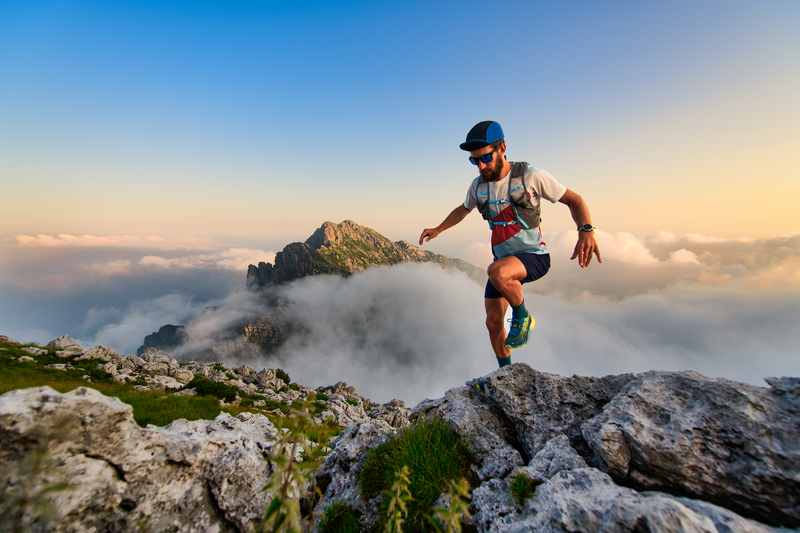
95% of researchers rate our articles as excellent or good
Learn more about the work of our research integrity team to safeguard the quality of each article we publish.
Find out more
ORIGINAL RESEARCH article
Front. Agron. , 14 October 2022
Sec. Plant-Soil Interactions
Volume 4 - 2022 | https://doi.org/10.3389/fagro.2022.1018251
Palmer amaranth (Amaranthus palmeri S. Watson) is a troublesome weed that can result in substantial crop yield loss in irrigated and rainfed agricultural systems of the U.S. Great Plains. Knowledge of the transpiration response to soil drying is necessary to better understand the competitive nature of Palmer amaranth against crops in limiting soil moisture conditions. The objective was to quantify the soil matric potential at which the transpiration rate of Palmer amaranth starts to decline during soil drying conditions. A greenhouse experiment consisting of six replications of well-watered and stressed plants growing in columns of a packed silt loam soil on automated load cells was established in May 2021 in Manhattan, KS. Hourly transpiration rates were computed as the difference of two consecutive mass readings. Stomatal conductance was measured every other day on the topmost fully developed leaf using a leaf porometer. Soil matric potential was estimated using a measured soil water retention curve. The stomatal conductance of plants undergoing soil drying conditions started to decrease at a soil matric potential of -120 kPa and the plant-level transpiration rate started to decrease at -176 kPa. Palmer amaranth exhibited high (246 g h-1) day-time transpiration rates when soil water was available at low tension levels (10 to 33 kPa). In advanced stages of development, well-watered plants also exhibited nighttime transpiration that accounted for ~10% of the day-time transpiration total. Mean transpiration use efficiency of well-watered and stressed plants considering total dry biomass was similar (P = 0.247), with values of 10.9 and 11.6 g kg-1, respectively. Overall, this study substantiates the competitive nature of Palmer amaranth for soil water in sub-humid and semi-arid environments and highlights the importance of early control of Palmer amaranth to minimize competition for soil moisture.
Palmer amaranth (Amaranthus palmeri S. Watson) has been ranked the most troublesome weed among all terrestrial crop and non-crop areas in the U.S. and Canada (Van Wychen, 2016) and is one of the most troublesome and economically detrimental warm-season annual weeds for cropping systems in the U.S. Great Plains (Massinga et al., 2003; Webster and Nichols, 2012; Kumar et al., 2020). This problem has been exacerbated by the appearance of biotypes resistant to ALS-inhibiting (Sprague et al., 1997; Burgos et al., 2001), dinitroaniline (Gossett et al., 1992), triazine (Jhala et al., 2014), 4-hydroxyphenylpyruvate dioxygenase (HPPD)-inhibiting (Nakka et al., 2017; Küpper et al., 2018), and glyphosate herbicides (Culpepper et al., 2006; Kumar et al., 2019), which reduces herbicide options and increases the costs of in-season weed control. Palmer amaranth is a C4 plant with a high photosynthetic capacity and a rapid growth rate (Ehleringer, 1983) that can compete with summer crops for limited light, soil nutrients, and soil moisture (Massinga et al., 2003; Korres et al., 2017). Thus, uncontrolled Palmer amaranth in agricultural fields can cause substantial crop yield loss in summer crops. For instance, previous research studies in east Texas showed that cotton (Gossypium hirsutum L.) lint yield was reduced by up to 54% due to competition with Palmer amaranth (Morgan et al., 2001). Similarly, field experiments in Kansas with up to eight plants of Palmer amaranth per linear meter resulted in up to 91% yield loss in irrigated corn (Zea mays L.) (Massinga et al., 2001) and 79% yield loss in rainfed soybean [Glycine max (L.) Merr.] (Bensch et al., 2003).
Competition for water resources between summer crops and Palmer amaranth becomes particularly relevant in rainfed cropping systems in sub-humid and semi-arid regions, where soil water availability is often limited during part or the entire growing season. Palmer amaranth is a native plant of the Sonoran Desert (Ehleringer, 1983) and has adaptations for drought conditions such as extended seedling emergence, rapid initial shoot and root growth rate, and a combination of fine shallow roots and deep fibrous roots that may provide a competitive advantage for soil water uptake against crops in water limiting conditions (Berger et al., 2015). Previous studies focusing on the physiological responses of Palmer amaranth under water-limited conditions have mainly described photosynthetic characteristics as a function of leaf conductance and leaf water potential (Ehleringer, 1983; Berger et al., 2015). Some studies have also investigated the impact of maternal water environments on germination properties of progeny seeds (Matzrafi et al., 2021). However, little research has been conducted to better understand the transpiration responses of Palmer amaranth to soil drying at the plant level at daily and sub-daily scales. From a physiological point of view, Palmer amaranth is expected to follow transpiration dynamics similar to that of agricultural crops. When soil water availability is adequate, plant transpiration can typically cope with the water demand imposed by atmospheric conditions (Denmead and Shaw, 1962). Under this circumstance, plants usually exhibit a transpiration rate that is at or near the potential transpiration rate (Ray et al., 2002). As the available soil water declines and plants have to do more work to uptake soil water (Minasny and McBratney, 2003), plants can no longer maintain maximum transpiration rates. At this point, a chain of physiological reactions trigger defense mechanisms like stomatal closure and leaf rolling to reduce water losses (Corlett et al., 1994; Ray et al., 2002). Thus, the objective of this experiment was to quantify the transpiration rate and the soil matric potential at which the transpiration rate of Palmer amaranth starts to decline during soil drying conditions compared to well-watered plants. Since Palmer amaranth is a native plant of desert areas and its competitive nature for resources has been well documented in the scientific literature, we hypothesize that the onset at which the transpiration rate of Palmer amaranth starts to decline occurs at lower soil matric potentials (i.e., more drought resistant) than typical agricultural crops. Quantifying the transpiration rate of Palmer amaranth under well-watered and water deficit conditions will contribute to the growing body of scientific literature characterizing the competitive nature of Palmer amaranth for water resources in agricultural cropping systems.
A greenhouse experiment was set to study transpiration responses of Palmer amaranth to soil drying from 5 May to 31 May 2021 in the facilities of Kansas State University in Manhattan, KS (39°11’39.2”N, 96°35’03.0”W, 312 m a.s.l.). The experiment consisted of growing plants of Palmer amaranth in packed soil columns under well-watered and a soil drying treatments with six replicates each (i.e., total of 12 plants, Figure 1) using a complete randomized design since no apparent gradients in incident solar radiation, air temperature, and air circulation were evident in the small area (4 m2) occupied by the experiment. White, plastic cylindrical containers (28.5 cm in inner diameter and 48.5 cm in height) were packed with a silt loam soil at a bulk density of 1.15 g cm-3. The soil in this study had 17% sand and 26% clay, which was determined using the hydrometer method (Gavlak et al., 2003). The chemical properties of the soil before any fertilizer application consisted of total organic carbon of 1.34% (loss-on-ignition method), total nitrogen (N) of 0.12%, phosphorus (P) of 5 ppm (Mehlich-3), potassium (K) of 200 ppm, a pH value of 7.3, and a bulk electrical conductivity of 0.5 μS cm-1 (analysis performed by the Kansas State University Soil Fertility Laboratory). Containers were packed gradually by dividing the container volume into three layers to better control the bulk density during the packing process. Containers were filled almost to the top, leaving a small gap of ~3 cm to contain water during irrigation events. The soil was amended with 51 g (17 g per layer) of a fast-release commercial fertilizer (Fertilizer analysis 24-8-16, Miracle Gro, Inc.), 192 g (64 g per layer) of a slow-release fertilizer (Osmocote, The Scotts Company, LLC.), and 23.1 g (7.7 g per layer) of fungicide (Marathon, active ingredient: Imidacloprid) according to the manufacturer’s label recommendation for healthy plants. All containers were watered and then left covered for two days before receiving the plants to allow for soil water redistribution across the entire soil column.
Figure 1 Image illustrating the experimental set up in the greenhouse. The image shows plants of Palmer amaranth growing in containers on top of automated load cells.
Seeds of Palmer amaranth used in this study were collected from plants grown at the Kansas State University Department of Agronomy Ashland Bottoms Experiment Field located near Manhattan, KS. Plants were grown individually using seedling trays until they had three true leaves. Twelve plants of similar size were selected and carefully transplanted into the individual containers with packed soil for the rest of the experiment. Thus, each container had one plant of Palmer amaranth located in the center of the container. The transplanting step was necessary to ensure uniformity in the initial size of the plants. Seedling roots were surrounded by soil at all times and the transplanting did not impose any visual stress on the plants. All plants were watered periodically for about two weeks until they adapted to the new container and had a size that allowed us to measure mass changes due to transpiration. Plants of Palmer amaranth grew three to eight leaves in ~15 days.
The day before starting the experiment, all the containers were watered and brought to a volumetric water content of 0.33 cm3 cm-3, which corresponded to a matric potential of ~8 kPa (Figure 2). This soil moisture condition ensured sufficient available soil water and an air-filled porosity >10% to avoid oxygen diffusion rate from becoming a limiting factor (Silva et al., 2004). The initial volumetric water content of each container was within 0.015 cm3 cm-3 of the average water content of all the containers. Then, the surface of each container was covered with an impermeable clear polyethylene film (1.52 mm thickness) carefully wrapped around the stem of Palmer amaranth plants to prevent evaporative losses during the experiment. To further prevent evaporative losses around the stem, a plastic clip (similar to a bread clip) was attached to seal any remaining gap around the stem. The wrapping of the film around the stem was checked periodically to ensure that plants were free to grow. Well-watered plants received periodic irrigation to maintain a matric potential of -10 kPa based on soil tensiometers installed at 20 cm depth, which was the depth with the highest root density. Tensiometers were checked twice a day and irrigation of well-watered plants was done manually. Stressed plants did not receive any irrigation after the onset of the experiment.
Figure 2 Soil water retention curve for the silt loam soil used in this study. Different markers represent observations for the three undisturbed soil samples and the solid line represents the fitted van Genucthen model. MAE is the mean absolute error of the fitted model.
During the entire study period, the mass of each container was tracked with automated load cells (model PL-100, Meter, Inc.) connected to a datalogger (model CR300, Campbell Scientific, Inc.). The load cells were factory-calibrated and included temperature compensation. The minimum resolution of the scales was 5 g, which is equivalent to a resolution of 0.078 mm given the area of the containers. The mass of each container was recorded at 15-minute intervals and averaged hourly. Hourly plant transpiration was calculated as the difference between two consecutive mass observations. In addition, we also recorded air temperature and relative humidity (model CS125, Campbell Scientific, Inc.), and photosynthetically active radiation (PAR, 389-692 nm) with a quantum sensor (model CS310, Campbell Scientific, Inc.). Both sensors were installed at about 1.5 m from the top of fully developed plants. Vapor pressure deficit (VPD) was estimated from observations of air temperature and relative humidity using Tetens equation (Campbell and Norman, 2000) (Figure 3). During the experiment, we recorded plant height, stem diameter, and stomatal conductance using a leaf porometer (model SC-1, Meter, Inc.). Plant height was measured from the base of the plant to the highest extended leaf. Stomatal conductance was measured on the topmost fully expanded leaf of each plant every other day at 1600 hours. In this experiment plant transpiration was determined solely from changes in mass. Leaf stomatal conductance was recorded as an auxiliary variable to help us refine the timing at which plants started to show signs of stress due to limiting soil water. The greenhouse ceiling consisted of clear glass without whitewash and supplemental light was provided from 0800 to 1800 hours using four 1200 W ballasts of high-pressure sodium uniformly distributed at about 1.5 m from the top of the canopy to closely match the solar irradiance during the summer period at this latitude.
Figure 3 Air temperature (A), vapor pressure deficit (B), and greenhouse and outdoors photosynthetically active radiation (PAR, 389-692 nm) (C) during the experiment from 5 May to 31 May 2021. Outdoors PAR was estimated as 48% of the incident solar radiation.
The experiment was terminated when plants in the stress treatment reached a maximum transpiration rate <6.4 g h-1, a value that is close to the resolution of 5 g of the automated scales. At this point, plant leaves and the stem apex exhibited severe wilting without recovery overnight (Hendrickson and Veihmeyer, 1945). At the end of the experiment, three undisturbed soil samples were collected from random containers to create a soil water retention curve. Since all the containers had the same soil type and packing procedure, the three soil samples from random containers were used to create a single soil water retention curve representative of the silt loam soil. The wet end of the soil water retention curve was determined in the laboratory using precision mini-tensiometers (model Hyprop 2, Meter, Inc.) and the dry end of the soil water retention curve was determined with a dew point soil water potential meter (model WP4C, Meter, Inc.) (Figure 2). The van Genuchten (Van Genuchten, 1980) soil water retention model was fitted to the observations using least squares using Python 3.7.9 and the SciPy optimize module (Virtanen et al., 2020). The van Genuchten model (Equation 1) is defined as:
where θ (cm3 cm-3) is the volumetric soil water content, θs(cm3 cm-3) is the saturated water content, θr (cm3 cm-3) is the residual water content, ψm (kPa) is the soil matric potential, α (kPa-1) is a fitting parameter sometimes related to the inverse of the air-entry pressure, n (unitless) is a parameter related to the pore size distribution, and m (unitless) was assumed equal to 1–1/n (Schaap et al., 2001). Statistical differences between treatment means for each variable were tested using an independent two-sample t-test. The statistical analysis was conducted using the PROC TTEST in SAS (SAS Institute version 9.4). The assumptions of normality and homoscedasticity were tested using quantile-quantile plots (i.e., Q-Q plots) and the equality of variance was also tested using a folded F-test, which are provided by default in the PROC TTEST routine. In cases where the assumption of equality of variance was not met, we used the output corresponding to the Satterthwaite approximation.
At the end of the experiment, plants of Palmer amaranth were harvested by separating the shoot and the root at the soil surface. Shoots were placed in paper bags and dried at 65 °C until constant mass. The root systems were extracted by damping the container soil on a large tray and removing individual roots by hand with the help of a 2 mm sieve. Roots were washed and dried at 65 °C until constant mass. The transpiration use efficiency (TUE, g kg-1) was computed by dividing the shoot dry biomass (TUEshoot) and the total plant dry biomass (TUEtotal) by the total transpiration over the studied period (Sinclair et al., 1984). In this study we favored the computation of TUE on a mass basis to avoid affecting the TUE calculations by the choice of container area.
The point at which the plant transpiration rate started to decrease due to limiting soil moisture conditions was defined using two independent approaches. The first approach consisted of using periodic readings of leaf-level stomatal conductance between well-watered and stressed plants. First appearance of significant differences in stomatal conductance enabled early detection of reduced transpiration rates as a result of soil water deficit, which may be difficult to capture with the load cells. A second plant-level approach consisted of defining the point at which the average cumulative transpiration between well-watered and stressed plants started to diverge. In this case, a stress inception region was defined between the point at which the cumulative transpiration starts to diverge and the point at which the 95% confidence intervals no longer overlap. Some previous studies have used a linear-plateau approach fitted to the normalized transpiration ratio as a function of the fraction of transpirable water (Ray and Sinclair, 1997; Schmidt et al., 2011; Gholipoor et al., 2012; Andrianasolo et al., 2016). The original methodology proposed by Ray and Sinclair (1997) is practical, but requires the computation of the fraction of transpirable soil water, which relies on the definition of a drained upper limit. This upper limit is usually estimated by allowing a thoroughly wetted soil to freely drain for 24-48 hours. This approach works well in experiments using potting soils and coarse-textured soils, but not with fine-textured mineral soils, which can result in soil water held at much lower tension levels than would otherwise occur in field conditions (Passioura, 2006). A recent study also found that the definition of upper limits for plant available water based on fixed soil matric potentials and flux thresholds do not constitute an adequate soil physical quantity to assess soil water availability (van Lier, 2017). Thus, in this study we favored the use of accurate hourly mass observations to estimate the soil matric potential at which stressed plants started to exhibit a lower transpiration rate, which is less subjected to researcher bias due to arbitrary selection of upper limits.
The experiment spanned atmospheric conditions that are typical of spring and summer weather conditions in this region, with air temperature ranging mostly from 18 to ~35 °C, VPD ranging from 1.0 to 4.8 kPa, and PAR reaching values of up to 2,000 μmol m-2 s-1 near solar noon in days with clear sky conditions (Figure 3). The minimum air temperature in this study was slightly above the typical base temperature of 16 °C for growth and development of Palmer amaranth, and the maximum temperature was similar to the optimal temperature for net photosynthesis of ~36 °C (Ward et al., 2013). Light conditions inside the greenhouse were similar to outdoors PAR levels observed at the Manhattan station of the Kansas Mesonet (Patrignani et al., 2020), which is located at about 1 km from the experiment site.
As expected, plants of both well-watered and stressed treatments had nearly identical transpiration rates during the initial stages of the experiment in which soil water was not limiting (Figure 4). The transpiration rate of both well-watered and stressed plants was lowest during the night and peaked during midday, when solar radiation and VPD values were highest. During this initial period, the stomatal conductance of well-watered and stressed plants was not significantly different (Figure 4), which supports the similar transpiration rates observed with the load cells. However, as plants of the stressed treatment started to deplete the available soil water, the transpiration rate started to decline compared to the transpiration rate of well-watered plants (Figure 4A). Similarly, the cumulative transpiration of well-watered and stressed plants started to diverge, a point that indicates the approximate onset of stress due to soil water deficit conditions (Figure 4B). The point at which we first observed significant differences in the mean stomatal conductance between treatments was on 17 May at 1600 hours (P = 0.047, point P1 in Figure 4A), which corresponded to a volumetric water content of 0.195 cm3 cm-3 and a soil matric potential of -120 kPa. Similarly, the point at which the average cumulative transpiration between the well-watered and stressed plants started to diverge occurred on 18 May 2021 at 1300 hours (dashed line and point P2 in Figure 4A). At this point, the soil of the stressed plants had an average volumetric water content of 0.182 cm3 cm-3 and an estimated soil matric potential of -176 kPa. Plants in the well-watered treatment had an average height of 36.7 cm (SD = 6.1 cm) and stressed treatments had an average height of 37.9 cm (SD = 5.3 cm), values that were not statistically different using a t-test (P = 0.723), thus providing evidence that plants of the two treatments were uniform at the inception of the soil water stress. The range in soil matric potential between -120 kPa at P1 and -176 kPa at P2 found in this study was similar to the recommended soil matric potential to irrigate crops like alfalfa (Medicago sativa), cotton (Gossypium hirsutum), and tomato (Solanum lycopersicum L.) (Taylor, 1965; Hanson et al., 2000), which typically represent plants on the tolerant spectrum for soil water deficits.
Figure 4 Transpiration rate (A) and cumulative transpiration (B) for well-watered and stressed plants of Palmer amaranth. The graphs shows the periods in which plants were clearly under no stress (light green area) and under stress (light orange area) due to soil water deficit. The figure also shows the onset at which stressed plants started to exhibit lower transpiration rates (vertical dashed line, point ②) than well-watered plants. The central white area represents a period of about 3 days in which plants started to exhibit evidence of water stress. Point ① represents the date at which we first detected significant differences in stomatal conductance between treatments. The interval between points ② and ③ (hatched area) represents a 58-hour period during which visual signs of turgor loss were observed for the first time in the stressed treatment. Between points ① and ③ the transpiration rate depended on the interaction between soil moisture conditions and atmospheric demand. The shaded area around cumulative transpiration curves represent the 95% confidence intervals based on six replicates. The soil matric potential is the median value of the six replicates. For the measurements of stomatal conductance, NS means non-significantly different at the 5% level, * means P<0.05, and ** means significant at P<0.01 using an independent two-sample t-test.
While during the period indicated between P1 and P2 we did not observe any visual signs of turgor loss on leaves and stems, all plants undergoing soil drying exhibited clear visual signs of turgor loss between P2 and the point at which the 95% CI between the treatment means no longer overlapped (point P3 in Figure 4A). The average volumetric water content at P3 was 0.154 cm3 cm-3 and the soil matric potential was -536 kPa. The period from P2 to P3 spanned a total of 58 hours and was characterized by a significantly different (P <0.001) mean stomatal conductance between well-watered plants (441 mmol m-2 s-1) and stressed plants (117 mmol m-2 s-1), a difference that, as expected, persisted until the end of the experiment. Our findings suggest that the transpiration rate and stomatal conductance of Palmer amaranth are affected by soil water deficits before exhibiting visual signs of turgor loss. An observation worth mentioning is that even well-watered plants occasionally showed temporary decreases in the transpiration rate as a consequence of the high and sustained midday atmospheric demand throughout the study period. Similar responses have been observed in other studies involving grain sorghum under well-watered conditions, possibly as a consequence of the internal hydraulic resistance of the plant vascular tissue or by the limited plant root water uptake (Sinclair et al., 2005).
Unlike previous studies in which plants were subjected to constant VPD conditions (Ray and Sinclair, 1997; Gholipoor et al., 2012), our study included diurnal oscillations in VPD conditions driven by changes in greenhouse temperature and relative humidity conditions, which are likely closer to field conditions. Thus, in this study, transpiration rates were modulated by both the availability of soil moisture and the atmospheric demand. This interaction across the soil-plant-atmosphere continuum was clearly evident between P2 and P3 in Figure 4A. For instance, on 18 May with a peak VPD of ~3.5 kPa, the midday transpiration rate of well-watered and stressed plants dropped for a few hours. On the other hand, on 19 May with a peak VPD of ~3 kPa and an overall lower atmospheric demand during the entire day, the well-watered and stressed plants exhibited comparable midday and daily transpiration rates. Our findings are in agreement with the landmark study by Denmead and Shaw (1962), in which pots with corn plants growing in the field exhibited different transpiration responses based on coupled soil moisture and atmospheric conditions.
From 20 May 2021 onwards, plants in the soil drying treatment were no longer able to cope with the atmospheric demand, and the transpiration rate started to decline rapidly compared to the well-watered plants. After 22 May 2021, plants were slowly approaching the permanent wilting point and exhibited severe water stress characterized by a generalized loss of turgor in leaves and stems. At this stage, well-watered plants were also growing rapidly, with average hourly transpiration rates at solar noon as high as 246 g h-1 (Figure 4A) and stomatal conductance values of the top fully expanded leaves of 394 mmol m-2 s-1 under VPD conditions of ~4.1 kPa on 27 May. The highest recorded transpiration rate for a single plant was 310 g h-1. The high hourly transpiration rate of plants of Palmer amaranth were likely a consequence of the lack of competition for soil water and a dense root system exploring the entire volume of the container. While this high transpiration rate remains to be validated in field conditions, our study shows that Palmer amaranth can exhibit high transpiration rates when soil water is available at or near field capacity conditions (i.e., soil matric potentials between -10 and -33 kPa). On 27 May 2021 plants of Palmer amaranth reached an average height of 79.9 cm (SD = 7.22 cm) and an average stem diameter at the base of the plant of 18.8 mm (SD = 2.67 mm). At this time, we recorded a median daily transpiration total across the six plants of 2,020 g, a maximum daily transpiration total for a single plant of 2,420 g, and a maximum day-time transpiration total of 1,857 g. High transpiration rates observed in this study coupled with the high photosynthetic rates (Ehleringer, 1983) reported by previous studies could be the key for outcompeting other plants during the growing season when resources are plentiful.
Hourly measurements of plant transpiration also allowed us to detect the occurrence of nighttime transpiration, which unlike daytime transpiration, is a far less reported process. Considering the entire duration of the experiment, nighttime transpiration in well-watered plants represented 7% of the total transpiration (Figure 5). In the period from 21 May to 29 May 2021, well-watered plants of Palmer amaranth exhibited measurable transpiration during nighttime hours (hours with PAR<1 μmol m-2 s-1), with the highest nighttime transpiration total reaching a value of 147 g from 28 May at 2200 hours to 29 May at 0600 hours, a value that represented ~10% of the average daytime transpiration on 28 and 29 May. During the nighttime, the average VPD of the greenhouse was 1.4 kPa. Our findings are in agreement with previous studies also conducted in controlled environmental conditions, in which the magnitude of nighttime transpiration of bean (Phaseolus vulgaris L.) and cotton resulted in 12 to 23% of daytime water losses (De Dios et al., 2015). In some plant species, nighttime transpiration is regulated by endogenous controls on stomatal conductance and has been associated with the nocturnal release of respiratory CO2, which in turn allows for root water uptake for use in leaf expansion, a positive feedback that would promote rapid growth rate (De Dios et al., 2015; Fricke, 2019). Nighttime transpiration can also be a consequence of residual (i.e., cuticular) transpiration. It remains unclear why nocturnal transpiration did not occur in earlier stages of the experiment. One possible reason is that in early stages of development, plants were too small to exhibit a measurable nocturnal transpiration rate with the automated scales. Additional studies in controlled conditions are necessary to confirm our findings and to investigate the underlying regulating mechanisms (if any) of nighttime transpiration in Palmer amaranth. The extent to which plants of Palmer amaranth exhibit nighttime transpiration in field conditions remains unknown and should also be the subject of future research studies. To our knowledge, this is the first study reporting the occurrence of nocturnal transpiration in Palmer amaranth.
At the end of the experiment, stressed plants still exhibited 138 g of daily transpiration even at soil matric potentials of -1,500 kPa. On the last day of the experiment, the average daily transpiration of the stressed plants was 32 g and the mean volumetric water content was 0.099 cm3 cm-3 (SD = 0.008 cm3 cm-3), a value 27% lower than the volumetric water content at the permanent wilting point (0.136 cm3 cm-3 at -1,500 kPa) and similar to the residual water content of 0.095 cm3 cm-3 obtained from the measured soil water retention curve. The soil matric potential values reported in this study are the average for the entire container, thus it is possible that plant roots were accessing pools of pore water held at higher (i.e., less negative) soil matric potentials. The small, but sustained soil water uptake at such low soil matric potentials observed in this study may be an additional competitive mechanism of Palmer amaranth to thrive in water-limited conditions. Our findings could be useful in modeling crop-weed competition studies, which often require the specification of parameters to constrain the lower limit for root water uptake in weeds and crops.
During the experiment, well-watered plants averaged a cumulative transpiration of 20.4 kg (equivalent to 321 mm based on selected container diameter), while stressed plants averaged a cumulative transpiration of only 6.5 kg (equivalent to 102 mm based on the selected container diameter) (Table 1). As expected, the mean cumulative transpiration between the treatments was significantly different based on a t-test (P <0.001). At the end of the experiment, well-watered plants had a mean dry shoot biomass of 198 g (SD = 9.5 g) and mean dry root biomass of 24 g (SD = 9.1 g), resulting in a calculated root-to-shoot ratio of 0.12. On the other hand, stressed plants had a mean dry shoot biomass of 63 g (SD = 5.3 g) and a mean dry root biomass of 12 g (SD = 1.8 g), which resulted in a mean root-to-shoot ratio of 0.19 (Table 1). In a similar study comparing corn plants under well-watered and soil water deficit conditions, shoot growth was more affected than root growth, resulting in an increased root-to-shoot ratio in plants under stress (Benjamin et al., 2014), which is the same response that we observed in our study. The resulting TUE based on shoot dry biomass (TUEshoot) and total dry biomass (TUEtotal) between the well-watered and stressed plants was similar and not significantly different among treatments (Table 1). The mean TUEshoot for the well-watered plants was 9.80 g kg-1 (SD = 1.01 g kg-1) and 9.72 g kg-1 (SD = 0.97 g kg-1) for the stressed plants (Table 1). Similarly, TUEtotal was 10.9 g kg-1 (SD = 1.01 g kg-1) for well-watered plants and 11.6 g kg-1 (SD = 0.79 g kg-1) for the stressed plants (Table 1). Given that both treatments were exposed to the same environmental conditions and soil nutritional level, our results follow the fundamental assumptions made by Tanner and Sinclair (1983), in which dry matter reduction is proportional to the decrease in transpiration caused by the soil water deficit.
Table 1 Total transpiration (T), shoot dry mass, root dry mass, total dry mass, shoot-to-root ratio, and transpiration use efficiency (TUE) based on shoot and total biomass for well-watered and stressed plants of Palmer amaranth.
In this study we measured the transpiration rate of Palmer amaranth under well-watered and soil drying conditions. The leaf stomatal conductance of plants undergoing soil drying conditions started to be affected at a soil matric potential of -120 kPa and the plant-level transpiration rate started to be affected at a soil matric potential of -176 kPa, both the reduction in stomatal conductance and plant transpiration rate occurring before plants exhibited visual symptoms of turgor loss. These values of soil matric potential provide some evidence to support our initial hypothesis that the transpiration response Palmer amaranth is similar to that of crops on the tolerant spectrum for soil water deficits. We also found that Palmer amaranth can exhibit high transpiration rates when soil water is available at low tension levels (i.e., field capacity), with individual well-watered plants reaching average transpiration rates of up to 246 g h-1 and up to 2,020 g per day for plants with an approximate height of 80 cm. The TUE of well-watered and stressed plants remained almost unchanged and during advanced stages of growth, nighttime transpiration accounted for ~10% of the day-time transpiration total. Our study offers key insights needed to better understand the competitive nature of Palmer amaranth and manage this troublesome weed in sub-humid and semi-arid environments. Future research studies should corroborate the TUE and the magnitude of nighttime transpiration reported in this study and should investigate the physiology of nighttime transpiration as a possible mechanism to outcompete agricultural crops for limited water resources.
The raw data supporting the conclusions of this article are available in the Supplementary Material. Additional information about the study will be made available by the authors, without undue reservation.
SC contributed with conceptualization, experiment design, data collection, numerical and statistical analyses, manuscript writing, and literature review. AP contributed with data processing and article writing. All authors contributed to the article and approved the submitted version.
This project was partly supported by the Agriculture and Food Research Initiative Competitive Grant no. 2019-68012-29888 from the USDA National Institute of Food and Agriculture and by the USDA Hatch Multistate Research Project W4188.
The authors declare no conflicts of interest. This project was partly supported by the Agriculture and Food Research Initiative Competitive Grant no. 2019-68012-29888 from the USDA National Institute of Food and Agriculture, by the Kansas Agricultural Experiment Station, and by the USDA Hatch Multistate Research Project W4188. We would like to thank Mithila Jugulam, Sarah Lancaster, and Anita Dille for their helpful edits, comments, and feedback on this manuscript. We are also thankful to Dr. Jugulam’s lab for providing us with the necessary seeds of Palmer amaranth to conduct this study, and to Maria Paula Garcia and Elizabeth Frieden for helping with experiment set up and root extraction. This manuscript is contribution no. 23-066-J from the Kansas Agricultural Experiment Station.
The authors declare that the research was conducted in the absence of any commercial or financial relationships that could be construed as a potential conflict of interest.
All claims expressed in this article are solely those of the authors and do not necessarily represent those of their affiliated organizations, or those of the publisher, the editors and the reviewers. Any product that may be evaluated in this article, or claim that may be made by its manufacturer, is not guaranteed or endorsed by the publisher.
The Supplementary Material for this article can be found online at: https://www.frontiersin.org/articles/10.3389/fagro.2022.1018251/full#supplementary-material
Andrianasolo F. N., Casadebaig P., Langlade N., Debaeke P., Maury P. (2016). Effects of plant growth stage and leaf aging on the response of transpiration and photosynthesis to water deficit in sunflower. Funct. Plant Biol. 43 (8), 797–805. doi: 10.1071/FP15235
Benjamin J. G., Nielsen D. C., Vigil M. F., Mikha M. M., Calderon F. (2014). Water deficit stress effects on corn (Zea mays, l.) root: shoot ratio. Open J. Soil Sci. 2014, 151–160. doi: 10.4236/ojss.2014.44018
Bensch C. N., Horak M. J., Peterson D. (2003). Interference of redroot pigweed (Amaranthus retroflexus), palmer amaranth (A. palmeri), and common waterhemp (A. rudis) in soybean. Weed Sci. 51 (1), 37–43. doi: 10.1614/00431745(2003)051[0037:IORPAR]2.0.CO;2
Berger S. T., Ferrell J. A., Rowland D. L., Webster T. M. (2015). Palmer amaranth (Amaranthus palmeri) competition for water in cotton. Weed Sci. 63 (4), 928–935. doi: 10.1614/WS-D-15-00062.1
Burgos N. R., Kuk Y. I., Talbert R. E. (2001). Amaranthus palmeri resistance and differential tolerance of amaranthus palmeri and amaranthus hybridus to ALS-inhibitor herbicides. Pest Manage. Sci: formerly Pesticide Sci. 57 (5), 449–457. doi: 10.1002/ps.308
Campbell G. S., Norman J. M. (2000). An introduction to environmental biophysics (New York:Springer Science & Business Media).
Corlett J. E., Jones H. G., Massacci A., Masojidek J. (1994). Water deficit, leaf rolling and susceptibility to photoinhibition in field grown sorghum. Physiologia Plantarum 92 (3), 423–430. doi: 10.1111/j.1399-3054.1994.tb08831.x
Culpepper A. S., Grey T. L., Vencill W. K., Kichler J. M., Webster T. M., Brown S. M., et al. (2006). Glyphosate-resistant palmer amaranth (Amaranthus palmeri) confirmed in Georgia. Weed Sci. 54 (4), 620–626. doi: 10.1614/WS-06-001R.1
De Dios V. R., Roy J., Ferrio J. P., Alday J. G., Landais D., Milcu A., et al. (2015). Processes driving nocturnal transpiration and implications for estimating land evapotranspiration. Sci. Rep. 5 (1), 1–8. doi: 10.1038/srep10975
Denmead O. T., Shaw R. H. (1962). Availability of soil water to plants as affected by soil moisture content and meteorological conditions 1. Agron. J. 54 (5), 385–390. doi: 10.2134/agronj1962.00021962005400050005x
Ehleringer J. (1983). Ecophysiology of Amaranthus palmeri, a sonoran desert summer annual. Oecologia 57 (1), 107–112. doi: 10.1007/BF00379568
Fricke W. (2019). Nighttime transpiration–favouring growth? Trends Plant Sci. 24 (4), 311–317. doi: 10.1016/j.tplants.2019.01.007
Gavlak R., Horneck D., Miller R. O., Kotuby-Amacher J. (2003). Soil, plant and water reference methods for the western region (Fort Collins, CO: WCC-103 Publication).
Gholipoor M., Sinclair T. R., Prasad P. V. (2012). Genotypic variation within sorghum for transpiration response to drying soil. Plant Soil 357 (1), 35–40. doi: 10.1007/s11104-012-1140-8
Gossett B. J., Murdock E. C., Toler J. E. (1992). Resistance of palmer amaranth (Amaranthus palmeri) to the dinitroaniline herbicides. Weed Technol. 6 (3), 587–591. doi: 10.1017/S0890037X00035843
Hanson B., Orloff S., Peters D. (2000). Monitoring soil moisture helps refine irrigation management. California Agric. 54 (3), 38–42. doi: 10.3733/ca.v054n03p38
Hendrickson A. H., Veihmeyer F. J. (1945). Permanent wilting percentages of soils obtained from field and laboratory trials. Plant Physiol. 20 (4), 517. doi: 10.1104/pp.20.4.517
Jhala A. J., Sandell L. D., Rana N., Kruger G. R., Knezevic S. Z. (2014). Confirmation and control of triazine and 4-hydroxyphenylpyruvate dioxygenase-inhibiting herbicide-resistant palmer amaranth (Amaranthus palmeri) in Nebraska. Weed Technol. 28 (1), 28–38. doi: 10.1614/WT-D-13-00090.1
Korres N. E., Norsworthy J. K., FitzSimons T., Roberts T. L., Oosterhuis D. M. (2017). Differential response of palmer amaranth (Amaranthus palmeri) gender to abiotic stress. Weed Sci. 65 (2), 213–227. doi: 10.1017/wsc.2016.34
Kumar V., Liu R., Boyer G., Stahlman P. W. (2019). Confirmation of 2, 4-d resistance and identification of multiple resistance in a Kansas palmer amaranth (Amaranthus palmeri) population. Pest Manage. Sci. 75 (11), 2925–2933. doi: 10.1002/ps.5400
Kumar V., Liu R., Stahlman P. W. (2020). Differential sensitivity of Kansas palmer amaranth populations to multiple herbicides. Agron. J. 112 (3), 2152–2163. doi: 10.1002/agj2.20178
Küpper A., Peter F., Zöllner P., Lorentz L., Tranel P. J., Beffa R., et al. (2018). Tembotrione detoxification in 4-hydroxyphenylpyruvate dioxygenase (HPPD) inhibitor-resistant palmer amaranth (Amaranthus palmeri s. wats.). Pest Manage. Sci. 74 (10), 2325–2334. doi: 10.1002/ps.4786
Massinga R. A., Currie R. S., Horak M. J., Boyer J. (2001). Interference of palmer amaranth in corn. Weed Sci. 49 (2), 202–208. doi: 10.1614/0043-1745(2001)049[0202:IOPAIC]2.0.CO;2
Massinga R. A., Currie R. S., Trooien T. P. (2003). Water use and light interception under palmer amaranth (Amaranthus palmeri) and corn competition. Weed Sci. 51 (4), 523–531. doi: 10.1614/0043-1745(2003)051[0523:WUALIU]2.0.CO;2
Matzrafi M., Osipitan O. A., Ohadi S., Mesgaran M. B. (2021). Under pressure: maternal effects promote drought tolerance in progeny seed of palmer amaranth (Amaranthus palmeri). Weed Sci. 69 (1), 31–38. doi: 10.1017/wsc.2020.75
Minasny B., McBratney A. (2003). Integral energy as a measure of soil-water availability. Plant Soil 249 (2), 253–262. doi: 10.1023/A:1022825732324
Morgan G. D., Baumann P. A., Chandler J. M. (2001). Competitive impact of palmer amaranth (Amaranthus palmeri) on cotton (Gossypium hirsutum) development and yield. Weed Technol. 15 (3), 408–412. doi: 10.1614/0890-037X(2001)015[0408:CIOPAA]2.0.CO;2
Nakka S., Godar A. S., Wani P. S., Thompson C. R., Peterson D. E., Roelofs J., et al. (2017). Physiological and molecular characterization of hydroxyphenylpyruvate dioxygenase (HPPD)-inhibitor resistance in Palmer amaranth (Amaranthus palmeri S. Wats.). Frontiers in Plant Science. 8, 555. doi: 10.3389/fpls.2017.00555
Passioura J. B. (2006). The perils of pot experiments. Funct. Plant Biol. 33 (12), 1075–1079. doi: 10.1071/FP06223
Patrignani A., Knapp M., Redmond C., Santos E. (2020). Technical overview of the Kansas mesonet. J. Atmospheric Oceanic Technol. 37 (12), 2167–2183. doi: 10.1175/JTECH-D-19-0214.1
Ray J. D., Gesch R. W., Sinclair T. R., Hartwell Allen L. (2002). The effect of vapor pressure deficit on maize transpiration response to a drying soil. Plant Soil 239 (1), 113–121. doi: 10.1023/A:1014947422468
Ray J. D., Sinclair T. R. (1997). Stomatal closure of maize hybrids in response to drying soil. Crop Sci. 37 (3), 803–807. doi: 10.2135/cropsci1997.0011183X003700030018x
Schaap M. G., Leij F. J., Van Genuchten M. T. (2001). Rosetta: A computer program for estimating soil hydraulic parameters with hierarchical pedotransfer functions. J. hydrol 251 (3-4), 163–176. doi: 10.1016/S0022-1694(01)00466-8
Schmidt J. J., Blankenship E. E., Lindquist J. L. (2011). Corn and velvetleaf (Abutilon theophrasti) transpiration in response to drying soil. Weed Sci. 59 (1), 50–54. doi: 10.1614/WS-D-10-00078.1
Silva A. P. D., Imhoff S., Kay B. (2004). Plant response to mechanical resistance and air-filled porosity of soils under conventional and no-tillage system. Scientia Agricola 61, 451–456. doi: 10.1590/S0103-90162004000400016
Sinclair T. R., Hammer G. L., Van Oosterom E. J. (2005). Potential yield and water-use efficiency benefits in sorghum from limited maximum transpiration rate. Funct. Plant Biol. 32 (10), 945–952. doi: 10.1071/FP05047
Sinclair T. R., Tanner C. B., Bennett J. M. (1984). Water-use efficiency in crop production. Bioscience 34 (1), 36–40. doi: 10.2307/1309424
Sprague C. L., Stoller E. W., Wax L. M., Horak M. J. (1997). Palmer amaranth (Amaranthus palmeri) and common waterhemp (Amaranthus rudis) resistance to selected ALS-inhibiting herbicides. Weed Sci. 45 (2), 192–197. doi: 10.1017/S0043174500092705
Tanner C. B., Sinclair T. R. (1983). Efficient water use in crop production: Research or re-search? Limitations to efficient Water Use Crop production, 1–27. doi: 10.2134/1983.limitationstoefficientwateruse.c1
Taylor S. A. (1965). Managing irrigation water on the farm. Trans. ASAE 8 (3), 433–0436. doi: 10.13031/2013.40540
Van Genuchten M. T. (1980). A closed-form equation for predicting the hydraulic conductivity of unsaturated soils. Soil Sci. Soc. America J. 44 (5), 892–898. doi: 10.2136/sssaj1980.03615995004400050002x
van Lier Q. D. J. (2017). Field capacity, a valid upper limit of crop available water? Agric. Water Manage. 193, 214–220. doi: 10.1016/j.agwat.2017.08.017
Van Wychen L.. (2016). 2015 Baseline Survey of the Most Common and Troublesome Weeds in the United States and Canada. Weed Science Society of America National Weed Survey Dataset. Available: https://wssa.net/wp-content/uploads/2015-Weed-Survey_Baseline.xlsx.
Virtanen P., Gommers R., Oliphant T. E., Haberland M., Reddy T., Cournapeau D., et al. (2020). SciPy 1.0: fundamental algorithms for scientific computing in Python. Nat. Methods 17 (3), 261–272. doi: 10.1038/s41592-019-0686-2
Ward S. M., Webster T. M., Steckel L. E. (2013). Palmer amaranth (Amaranthus palmeri): a review. Weed Technol. 27 (1), 12–27. doi: 10.1614/WT-D-12-00113.1
Keywords: soil water deficit, palmer amaranth, soil moisture, transpiration, soil water stress
Citation: Cominelli S and Patrignani A (2022) Transpiration response of palmer amaranth (Amaranthus palmeri) to drying soil in greenhouse conditions. Front. Agron. 4:1018251. doi: 10.3389/fagro.2022.1018251
Received: 12 August 2022; Accepted: 27 September 2022;
Published: 14 October 2022.
Edited by:
Sowbiya Muneer, VIT University, IndiaReviewed by:
Parthasarathi Theivasigamani, Vellore Institute of Technology (VIT), IndiaCopyright © 2022 Cominelli and Patrignani. This is an open-access article distributed under the terms of the Creative Commons Attribution License (CC BY). The use, distribution or reproduction in other forums is permitted, provided the original author(s) and the copyright owner(s) are credited and that the original publication in this journal is cited, in accordance with accepted academic practice. No use, distribution or reproduction is permitted which does not comply with these terms.
*Correspondence: Andres Patrignani, YW5kcmVzcGF0cmlnbmFuaUBrc3UuZWR1
†ORCID: Sofia Cominelli, orcid.org/0000-0002-0075-1512
Andres Patrignani, orcid.org/0000-0001-5955-5877
Disclaimer: All claims expressed in this article are solely those of the authors and do not necessarily represent those of their affiliated organizations, or those of the publisher, the editors and the reviewers. Any product that may be evaluated in this article or claim that may be made by its manufacturer is not guaranteed or endorsed by the publisher.
Research integrity at Frontiers
Learn more about the work of our research integrity team to safeguard the quality of each article we publish.