inputs, especially the MAOM pool (Poeplau and Don, 2015; Austin et al., 2017; Jackson et al., 2017; Poirier et al., 2018; Gross and Harrison, 2019; Sokol and Bradford, 2019). In fact, one study observed that the conversion efficiency of belowground vs. aboveground plant inputs toward SOM stabilization is as much as fivefold (Jackson et al., 2017). This aligns with another study, which found that the belowground fraction of cover crop inputs were three times more likely to remain as SOC in the 5 months after cover crop termination (Austin et al., 2017). This belowground input efficiency during the formation of stable SOM is assumed to result from a combination of (1) the relatively higher lability of belowground inputs, (2) close proximity and physical accessibility of belowground inputs to both the rhizosphere microbial community and soil mineral surfaces, and (3) higher deposition frequency of belowground inputs into the rhizosphere (Farrar et al., 2003; Rasse et al., 2005; Jackson et al., 2017; Sokol and Bradford, 2019).
The belowground plant fraction is composed of both root litter (e.g., root hairs, root debris) and living root inputs, rhizodeposition (e.g., root exudates, secretions; Rasse et al., 2005; Oburger and Jones, 2018). These rhizodepositions are diverse and dynamic in composition and can be either water-soluble—such as sugars, amino acids, and organic acids—or water-insoluble—such as cell wall components, lipids, and mucilage (Canarini et al., 2019). Belowground organic carbon deposits are stabilized through different mechanisms depending on the origin of the input (Poirier et al., 2018). Root functional traits that are associated with storing POM are generally those that favor soil aggregation, which in turn limit the accessibility of occluded SOC to decomposers (von Lützow et al., 2008). Root litter, composed of structural materials such as cellulose and hemicellulose, are predominately stabilized through incorporation within soil aggregates (occluded POM; Hättenschwiler and Vitousek, 2000; Rasse et al., 2005). Polyphenols and polysaccharides, produced by root mucilage, play an important role as a binding agent in soil aggregate synthesis (Oades, 1984; Martens, 2002), as does fungal hyphae both from symbiotic root-associations with mycorrhizal fungi (Wilson et al., 2009) and fungi that preferentially utilize rhizodeposits (Baumert et al., 2018).
On the other hand, root chemical characteristics such as higher root N and suberin content, and rhizodeposition of low molecular weight organic compounds, can promote SOC stabilization through interactions with soil minerals, thus forming MAOM (Rasse et al., 2005; Cotrufo et al., 2013, 2015; Austin et al., 2017). Soluble rhizodeposition such as exudated sugars and organic acids have direct stabilization pathways through sorption to mineral surfaces (MAOM) (Figure 1; Kraffczyk et al., 1984; Austin et al., 2017; Liang et al., 2017; Sokol et al., 2019) so much so that they may not even require microbial processing beforehand (Mikutta et al., 2019; Angst et al., 2021). Notably, these soluble rhizodeposition may be the most efficient and effective mode of SOC stabilization, especially in subsoils where SOC content is below mineral saturation thresholds (Rasse et al., 2005; Austin et al., 2017). Even where mineral saturation is near thresholds, rhizodeposition are also essential in aggregate synthesis and SOC occlusion. To that point, one study observed living root rhizodeposition to be 2–13 times more efficient than litter inputs (above- and belowground) in contributing to both MAOM and POM accrual—and much of the rhizodeposition were persistent up to 3 years later (Sokol et al., 2019). The efficiency of forming MAOM and POM from the belowground inputs (roots and rhizodeposition) is notably different, where rhizodeposition inputs have the highest MAOM efficiency compared to roots and shoots, and root litter has the highest POM formation efficiency (Villarino et al., 2021). The high efficiency of the belowground fraction in storing and stabilizing SOC, as well as the complementary role between rhizodeposition inputs and roots in forming MAOM and POM respectively, is useful information that can help advise novel cover crop management practices to capitalize on the role of the belowground fraction for increasing SOC sequestration in Mediterranean croplands.
Harnessing the potential of cover crops to increase SOC sequestration will require strategic implementation of management considerations related to net primary productivity, fertility, plant community composition, termination method and residue management, and soil disturbance regime. Ultimately, the SOC sequestration potential of cover cropping will be determined by cumulative effects of climate and management on organic input quantity and quality, input and output flux rates, and the spatial distribution of deposits within the soil profile. Soil management practices also induces shifts in the physical habitat and resource availability of microbial communities and therefore CUE (Six et al., 2006). Here we consider cover crop selection and management approaches that could increase the efficacy of cover cropping in Mediterranean croplands for C sequestration (Table 1).
Table 1. Summary table of considerations for cover crop management to promote soil organic carbon sequestration.
Current understanding of SOC fluxes and sequestration mechanisms highlights the key role of belowground structure and soluble plant inputs in regulating soil and microbial processes associated with long term SOC storage. Strategic integration of cover crop functional groups has been shown to partially regulate SOC storage potential. Since a broad range of species can be cultivated as cover crops in Mediterranean agroecosystems, informing species selection and community assembly with the specific root functional traits shown to increase SOC and stabilization potential offers new opportunities to optimize management for long-term C storage (Poirier et al., 2018).
Plant growth strategies and root traits have significant legacy effects on soil C storage through nutrient uptake dynamics, quantity and quality of organic inputs and shaping of active microbial communities through exudation (De Deyn et al., 2008; Cantarel et al., 2015; Guyonnet et al., 2018). Frameworks examining linkages between root functional traits and C storage pathways have shown that root tissue chemical recalcitrance promotes MAOM formation while morphological traits seldom relate to stabilization mechanisms, except for rooting depth which is an important trait controlling root-C storage and stability in the subsoil (Poirier et al., 2018). Developing cover crop systems with high root depth distribution results in greater contact between root tissues and mineral surfaces in deeper soil layers and favor stable MAOM associations on less saturated mineral surfaces (Kell, 2011). Diverse species mixtures are increasingly favored to increase the array of beneficial functions one can obtain from cover crops. A positive relationship between plant diversity and SOC sequestration (De Deyn et al., 2008; Fornara and Tilman, 2008; Cong et al., 2014) has been demonstrated, especially in extensively managed systems.
Growing deep-rooted species with species that colonize complementary spatial-temporal niches could favor formation of both MAOM at depth and POM in the topsoil through a greater diversity of root traits, impacts on soil structure and spatial and temporal C inputs to soil ecosystems (Cong et al., 2014; DuPont et al., 2014; Lange et al., 2015; Kravchenko et al., 2019). For instance, species differently impact structural stability of soil particles important for POM storage. Whereas, deep rooted species with coarse roots can increase soil bulk density adjacent to the root, smaller diameter roots can decrease bulk density by increasing soil porosity (Gyssels et al., 2005). Species with denser, finer root systems such as grasses bind soil more effectively than do large taproot systems (Loades et al., 2010), leading to greater macro aggregation in topsoil and accumulation of labile SOC in both macro and micro aggregates (Angers and Caron, 1998). Mixtures of grasses and legumes may be particularly effective and could include species with functional traits attributed to acquisitive strategies such as combining deep-rooted legume species i.e., alfalfa (Medicago sativa) and faba beans (Vicia faba) with commonly managed grass species with extensive shallower fibrous root structures. Deeply rooted C4 grasses and shallow rooted legumes are also highly complementary functional groups with high C storage potential (Fornara and Tilman, 2008).
Prioritizing species mixtures could also be beneficial to enhance the amount and diversity of labile C inputs and microbial populations instrumental in building both POC and MAOM fractions (Lange et al., 2015; Tiemann et al., 2015). The amount and composition of root C efflux via root respiration, exudation and rhizodeposition vary among plant species (Bais et al., 2006; Herz et al., 2018; Dietz et al., 2020; Henneron et al., 2020) and it is likely possible to manipulate the abundance and composition of root exudates by modifying species composition. In general, in an agricultural context, choosing acquisitive growth types according to plant economic spectrum might be more suitable and effective for SOC storage than slow growing conservative species, since the higher photosynthesis and nutrient demand of acquisitive species triggers greater rhizodeposition (Henneron et al., 2020). However, our understanding of exudation patterns and quality in agricultural systems is very limited and a major knowledge gap in designing plant covers that harness this potential for long term SOC storage. Plants species that release more exudates will probably enhance their impact on aggregation and SOC occlusion pathways, along with species that exude larger molecules that can act as binding agents (Whalley et al., 2005). Root exudates from legumes are of particular interest since they have high concentrations of N-rich compounds compared to other species (Fustec et al., 2010) which can be rapidly assimilated in microbial biomass, increasing microbial CUE and MAOM content (de Neergaard and Gorissen, 2004; Cotrufo et al., 2013; Kopittke et al., 2020). Legumes ability to provide adequate N requirements for maximizing C storage often leads to greater SOC contents and accumulation of SOC is frequently observed across contexts and in macroaggregate shortly after legume growth (Topps et al., 2021). Legumes in cover crop mixtures with grasses are often found beneficial to cover crop productivity and C input from biomass (Fornara and Tilman, 2008; Freund et al., 2021).
Favoring species with beneficial biotic root traits can also impact SOC quantity and persistence through association with differing rhizosphere communities and mycorrhizal fungi (Langley et al., 2006; Averill et al., 2014). For instance, plants in symbiosis with ectomycorrhizal fungi, which lower decomposer activity by outcompeting them for N, store largely more SOC than arbuscular mycorrhizal associated plants. It has also been proposed that species with root traits that stimulate the growth of fungi over bacteria, such as high lignin and low root N content can promote SOC sequestration (De Deyn et al., 2008; Bardgett et al., 2013; Poirier et al., 2018).
This view is consistent with recent knowledge that fungal structures reside longer in soil whereas bacterial membranes are more quickly mineralized (Domeignoz-Horta et al., 2021). As such, bacteria-only communities can lead to more unstable and labile SOC pools compared to communities with bacteria and fungi (Domeignoz-Horta et al., 2021). Rhizobia in legumes can also promote aggregate formation and stability through the binding action of rhizobia exocellular polysaccharides (Haynes and Beare, 1997; Alami et al., 2000).
Research in extensively managed grassland systems shows that more diverse plant covers lead to more rapid annual carbon accumulation rates compared to monocultures thanks to greater above and belowground C inputs and soil N mineralization which fuel the soil microbial pump and prime positive diversity–productivity relationship (Cong et al., 2014; Yang et al., 2019). Promoting a diverse array of C inputs through diversifying crop rotations with cover crops and adopting diverse cover crop species mixtures likely provide various resources that better optimize CUE (Kallenbach et al., 2019). In mild Mediterranean climates, extending the growth period of active living roots and inputs of C into the soil through cover cropping will also increase the period where microbial communities remain active. Mitigating long microbial dormancy periods may improve CUE over time (Kallenbach et al., 2019). Either way, maintaining more prolonged periods of metabolic activity annually, even with lower CUE, would presumably result in more microbially-derived C (necromass) than management strategies that facilitate low C inputs, high loss pathways, and longer periods of microbial dormancy, which may be typical of conventional systems (Kallenbach et al., 2019).
However, linkages between cover crop diversity, species choice and SOC outcomes remain to be tested in Mediterranean agroecosystems. Root functional frameworks and characterization of common cover crop species can inform cover crop management (Tribouillois et al., 2015; Wang et al., 2021) but outcomes remain context specific, especially when considering plastic root traits which vary considerably within species in response to resource gradients and ecological interactions. Cover crop species choice is also a function of other system management goals that must be reconciled with desired C storage outcomes. For instance, planting leguminous crops is preferred to maintain or increase soil N content (Ordóñez-Fernández et al., 2018), while other cover crop types such as grasses and brassicas might be useful for scavenging nutrients, increasing biomass inputs, and reducing N leaching. Finally, modifications of soil properties regulating SOC storage, including microbial communities, are strongly linked to cover crop management and edaphic properties, in some cases more so than species choice and diversity (Romdhane et al., 2019; Cloutier et al., 2020).
Adjusting cover crop growth duration by selecting cover crop planting and termination times is an intrinsic consideration when managing cover crops (Alonso-Ayuso et al., 2014), especially in Mediterranean agroecosystems which may facilitate a wide range of growth periods. For the objective of increasing SOC sequestration, cover crop growth duration is the most important predictor of SOC responses to cover cropping, as was recently highlighted in a meta-analysis study (McClelland et al., 2021). This importance is likely due to interactions between the cover crop growth period and other factors like plant establishment, plant biomass (McClelland et al., 2021) and residue quality (Clark et al., 1994; Alonso-Ayuso et al., 2014).
Organic C preservation depends on the balance between SOC stabilization and destabilization mechanisms (Janzen, 2006; Harden et al., 2018; Bailey et al., 2019), and litter quality affects the balance between these two mechanisms (Bailey et al., 2019). For example, high quality residues characterized by low C:N ratios or the predominance of simple compounds, have a high microbial CUE (Cotrufo et al., 2013), where the resulting microbial by-products can be efficiently stabilized within the MAOM pool (Six et al., 2002; von Lützow et al., 2007; Cotrufo et al., 2013). These same labile products can act as destabilizing agents via the priming effect (Jobbágy and Jackson, 2000; Fontaine et al., 2007; Bailey et al., 2019). Therefore, we propose that adjusting cover crop growth duration as a function of litter quality and SOC saturation status could contribute toward balancing stabilization and destabilization mechanisms, hence optimizing SOC sequestration through proper cover crop management. Cover crop termination at early stages of plant development may result in higher plant litter quality that is more easily assimilated by microbes. As a result, the increased microbial necromass is likely to favor MAOM stabilization mechanisms. After MAOM saturation, further accrual of SOC will shift to POM stabilization mechanisms. Thus, at this stage, cover crop management practices that favor promotion of aggregation will become the dominant pathway for continued SOC sequestration. However, more studies are needed to effectively assess cover crop termination time with regards to SOC stabilization and destabilization mechanisms across contexts. In particular, the impacts of soil fertility on plant growth and optimal termination for SOC accrual remain elusive, yet critical to promote plant allocation of carbon to preferential storage flows, especially belowground.
Recent literature suggests that conditions that repress aboveground growth, while still allowing photosynthesis to continue, facilitates the allocation of surplus photosynthates toward exudation and root structures (Prescott et al., 2020). This is especially relevant when considering strategic nitrogen (N) management. For instance, the beneficial effects of N availability for MAOM formation may be counteracted as N fertilizers also reduce biomass allocation toward roots and therefore decrease belowground C deposition (Bonifas et al., 2005; Lazicki et al., 2016; Pausch and Kuzyakov, 2018; Ordóñez et al., 2021), the quantity of exudates produced (Kaštovská et al., 2017; Mortensen et al., 2021), and plant C flux rates toward soil communities (Gorka et al., 2019). Another strategic consideration is that the release of exudates appears to be maximized in leguminous species when phosphorous (P) is limited (Cardenas et al., 2021). Likewise, moderate drought can increase root exudation and rhizodeposition (Karlowsky et al., 2018). Managing cover crop growth conditions to favor photosynthate transport belowground in consideration of root growth, exudation, and the spatial hotspots of microbial activity (such as within the rhizosphere) may therefore be an important regulation factor to maximize SOC stabilization outcomes.
Alternatively, maintaining sufficient soil N availability over the long-term has been shown as essential toward increasing both MOAM and POM (Spohn et al., 2016; Ordóñez et al., 2021; Rocci et al., 2021). This may be especially true when targeting increases in aboveground productivity and POM accumulation, such as when MOAM storage thresholds are near saturation (Rocci et al., 2021). Additionally, higher N fertility suppresses the breakdown of lignin in plant residues, which slows decompositional processes and may increase POM persistance (Talbot and Treseder, 2012; Li et al., 2017). Managing for optimal N fertility is essential toward improving microbial CUE and, therefore, the efficiency of SOC formation (Li et al., 2017; Mahal et al., 2019; Ordóñez et al., 2021). Optimizing SOC benefits of cover cropping will therefore require more precise nutrient management approaches that balance higher nutrient requirements of the crop, for optimizing aboveground productivity, with maintaining lower, yet sufficient, nutrient status during the cover crop phase to optimize belowground productivity and SOC sequestration. Deciding on the growth period of cover crops could be considered a potential management tool to optimize SOC preservation in croplands with Mediterranean climate, especially with respect to fertility management, the promotion of belowground productivity, and optimizing litter quality inputs. This decision is site-specific and depends on intrinsic soil characteristics, as well as external factors such as climate and other co-management variables. Hence, more research is needed in order to delineate easy-to-use indicators that would guide farmers' decisions to choose proper cover crop plantation and termination times.
The design and application of cover crop termination strategies are a readily accessible tool available for growers to optimize cover cropping for increased C sequestration. Modes of cover crop termination may be classified into three broad categories that include chemical (herbicides), mechanical, and natural methods. Mechanical termination methods have the broadest applications and include mowing, rolling/crimping, and a wide array of tillage options. Natural termination methods may include planting of winter-kill species (though not common in Mediterranean climates) and the use of animal grazing on residues. Cover crop residue management is an additional consideration and options may include retention on the soil surface, incorporation into soil profile, and/or biomass removal through grazing or harvesting. The various combinations of management choices have significant impacts on the quantity and quality of above- and belowground carbon deposition, as well as the spatial distribution dynamics of deposited organic and inorganic inputs. Linking cover crop management with SOC accumulation and persistence frameworks should guide our efforts to adapt cover crop termination methods with the objective of sequestering more carbon and ensuring its long-term storage.
A predominant determinant of cover cropping efficacy with respect to SOC sequestration is the choice to retain or incorporate residues upon termination. The choice to incorporate residues is done through the use of tillage, which may occur for several reasons including to mix organic and inorganic materials throughout the soil profile, mitigate weed pressure, and/or break apart soil surface compaction for improved planting and water infiltration (Derpsch, 2003; Derpsch et al., 2014). Yet, while tillage facilitates the incorporation of aboveground residue-derived POM throughout the soil profile (Duval et al., 2016; Schaefer et al., 2020), heavy tillage has also been shown to stimulate SOC losses through disrupting aggregate stability and increasing microbial access to previously occluded intra-aggregate POM (Franzluebbers et al., 1999; Six et al., 2000; Salvo et al., 2010; Poeplau and Don, 2015). Heavy tillage is also associated with significantly higher rates of SOC loss via surface erosion (Van Oost et al., 2006). To this extent, POM is actually most often lower in the surface soils of conventionally tilled systems, despite this increased incorporation of aboveground cover crop residues (Motta et al., 2007; Olchin et al., 2008; Franzluebbers and Stuedemann, 2014; Jilling et al., 2020). Though more variable, the use of tillage is also sometimes associated with reductions in MAOM (Salvo et al., 2010; Jilling et al., 2020). These factors therefore contribute to a well-established negative relationship between conventional tillage and total SOC stocks of surface soils when compared to no-till and conservation tillage strategies, particularly within the tillage zone where significant disturbance occurs (Hobbs et al., 2008; Salvo et al., 2010; Franzluebbers and Stuedemann, 2014; Higashi et al., 2014; Poeplau and Don, 2015; Nunes et al., 2020; McClelland et al., 2021; Wulanningtyas et al., 2021). Notably, our understanding of tillage dynamics is limited by the tendency to discuss the various types of conversation tillage, and their underlying impacts, as a monolith rather than as a dynamic tool that differs in application and subsequent SOC dynamics (Derpsch et al., 2014).
To this extent, the impacts of various tillage regimes on sub-surface SOC storage are a current point of controversy. A growing body of evidence has observed decreased SOC stocks in subsoils with the conversion from conventional tillage to no-till management of cover crops (Franzluebbers and Stuedemann, 2014; Camarotto et al., 2020). While the underlying mechanisms are not yet well-understood, this decrease in deeper SOC sequestration during the transition toward no-till may result from reductions in cover crop establishment and subsequent decreases in above- and belowground biomass production (Constantin et al., 2010; Camarotto et al., 2020). This may potentially be remediated by some conservation tillage methods (Franzluebbers et al., 1999; Jilling et al., 2020), increasing cover crop seeding rates, and/or extending the cover crop cultivation window. Further, it may also be that the negative subsoil SOC response with the conversion to no-till results partially from increases in SOC priming, which may be especially prevalent within conventionally managed systems with significant soluble N inputs (Duval et al., 2016; Tautges et al., 2019; Camarotto et al., 2020). Some research suggests that this same priming response may be mitigated when organic inputs, such as compost, are paired with cover cropping in both tilled and no-till systems (Fronning et al., 2008; Liang et al., 2018; Tautges et al., 2019; Schaefer et al., 2020; White et al., 2020; Rath et al., 2021).
Though significantly understudied in agricultural systems, residue incorporation may also occur through bioturbation and the physical transport of materials deeper into the soil profile (Lavelle et al., 2006; Frouz et al., 2009). While the negative impacts of tillage on soil fauna populations are well documented (House and Parmelee, 1985; Emmerling, 2001), less is understood on how this directly impacts the transport dynamics of SOC throughout agroecosystem soils. Nevertheless, retention of surface residues has been shown as essential to the prosperity of soil fauna communities (Tian et al., 1995; Lavelle et al., 2006, 2016), and research has increasingly shown the role these organisms may play in SOC cycling within natural ecosystems (Filser et al., 2016). While further research is necessary, this may partially explain why POM stocks are often found to be higher in surface soils under no-till with residue retention strategies when compared to residue incorporation via tillage. Notably, there is scarce research conducted on the impacts of lower frequency tillage events. Thus, our understanding of the benefits and/or tradeoffs associated with the infrequent use of tillage in more temporally dynamic cover crop termination rotations are not well-understood. Ultimately, keeping cover crop residues on-field, whether through promoting residue retention on the soil surface or through incorporation, has been shown as essential to improving SOC storage (Kan et al., 2020; Wang et al., 2020; Zhao et al., 2020).
Yet, residue surface retention strategies, such as herbicides, mowing, and roller-crimping, have shown a wide array of benefits associated with the “soil armor” effect of reducing bare soil exposure. This includes increases in SOC (Turmel et al., 2015; Chen et al., 2017; Alam et al., 2018; Li et al., 2019; Sharma et al., 2019; Wang et al., 2019; Zhao et al., 2020) and soil structure (Chen et al., 2017; Li et al., 2019; Wang et al., 2019) that, in tandem with the insulating effect of residue layers, improves the regulation of soil temperature (Schonbeck and Evanylo, 1998; Sarkar and Singh, 2007) and retention of soil water (Schonbeck and Evanylo, 1998; Sarkar and Singh, 2007; Turmel et al., 2015; Yin et al., 2020; Zhao et al., 2020). Some studies have shown a decrease in soil carbon priming with the interaction of no-till and residue retention strategies when compared to residue incorporation (Kan et al., 2020; Mo et al., 2021). Residue incorporation may, in fact, only further increase SOC priming as the quantity of biomass incorporated increases (Shahbaz et al., 2017a,b). However, little is known about the varying relative impacts of chopping (via mowing) and matting (via roller-crimping) of cover crop residues. The relative effects of evenly distributing or spatially concentrating residues are also not well-understood. Residue retention strategies vary widely in application, both in time and space. Yet, the spatial and temporal diversity of these strategies are not captured well in research, and instead are often conflated and inaccurately represented as a single management practice rather a suite of management options. Thus, the best management practices are not yet well-identified in Mediterranean climates.
Depending on the cover crop composition, one of the drawbacks associated with surface residue retention is potential reductions in available N (Ordóñez-Fernández et al., 2018; Jani et al., 2019; Hefner et al., 2020). This may both reduce crop productivity (Hefner et al., 2020) and stoichiometrically limit stable SOC formation (Bertrand et al., 2019), though these outcomes are likely highly dependent on residue nutrient content and carbon quality (Bertrand et al., 2019; Mo et al., 2021) and, as previously mentioned, may be a strategic benefit for SOC stabilization in cropland soils with high N application rates. Where N availability places stoichiometric limitations, this may potentially be addressed through increasing the planting of leguminous species thereby improving the N content and mineralization rates of surface retained residues (Büchi et al., 2018; De Notaris et al., 2020). The CUE of cover crop residues may also be increased through the strategic application of nutrients, whether during the cash crop or cover crop phase, to help balance stoichiometric needs between C and other essential nutrients that may limit microbial assimilation and anabolism of C substrates.
Another option may be the strategic introduction of animal grazing into cover cropping rotations. While grazing animals on cropland, especially as a cover crop termination method, is fairly understudied, there is some validation that well-managed cropland grazing may increase soil C sequestration (Franzluebbers and Stuedemann, 2010; Fultz et al., 2013; Assmann et al., 2014; Cecagno et al., 2018; Bieluczyk et al., 2020; Brewer and Gaudin, 2020; Liebig et al., 2020; Sekaran et al., 2021; Zani et al., 2021). Animal grazing of cover crops alters carbon and nutrient input flows into the soil through the transformation of aboveground residues into soluble, nutrient-rich, and labile animal excreta, where the DOC and nutrients are stoichiometrically decoupled and more easily diffused and transported throughout the soil profile (Carvalho et al., 2010; Ramos et al., 2010; Rumpel and Kögel-Knabner, 2011; Rumpel et al., 2015; Eldridge et al., 2017; Sekaran et al., 2021). Soil carbon and nutrient inputs from grazing may therefore be both more readily available for plant uptake (Holford, 1980; Lemaire et al., 2014; Zhou et al., 2017) and easily transformed by soil microbes (Acosta-Martínez et al., 2004; Wilson et al., 2018; Sekaran et al., 2021), potentially facilitating a more direct pathway toward long-term MAOM stabilization and persistence (Cotrufo et al., 2013; Mosier et al., 2021). However, differences in MAOM are often insignificant between grazed and ungrazed cropland (Salvo et al., 2010; Assmann et al., 2014; Zani et al., 2021) and long-term grazing can reduce total soil N content over time through export within animal biomass.
Grazing, especially when conducted multiple times throughout the cover crop growing season, may also result in both changes in cover crop biomass productivity and a reallocation of resources above- and belowground (Dawson et al., 2000; Hamilton et al., 2008; Zhou et al., 2017). Multiple studies have shown an increase in cover crop root biomass (Piñeiro et al., 2010; Assmann et al., 2014; Chen et al., 2015) and rhizodeposition of labile carbon (Dawson et al., 2000; Wilson et al., 2018) with the introduction of grazing. The magnitude and direction of SOC impacts will be determined by the frequency and intensity (density and duration) under which grazing occurs. Low intensity stocking rates and/or less frequent grazing events have broadly shown positive results for SOC sequestration (Conant et al., 2003; Teague et al., 2011; Assmann et al., 2014; Chen et al., 2015; Zhou et al., 2017; Alves et al., 2020; Mosier et al., 2021). Thus, optimizing best management practices for cover crop grazing will require strategic consideration of site-specific thresholds at which high intensity grazing becomes negatively associated with SOC storage (Teague et al., 2011; McSherry and Ritchie, 2013; Chen et al., 2015; Plaza-Bonilla et al., 2015).
In the final analysis, cover crop termination and residue management schemes may best be designed in a way to avoid prescriptive implementation—utilizing the same regime year-after-year regardless of changes in climatic, ecological, and soil biogeochemical characteristics—and instead applied strategically in a way to adapt to these same changes over time. As such, a strategic and temporally dynamic termination rotation of residue retention (via mowing, herbicides, or roller-crimping), residue incorporation (via conservation tillage), and residue grazing practices may yield the best results for cover crop management with respect to increasing SOC sequestration. This may be guided by the simultaneous goals of maintaining continuous inputs of shoot and root-derived C, diversifying the spatial and temporal input of shoot-derived C, reducing soil disturbance, and improving soil microbial efficiency through balancing the C-to-nutrient stoichiometric demands of SOC formation processes (Ferreira et al., 2018; Bertrand et al., 2019).
We considered cover crop selection and management approaches that could increase the effectiveness of cover cropping in the Mediterranean croplands for C sequestration following three main guiding principles (Table 1). However, significant gaps remain in understanding best management practices for C accrual, especially in Mediterranean systems. We highlight the need for:
1) Clearer SOC measurements and monitoring in these systems to guide cover crop management efforts toward targeting increases in both POM and MAOM fractions (when under mineral C saturation thresholds), or maintaining MAOM content while increasing the POM fraction (when at mineral C saturation thresholds);
2) Specifically outlining the determining root functional traits associated with SOC storage in both POM and MAOM fractions, and identify cover crop species with greater contributions from belowground fractions.
3) Synthesis of depth dependent SOC stabilization and destabilization mechanisms in the MAOM fraction, and interpretation and application of cover crop management strategies that may shift the equilibrium between these two processes toward net stabilization.
Considering the evolution of SOC sequestration theory, we see opportunities for growers to implement new cover crop management strategies that capitalize on this knowledge. In-turn, as these strategies become standard, more systematic evaluations in field settings across soils and systems in Mediterranean climates will occur and advance our understanding of agriculturally managed SOC sequestration.
NM, KB, AG, and AO'G wrote, read, reviewed, edited, and approved the final version of the manuscript. All authors contributed to the article and approved the submitted version.
The authors declare that the research was conducted in the absence of any commercial or financial relationships that could be construed as a potential conflict of interest.
All claims expressed in this article are solely those of the authors and do not necessarily represent those of their affiliated organizations, or those of the publisher, the editors and the reviewers. Any product that may be evaluated in this article, or claim that may be made by its manufacturer, is not guaranteed or endorsed by the publisher.
The authors would like to thank the Charlesworth Author Services for providing figure and reference formatting services.
Acosta-Martínez, V., Zobeck, T. M., and Allen, V. (2004). Soil microbial, chemical and physical properties in continuous cotton and integrated crop–livestock systems. Soil Sci. Soc. Am. J. 68, 1875–1884. doi: 10.2136/sssaj2004.1875
Aguilera, E., Lassaletta, L., Gattinger, A., and Gimeno, B. S. (2013). Managing soil carbon for climate change mitigation and adaptation in Mediterranean cropping systems: a meta-analysis. Agric. Ecosyst. Environ. 168, 25–36. doi: 10.1016/j.agee.2013.02.003
Alam, K. M., Bell, R. W., Haque, M. E., and Kader, M. A. (2018). Minimal soil disturbance and increased residue retention increase soil carbon in rice-based cropping systems on the Eastern Gangetic Plain. Soil Tillage Res. 183, 28–41. doi: 10.1016/j.still.2018.05.009
Alami, Y., Achouak, W., Marol, C., and Heulin, T. (2000). Rhizosphere soil aggregation and plant growth promotion of sunflowers by an exopolysaccharide-producing Rhizobium sp. strain isolated from sunflower roots. Appl. Environ. Microbiol. 66, 3393–3398. doi: 10.1128/AEM.66.8.3393-3398.2000
Alonso-Ayuso, M., Gabriel, J. L., and Quemada, M. (2014). The kill date as a management tool for cover cropping success. PLoS ONE 9:e109587. doi: 10.1371/journal.pone.0109587
Alves, L. A., Denardin, L. G. O., Martins, A. P., Bayer, C., Veloso, M. G., Bremm, C., et al. (2020). The effect of crop rotation and sheep grazing management on plant production and soil C and N stocks in a long-term integrated crop-livestock system in Southern Brazil. Soil Tillage Res. 203:104678. doi: 10.1016/j.still.2020.104678
Angers, D. A., and Caron, J. (1998). Plant-induced changes in soil structure: processes and feedbacks. Biogeochemistry 45, 55–72. doi: 10.1023/A:1005944025343
Angers, D. A., Recous, S., and Aita, C. (1997). Fate of carbon and nitrogen in water-stable aggregates during decomposition of 13C15N-labelled wheat straw in situ. Eur. J. Soil Sci. 48, 295–300. doi: 10.1111/j.1365-2389.1997.tb00549.x
Angst, G., Mueller, K. E., Nierop, K. G. J., and Simpson, M. J. (2021). Plant- or microbial-derived? A review on the molecular composition of stabilized soil organic matter. Soil Biol. Biochem. 156:108189. doi: 10.1016/j.soilbio.2021.108189
Aschmann, H.. (1984). A restrictive definition of mediterranean climates. Bull. Soc. Bot. Fr. Actual. Bot. 131, 21–30. doi: 10.1080/01811789.1984.10826643
Assmann, J. M., Anghinoni, I., Martins, A. P., Costa, S. E. V. G. A., Cecagno, D., Carlos, F. S., et al. (2014). Soil carbon and nitrogen stocks and fractions in a long-term integrated crop–livestock system under no-tillage in southern Brazil. Agric. Ecosyst. Environ. 190, 52–59. doi: 10.1016/j.agee.2013.12.003
Austin, E. E., Wickings, K., McDaniel, M. D., Robertson, G. P., and Grandy, A. S. (2017). Cover crop root contributions to soil carbon in a no-till corn bioenergy cropping system. GCB Bioenergy 9, 1252–1263. doi: 10.1111/gcbb.12428
Averill, C., Turner, B. L., and Finzi, A. C. (2014). Mycorrhiza-mediated competition between plants and decomposers drives soil carbon storage. Nature 505, 543–545. doi: 10.1038/nature12901
Bailey, V. L., Pries, C. H., and Lajtha, K. (2019). What do we know about soil carbon destabilization? Environ. Res. Lett. 14:083004. doi: 10.1088/1748-9326/ab2c11
Bais, H. P., Weir, T. L., Perry, L. G., Gilroy, S., and Vivanco, J. M. (2006). The role of root exudates in rhizosphere interactions with plants and other organisms. Annu. Rev. Plant Biol. 57, 233–266. doi: 10.1146/annurev.arplant.57.032905.105159
Bardgett, R. D., Manning, P., Morriën, E., and De Vries, F. T. (2013). Hierarchical responses of plant–soil interactions to climate change: consequences for the global carbon cycle. J. Ecol. 101, 334–343. doi: 10.1111/1365-2745.12043
Barré, P., Quénéa, K., Vidal, A., Cécillon, L., Christensen, B. T., Kätterer, T., et al. (2018). Microbial and plant-derived compounds both contribute to persistent soil organic carbon in temperate soils. Biogeochemistry 140, 81–92. doi: 10.1007/s10533-018-0475-5
Basile-Doelsch, I., Balesdent, J., and Pellerin, S. (2020). Reviews and syntheses: the mechanisms underlying carbon storage in soil. Biogeosciences 17, 5223–5242. doi: 10.5194/bg-17-5223-2020
Baumert, V. L., Vasilyeva, N. A., Vladimirov, A. A., Meier, I. C., Kögel-Knabner, I., and Mueller, C. W. (2018). Root exudates induce soil macroaggregation facilitated by fungi in subsoil. Front. Environ. Sci. 6:140. doi: 10.3389/fenvs.2018.00140
Berg, B., and Meentemeyer, V. (2002). Litter quality in a north European transect vs. carbon storage potential. Plant Soil 242, 83–92. doi: 10.1023/A:1019637807021
Bertrand, I., Viaud, V., Daufresne, T., Pellerin, S., and Recous, S. (2019). Stoichiometry constraints challenge the potential of agroecological practices for the soil C storage. A review. Agron. Sustainable Dev. 39:54. doi: 10.1007/s13593-019-0599-6
Bieluczyk, W., Piccolo, M. C., Pereira, M. G., Moraes, M. T., Soltangheisi, A., Bernardi, A. C. C., et al. (2020). Integrated farming systems influence soil organic matter dynamics in southeastern Brazil. Geoderma 371:114368. doi: 10.1016/j.geoderma.2020.114368
Bongiovanni, M. D., and Lobartini, J. C. (2006). Particulate organic matter, carbohydrate, humic acid contents in soil macro- and microaggregates as affected by cultivation. Geoderma 136, 660–665. doi: 10.1016/j.geoderma.2006.05.002
Bonifas, K. D., Walters, D. T., Cassman, K. G., and Lindquist, J. L. (2005). Nitrogen supply affects root:shoot ratio in corn and velvetleaf (Abutilon theophrasti). Weed Sci. 53, 670–675. doi: 10.1614/WS-05-002R.1
Bottinelli, N., Jouquet, P., Capowiez, Y., Podwojewski, P., Grimaldi, M., and Peng, X. (2015). “Why is the influence of soil macrofauna on soil structure only considered by soil ecologists?,” in Soil and Tillage Research, Soil Structure and its Functions in Ecosystems: Phase Matter & Scale Matter, eds X. Peng, R. Horn, and R. Hallett (Soil and Tillage Research), 118–24. doi: 10.1016/j.still.2014.01.007
Brewer, K. M., and Gaudin, A. C. M. (2020). Potential of crop-livestock integration to enhance carbon sequestration and agroecosystem functioning in semi-arid croplands. Soil Biol. Biochem. 149:107936. doi: 10.1016/j.soilbio.2020.107936
Büchi, L., Wendling, M., Amossé, C., Necpalova, M., and Charles, R. (2018). Importance of cover crops in alleviating negative effects of reduced soil tillage and promoting soil fertility in a winter wheat cropping system. Agric. Ecosyst. Environ. 256, 92–104. doi: 10.1016/j.agee.2018.01.005
Butman, D. E., Wilson, H. F., Barnes, R. T., Xenopoulos, M. A., and Raymond, P. A. (2015). Increased mobilization of aged carbon to rivers by human disturbance. Nat. Geosci. 8, 112–116. doi: 10.1038/ngeo2322
Camarotto, C., Piccoli, I., Ferro, N. D., Polese, R., Chiarini, F., Furlan, L., et al. (2020). Have we reached the turning point? Looking for evidence of SOC increase under conservation agriculture and cover crop practices. Eur. J. Soil Sci. 71, 1050–1063. doi: 10.1111/ejss.12953
Cambardella, C. A., and Elliott, E. T. (1993). “Methods for physical separation and characterization of soil organic matter fractions,” in Soil Structure/Soil Biota Interrelationships, eds L. Brussaard, and M. J. Kooistra (Amsterdam: Elsevier), 449–457. doi: 10.1016/B978-0-444-81490-6.50036-4
Canarini, A., Kaiser, C., Merchant, A., Richter, A., and Wanek, W. (2019). Root exudation of primary metabolites: mechanisms and their roles in plant responses to environmental stimuli. Front. Plant Sci. 10:157. doi: 10.3389/fpls.2019.00157
Cantarel, A. A. M., Pommier, T., Desclos-Theveniau, M., Diquélou, S., Dumont, M., Grassein, F., et al. (2015). Using plant traits to explain plant–microbe relationships involved in nitrogen acquisition. Ecology 96, 788–799. doi: 10.1890/13-2107.1
Cardenas, J., Santa, F., and Kaštovská, E. (2021). The exudation of surplus products links plant functional traits and plant-microbial stoichiometry. Land 10:840. doi: 10.3390/land10080840
Carvalho, J. L. N., Raucci, G. S., Cerri, C. E. P., Bernoux, M., Feigl, B. J., Wruck, F. J., et al. (2010). Impact of pasture, agriculture and crop-livestock systems on soil C stocks in Brazil. Soil Tillage Res. 110, 175–186. doi: 10.1016/j.still.2010.07.011
Castellano, M. J., Mueller, K. E., Olk, D. C., Sawyer, J. E., and Six, J. (2015). Integrating plant litter quality, soil organic matter stabilization, and the carbon saturation concept. Glob. Chang. Biol. 21, 3200–3209. doi: 10.1111/gcb.12982
Cecagno, D., Gomes, M. V., Costa, S. E. V. G. A., Martins, A. P., Denardin, L. G. O., Bayer, C., et al. (2018). Soil organic carbon in an integrated crop-livestock system under different grazing intensities. Rev. Bras. Cienc. Agrar. 13, 1–7. doi: 10.5039/agraria.v13i3a5553
Celette, F., Findeling, A., and Gary, C. (2009). Competition for nitrogen in an unfertilized intercropping system: the case of an association of grapevine and grass cover in a Mediterranean climate. Eur. J. Agron. 30, 41–51. doi: 10.1016/j.eja.2008.07.003
Chen, W., Huang, D., Liu, N., Zhang, Y., Badgery, W. B., Wang, X., et al. (2015). Improved grazing management may increase soil carbon sequestration in temperate steppe. Sci. Rep. 5:10892. doi: 10.1038/srep10892
Chen, Z., Ti, J., and Chen, F. (2017). Soil aggregates response to tillage and residue management in a double paddy rice soil of the Southern China. Nutri. Cycl. Agroecosyst. 109, 103–114. doi: 10.1007/s10705-017-9864-8
Chiti, T., Gardin, L., Perugini, L., Quaratino, R., Vaccari, F. P., Miglietta, F., et al. (2012). Soil organic carbon stock assessment for the different cropland land uses in Italy. Biol. Fertil. Soils 48, 9–17. doi: 10.1007/s00374-011-0599-4
Clark, A. J., Decker, A. M., and Meisinger, J. J. (1994). Seeding rate and kill date effects on hairy vetch-cereal rye cover crop mixtures for corn production. Agron. J. 86, 1065–1070. doi: 10.2134/agronj1994.00021962008600060025x
Cloutier, M. L., Murrell, E., Barbercheck, M., Kaye, J., Finney, D., García-González, I., et al. (2020). Fungal community shifts in soils with varied cover crop treatments and edaphic properties. Sci. Rep. 10:6198. doi: 10.1038/s41598-020-63173-7
Collins, H. P., Christenson, D. R., Blevins, R. L., Bundy, L. G., Dick, W. A., Huggins, D. R., et al. (1999). Soil carbon dynamics in corn-based agroecosystems: results from carbon-13 natural Aaundance. Soil Sci. Soc. Am. J. 63, 584–591. doi: 10.2136/sssaj1999.03615995006300030022x
Conant, R. T., Six, J., and Paustian, K. (2003). Land use effects on soil carbon fractions in the southeastern United States. I. Management-intensive vs. extensive grazing. Biol. Fertil. Soils 38, 386–392. doi: 10.1007/s00374-003-0652-z
Cong, W.-F., van Ruijven, J., Mommer, L., De Deyn, G. B., Berendse, F., and Hoffland, E. (2014). Plant species richness promotes soil carbon and nitrogen stocks in grasslands without legumes. J. Ecol. 102, 1163–1170. doi: 10.1111/1365-2745.12280
Constantin, J., Mary, B., Laurent, F., Aubrion, G., Fontaine, A., Kerveillant, P., et al. (2010). Effects of catch crops, no till and reduced nitrogen fertilization on nitrogen leaching and balance in three long-term experiments. Agric. Ecosyst. Environ. 135, 268–278. doi: 10.1016/j.agee.2009.10.005
Cotrufo, M. F., Ranalli, M. G., Haddix, M. L., Six, J., and Lugato, E. (2019). Soil carbon storage informed by particulate and mineral-associated organic matter. Nat. Geosci. 12, 989–994. doi: 10.1038/s41561-019-0484-6
Cotrufo, M. F., Soong, J. L., Horton, A. J., Campbell, E. E., Haddix, M. L., Wall, D. H., et al. (2015). Formation of soil organic matter via biochemical and physical pathways of litter mass loss. Nat. Geosci. 8, 776–779. doi: 10.1038/ngeo2520
Cotrufo, M. F., Wallenstein, M. D., Boot, C. M., Denef, K., and Paul, E. (2013). The Microbial Efficiency-Matrix Stabilization (MEMS) framework integrates plant litter decomposition with soil organic matter stabilization: do labile plant inputs form stable soil organic matter? Glob. Chang. Biol. 19, 988–995. doi: 10.1111/gcb.12113
Dawson, L. A., Grayston, S. J., and Paterson, E. (2000). “Effects of grazing on the roots and rhizosphere of grasses,” in Grassland Ecophysiology and Grazing Ecology, eds G. Lemaire, J. Hodgson, A. de Moraes, C. Nabinger, and P. C. de F. Carvalho (Wallingford: CABI), 61–84. doi: 10.1079/9780851994529.0061
De Deyn, G. B., Cornelissen, J. H. C., and Bardgett, R. D. (2008). Plant functional traits and soil carbon sequestration in contrasting biomes. Ecol. Lett. 11, 516–531. doi: 10.1111/j.1461-0248.2008.01164.x
de Neergaard, A., and Gorissen, A. (2004). Carbon allocation to roots, rhizodeposits and soil after pulse labelling: a comparison of white clover (Trifolium repens L.) and perennial ryegrass (Lolium perenne L.). Biol. Fertil. Soils 39, 228–234. doi: 10.1007/s00374-003-0699-x
De Notaris, C., Olesen, J. E., Sørensen, P., and Rasmussen, J. (2020). Input and mineralization of carbon and nitrogen in soil from legume-based cover crops. Nutri. Cycl. Agroecosyst. 116, 1–18. doi: 10.1007/s10705-019-10026-z
Derpsch, R.. (2003). “Conservation tillage, no-tillage and related technologies,” in Conservation Agriculture: Environment, Farmers Experiences, Innovations, Socio-economy, Policy, eds L. García-Torres, J. Benites, A. Martínez-Vilela, and A. Holgado-Cabrera (Dordrecht: Springer Netherlands), 181–190. doi: 10.1007/978-94-017-1143-2_23
Derpsch, R., Franzluebbers, A. J., Duiker, S. W., Reicosky, D. C., Koeller, K., Friedrich, T., et al. (2014). Why do we need to standardize no-tillage research? Soil Tillage Res. 137, 16–22. doi: 10.1016/j.still.2013.10.002
Devine, S. M., Steenwerth, K. L., and O'Geen, A. T. (2021). A regional soil classification framework to improve soil health diagnosis and management. Soil Sci. Soc. Am. J. 85, 361–378. doi: 10.1002/saj2.20200
Dietz, S., Herz, K., Gorzolka, K., Jandt, U., Bruelheide, H., and Scheel, D. (2020). Root exudate composition of grass and forb species in natural grasslands. Sci. Rep. 10:10691. doi: 10.1038/s41598-019-54309-5
Dijkstra, F. A., Zhu, B., and Cheng, W. (2021). Root effects on soil organic carbon: a double-edged sword. New Phytol. 230, 60–65. doi: 10.1111/nph.17082
Domeignoz-Horta, L. A., Shinfuku, M., Junier, P., Poirier, S., Verrecchia, E., Sebag, D., et al. (2021). Direct evidence for the role of microbial community composition in the formation of soil organic matter composition and persistence. ISME Commun. 1, 1–4. doi: 10.1038/s43705-021-00071-7
DuPont, S. T., Beniston, J., Glover, J. D., Hodson, A., Culman, S. W., Lal, R., et al. (2014). Root traits and soil properties in harvested perennial grassland, annual wheat, and never-tilled annual wheat. Plant Soil 381, 405–420. doi: 10.1007/s11104-014-2145-2
Duval, M. E., Galantini, J. A., Capurro, J. E., and Martinez, J. M. (2016). Winter cover crops in soybean monoculture: effects on soil organic carbon and its fractions. Soil Tillage Res. 161, 95–105. doi: 10.1016/j.still.2016.04.006
Duval, M. E., Galantini, J. A., Iglesias, J. O., Canelo, S., Martinez, J. M., and Wall, L. (2013). Analysis of organic fractions as indicators of soil quality under natural and cultivated systems. Soil Tillage Res. 131, 11–19. doi: 10.1016/j.still.2013.03.001
Dynarski, K. A., Bossio, D. A., and Scow, K. M. (2020). Dynamic stability of soil carbon: reassessing the “permanence” of soil carbon sequestration. Front. Environ. Sci. 8:218. doi: 10.3389/fenvs.2020.514701
Eldridge, D. J., Delgado-Baquerizo, M., Travers, S. K., Val, J., and Oliver, I. (2017). Do grazing intensity and herbivore type affect soil health? Insights from a semi-arid productivity gradient. J. Appl. Ecol. 54, 976–985. doi: 10.1111/1365-2664.12834
Ellis, E. C., Goldewijk, K. K., Siebert, S., Lightman, D., and Ramankutty, N. (2010). Anthropogenic transformation of the biomes, 1700 to 2000. Glob. Ecol. Biogeogr. 19, 589–606. doi: 10.1111/j.1466-8238.2010.00540.x
Emmerling, C.. (2001). Response of earthworm communities to different types of soil tillage. Appl. Soil Ecol. 17, 91–96. doi: 10.1016/S0929-1393(00)00132-3
Erktan, A., Or, D., and Scheu, S. (2020). The physical structure of soil: determinant and consequence of trophic interactions. Soil Biol. Biochem. 148:107876. doi: 10.1016/j.soilbio.2020.107876
Evans, C. D., Freeman, C., Cork, L. G., Thomas, D. N., Reynolds, B., Billett, M. F., et al. (2007). Evidence against recent climate-induced destabilisation of soil carbon from 14C analysis of riverine dissolved organic matter. Geophys. Res. Lett. 34:L07407. doi: 10.1029/2007GL029431
Farrar, J., Hawes, M., Jones, D., and Lindow, S. (2003). How roots control the flux of carbon to the rhizosphere. Ecology 84, 827–837. doi: 10.1890/0012-9658(2003)084[0827:HRCTFO]2.0.CO;2
Ferreira, A. O., Amado, T. J. C., Rice, C. W., Diaz, D. A. R., Briedis, C., Inagaki, T. M., et al. (2018). Driving factors of soil carbon accumulation in Oxisols in long-term no-till systems of South Brazil. Sci. Total Environ. 622–623, 735–742. doi: 10.1016/j.scitotenv.2017.12.019
Filser, J., Faber, J. H., Tiunov, A. V., Brussaard, L., Frouz, J., De Deyn, G., et al. (2016). Soil fauna: key to new carbon models. Soil 2, 565–582. doi: 10.5194/soil-2-565-2016
Fontaine, S., Barot, S., Barré, P., Bdioui, N., Mary, B., and Rumpel, C. (2007). Stability of organic carbon in deep soil layers controlled by fresh carbon supply. Nature 450, 277–280. doi: 10.1038/nature06275
Fornara, D. A., and Tilman, D. (2008). Plant functional composition influences rates of soil carbon and nitrogen accumulation. J. Ecol. 96, 314–322. doi: 10.1111/j.1365-2745.2007.01345.x
Franzluebbers, A. J., Langdale, G. W., and Schomberg, H. H. (1999). Soil carbon, nitrogen, and aggregation in response to type and frequency of tillage. Soil Sci. Soc. Am. J. 63, 349–355. doi: 10.2136/sssaj1999.03615995006300020012x
Franzluebbers, A. J., and Stuedemann, J. A. (2010). Surface soil changes during twelve years of pasture management in the Southern Piedmont USA. Soil Sci. Soc. Am. J. 74, 2131–2141. doi: 10.2136/sssaj2010.0034
Franzluebbers, A. J., and Stuedemann, J. A. (2014). Crop and cattle production responses to tillage and cover crop management in an integrated crop–livestock system in the southeastern USA. Eur. J. Agron. 57, 62–70. doi: 10.1016/j.eja.2013.05.009
Freund, L., Mariotte, P., Santonja, M., Buttler, A., and Jeangros, B. (2021). Species identity, rather than species mixtures, drives cover crop effects on nutrient partitioning in unfertilized agricultural soil. Plant Soil 460, 149–162. doi: 10.1007/s11104-020-04782-z
Fronning, B. E., Thelen, K. D., and Min, D.-H. (2008). Use of manure, compost, and cover crops to supplant crop residue carbon in corn stover removed cropping systems. Agron. J. 100, 1703–1710. doi: 10.2134/agronj2008.0052
Frouz, J., PiŽl, V., Cienciala, E., and Kalčík, J. (2009). Carbon storage in post-mining forest soil, the role of tree biomass and soil bioturbation. Biogeochemistry 94, 111–121. doi: 10.1007/s10533-009-9313-0
Fultz, L. M., Moore-Kucera, J., Zobeck, T. M., Acosta-Martínez, V., Wester, D. B., and Allen, V. G. (2013). Organic carbon dynamics and soil stability in five semiarid agroecosystems. Agric. Ecosyst. Environ. 181, 231–240. doi: 10.1016/j.agee.2013.10.004
Fustec, J., Lesuffleur, F., Mahieu, S., and Cliquet, J.-B. (2010). Nitrogen rhizodeposition of legumes. A review. Agron. Sustainable Dev. 30, 57–66. doi: 10.1051/agro/2009003
García-Orenes, F., Guerrero, C., Roldán, A., Mataix-Solera, J., Cerdà, A., Campoy, M., et al. (2010). Soil microbial biomass and activity under different agricultural management systems in a semiarid Mediterranean agroecosystem. Soil Tillage Res. 109, 110–115. doi: 10.1016/j.still.2010.05.005
Gleixner, G., Poirier, N., Bol, R., and Balesdent, J. (2002). Molecular dynamics of organic matter in a cultivated soil. Org. Geochem. 33, 357–366. doi: 10.1016/S0146-6380(01)00166-8
Gómez, J. A.. (2017). Sustainability using cover crops in Mediterranean tree crops, olives and vines – challenges and current knowledge. Hung. Geogr. Bull. 66, 13–28. doi: 10.15201/hungeobull.66.1.2
Gorka, S., Dietrich, M., Mayerhofer, W., Gabriel, R., Wiesenbauer, J., Martin, V., et al. (2019). Rapid transfer of plant photosynthates to soil bacteria via ectomycorrhizal hyphae and its interaction with nitrogen availability. Front. Microbiol. 10:168. doi: 10.3389/fmicb.2019.00168
Gristina, L., Scalenghe, R., García-Díaz, A., Matranga, M. G., Ferraro, V., Guaitoli, F., et al. (2020). Soil organic carbon stocks under recommended management practices in different soils of semiarid vineyards. Land Degrad. Dev. 31, 1906–1914. doi: 10.1002/ldr.3339
Gross, C. D., and Harrison, R. B. (2019). The case for digging deeper: soil organic carbon storage, dynamics, and controls in our changing world. Soil Syst. 3:28. doi: 10.3390/soilsystems3020028
Guan, X., Huang, J., Guo, N., Bi, J., and Wang, G. (2009). Variability of soil moisture and its relationship with surface albedo and soil thermal parameters over the Loess Plateau. Adv. Atmos. Sci. 26, 692–700. doi: 10.1007/s00376-009-8198-0
Guggenberger, G., and Kaiser, K. (2003). Dissolved organic matter in soil: challenging the paradigm of sorptive preservation. Geoderma 113, 293–310. doi: 10.1016/S0016-7061(02)00366-X
Guyonnet, J. P., Guillemet, M., Dubost, A., Simon, L., Ortet, P., Barakat, M., et al. (2018). Plant nutrient resource use strategies shape active rhizosphere microbiota through root exudation. Front. Plant Sci. 9:1662. doi: 10.3389/fpls.2018.01662
Gyssels, G., Poesen, J., Bochet, E., and Li, Y. (2005). Impact of plant roots on the resistance of soils to erosion by water: a review. Prog. Phys. Geog. Earth Environ. 29, 189–217. doi: 10.1191/0309133305pp443ra
Hamilton, E. W., Frank, D. A., Hinchey, P. M., and Murray, T. R. (2008). Defoliation induces root exudation and triggers positive rhizospheric feedbacks in a temperate grassland. Soil Biol. Biochem. 40, 2865–2873. doi: 10.1016/j.soilbio.2008.08.007
Harden, J. W., Hugelius, G., Ahlström, A., Blankinship, J. C., Bond-Lamberty, B., Lawrence, C. R., et al. (2018). Networking our science to characterize the state, vulnerabilities, and management opportunities of soil organic matter. Glob. Chang. Biol. 24, e705–e718. doi: 10.1111/gcb.13896
Harper, R. J., and Tibbett, M. (2013). The hidden organic carbon in deep mineral soils. Plant Soil 368, 641–648. doi: 10.1007/s11104-013-1600-9
Harrison, R. B., Footen, P. W., and Strahm, B. D. (2011). Deep soil horizons: contribution and importance to soil carbon pools and in assessing whole-ecosystem response to management and global change. For. Sci. 57, 67–76. doi: 10.1093/forestscience/57.1.67
Hättenschwiler, S., and Vitousek, P. M. (2000). The role of polyphenols in terrestrial ecosystem nutrient cycling. Trends Ecol. Evol. 15, 238–243. doi: 10.1016/S0169-5347(00)01861-9
Haynes, R. J., and Beare, M. H. (1997). Influence of six crop species on aggregate stability and some labile organic matter fractions. Soil Biol. Biochem. 29, 1647–1653. doi: 10.1016/S0038-0717(97)00078-3
Hefner, M., Canali, S., Willekens, K., Lootens, P., Deltour, P., Beeckman, A., et al. (2020). Termination method and time of agro-ecological service crops influence soil mineral nitrogen, cabbage yield and root growth across five locations in Northern and Western Europe. Eur. J. Agron. 120:126144. doi: 10.1016/j.eja.2020.126144
Henneron, L., Kardol, P., Wardle, D. A., Cros, C., and Fontaine, S. (2020). Rhizosphere control of soil nitrogen cycling: a key component of plant economic strategies. New Phytol. 228, 1269–1282. doi: 10.1111/nph.16760
Herz, K., Dietz, S., Gorzolka, K., Haider, S., Jandt, U., Scheel, D., et al. (2018). Linking root exudates to functional plant traits. PLoS ONE 13:e0204128. doi: 10.1371/journal.pone.0204128
Hessen, D. O., Ågren, G. I., Anderson, T. R., Elser, J. J., and de Ruiter, P. C. (2004). Carbon sequestration in ecosystems: the role of stoichiometry. Ecology 85, 1179–1192. doi: 10.1890/02-0251
Hicks Pries, C. E., Sulman, B. N., West, C., O'Neill, C., Poppleton, E., Porras, R. C., et al. (2018). Root litter decomposition slows with soil depth. Soil Biol. Biochem. 125, 103–114. doi: 10.1016/j.soilbio.2018.07.002
Higashi, T., Yunghui, M., Komatsuzaki, M., Miura, S., Hirata, T., Araki, H., et al. (2014). Tillage and cover crop species affect soil organic carbon in Andosol, Kanto, Japan. Soil Tillage Res. 138, 64–72. doi: 10.1016/j.still.2013.12.010
Hobbs, P. R., Sayre, K., and Gupta, R. (2008). The role of conservation agriculture in sustainable agriculture. Phil. Trans. R. Soc. B. 363, 543–555. doi: 10.1098/rstb.2007.2169
Holford, I. C. R.. (1980). Effects of duration of grazed lucerne on long-term yields and nitrogen uptake of subsequent wheat. Aust. J. Agric. Res. 31, 239–250. doi: 10.1071/AR9800239
House, G. J., and Parmelee, R. W. (1985). Comparison of soil arthropods and earthworms from conventional and no-tillage agroecosystems. Soil Tillage Res. 5, 351–360. doi: 10.1016/S0167-1987(85)80003-9
Hu, F., Xu, C., Li, H., Li, S., Yu, Z., Li, Y., et al. (2015). Particles interaction forces and their effects on soil aggregates breakdown. Soil Tillage Res. 147, 1–9. doi: 10.1016/j.still.2014.11.006
Huang, X., Jiang, H., Li, Y., Ma, Y., Tang, H., Ran, W., et al. (2016). The role of poorly crystalline iron oxides in the stability of soil aggregate-associated organic carbon in a rice–wheat cropping system. Geoderma 279, 1–10. doi: 10.1016/j.geoderma.2016.05.011
Jackson, R. B., Lajtha, K., Crow, S. E., Hugelius, G., Kramer, M. G., and Piñeiro, G. (2017). The ecology of soil carbon: pools, vulnerabilities, and biotic and abiotic controls. Annu. Rev. Ecol. Evol. Syst. 48, 419–445. doi: 10.1146/annurev-ecolsys-112414-054234
Jani, A. D., Mulvaney, M. J., Enloe, H. A., Erickson, J. E., Leon, R. G., Rowland, D. L., et al. (2019). Peanut residue distribution gradients and tillage practices determine patterns of nitrogen mineralization. Nutri. Cycl. Agroecosyst. 113, 63–76. doi: 10.1007/s10705-018-9962-2
Janzen, H. H.. (2006). The soil carbon dilemma: shall we hoard it or use it? Soil Biol. Biochem. 38, 419–424. doi: 10.1016/j.soilbio.2005.10.008
Jardine, P. M., McCarthy, J. F., and Weber, N. L. (1989). Mechanisms of dissolved organic carbon adsorption on soil. Soil Sci. Soc. Am. J. 53, 1378–1385. doi: 10.2136/sssaj1989.03615995005300050013x
Jastrow, J. D., Amonette, J. E., and Bailey, V. L. (2007). Mechanisms controlling soil carbon turnover and their potential application for enhancing carbon sequestration. Clim. Chang. 80, 5–23. doi: 10.1007/s10584-006-9178-3
Jastrow, J. D., and Miller, R. M. (1996). Soil Aggregate Stabilization and Carbon Sequestration: Feedbacks Through Organomineral Associations. United States. Available online at: https://www.osti.gov/biblio/464188 (accessed February 8, 2022)
Jenkinson, D. S.. (1990). The turnover of organic carbon and nitrogen in soil. Phil. Trans. R. Soc. Lond. B 329, 361–368. doi: 10.1098/rstb.1990.0177
Jilling, A., Kane, D., Williams, A., Yannarell, A. C., Davis, A., Jordan, N. R., et al. (2020). Rapid and distinct responses of particulate and mineral-associated organic nitrogen to conservation tillage and cover crops. Geoderma 359:114001. doi: 10.1016/j.geoderma.2019.114001
Jobbágy, E. G., and Jackson, R. B. (2000). The vertical distribution of soil organic carbon and Its relation to climate and vegetation. Ecol. Appl. 10, 423–436. doi: 10.1890/1051-0761(2000)010[0423:TVDOSO]2.0.CO;2
Johnston, W., and Carter, H. (2000). Structural adjustment, resources, global economy to challenge California agriculture. Calif. Agric. 54, 16–22. doi: 10.3733/ca.v054n04p16
Kaiser, K., and Kalbitz, K. (2012). Cycling downwards – dissolved organic matter in soils. Soil Biol. Biochem. 52, 29–32. doi: 10.1016/j.soilbio.2012.04.002
Kaiser, K., and Zech, W. (1997). Competitive sorption of dissolved organic matter fractions to soils and related mineral phases. Soil Sci. Soc. Am. J. 61, 64–69. doi: 10.2136/sssaj1997.03615995006100010011x
Kaiser, K., and Zech, W. (2000). Dissolved organic matter sorption by mineral constituents of subsoil clay fractions. J. Plant Nutr. Soil Sci. 163, 531–535. doi: 10.1002/1522-2624(200010)163:5<531::AID-JPLN531>3.0.CO;2-N
Kalbitz, K., and Kaiser, K. (2008). Contribution of dissolved organic matter to carbon storage in forest mineral soils. J. Plant Nutr. Soil Sci. 171, 52–60. doi: 10.1002/jpln.200700043
Kallenbach, C. M., Frey, S. D., and Grandy, A. S. (2016). Direct evidence for microbial-derived soil organic matter formation and its ecophysiological controls. Nat. Commun. 7:13630. doi: 10.1038/ncomms13630
Kallenbach, C. M., Grandy, A. S., Frey, S. D., and Diefendorf, A. F. (2015). Microbial physiology and necromass regulate agricultural soil carbon accumulation. Soil Biol. Biochem. 91, 279–290. doi: 10.1016/j.soilbio.2015.09.005
Kallenbach, C. M., Wallenstein, M. D., Schipanksi, M. E., and Grandy, A. S. (2019). Managing agroecosystems for soil microbial carbon use efficiency: Ecological unknowns, potential outcomes, and a path forward. Front. Microbiol. 10, 1146. doi: 10.3389/fmicb.2019.01146
Kan, Z.-R., Ma, S.-T., Liu, Q.-Y., Liu, B.-Y., Virk, A. L., Qi, J.-Y., et al. (2020). Carbon sequestration and mineralization in soil aggregates under long-term conservation tillage in the North China Plain. Catena 188:104428. doi: 10.1016/j.catena.2019.104428
Karlowsky, S., Augusti, A., Ingrisch, J., Akanda, M. K. U., Bahn, M., and Gleixner, G. (2018). Drought-induced accumulation of root exudates supports post-drought recovery of microbes in mountain grassland. Front. Plant Sci. 9:1593. doi: 10.3389/fpls.2018.01593
Kaspar, T. C., Parkin, T. B., Jaynes, D. B., Cambardella, C. A., Meek, D. W., and Jung, Y. S. (2006). Examining changes in soil organic carbon with oat and rye cover crops using terrain covariates. Soil Sci. Soc. Am. J. 70, 1168–1177. doi: 10.2136/sssaj2005.0095
Kaštovská, E., Edwards, K., and Šantručková, H. (2017). Rhizodeposition flux of competitive versus conservative graminoid: contribution of exudates and root lysates as affected by N loading. Plant Soil 412, 331–344. doi: 10.1007/s11104-016-3066-z
Keiluweit, M., Bougoure, J. J., Nico, P. S., Pett-Ridge, J., Weber, P. K., and Kleber, M. (2015). Mineral protection of soil carbon counteracted by root exudates. Nat. Clim. Chang. 5, 588–595. doi: 10.1038/nclimate2580
Kell, D. B.. (2011). Breeding crop plants with deep roots: their role in sustainable carbon, nutrient and water sequestration. Ann. Bot. 108, 407–418. doi: 10.1093/aob/mcr175
Kiem, R., and Kögel-Knabner, I. (2003). Contribution of lignin and polysaccharides to the refractory carbon pool in C-depleted arable soils. Soil Biol. Biochem. 35, 101–118. doi: 10.1016/S0038-0717(02)00242-0
Kirchmann, H., Haberhauer, G., Kandeler, E., Sessitsch, A., and Gerzabek, M. H. (2004). Effects of level and quality of organic matter input on carbon storage and biological activity in soil: synthesis of a long-term experiment. Glob. Biogeochem. Cycles 18:GB4011. doi: 10.1029/2003GB002204
Kirkby, C. A., Richardson, A. E., Wade, L. J., Batten, G. D., Blanchard, C., and Kirkegaard, J. A. (2013). Carbon-nutrient stoichiometry to increase soil carbon sequestration. Soil Biol. Biochem. 60, 77–86. doi: 10.1016/j.soilbio.2013.01.011
Kleber, M., Eusterhues, K., Keiluweit, M., Mikutta, C., Mikutta, R., and Nico, P. S. (2015). “Chapter one - Mineral–organic associations: formation, properties, and relevance in soil environments”, in Advances in Agronomy, ed D. L. Sparks (Cambridge, MA: Academic Press), 1–140. doi: 10.1016/bs.agron.2014.10.005
Kleber, M., Sollins, P., and Sutton, R. (2007). A conceptual model of organo-mineral interactions in soils: self-assembly of organic molecular fragments into zonal structures on mineral surfaces. Biogeochemistry 85, 9–24. doi: 10.1007/s10533-007-9103-5
Knicker, H.. (2004). Stabilization of N-compounds in soil and organic-matter-rich sediments—what is the difference? Mar. Chem. 92, 167–195. doi: 10.1016/j.marchem.2004.06.025
Kögel-Knabner, I., Guggenberger, G., Kleber, M., Kandeler, E., Kalbitz, K., Scheu, S., et al. (2008). Organo-mineral associations in temperate soils: integrating biology, mineralogy, and organic matter chemistry. J. Plant Nutr. Soil Sci. 171, 61–82. doi: 10.1002/jpln.200700048
Kopittke, P. M., Dalal, R. C., Hoeschen, C., Li, C., Menzies, N. W., and Mueller, C. W. (2020). Soil organic matter is stabilized by organo-mineral associations through two key processes: the role of the carbon to nitrogen ratio. Geoderma 357:113974. doi: 10.1016/j.geoderma.2019.113974
Kraffczyk, I., Trolldenier, G., and Beringer, H. (1984). Soluble root exudates of maize: influence of potassium supply and rhizosphere microorganisms. Soil Biol. Biochem. 16, 315–322. doi: 10.1016/0038-0717(84)90025-7
Kramer, M. G., Lajtha, K., and Aufdenkampe, A. K. (2017). Depth trends of soil organic matter C:N and 15N natural abundance controlled by association with minerals. Biogeochemistry 136, 237–248. doi: 10.1007/s10533-017-0378-x
Kravchenko, A. N., Guber, A. K., Razavi, B. S., Koestel, J., Quigley, M. Y., Robertson, G. P., et al. (2019). Microbial spatial footprint as a driver of soil carbon stabilization. Nat. Commun. 10:3121. doi: 10.1038/s41467-019-11057-4
Lajtha, K., Townsend, K. L., Kramer, M. G., Swanston, C., Bowden, R. D., and Nadelhoffer, K. (2014). Changes to particulate versus mineral-associated soil carbon after 50 years of litter manipulation in forest and prairie experimental ecosystems. Biogeochemistry 119, 341–360. doi: 10.1007/s10533-014-9970-5
Lal, R.. (2016). Soil health and carbon management. Food Energy Secur. 5, 212–222. doi: 10.1002/fes3.96
Lal, R.. (2020). Regenerative agriculture for food and climate. J. Soil Water Conserv. 75, 123A−124A. doi: 10.2489/jswc.2020.0620A
Lange, M., Eisenhauer, N., Sierra, C. A., Bessler, H., Engels, C., Griffiths, R. I., et al. (2015). Plant diversity increases soil microbial activity and soil carbon storage. Nat. Commun. 6:6707. doi: 10.1038/ncomms7707
Langley, J. A., Chapman, S. K., and Hungate, B. A. (2006). Ectomycorrhizal colonization slows root decomposition: the post-mortem fungal legacy. Ecol. Lett. 9, 955–959. doi: 10.1111/j.1461-0248.2006.00948.x
Larson, W. E., Clapp, C. E., Pierre, W. H., and Morachan, Y. B. (1972). Effects of increasing amounts of organic residues on continuous corn: II. Organic carbon, nitrogen, phosphorus, and sulfur. Agron. J. 64, 204–209. doi: 10.2134/agronj1972.00021962006400020023x
Lavallee, J. M., Soong, J. L., and Cotrufo, M. F. (2020). Conceptualizing soil organic matter into particulate and mineral-associated forms to address global change in the 21st century. Glob. Chang. Biol. 26, 261–273. doi: 10.1111/gcb.14859
Lavelle, P., Decaëns, T., Aubert, M., Barot, S., Blouin, M., Bureau, F., et al. (2006). Soil invertebrates and ecosystem services. Europ. J. Soil Biol. 42, S3–S15. doi: 10.1016/j.ejsobi.2006.10.002
Lavelle, P., Spain, A., Blouin, M., Brown, G., Decaëns, T., Grimaldi, M., et al. (2016). Ecosystem engineers in a self-organized soil: a review of concepts and future research questions. Soil Sci. 181, 91–109. doi: 10.1097/SS.0000000000000155
Lazicki, P. A., Liebman, M., and Wander, M. M. (2016). Root parameters show how management alters resource distribution and soil quality in conventional and low-input cropping systems in central Iowa. PLoS ONE 11:e0164209. doi: 10.1371/journal.pone.0164209
Lehmann, J., and Kleber, M. (2015). The contentious nature of soil organic matter. Nature 528, 60–68. doi: 10.1038/nature16069
Lekkerkerk, L., Lundkvist, H., Ågren, G. I., Ekbohm, G., and Bosatta, E. (1990). Decomposition of heterogeneous substrates; an experimental investigation of a hypothesis on substrate and microbial properties. Soil Biol. Biochem. 22, 161–167. doi: 10.1016/0038-0717(90)90081-A
Lemaire, G., Franzluebbers, A., Carvalho, P. C. F., and Dedieu, B. (2014). Integrated crop–livestock systems: strategies to achieve synergy between agricultural production and environmental quality. Agric. Ecosyst. Environ. 190, 4–8. doi: 10.1016/j.agee.2013.08.009
Li, X. G., Jia, B., Lv, J., Ma, Q., Kuzyakov, Y., and Li, F. (2017). Nitrogen fertilization decreases the decomposition of soil organic matter and plant residues in planted soils. Soil Biol. Biochem. 112, 47–55. doi: 10.1016/j.soilbio.2017.04.018
Li, Y., Li, Z., Cui, S., Jagadamma, S., and Zhang, Q. (2019). Residue retention and minimum tillage improve physical environment of the soil in croplands: a global meta-analysis. Soil Tillage Res.194:104292. doi: 10.1016/j.still.2019.06.009
Liang, A.-Z., Zhang, X.-P., Fang, H.-J., Yang, X.-M., and Drury, C. F. (2007). Short-term effects of tillage practices on organic carbon in clay loam soil of Northeast China. Pedosphere 17, 619–623. doi: 10.1016/S1002-0160(07)60073-3
Liang, C.. (2020). Soil microbial carbon pump: mechanism and appraisal. Soil Ecol. Lett. 2, 241–254. doi: 10.1007/s42832-020-0052-4
Liang, C., Amelung, W., Lehmann, J., and Kästner, M. (2019). Quantitative assessment of microbial necromass contribution to soil organic matter. Glob. Chang. Biol. 25, 3578–3590. doi: 10.1111/gcb.14781
Liang, C., Schimel, J. P., and Jastrow, J. D. (2017). The importance of anabolism in microbial control over soil carbon storage. Nat. Microbiol. 2:17105. doi: 10.1038/nmicrobiol.2017.105
Liang, J., Zhou, Z., Huo, C., Shi, Z., Cole, J. R., Huang, L., et al. (2018). More replenishment than priming loss of soil organic carbon with additional carbon input. Nat. Commun. 9:3175. doi: 10.1038/s41467-018-05667-7
Liebig, M. A., Faust, D. R., Archer, D. W., Kronberg, S. L., Hendrickson, J. R., and Aukema, K. D. (2020). Grazing effects on nitrous oxide flux in an integrated crop-livestock system. Agric. Ecosyst. Environ. 304:107146. doi: 10.1016/j.agee.2020.107146
Liebmann, P., Wordell-Dietrich, P., Kalbitz, K., Mikutta, R., Kalks, F., Don, A., et al. (2020). Relevance of aboveground litter for soil organic matter formation – a soil profile perspective. Biogeosciences 17, 3099–3113. doi: 10.5194/bg-17-3099-2020
Loades, K. W., Bengough, A. G., Bransby, M. F., and Hallett, P. D. (2010). Planting density influence on fibrous root reinforcement of soils. Ecol. Eng. 36, 276–284. doi: 10.1016/j.ecoleng.2009.02.005
Mahal, N. K., Osterholz, W. R., Miguez, F. E., Poffenbarger, H. J., Sawyer, J. E., Olk, D. C., et al. (2019). Nitrogen fertilizer suppresses mineralization of soil organic matter in maize agroecosystems. Front. Ecol. Evol. 7:59. doi: 10.3389/fevo.2019.00059
Malik, A. A., Martiny, J. B. H., Brodie, E. L., Martiny, A. C., Treseder, K. K., and Allison, S. D. (2020). Defining trait-based microbial strategies with consequences for soil carbon cycling under climate change. ISME J. 14, 1–9. doi: 10.1038/s41396-019-0510-0
Malik, A. A., Roth, V.-N., Hébert, M., Tremblay, L., Dittmar, T., and Gleixner, G. (2016). Linking molecular size, composition and carbon turnover of extractable soil microbial compounds. Soil Biol. Biochem. 100, 66–73. doi: 10.1016/j.soilbio.2016.05.019
Manzoni, S., Taylor, P., Richter, A., Porporato, A., and Ågren, G. I. (2012). Environmental and stoichiometric controls on microbial carbon-use efficiency in soils. New Phytol. 196, 79–91. doi: 10.1111/j.1469-8137.2012.04225.x
Martens, D. A.. (2002). Relationship between plant phenolic acids released during soil mineralization and aggregate stabilization. Soil Sci. Soc. Am. J. 66, 1857–1867. doi: 10.2136/sssaj2002.1857
McClelland, S. C., Paustian, K., and Schipanski, M. E. (2021). Management of cover crops in temperate climates influences soil organic carbon stocks: a meta-analysis. Ecol. Appl. 31:e02278. doi: 10.1002/eap.2278
McSherry, M. E., and Ritchie, M. E. (2013). Effects of grazing on grassland soil carbon: a global review. Glob. Chang. Biol. 19, 1347–1357. doi: 10.1111/gcb.12144
Mikutta, R., Turner, S., Schippers, A., Gentsch, N., Meyer-Stüve, S., Condron, L. M., et al. (2019). Microbial and abiotic controls on mineral-associated organic matter in soil profiles along an ecosystem gradient. Sci. Rep. 9:10294. doi: 10.1038/s41598-019-46501-4
Miltner, A., Bombach, P., Schmidt-Brücken, B., and Kästner, M. (2012). SOM genesis: microbial biomass as a significant source. Biogeochemistry 111, 41–55. doi: 10.1007/s10533-011-9658-z
Mo, F., Zhang, Y.-Y., Liu, Y., and Liao, Y.-C. (2021). Microbial carbon-use efficiency and straw-induced priming effect within soil aggregates are regulated by tillage history and balanced nutrient supply. Biol. Fertil. Soils 57, 409–420. doi: 10.1007/s00374-021-01540-w
Moreland, K., Tian, Z., Berhe, A. A., Mcfarlane, K. J., Hartsough, P., Hart, S. C., et al. (2021). Deep in the Sierra Nevada critical zone: saprock represents a large terrestrial organic carbon stock. Environ. Res. Lett. 16:124059. doi: 10.1088/1748-9326/ac3bfe
Mortensen, E. Ø., De Notaris, C., Peixoto, L., Olesen, J. E., and Rasmussen, J. (2021). Short-term cover crop carbon inputs to soil as affected by long-term cropping system management and soil fertility. Agric. Ecosyst. Environ. 311:107339. doi: 10.1016/j.agee.2021.107339
Mosier, S., Apfelbaum, S., Byck, P., Calderon, F., Teague, R., Thompson, R., et al. (2021). Adaptive multi-paddock grazing enhances soil carbon and nitrogen stocks and stabilization through mineral association in southeastern U.S. grazing lands. J. Environ. Manage. 288:112409. doi: 10.1016/j.jenvman.2021.112409
Motta, A. C. V., Reeves, D. W., Burmester, C., and Feng, Y. (2007). Conservation tillage, rotations, and cover crop affecting soil quality in the tennessee valley: particulate organic matter, organic matter, and microbial biomass. Commun. Soil Sci. Plant Anal. 38, 2831–2847. doi: 10.1080/00103620701663065
Nieminen, M.. (2004). Export of dissolved organic carbon, nitrogen and phosphorus following clear-cutting of three Norway spruce forests growing on drained peatlands in southern Finland. Silva Fenn. 38, 123–132. doi: 10.14214/sf.422
Novara, A., Cerda, A., Barone, E., and Gristina, L. (2021). Cover crop management and water conservation in vineyard and olive orchards. Soil Tillage Res. 208:104896. doi: 10.1016/j.still.2020.104896
Novara, A., Poma, I., Sarno, M., Venezia, G., and Gristina, L. (2016). Long-term durum wheat-based cropping systems result in the rapid saturation of soil carbon in the mediterranean semi-arid environment. Land Degrad Dev. 27, 612–619. doi: 10.1002/ldr.2468
Nunes, M. R., Karlen, D. L., Veum, K. S., Moorman, T. B., and Cambardella, C. A. (2020). Biological soil health indicators respond to tillage intensity: a US meta-analysis. Geoderma 369:114335. doi: 10.1016/j.geoderma.2020.114335
Oades, J. M.. (1984). Soil organic matter and structural stability: mechanisms and implications for management. Plant Soil 76, 319–337. doi: 10.1007/BF02205590
Oburger, E., and Jones, D. L. (2018). Sampling root exudates – mission impossible? Rhizosphere 6, 116–133. doi: 10.1016/j.rhisph.2018.06.004
Olchin, G. P., Ogle, S., Frey, S. D., Filley, T. R., Paustian, K., and Six, J. (2008). Residue carbon stabilization in soil aggregates of no-till and tillage management of dryland cropping systems. Soil Sci. Soc. Am. J. 72, 507–513. doi: 10.2136/sssaj2006.0417
Oliveira, F. É. R., Oliveira, J. M., and Xavier, F. A. S. (2016). Changes in soil organic carbon fractions in response to cover crops in an orange orchard. Rev. Bras. Cienc. Solo 40:e0150105. doi: 10.1590/18069657rbcs20150105
Ordóñez, R. A., Castellano, M. J., Danalatos, G. N., Wright, E. E., Hatfield, J. L., Burras, L., et al. (2021). Insufficient and excessive N fertilizer input reduces maize root mass across soil types. Field Crops Res. 267:108142. doi: 10.1016/j.fcr.2021.108142
Ordóñez-Fernández, R., Torres, M. A. R.-R., Márquez-García, J., Moreno-García, M., and Carbonell-Bojollo, R. M. (2018). Legumes used as cover crops to reduce fertilisation problems improving soil nitrate in an organic orchard. Eur. J. Agron. 95, 1–13. doi: 10.1016/j.eja.2018.02.001
Parton, W. J., Stewart, J. W. B., and Cole, C. V. (1988). Dynamics of C, N, P and S in grassland soils: a model. Biogeochemistry 5, 109–131. doi: 10.1007/BF02180320
Pausch, J., and Kuzyakov, Y. (2018). Carbon input by roots into the soil: quantification of rhizodeposition from root to ecosystem scale. Glob. Chang. Biol. 24, 1–12. doi: 10.1111/gcb.13850
Peregrina, F., Larrieta, C., Ibáñez, S., and García-Escudero, E. (2010). Labile organic matter, aggregates, and stratification ratios in a semiarid vineyard with cover crops. Soil Sci. Soc. Am. J. 74, 2120–2130. doi: 10.2136/sssaj2010.0081
Piñeiro, G., Paruelo, J. M., Oesterheld, M., and Jobbágy, E. G. (2010). Pathways of grazing effects on soil organic carbon and nitrogen. Rangeland Ecol. Manage. 63, 109–119. doi: 10.2111/08-255.1
Plaza, C., Courtier-Murias, D., Fernández, J. M., Polo, A., and Simpson, A. J. (2013). Physical, chemical, and biochemical mechanisms of soil organic matter stabilization under conservation tillage systems: a central role for microbes and microbial by-products in C sequestration. Soil Biol. Biochem. 57, 124–134. doi: 10.1016/j.soilbio.2012.07.026
Plaza-Bonilla, D., Arrúe, J. L., Cantero-Martínez, C., Fanlo, R., Iglesias, A., and Álvaro-Fuentes, J. (2015). Carbon management in dryland agricultural systems. A review. Agron. Sustainable Dev. 35, 1319–1334. doi: 10.1007/s13593-015-0326-x
Poeplau, C., and Don, A. (2015). Carbon sequestration in agricultural soils via cultivation of cover crops—a meta-analysis. Agric. Ecosyst. Environ. 200, 33–41. doi: 10.1016/j.agee.2014.10.024
Poirier, V., Roumet, C., and Munson, A. D. (2018). The root of the matter: linking root traits and soil organic matter stabilization processes. Soil Biol. Biochem. 120, 246–259. doi: 10.1016/j.soilbio.2018.02.016
Prescott, C. E., Grayston, S. J., Helmisaari, H.-S., Kaštovská, E., Körner, C., Lambers, H., et al. (2020). Surplus carbon drives allocation and plant–soil interactions. Trends Ecol. Evol. 35, 1110–1118. doi: 10.1016/j.tree.2020.08.007
Rabbi, S. M. F., Wilson, B. R., Lockwood, P. V., Daniel, H., and Young, I. M. (2014). Soil organic carbon mineralization rates in aggregates under contrasting land uses. Geoderma 216, 10–18. doi: 10.1016/j.geoderma.2013.10.023
Rahman, M. T., Guo, Z. C., Zhang, Z. B., Zhou, H., and Peng, X. H. (2018). Wetting and drying cycles improving aggregation and associated C stabilization differently after straw or biochar incorporated into a Vertisol. Soil Tillage Res. 175, 28–36. doi: 10.1016/j.still.2017.08.007
Ramos, M. E., Benítez, E., García, P. A., and Robles, A. B. (2010). Cover crops under different managements vs. frequent tillage in almond orchards in semiarid conditions: effects on soil quality. Appl. Soil Ecol. 44, 6–14. doi: 10.1016/j.apsoil.2009.08.005
Rasmussen, C., Heckman, K., Wieder, W. R., Keiluweit, M., Lawrence, C. R., Berhe, A. A., et al. (2018). Beyond clay: towards an improved set of variables for predicting soil organic matter content. Biogeochemistry 137, 297–306. doi: 10.1007/s10533-018-0424-3
Rasmussen, C., Southard, R. J., and Horwath, W. R. (2006). Mineral control of organic carbon mineralization in a range of temperate conifer forest soils. Glob. Change Biol. 12, 834–847. doi: 10.1111/j.1365-2486.2006.01132.x
Rasmussen, P. E., Allmaras, R. R., Rohde, C. R., and Roager, N. C. (1980). Crop residue influences on soil carbon and nitrogen in a wheat-fallow system. Soil Sci. Soc. Am. J. 44, 596–600. doi: 10.2136/sssaj1980.03615995004400030033x
Rasse, D. P., Rumpel, C., and Dignac, M.-F. (2005). Is soil carbon mostly root carbon? Mechanisms for a specific stabilisation. Plant Soil 269, 341–356. doi: 10.1007/s11104-004-0907-y
Rath, D., Bogie, N., Deiss, L., Parikh, S., Wang, D., Ying, S., et al. (2021). Synergy between compost and cover crops leads to increased subsurface soil carbon storage. Soil Discuss. 8, 59–83. doi: 10.5194/soil-2021-19
Richter, D. D., and Markewitz, D. (1995). How deep is soil? BioScience 45, 600–609. doi: 10.2307/1312764
Robertson, A. D., Paustian, K., Ogle, S., Wallenstein, M. D., Lugato, E., and Cotrufo, M. F. (2019). Unifying soil organic matter formation and persistence frameworks: the MEMS model. Biogeosciences 16, 1225–1248. doi: 10.5194/bg-16-1225-2019
Rocci, K. S., Lavallee, J. M., Stewart, C. E., and Cotrufo, M. F. (2021). Soil organic carbon response to global environmental change depends on its distribution between mineral-associated and particulate organic matter: a meta-analysis. Sci. Total Environ. 793:148569. doi: 10.1016/j.scitotenv.2021.148569
Romdhane, S., Spor, A., Busset, H., Falchetto, L., Martin, J., Bizouard, F., et al. (2019). Cover crop management practices rather than composition of cover crop mixtures affect bacterial communities in no-till agroecosystems. Front. Microbiol. 10:1618. doi: 10.3389/fmicb.2019.01618
Rumpel, C., Crème, A., Ngo, P. T., Velásquez, G., Mora, M. L., and Chabbi, A. (2015). The impact of grassland management on biogeochemical cycles involving carbon, nitrogen and phosphorus. J. Soil Sci. Plant Nutr. 15, 353–371. doi: 10.4067/S0718-95162015005000034
Rumpel, C., and Kögel-Knabner, I. (2011). Deep soil organic matter—a key but poorly understood component of terrestrial C cycle. Plant Soil 338, 143–158. doi: 10.1007/s11104-010-0391-5
Safriel, U., Adeel, Z., Niemeijer, D., Puigdefabregas, J., White, R., Lal, R., et al. (2005). “Chapter 22: Drylands systems”, in Ecosystems and Human Well-Being: Current State and Trends (Washington, DC: Island Press), 625–664. Available online at: https://www.alnap.org/help-library/ecosystems-and-human-well-being-current-state-and-trends (accessed February 8, 2022)
Salvo, L., Hernández, J., and Ernst, O. (2010). Distribution of soil organic carbon in different size fractions, under pasture and crop rotations with conventional tillage and no-till systems. Soil Tillage Res. 109, 116–122. doi: 10.1016/j.still.2010.05.008
Sanderman, J., Maddern, T., and Baldock, J. (2014). Similar composition but differential stability of mineral retained organic matter across four classes of clay minerals. Biogeochemistry 121, 409–424. doi: 10.1007/s10533-014-0009-8
Sarkar, S., and Singh, S. R. (2007). Interactive effect of tillage depth and mulch on soil temperature, productivity and water use pattern of rainfed barley (Hordium vulgare L.). Soil Tillage Res. 92, 79–86. doi: 10.1016/j.still.2006.01.014
Schaefer, M. V., Bogie, N. A., Rath, D., Marklein, A. R., Garniwan, A., Haensel, T., et al. (2020). Effect of cover crop on carbon distribution in size and density separated soil aggregates. Soil Syst. 4:6. doi: 10.3390/soilsystems4010006
Scharlemann, J. P. W., Tanner, E. V. J., Hiederer, R., and Kapos, V. (2014). Global soil carbon: understanding and managing the largest terrestrial carbon pool. Carbon Manag. 5, 81–91. doi: 10.4155/cmt.13.77
Schimel, J., Balser, T. C., and Wallenstein, M. (2007). Microbial stress-response physiology and its implications for ecosystem function. Ecology 88, 1386–1394. doi: 10.1890/06-0219
Schmidt, M. W. I., Torn, M. S., Abiven, S., Dittmar, T., Guggenberger, G., Janssens, I. A., et al. (2011). Persistence of soil organic matter as an ecosystem property. Nature 478, 49–56. doi: 10.1038/nature10386
Schonbeck, M. W., and Evanylo, G. K. (1998). Effects of mulches on soil properties and tomato production II. Plant-available nitrogen, organic matter input, and tilth-related properties. J. Sustainable Agric. 13, 83–100. doi: 10.1300/J064v13n01_07
Sekaran, U., Sagar, K. L., and Kumar, S. (2021). Soil aggregates, aggregate-associated carbon and nitrogen, and water retention as influenced by short and long-term no-till systems. Soil Tillage Res. 208:104885. doi: 10.1016/j.still.2020.104885
Shackelford, G. E., Kelsey, R., and Dicks, L. V. (2019). Effects of cover crops on multiple ecosystem services: ten meta-analyses of data from arable farmland in California and the Mediterranean. Land Use Policy 88:104204. doi: 10.1016/j.landusepol.2019.104204
Shahbaz, M., Kuzyakov, Y., and Heitkamp, F. (2017a). Decrease of soil organic matter stabilization with increasing inputs: mechanisms and controls. Geoderma 304, 76–82. doi: 10.1016/j.geoderma.2016.05.019
Shahbaz, M., Kuzyakov, Y., Sanaullah, M., Heitkamp, F., Zelenev, V., Kumar, A., et al. (2017b). Microbial decomposition of soil organic matter is mediated by quality and quantity of crop residues: mechanisms and thresholds. Biol. Fertil. Soils 53, 287–301. doi: 10.1007/s00374-016-1174-9
Shahzad, T., Anwar, F., Hussain, S., Mahmood, F., Arif, M. S., Sahar, A., et al. (2019). Carbon dynamics in surface and deep soil in response to increasing litter addition rates in an agro-ecosystem. Geoderma 333, 1–9. doi: 10.1016/j.geoderma.2018.07.018
Sharma, S., Thind, H. S., Yadvinder-Singh, A., Sidhu, H. S., Jat, M. L., and Parihar, C. M. (2019). Effects of crop residue retention on soil carbon pools after 6 years of rice–wheat cropping system. Environ. Earth Sci. 78:296. doi: 10.1007/s12665-019-8305-1
Shroder, S.. (2020). Cover Crops in California Agriculture: An Overview of Current Research. Progressive Crop Consultant. Available online at: https://progressivecrop.com/2020/07/cover-crops-in-california-agriculture-an- overview-of-current-research/ (accessed February 8, 2022)
Six, J., Bossuyt, H., Degryze, S., and Denef, K. (2004). A history of research on the link between (micro) aggregates, soil biota, and soil organic matter dynamics. Soil Tillage Res. 79, 7-31. doi: 10.1016/j.still.2004.03.008
Six, J., Conant, R. T., Paul, E. A., and Paustian, K. (2002). Stabilization mechanisms of soil organic matter: implications for C-saturation of soils. Plant Soil 241, 155–176. doi: 10.1023/A:1016125726789
Six, J., Elliott, E. T., and Paustian, K. (2000). Soil macroaggregate turnover and microaggregate formation: a mechanism for C sequestration under no-tillage agriculture. Soil Biol. Biochem. 32, 2099–2103. doi: 10.1016/S0038-0717(00)00179-6
Six, J., Frey, S. D., Thiet, R. K., and Batten, K. M. (2006). Bacterial and fungal contributions to carbon sequestration in agroecosystems. Soil Sci. Soc. Am. J. 70, 555–569. doi: 10.2136/sssaj2004.0347
Six, J., Guggenberger, G., Paustian, K., Haumaier, L., Elliott, E. T., and Zech, W. (2001). Sources and composition of soil organic matter fractions between and within soil aggregates. Eur. J. Soil Sci. 52, 607–618. doi: 10.1046/j.1365-2389.2001.00406.x
Sokol, N. W., and Bradford, M. A. (2019). Microbial formation of stable soil carbon is more efficient from belowground than aboveground input. Nat. Geosci. 12, 46–53. doi: 10.1038/s41561-018-0258-6
Sokol, N. W., Kuebbing, S. E., Karlsen-Ayala, E., and Bradford, M. A. (2019). Evidence for the primacy of living root inputs, not root or shoot litter, in forming soil organic carbon. New Phytol. 221, 233–246. doi: 10.1111/nph.15361
Soong, J. L., Castanha, C., Hicks Pries, C. E., Ofiti, N., Porras, R. C., Riley, W. J., et al. (2021). Five years of whole-soil warming led to loss of subsoil carbon stocks and increased CO2 efflux. Sci. Adv. 7:eabd1343. doi: 10.1126/sciadv.abd1343
Spohn, M., Karoline, K., Wolfgang, W., and Andreas, R. (2016). Microbial carbon use efficiency and biomass turnover times depending on soil depth – implications for carbon cycling. Soil Biol. Biochem. 96, 74–81. doi: 10.1016/j.soilbio.2016.01.016
Steele, M. K., Coale, F. J., and Hill, R. L. (2012). Winter annual cover crop impacts on no-till soil physical properties and organic matter. Soil Sci. Soc. Am. J. 76, 2164–2173. doi: 10.2136/sssaj2012.0008
Stewart, C. E., Paustian, K., Conant, R. T., Plante, A. F., and Six, J. (2007). Soil carbon saturation: concept, evidence and evaluation. Biogeochemistry 86, 19–31. doi: 10.1007/s10533-007-9140-0
Stewart, C. E., Paustian, K., Conant, R. T., Plante, A. F., and Six, J. (2009). Soil carbon saturation: implications for measurable carbon pool dynamics in long-term incubations. Soil Biol. Biochem. 41, 357–366. doi: 10.1016/j.soilbio.2008.11.011
Stockmann, U., Adams, M. A., Crawford, J. W., Field, D. J., Henakaarchchi, N., Jenkins, M., et al. (2013). The knowns, known unknowns and unknowns of sequestration of soil organic carbon. Agric. Ecosyst. Environ. 164, 80–99. doi: 10.1016/j.agee.2012.10.001
Talbot, J. M., and Treseder, K. K. (2012). Interactions among lignin, cellulose, and nitrogen drive litter chemistry–decay relationships. Ecology 93, 345–354. doi: 10.1890/11-0843.1
Tautges, N. E., Chiartas, J. L., Gaudin, A. C. M., O'Geen, A. T., Herrera, I., and Scow, K. M. (2019). Deep soil inventories reveal that impacts of cover crops and compost on soil carbon sequestration differ in surface and subsurface soils. Glob. Chang. Biol. 25, 3753–3766. doi: 10.1111/gcb.14762
Teague, W. R., Dowhower, S. L., Baker, S. A., Haile, N., DeLaune, P. B., and Conover, D. M. (2011). Grazing management impacts on vegetation, soil biota and soil chemical, physical and hydrological properties in tall grass prairie. Agric. Ecosyst. Environ. 141, 310–322. doi: 10.1016/j.agee.2011.03.009
Tian, G., Brussaard, L., and Kang, B. T. (1995). An index for assessing the quality of plant residues and evaluating their effects on soil and crop in the (sub-) humid tropics. Appl. Soil Ecol. 2, 25–32. doi: 10.1016/0929-1393(94)00033-4
Tiemann, L. K., Grandy, A. S., Atkinson, E. E., Marin-Spiotta, E., and McDaniel, M. D. (2015). Crop rotational diversity enhances belowground communities and functions in an agroecosystem. Ecol. Lett. 18, 761–771. doi: 10.1111/ele.12453
Topps, D., Khabir, M. I. u., Abdelmagid, H., Jackson, T., Iqbal, J., Robertson, B. K., et al. (2021). Impact of cover crop monocultures and mixtures on organic carbon contents of soil aggregates. Soil Syst. 5:43. doi: 10.3390/soilsystems5030043
Tribouillois, H., Fort, F., Cruz, P., Charles, R., Flores, O., Garnier, E., et al. (2015). A functional characterisation of a wide range of cover crop species: growth and nitrogen acquisition rates, leaf traits and ecological strategies. PLoS ONE 10:e0122156. doi: 10.1371/journal.pone.0122156
Trumbore, S.. (2009). Radiocarbon and soil carbon dynamics. Annu. Rev. Earth Planet. Sci. 37, 47–66. doi: 10.1146/annurev.earth.36.031207.124300
Turmel, M.-S., Speratti, A., Baudron, F., Verhulst, N., and Govaerts, B. (2015). Crop residue management and soil health: a systems analysis. Agric. Syst. 134, 6–16. doi: 10.1016/j.agsy.2014.05.009
Underwood, E. C., Klausmeyer, K. R., Cox, R. L., Busby, S. M., Morrison, S. A., and Shaw, M. R. (2009). Expanding the global network of protected areas to save the imperiled mediterranean biome. Conserv. Biol. 23, 43–52. doi: 10.1111/j.1523-1739.2008.01072.x
Van Oost, K., Govers, G., De Alba, S., and Quine, T. A. (2006). Tillage erosion: a review of controlling factors and implications for soil quality. Prog. Phys. Geogr. 30, 443–466. doi: 10.1191/0309133306pp487ra
Van-Camp, L., Bujarrabal, B., Gentile, A. R., Jones, R. J. A., Montanarella, L., Olazabal, C., et al. (2004). Reports of the Technical Working Groups Established Under the Thematic Strategy for Soil Protection. EUR 21319 EN/1. Luxembourg: Office for Official Publications of the European Communities, 872.
Villarino, S. H., Pinto, P., Jackson, R. B., and Piñeiro, G. (2021). Plant rhizodeposition: a key factor for soil organic matter formation in stable fractions. Sci. Adv. 7:eabd3176. doi: 10.1126/sciadv.abd3176
von Lützow, M., Kögel-Knabner, I., Ekschmitt, K., Flessa, H., Guggenberger, G., Matzner, E., et al. (2007). SOM fractionation methods: relevance to functional pools and to stabilization mechanisms. Soil Biol. Biochem. 39, 2183–2207. doi: 10.1016/j.soilbio.2007.03.007
von Lützow, M., Kögel-Knabner, I., Ludwig, B., Matzner, E., Flessa, H., Ekschmitt, K., et al. (2008). Stabilization mechanisms of organic matter in four temperate soils: development and application of a conceptual model. J. Plant Nutr. Soil Sci. 171, 111–124. doi: 10.1002/jpln.200700047
Wang, B., Gao, L., Yu, W., Wei, X., Li, J., Li, S., et al. (2019). Distribution of soil aggregates and organic carbon in deep soil under long-term conservation tillage with residual retention in dryland. J. Arid Land 11, 241–254. doi: 10.1007/s40333-019-0094-6
Wang, R., Yu, G., and He, N. (2021). Root community traits: scaling-up and incorporating roots into ecosystem functional analyses. Front. Plant Sci. 12:1500. doi: 10.3389/fpls.2021.690235
Wang, X., He, C., Liu, B., Zhao, X., Liu, Y., Wang, Q., et al. (2020). Effects of residue returning on soil organic carbon storage and sequestration rate in China's croplands: a meta-analysis. Agron 10:691. doi: 10.3390/agronomy10050691
Weiss, M. S., Abele, U., Weckesser, J., Welte, W., Schiltz, E., and Schulz, G. E. (1991). Molecular architecture and electrostatic properties of a bacterial porin. Science 254, 1627–1630. doi: 10.1126/science.1721242
Whalley, W. R., Riseley, B., Leeds-Harrison, P. B., Bird, N. R. A., Leech, P. K., and Adderley, W. P. (2005). Structural differences between bulk and rhizosphere soil. Eur. J. Soil Sci. 56, 353–360. doi: 10.1111/j.1365-2389.2004.00670.x
White, K. E., Brennan, E. B., Cavigelli, M. A., and Smith, R. F. (2020). Winter cover crops increase readily decomposable soil carbon, but compost drives total soil carbon during eight years of intensive, organic vegetable production in California. PLoS ONE 15:e0228677. doi: 10.1371/journal.pone.0228677
Wiesmeier, M., Hübner, R., Barthold, F., Spörlein, P., Geuß, U., Hangen, E., et al. (2013). Amount, distribution and driving factors of soil organic carbon and nitrogen in cropland and grassland soils of southeast Germany (Bavaria). Agric. Ecosyst. Environ. 176, 39–52. doi: 10.1016/j.agee.2013.05.012
Wilson, C. H., Strickland, M. S., Hutchings, J. A., Bianchi, T. S., and Flory, S. L. (2018). Grazing enhances belowground carbon allocation, microbial biomass, and soil carbon in a subtropical grassland. Glob. Chang. Biol. 24, 2997–3009. doi: 10.1111/gcb.14070
Wilson, G. W. T., Rice, C. W., Rillig, M. C., Springer, A., and Hartnett, D. C. (2009). Soil aggregation and carbon sequestration are tightly correlated with the abundance of arbuscular mycorrhizal fungi: results from long-term field experiments. Ecol Lett. 12, 452–461. doi: 10.1111/j.1461-0248.2009.01303.x
Witzgall, K., Vidal, A., Schubert, D. I., Höschen, C., Schweizer, S. A., Buegger, F., et al. (2021). Particulate organic matter as a functional soil component for persistent soil organic carbon. Nat. Commun. 12:4115. doi: 10.1038/s41467-021-24192-8
Wulanningtyas, H. S., Gong, Y., Li, P., Sakagami, N., Nishiwaki, J., and Komatsuzaki, M. (2021). A cover crop and no-tillage system for enhancing soil health by increasing soil organic matter in soybean cultivation. Soil Tillage Res. 205:104749. doi: 10.1016/j.still.2020.104749
Yang, Y., Tilman, D., Furey, G., and Lehman, C. (2019). Soil carbon sequestration accelerated by restoration of grassland biodiversity. Nat. Commun. 10:718. doi: 10.1038/s41467-019-08636-w
Yin, W., Qiang, C., Guo, Y., Fan, Z., Hu, F., Fan, H., et al. (2020). Straw and plastic management regulate air-soil temperature amplitude and wetting-drying alternation in soil to promote intercrop productivity in arid regions. Field Crops Res. 249:107758. doi: 10.1016/j.fcr.2020.107758
Zani, C. F., Lopez-Capel, E., Abbott, G. D., Taylor, J. A., and Cooper, J. M. (2021). Effects of integrating grass-clover leys with livestock into arable crop rotations on soil carbon stocks and particulate and mineral-associated soil organic matter fractions in conventional and organic systems. Soil Use Manag. 38, 448–465. doi: 10.1111/sum.12754
Zhao, X., Liu, B.-Y., Liu, S.-L., Qi, J.-Y., Wang, X., Pu, C., et al. (2020). Sustaining crop production in China's cropland by crop residue retention: a meta-analysis. Land Degrad. Dev. 31, 694–709. doi: 10.1002/ldr.3492
Zhou, G., Zhou, X., He, Y., Shao, J., Hu, Z., Liu, R., et al. (2017). Grazing intensity significantly affects belowground carbon and nitrogen cycling in grassland ecosystems: a meta-analysis. Glob. Chang. Biol. 23, 1167–1179. doi: 10.1111/gcb.13431
Keywords: cover crops, deep soil, stabilization, destabilization, rhizosphere, particulate organic matter (POM), mineral-associated organic matter (MAOM), management
Citation: Moukanni N, Brewer KM, Gaudin ACM and O'Geen AT (2022) Optimizing Carbon Sequestration Through Cover Cropping in Mediterranean Agroecosystems: Synthesis of Mechanisms and Implications for Management. Front. Agron. 4:844166. doi: 10.3389/fagro.2022.844166
Received: 27 December 2021; Accepted: 08 March 2022;
Published: 21 April 2022.
Edited by:
Mohamed Hijri, Université de Montréal, CanadaReviewed by:
Zhenke Zhu, Institute of Subtropical Agriculture (CAS), ChinaCopyright © 2022 Moukanni, Brewer, Gaudin and O'Geen. This is an open-access article distributed under the terms of the Creative Commons Attribution License (CC BY). The use, distribution or reproduction in other forums is permitted, provided the original author(s) and the copyright owner(s) are credited and that the original publication in this journal is cited, in accordance with accepted academic practice. No use, distribution or reproduction is permitted which does not comply with these terms.
*Correspondence: Nadia Moukanni, bm1vdWthbm5pQHVjZGF2aXMuZWR1; Anthony T. O'Geen, YXRvZ2VlbkB1Y2RhdmlzLmVkdQ==
†These authors have contributed equally to this work
Disclaimer: All claims expressed in this article are solely those of the authors and do not necessarily represent those of their affiliated organizations, or those of the publisher, the editors and the reviewers. Any product that may be evaluated in this article or claim that may be made by its manufacturer is not guaranteed or endorsed by the publisher.
Research integrity at Frontiers
Learn more about the work of our research integrity team to safeguard the quality of each article we publish.