- 1Instituto de Investigaciones para la Industria Química (INIQUI), Universidad Nacional de Salta (UNSa), Consejo Nacional de Investigaciones Científicas y Técnicas (CONICET), Salta, Argentina
- 2Facultad de Ciencias Naturales, Universidad Nacional de Salta (UNSa), Salta, Argentina
- 3Facultad de Ingeniería, Universidad Nacional de Salta (UNSa), Salta, Argentina
- 4Singapore Centre for Environmental Life Sciences Engineering (SCELSE), School of Biological Sciences, Nanyang Technological University (NTU), Singapore, Singapore
The rhizosphere and microbiome of halotolerant plants could be crucial for alleviating salinity stress during plant growth. The aims of this work were (1) to isolate bacteria from rhizosphere and bulk soil samples from the Salar del Hombre Muerto (Catamarca, Argentina), (2) to characterize different plant growth-promoting (PGP) activities produced by these bacterial isolates, and (3) to evaluate their effect on the initial growth of chia (Salvia hispanica L.) under saline stress. A total of 667 microorganisms were isolated, using different culture media with NaCl, and their abilities for nitrogen fixation, phosphate solubilization, siderophores production, and indole-3-acetic acid production were evaluated. Thirteen strains were selected for showing all the tested PGP activities; they belonged to the genera Kushneria, Halomonass, Pseudomonas, Planomicrobium, and Pseudarthrobacter. The strains Kushneria sp. and Halomonas sp. showed the highest salinity tolerance (from 50 to 2,000 mM NaCl) and biomass and biofilm production. Chia seeds were treated with six of the first 13 selected strains to evaluate their plant growth-promoting effect under saline stress (without and with 50 and 100 mM NaCl). Halomonas sp. 3R.12 and Kushneria sp. T3.7 produced heavier seedlings with a balanced shoot/root length ratio, while Pseudomonas sp. AN23 showed the best effect upon chia seedlings, with a morphological response similar to non-stressed seedlings. On the other hand, seedlings displayed no responses when inoculated with Planomicrobium sp. 3S.31 and Pseudarthrobacter sp. ER25. This study contributes to the knowledge on microorganisms from hypersaline environments as plant growth promoters for their use in the production of salt-sensitive crops, among other potential uses.
Introduction
Agricultural production more than tripled between 1960 and 2015, partly due to the Green Revolution (productivity-enhancing technologies) and, also, to a significant expansion in the use of land, water, and other natural resources for agriculture (FAO, 2018). Demographers estimate that by 2050 the population of the world will reach 9.1 billion people (Alexandratos and Bruinsma, 2012); therefore, food production must increase through strategies such as yield optimization, cropping intensification, or arable land expansion (Food and Agricultural Organization, 2009).
Estimates point to a global 33% of moderately to highly degraded farmland (Food and Agriculture Organization of the United Nations, 2017). Much of the available surface area in the world is not suitable land for agriculture. Thus, bringing additional land into agricultural production could carry heavy environmental, social, and economic costs (Food and Agriculture Organization of the United Nations, and I. T. P. on S, 2015).
Salinization is one of the major causes of soil degradation and is becoming a significant problem worldwide (Srivastava et al., 2019). Apart from the scarce information on the extent and characteristics of salt-affected soils, salinity, and sodicity (a high proportion of sodium ions relative to other cations in the soil) are rapidly increasing in many irrigated and non-irrigated lands (Ivushkin et al., 2019).
Many physical, chemical, and biological strategies to address the problems of soil degradation contain the threat of salinity, such as salts leaching, chemical amelioration, organic amendments application, and planting of salt-tolerant species or varieties (Shankar and Evelin, 2019). Another biological approach to reclamation of salt-affected soils and recovery of their productivity is the use of biofertilizers based on halotolerant or halophile bacteria with plant growth-promoting (PGP) properties (Srivastava et al., 2019; Romano-Armada et al., 2020).
The natural ability of halophile microorganisms to cope with harsh environmental conditions has been attributed to their high-genetic diversity and physiological adaptations (Edbeib et al., 2016). They hold the biotechnological potential to produce secondary metabolites antibiotics, antitumor agents, and enzymes, and to favor beneficial plant-microbe interactions through plant growth-promoting features (Jorquera et al., 2016). Many authors have described the use of bacteria isolated from hypersaline environments, particularly from the rhizosphere of halotolerant plants located in saline deserts, hypersaline soils, and salt-affected areas, as growth promoters in traditional crops (Sáenz-Mata et al., 2016; Mukhtar et al., 2019; Wu et al., 2019; Kumawat et al., 2021). Thus, rhizosphere microbiomes play an essential role in the survival of plants in nature, and growth-promoting rhizobacteria isolated from salt-tolerant plants could be crucial for alleviating soil salinity stress during plant growth (Banerjee et al., 2019).
In this sense, biofertilizers based on halophilic PGP-bacteria (PGPB) constitute an as yet largely unexplored sustainable alternative to the reuse of salinized agricultural soils. Such is the case of chia (Salvia hispanica L.), an emergent crop native to Mexico's central valleys and to northern Guatemala, which played an essential role in the diet of pre-Columbian societies. Also, chia has undeniable importance as a source of high-quality nutrients (Muñoz et al., 2013).
Chia seeds have been rediscovered because of their vast potential in several industries due to their nutritional content (Orona-Tamayo et al., 2017). Moreover, the cropping area of chia has increased in México, Bolivia, Argentina, Paraguay, Ecuador, and Guatemala in recent years (Ixtaina et al., 2008). Consequently, chia is an interesting alternative to the productive diversification of crops, particularly in Argentina's northwest (Pérez Brandán et al., 2020). However, several authors have reported that chia plants have a low tolerance to salinity. Values of saline stress such as 60 mM NaCl inhibited germination and decreased the viability and vigor of seeds, plant height, number of leaves and flowers, a leaf area, and plant weight (Jardim Rosa et al., 2014; Stefanello et al., 2015; Raimondi et al., 2017). Therefore, increased knowledge about the interaction of this species with halophilic PGPB that could enhance saline stress tolerance would be an important step in encouraging increased production of chia in areas with salt-affected soils.
The aims of this work were to isolate bacteria from the rhizosphere of native plants and soil samples from the Salar del Hombre Muerto in Catamarca (in the northwest of Argentina), to characterize various plant growth-promoting activities from these isolated bacteria, and to evaluate their effect on the germination and initial growth of chia (Salvia hispanica L.) under saline stress. To the best of our knowledge, this is the first study to assess the performance of chia plants under saline stress when treated with halophile PGPB isolated from the rhizosphere of halophyte plants and extreme environments.
Materials and Methods
Sampling Sites and Sample Collection
A total of six samples of bulk soil (n = 3) and rhizosphere (n = 3) were collected from a lithium mining deposit in operation in the Salar del Hombre Muerto (Catamarca, Argentina) from two sites in a salt flat: site one (S1) (25°28′49.38″S, 67°6′22.74″W) and Site two (S2) (25°29′4.62″S, 67°6′19.5″W) (Figure 1A). Roots with rhizosphere were collected from three halophyte plants from different families: Adesmia horrida (Fabaceae) (Figure 1B) and Senecio punae (Asteraceae) (Figure 1C), which were in S1, and Pappostipa frigida (Poaceae) (Figure 1D), which was in S2. Bulk soil samples were collected from the areas surrounding each plant. The samples were stored in black plastic bags at 4°C and taken to the laboratory for microbial isolation.
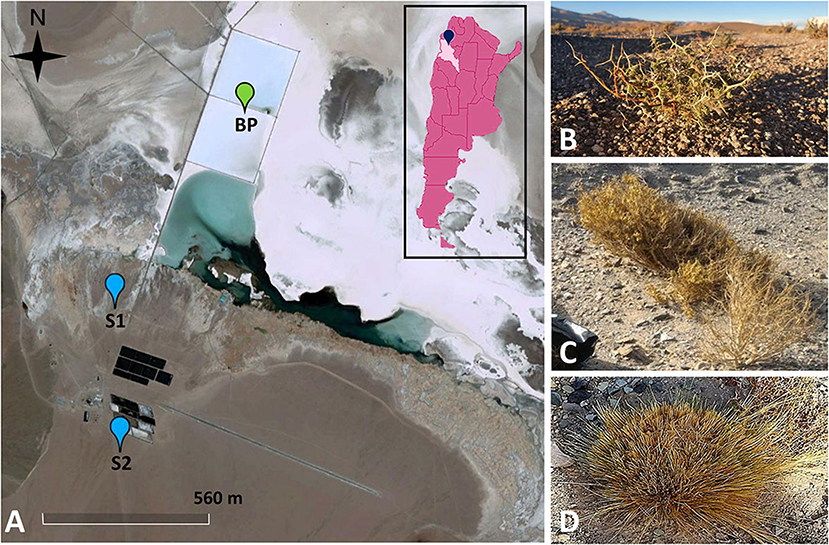
Figure 1. Sampling points located in a lithium mining deposit in operation in the salt flat Salar del Hombre Muerto, Catamarca Province, Argentina (A). Site one (S1) (25°28′49.38″S, 67°6′22.74″W), Site two (S2) (25°29′4.62″S, 67°6′19.5″W), lithium brine concentration pools (BP). Samples were collected from soil and roots of native vegetation from the families Fabaceae (B) and Asteraceae (C), located in S1, and Poaceae (D), located in S2.
Physicochemical Analyses of Bulk Soil Samples
The following variables were determined for all the bulk soil samples in the Laboratory of Soil, Water and Fertilizers (LABsaf) of the Instituto Nacional de Tecnología Agropecuaria (INTA, Cerrillos, Salta, Argentina): texture (according to the percentage of sand, silt, and clay), available water capacity, pH in the soil solution (pHsw) (1:2.5 soil to water ratio) and pH in the saturated paste (pHsp), electric conductivity (EC), exchangeable sodium, potassium, calcium, and magnesium, organic carbon, organic matter, total nitrogen, phosphorous availability, sodium adsorption ratio, exchangeable sodium percentage, and exchangeable chloride. The methods used for soil characterization were the Bouyoucos method for texture, micro Walkley-Black technique for organic matter, the micro-Kjeldahl method for total nitrogen, and the Bray–Kurtz No. I (modified) method for “extractable” phosphorus. Exchange cations were extracted with 1.0 N ammonium acetate (pH 7.0).
Isolation of Microorganisms
Two different microbial isolations were carried out. For the rhizosphere microbial isolation from each plant, 10 g of roots with adhered soil were placed in Erlenmeyer flasks, containing 90 ml of a saline solution (45 ml of sterile distilled water, 45 ml of brine from the salt flat), with a final Na concentration of 2.13 M (Martínez et al., 2019). For bulk soil microbial isolation, 10 g of each soil sample were suspended in 90 ml of the aforementioned salty solution in the Erlenmeyer flasks. For the microbial extraction, the flasks containing roots and bulk soil were stirred for 30 min at 200 rpm at room temperature, and then decanted for 1 h. Afterward, 100 μl from each Erlenmeyer were plated on four different culture media: TSA 100 (Tryptic Soy Agar, Britania® supplemented with salt to a final concentration of NaCl 1.75 M), PCA 25 (Plate Count Agar, Britania® supplemented with brine from the salt flat to a final concentration of NaCl200 mM), SEA (soil extract agar) made with bulk soil from the salt flat (a suspension of 500 g of soil in 1 L of distilled water was stirred during 30 min, decanted for 1 h, and filtered with regular filter paper. Next, agar-agar was added to the supernatant up to 2% as a gelling agent, and, finally, the medium was sterilized), and ANF (Ashby nitrogen-free agar) (Kryuchkova et al., 2014) supplemented with 1 and 2 M of NaCl. Also, root cuttings from each plant were placed in tubes, containing a semisolid NFb medium (Döbereiner et al., 1976), supplemented with salt (final concentration of NaCl 1 M) to isolate nitrogen-fixing bacteria. Cultures were incubated at 30°C until growth was observed.
Stepwise Screening of Plant Growth-Promoting Abilities
The isolated microorganisms were evaluated for different in vitro PGP (plant growth-promoting) traits, both without salt and in the presence of 1 M NaCl. First, the strains isolated in PCA 25, TSA 100, and SEA were assessed for a biological N2 fixation. Then, those with this capacity and those isolated in nitrogen-free media (ANF and NFb) were evaluated for their ability to solubilize phosphates. Strains that had the activity in any salinity condition were then evaluated to test their siderophore and Indole-3-acetic acid (IAA) production. In all cases, experiments were conducted in duplicate, using fresh bacterial inoculum (24-h growth at 30°C and 200 rpm).
Biological Nitrogen Fixation (BNF)
Bacteria were cultured at 30°C for 7 days in two different nitrogen-free media (NFb and ANF), with the addition of NaCl to a final concentration of 1 M. Positive activity was revealed by a veil formation, bacterial growth, and the change of color from green to blue in tubes with 5 ml of semisolid NFb medium (Döbereiner et al., 1976) and by colony growth in plates with 20 ml of ANF agar (Kryuchkova et al., 2014).
Phosphates Solubilization
A qualitative assay was performed, plating the isolates in two culture media with different phosphate sources: NBRIP agar (Shekhar Nautiyal, 1999), containing tricalcium phosphate (Ca3(PO4)2) and Muromtsev agar (Kryuchkova et al., 2014), containing dibasic sodium phosphate (Na2HPO4). Response to salinity was evaluated at 1 M NaCl. After 7 days of incubation at 30°C, the formation of clear halos surrounding the bacterial colonies indicated positive activity.
Siderophores Production
Bacterial strains were spiked on Chrome azurol-S (CAS) agar plates (without salts and with 1 M NaCl). Plates were incubated for 5 days at 30°C. The formation of orange-yellowish halos surrounding the bacterial colonies indicated positive siderophores production. CAS agar was prepared according to the protocol described by Schwyn and Neilands (Schwyn and Neilands, 1987) with slight modifications, replacing cetyl-trimethyl-ammonium bromide (CTAB) with cetrimide (Anedra®) and using plate count agar with brine (PCA25) as the base medium for CAS agar plates.
Indole-3-Acetic Acid Production
Bacteria were cultured in nutrient broth supplemented with salts (NB-salt) (2.5% v/v of salt solution: NaCl 98 g L−1, KCl 8.3 g L−1, MgSO4 3.3 g L−1) without and with the addition of tryptophan (0.5 g L−1) in an orbital incubator (ES-20, Biosan) at 150 rpm during 5 days at 30°C. The production of IAA was determined by colorimetry (Glickmann and Dessaux, 1995). For color development, 1 ml of reagent R1 (12 g L−1 of FeCl3 in 7.9 M H2SO4) (Pilet and Chollet, 1970) was added to 1 ml of culture supernatant, then mixed and left in the dark for 30 min at room temperature. Absorbance was measured in a spectrophotometer (model 752, BIOTRAZA) at 530 nm, and values were referred to an IAA calibration curve.
Bacterial Identification
To identify the isolated bacteria, genomic DNA was extracted as described in Martínez et al. (2019) and Pospiech and Neumann (1995). For each sample, genes encoding 16S rRNA were amplified by conventional PCR, using universal primers 63F (5′-CAGGCCTAACACATGCAAGTC-3′) and 1389R (5′-GGGCGGWGTGTACAAGGC-3′) (Lowe et al., 2011). PCR was conducted as described by Martínez et al. (2019) (GeneAmp 9700, Applied Biosystems), and sequencing of the PCR products was carried out by Macrogen (Seoul, South Korea), following Sanger sequencing protocol. The strains of interest were partially identified (genus and species) with the obtained 16S rRNA gene sequences through a BLAST (Basic Local Alignment Search Tool) search on the NIH/NCBI bacterial database. The nucleotide sequences were submitted to the GenBank® database [National Center for Biotechnology Information (NCBI)]1.
Growth Curves and Biofilms Production
Cultures were grown in 96-well microplates, using nutrient broth supplemented with NaCl to reach 12 different concentrations: 0, 10, 50, 100, 250, 500, 750, 1,000, 1,250, 1,500, 1,750, and 2,000 mM. Each well was filled with 200 μL of fresh culture (cells grown for 24 h in nutrient broth at 30°C, washed, and resuspended in fresh nutrient broth; optical density adjusted to 0.1 at 600 nm). Plates were incubated at 30°C and 150 rpm for 5 days. Optical density at 600 nm was measured in a microplate reader spectrophotometer (Thermo Scientific® Multiskan FC) every hour until the stationary phase was reached and then every 24 h.
After growth was completed, biofilm production was quantified according to a previously described protocol (Merritt et al., 2011). Briefly, after removing the supernatant, the plates were stained with 250 μl of 0.1% (w/v) crystal violet. The dye was solubilized with 300 μl of acetic acid, 30% (v/v), and absorbance was measured in a microplate reader spectrophotometer (Thermo Scientific® Multiskan FC) at 595 nm. The absorbance values were directly proportional to the amount of biofilm produced.
Effect of Bacteria in Chia Seedlings
After screening, the bacteria showing a greater number of positive PGP activities were selected and inoculated in chia seeds to evaluate their growth-promoting effect.
The culture media used for seed germination and plant growth was half-strength Murashige–Skoog medium (MS/2) (Murashige and Skoog, 1962) with sugar (2 g L−1 sucrose), gelled with 0.8% plant agar (Duchefa Biochemie). Six bacterial strains were evaluated, accounting for seven treatments, including the Control (NB-Salt without bacteria). Before seed treatment, bacterial strains were grown in NB-Salt at 30°C in an orbital shaker-incubator (model ES-20, Biosan) at 200 rpm for 24 h. Then, culture OD600 was adjusted to a final value of 0.6 to attain a bacterial suspension of ~108–109 CFU/ml cell density for the seed bacterization.
The salinity treatments were prepared by adding NaCl to the MS/2 agar to final concentrations of 50 and 100 mM, achieving EC values of ~7.5 and 12.5 dS/m, respectively. Control treatment was prepared with the MS/2 medium without salt addition (EC = 2.82 dS/m). Chia seeds were surface sterilized, using vapor-phase sterilization. Seeds were placed in open Petri dishes inside a 10-L desiccator. Next to the seeds container, a 250-ml glass beaker with 100-ml household bleach (NaClO) was added with 3 ml of hydrochloric acid (HCl). The desiccator was closed and sealed hermetically, allowing the chlorine gas to act from 4 to 6 h (Romano Armada et al., 2019).
Ten seeds were plated in each MS/2 plate, each of which was inoculated with 20 μl of bacterial culture. The plates were placed in the dark at 4°C for 24 h for seed stratification. Afterward, the plates were placed vertically in a growth chamber with a photoperiod of 8-h light: 16-h dark and a constant temperature of 23°C. After 7 days, the germination percentage was registered, and the plant total length (from the tip of the root until the cotyledons) and primary root length (from the base of the hypocotyl to the root tip) were measured for each plant. Also, the dry weight of the plants was measured for each plate replicate.
Statistical Analysis
Treatments were arranged in a growth chamber in a completely randomized design with three replicates. Image processing and data collection were done with the image processing package FIJI (Schindelin et al., 2012). All of the observed data were normally distributed and had homogeneous variance errors. Results were compared by one-way ANOVA with Tukey's post-test to correct for multiple comparisons. Treatment effects with an alpha (α) level of 0.05 or lower were considered significant. Also, correlation analysis was used to evaluate the association between growth variables: (shoot length/root length) against (dry weight × total length) to assess the effect of each treatment on the morphological response of the seedlings. Statistical data processing was performed with InfoStat (Di Rienzo et al., 2008).
Results
Physicochemical Characterization of Soil Samples
Soils in both sampling sites were mainly composed of sand (Table 1). The pH in the soil solution (pHsw) was above 8 at both sites, while pH in the saturated paste (pHsp) was lower for S1 (7.8). The EC was high (22.6 dS/m) in both sites. However, other salinity-related variables such as exchangeable sodium percentage and a sodium adsorption ratio showed higher values in S2. Organic matter and nitrogen content were low at both sites, with the same value of carbon to nitrogen ratio (10). Soluble potassium and chloride showed similar values in both sites. Soluble sodium was slightly higher in S2; conversely, soluble calcium and magnesium were higher in S1 (Table 1).
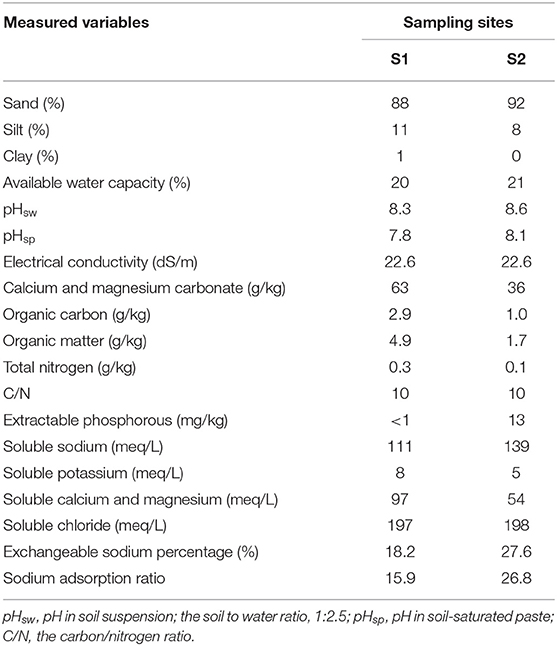
Table 1. Physicochemical analysis of soil samples in both sampling sites: Site 1 (S1) and Site 2 (S2) in the Salar del Hombre Muerto.
Isolation of Bacteria
A total of 667 microorganisms were isolated from culturing the samples of the rhizosphere and bulk soil surrounding plants from the genera Fabaceae (Adesmia horrida), Asteraceae (Senecio punae), and Poaceae (Pappostipa frigida). Most microorganisms were isolated from bulk soil samples (456 isolates), while a smaller number came from rhizosphere and root samples (211 isolates). A total of 29.5% (197 isolates) were recovered from Fabaceae-associated samples, 26.0% (173 isolates) from Asteraceae-associated samples, and 44.5% (297 isolates) from Poaceae-associated samples (Figure 2A). When considering the culture media used, most microorganisms were isolated from PCA 25 (41.7%), followed by ANF1 M and 2 M (23.8%), SEA (17.4%), and TSA 100 (14.2%). The NFb1 M medium allowed retrieval of the lowest number of isolates (2.8%) (Figure 2A).
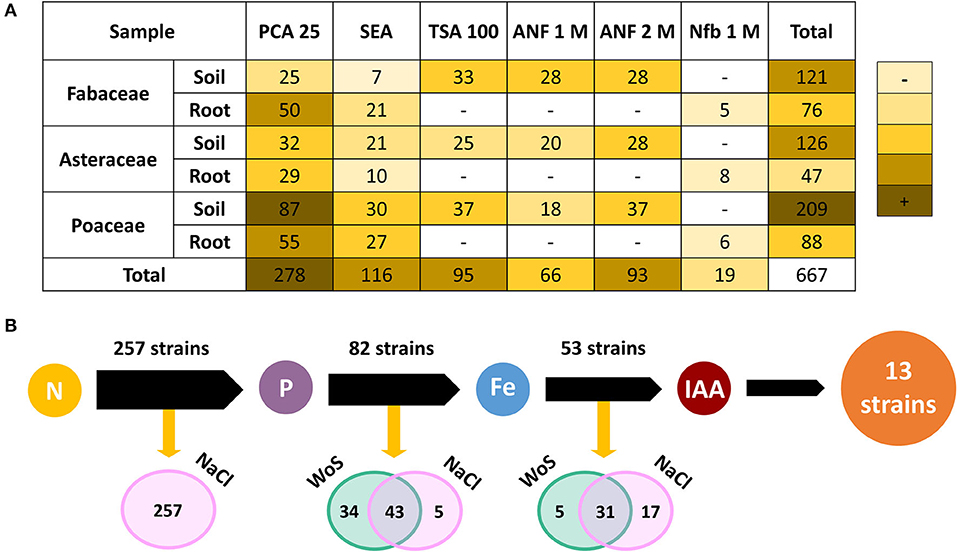
Figure 2. Microbial isolation from samples collected from two sampling sites in the Salar del Hombre Muerto, Catamarca (Argentina). (A) A heat map of the number of bacterial isolates from samples that correspond to the surrounding bulk soil and rhizosphere of three plants from different genera: Fabaceae, Asteraceae, and Poaceae. The microbial isolation varied according to the culture media used: plate count agar (PCA 25), soil extract agar (SEA), tryptic soy agar (TSA 100), Ashby nitrogen-free agar (ANF) (1 and 2 M NaCl), and a semisolid NFb medium for nitrogen-fixing bacteria, with 1 M NaCl. (B) Stepwise screening of plant growth-promoting (PGP) properties on the isolated bacteria: a nitrogen fixation (N), phosphates solubilization (P), iron chelation (Fe), and indole-3-acetic acid production (IAA) without salt (WoS) and with 1 M of NaCl (NaCl). All isolated strains were first evaluated for a nitrogen fixation; only positive strains for each activity in any salinity condition were evaluated on the next PGP activity indicated by the black arrows.
Strain Identification and PGP Traits
The stepwise screening of PGP properties on the isolated bacteria showed that 257 strains were able to fix N2 in the presence of 1 M NaCl, and 82 of them solubilized under different salinity conditions. About half of the strains (43) were able to solubilize regardless of the NaCl concentration in the media, while 34 showed the activity only in the absence of NaCl, and five did so exclusively in the presence of 1 M NaCl. Out of the 82 strains that were able to fix nitrogen and solubilize phosphates, 53 were able to produce siderophores in the presence or absence of NaCl, similarly to the case of phosphates solubilization (Figure 2B). However, five strains produced siderophores in the absence of NaCl, while 17 required the salt. Finally, all siderophores-positive strains were evaluated for their IAA production.
Only 13 strains produced siderophores in any salinity condition (Figure 2B). Those 13 strains, which showed all the tested PGP activities, were partially identified by 16S rRNA. Sequencing showed that isolates belonged to five bacterial genera: Kushneria, Halomonas, and Pseudomonas were the most common, and Planomicrobium and Pseudarthrobacter, with only one isolate from each (Table 2).
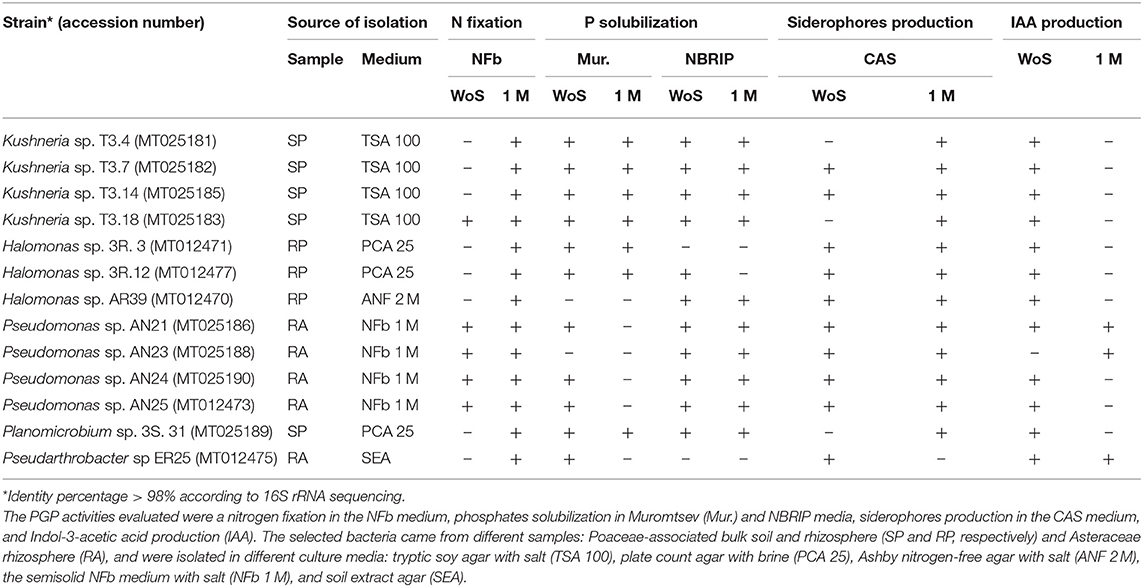
Table 2. Molecular identification by 16S rRNA (with GenBank accession number) and in vitro plant growth-promoting (PGP) properties of bacterial isolates under different saline conditions: without salt (WoS) and with 1 M NaCl (1 M).
All the selected strains could fix nitrogen at 1 M NaCl, but only five displayed the activity without salt present. Five strains showed phosphate solubilization activity in both culture media and under both salinity conditions tested. While Halomonas sp. 3R.3 and Pseudarthrobacter sp. ER25 only solubilized phosphates in Muromtsev agar, Halomonas sp. AR39 and Pseudomonas sp. AN23 could only solubilize phosphates when grown in the NBRIP medium. All 13 strains (except Pseudarthrobacter sp. ER25) showed siderophores production activity in the presence of 1 M NaCl. Moreover, all Halomonas and Pseudomonas strains also displayed activity without salt, unlike strains T3.4, T3.14, and T3.18 from the genus Kushneria, and Planomicrobium sp. 3S.31. Considering IAA production, all strains showed activity without salts, except for Pseudomonas sp. AN23, while only three strains, Pseudarthrobacter sp. ER25, Pseudomonas sp. AN21, and Pseudomonas sp. AN23 produced IAA under the saline condition (Table 2).
Growth and Biofilm Production Under Different Saline Conditions
The strains Kushneria sp. T3.18 and Halomonas sp. 3R.3, 3R.12, and AR39 showed the highest biomass production and salinity tolerance (Figures 3D,G), growing in a wide range of NaCl concentrations, from 50 mM up to 2,000 mM. Even though the strains Kushneria sp. T3.4 (Figure 3A), Kushneria sp. T3.7 (Figure 3B), and Pseudomonas sp. AN21 (Figure 3H) showed overall low OD values, they maintained a relatively constant biomass production throughout all the tested salinity levels in the higher saline conditions. The strain Pseudarthrobacter sp. ER25 (Figure 3M) showed a higher biomass production in the lowest salinity concentrations (from no salt to 100 mM NaCl) (Figure 3).
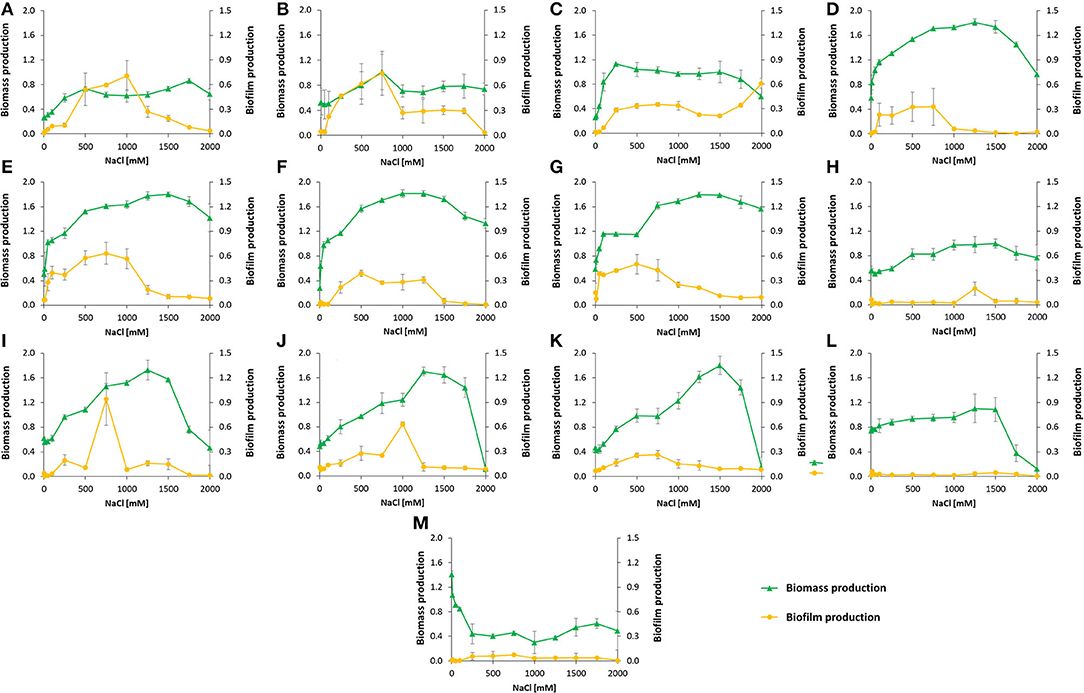
Figure 3. Growth and biofilm production of 13 strains isolated from the Salar del Hombre Muerto: (A) Kushneria sp. T3.4, (B) Kushneria sp. T3.7, (C) Kushneria sp. T3.14, (D) Kushneria sp. T3.18, (E) Halomonas sp. 3R.3, (F) Halomonas sp. 3R.12, (G) Halomonas sp. AR39, (H) Pseudomonas sp. AN21, (I) Pseudomonas sp. AN23, (J) Pseudomonas sp. AN24, (K) Pseudomonas sp. AN25, (L) Planomicrobium sp. 3S.31, and (M) Pseudarthrobacter sp. ER25, cultured in nutrient broth with different NaCl concentrations (mM): 0, 10, 50, 100, 250, 500, 750, 1,000, 1,250, 1,500, 1,750, and 2,000, for 5 days at 30°C and 150 rpm. Each point marks the mean of maximum absorbance values measured at 600 nm for biomass production and 595 nm for biofilm production in each NaCl concentration (n = 3), and error bars the standard deviation.
Strains Kushneria sp. T3.4, Kushneria sp. T3.7, Halomonas sp. 3R.3, and Halomonas sp. AR39 were the best producers of biofilm in saline concentrations below ~1,000 mM NaCl. Pseudomonas sp. AN21, Planomicrobium sp. 3S.31, and Pseudarthrobacter sp. ER25 did not produce biofilm under almost all of the tested saline conditions (Figure 3).
Selection of Strains for Further Studies With Chia
Taking into account the PGP activity screening and the biomass and biofilm production at different saline conditions, six strains were selected to evaluate their effect upon chia: Kushneria sp. T3.7, Halomonas sp. 3R.12, Pseudomonas sp. AN21, Pseudomonas sp. AN23, Planomicrobium sp. 3S.31, and Pseudarthrobacter sp. ER25.
The strains Planomicrobium sp. 3S.31 and Pseudarthrobacter sp. ER25 were the only ones isolated within those genera while Kushneria sp. T3.7 showed a combination of low sensitivity to salinity regarding growth and lack of stress response to saline conditions (lower biofilm production at higher NaCl concentrations). Halomonas sp. 3R.12 was chosen because of its in vitro PGP activities performance. Finally, Pseudomonas sp. AN21 showed more PGP activities and different behaviors from the other three strains, with steady growth values at all saline concentrations; Pseudomonas sp. AN23 showed a high biomass production in a wide range of salinity concentrations and was the strain that produced IAA only under high NaCl concentrations.
Bacterial Effect Upon Chia Seedlings
All bacterial treatments showed high percentages of germination, near 100%, except for the seeds inoculated with Pseudarthrobacter sp. ER25, which showed lower values (85%).
There was a significant decline in shoot length of the uninoculated controls as the saline concentration in the growth media increased (Figure 4).
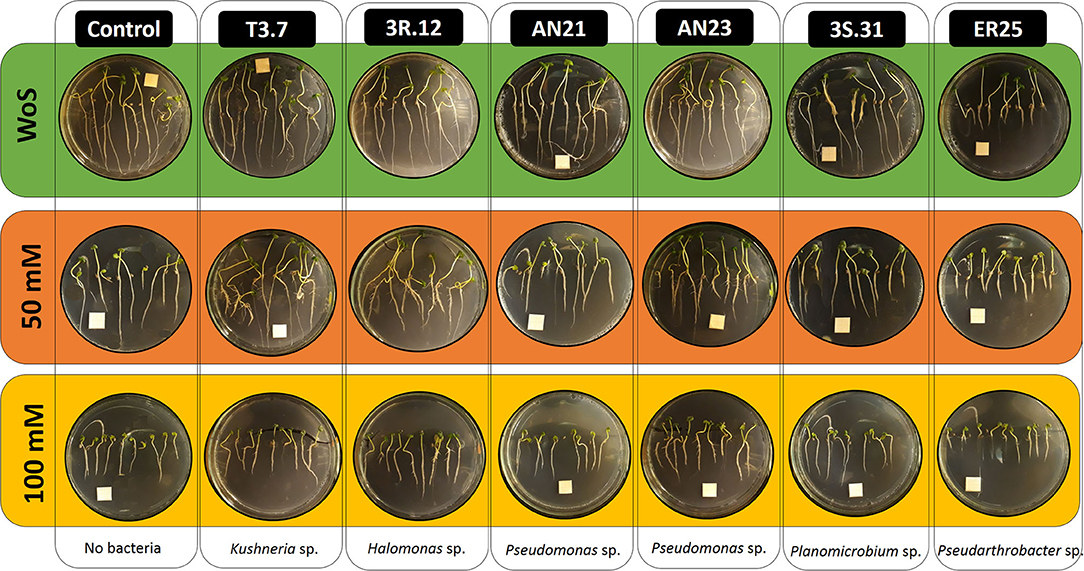
Figure 4. Photographs of seedlings grown vertically on agar plates containing an MS/2 medium 7 days after seed plating. White squares in the Petri dishes are 1 cm2.
There were no effects of bacterial inoculation in the absence of salt (WoS). However, under saline stress, Halomonas sp. 3R.12 and Kushneria sp. T3.7 were able to compensate for the saline effect of 50-mM NaCl (both strains showed no significant differences between the conditions WS and 50-mM NaCl) (Figures 4, 5). Seedlings inoculated with Kushneria sp. T3.7 grew significantly longer shoots at 50 and 100 mM NaCl than the rest of the treatments, with increments of 37.9 and 93.0% when compared with their respective controls. Halomonas sp. 3R.12 (18.7 and 45.9%, respectively) and Pseudomonas sp. AN23 (14.5 and 69.0%, respectively) also showed significantly increased growth compared with the controls at 50 and 100 mM NaCl (Figures 4, 5). Strains Planomicrobium sp. 3S.31, Pseudomonas sp. AN21, and Pseudarthrobacter sp. ER25 showed significantly shorter shoots than the controls with 50 mM NaCl, but this effect was not shown at 100 mM NaCl (Figure 4).
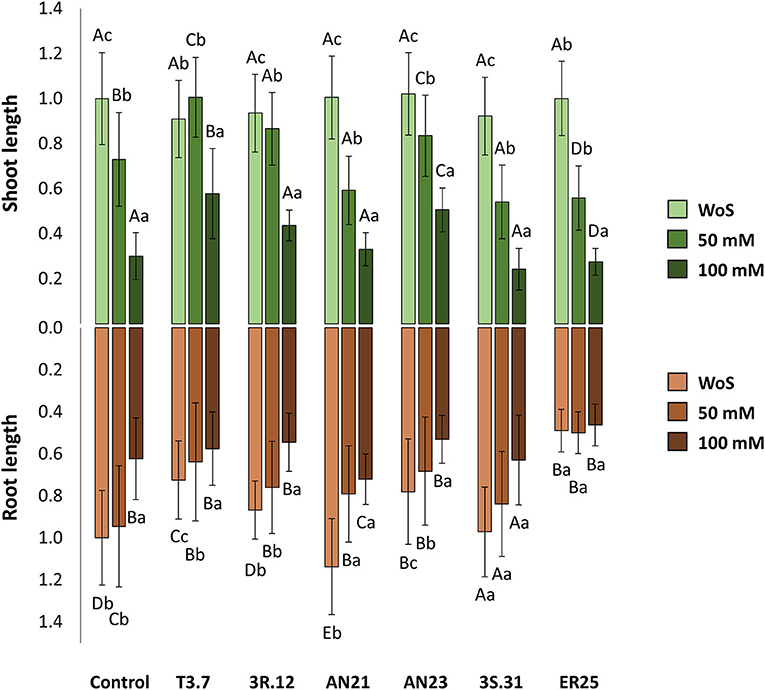
Figure 5. Root and shoot length of 7-day old chia (Salvia hispanica L.), seedlings grown on MS/2 agar medium, under different saline conditions: without salt (WoS), with 50 and 100 mM NaCl. The seedlings were subjected to treatment with different bacterial strains: Control (without any bacteria), Kushneria sp. T3.7, Halomonas sp. 3R.12, Pseudomonas sp. AN21, Pseudomonas sp. AN23, Planomicrobium sp. 3S.31, and Pseudarthrobacter sp. ER25. Values were normalized for the control without bacteria and without salt. Capital letters refer to the comparison between different treatments in the same saline level, while lower case refers to the comparison of different salinity levels within the same bacterial treatment. Mean values sharing a common letter are not significantly different from each other (p > 0.05).
The salinity effect on root length without bacteria (control) was only apparent at 100 mM, showing no differences between the condition without salt and 50 mM of NaCl in the culture media. Pseudarthrobacter sp. ER25 and Planomicrobium sp. 3S.31 were the only treatments that did not differ significantly between any saline condition, while Pseudomonas sp. AN21 was the only treatment without significant differences between 50 and 100 mM of NaCl.
Roots were significantly longer when chia seedlings were treated with Pseudomonas sp. AN21, showing increments of 13.7 and 15.6% compared with the control treatment in the conditions without salt and in the presence of 100 mM NaCl, respectively (Figure 4). Planomicrobium sp. 3S.31 behaved similarly to the control, except with 50 mM NaCl, which showed shorter roots. In the case of Halomonas sp. 3R.12 and Pseudomonas sp. AN23, there was a significant decrease in roots length in each saline condition as salinity increased; however, there were no differences from the control at the highest salinity level. Pseudarthrobacter sp. ER25 showed a negative bacterial effect on the seedlings, displaying the shortest roots in all tested conditions (Figure 5).
There was little to no correlation between the variables (shoot length/root length) against (dry weight × total length). However, the data points showed clusters with different behaviors in each salinity condition and with different bacterial treatments (Figure 6). Overall, Control treatment clusters (seedlings without bacteria) were clearly defined and showed a reduction in seedling size and weight as the saline stress increased.
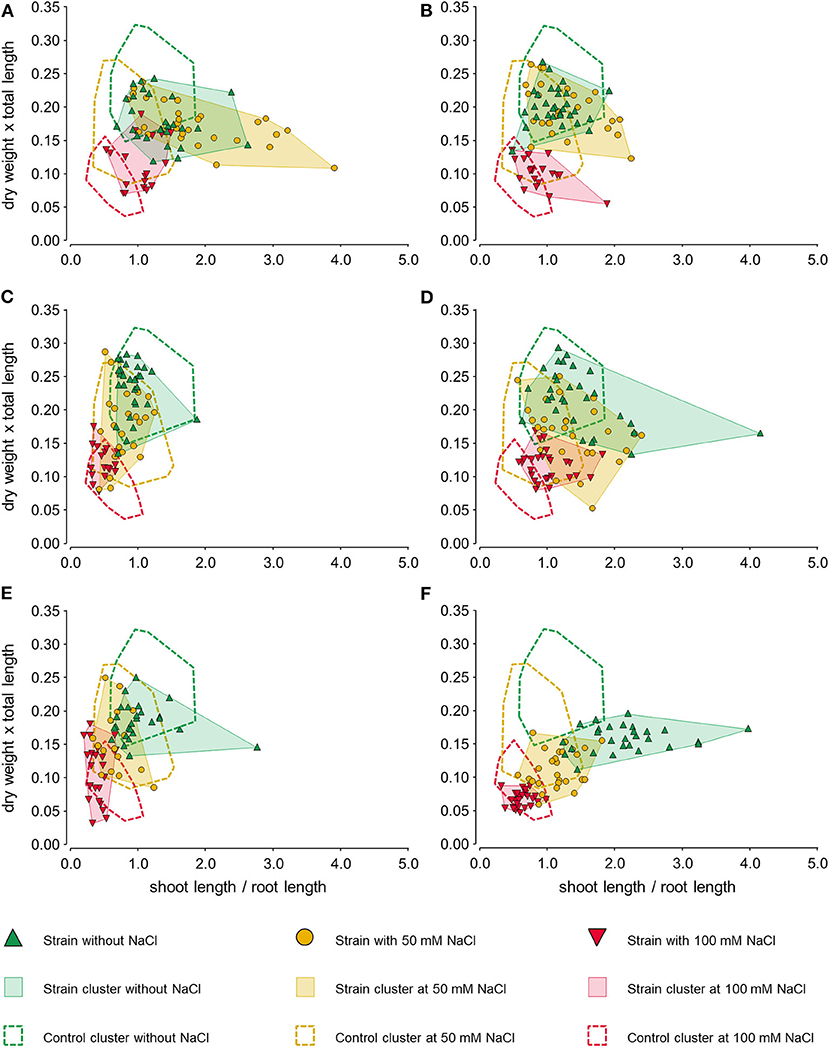
Figure 6. Scatter plots relating the ratios of growth variables of the 7-day-old chia (Salvia hispanica L.) seedlings grown on MS/2 agar with different saline conditions: without salt, with 50 and 100 mM NaCl. The seedlings grew in the presence of six bacterial strains, being the treatments: (A) Kushneria sp. T3.7, (B) Halomonas sp. 3R.12, (C) Pseudomonas sp. AN21, (D) Pseudomonas sp. AN23, (E) Planomicrobium sp. 3S.31, and (F) Pseudarthrobacter sp. ER25. Values were transformed with respect to the mean values of the Control treatment without bacteria and without salt. Areas with dashed-line borders show the cluster distribution of the control treatment (without bacteria) for each saline condition.
When the seedlings were treated with Planomicrobium sp. 3S.31 (Figure 6E) and Pseudarthrobacter sp. ER25 (Figure 6F), the arrangement of the clusters displayed negative morphological responses of the plants to the bacterial treatments. For Planomicrobium sp. 3S.31 (Figure 6E), shifting of the clusters to the left bottom region showed a higher presence of smaller seedlings with longer roots. Conversely, in the case of Pseudarthrobacter sp. ER25 (Figure 6F), there was a movement of clusters to the right and lower side of the Control clusters for each saline condition, evidencing smaller seedlings and root growth inhibition. Pseudomonas sp. AN21 (Figure 6C) showed a somewhat positive effect on chia seedlings by shifting the clusters of higher saline concentrations upwards and overlapping them with the cluster of seedlings grown without salt. However, the clusters compacted toward the left-hand side, indicating higher root elongation than in the Control treatment for each saline condition.
In the case of Halomonas sp. 3R.12 (Figure 6B), Pseudomonas sp. AN23 (Figure 6D), and Kushneria sp. T3.7 (Figure 6A), clear positive bacterial effects were shown in chia seedlings by the distribution of the clusters. When seedlings were treated with Halomonas sp. 3R.12 (Figure 6B), the clusters of the saline conditions moved upwards with respect to their Control clusters, overlapping the 50 mM NaCl saline-stress cluster over the cluster of the seedlings grown without salt. The same overlapping (the 50 mM NaCl cluster over the no-salt cluster) was also evidenced by the seedlings treated with Kushneria sp. T3.7 (Figure 6A), with an additional slight movement and overlapping of the 100-mM NaCl cluster over the cluster of the seedlings without salt as well. The shifting upwards and to the right of the clusters of saline stress evidenced a higher number of the heavier seedlings with a balanced relationship of shoot-to-root length. However, there was also a downward movement with respect to the Control cluster without salt, showing overall smaller seedlings in the absence of the saline conditions when chia was treated with Kushneria sp. T3.7 (Figure 6A). Lastly, Pseudomonas sp. AN23 (Figure 6D) showed the best effect on chia seedlings among all the treatments. There was shifting upward and to the right of the clusters of saline stress (50 and 100 mM NaCl) over the cluster of the seedlings without salt. This overlapping and condensation of data points showed a higher proportion of the seedlings with a morphological response similar to that of the unstressed plants.
Discussion
Soil salinization is a global threat to agriculture, and urgent solutions are needed to stop its progress and recover salt-affected soils. One of the aims of this study was to isolate microorganisms from soil and plant rhizosphere samples from a hypersaline environment to assess their performance as bioinoculants in the early growth of chia (Salviahispanica L.) under saline stress.
The bulk soil analysis showed that, in both sites, the soil had low quality. Due to their texture (composed mainly of sand and without a clay fraction), the soils showed all the typical features of sandy soils: low fertility (low-nutrient content), low-water retention, and poor structure (Thompson and Troeh, 2002). As expected, both sites showed high values of EC (22.6 dS/m) because of the proximity to the salt flat. According to the salinity classification of Kissel and Sonon (2008) for agricultural soils, the EC values measured in S1 and S2 exceeded by far the limit value of 2 dS/m, which sets the last range (out of six) in the classification, considered as excessively saline and unable to support plant growth.
Although S2 showed more adverse environmental conditions than S1, overall, it allowed the isolation of a higher number of culturable bacteria. Most microorganisms were isolated from the bulk soil and the rhizosphere, associated with the Poaceae plant located in S2, and were retrieved in the PCA 25 medium. This could be due to the enhancement of the soil quality promoted by the plant and the consequent higher biological activity in the adjacent soil. Most Poaceae species are facultative halophytes that can grow under saline stress and can be used in the phytoremediation of salt-impacted soils (Gerhardt et al., 2017). These plants increase their sodium uptake capacity and reduce soil salinity and sodicity by increasing their root biomass and root hairs when stimulated by plant growth-promoting rhizobacteria (PGPR) (Hasanuzzaman et al., 2014). Moreover, the low number of isolates recovered from the SEA medium, which was prepared with soil from the salt flat, showed the stressful conditions of the soil and poor nutritive quality in general when compared with the soil associated with the Poaceae plant in S2.
In accordance with our findings, Halomonas and Pseudomonas, two of the most abundant genera found, are reported as two of the three most commonly found genera in roots and rhizosphere of halophytes in extreme environments (Albdaiwi et al., 2019; Alexander et al., 2019; Kearl et al., 2019). However, Kushneria, Pseudarthrobacter, and Planomicrobium are less common, the last being not frequently reported as a PGP strain (Alexander et al., 2019; Banerjee et al., 2019; Mukhtar et al., 2019; Panda and Parida, 2019).
Although the highest number of isolates came from bulk soil samples initially, most of the strains selected based on their in vitro PGP activities came from rhizosphere samples. The rhizosphere of the plants from hypersaline environments allows the establishment of microorganisms that can withstand high-salt concentrations and benefit the host plant with potential use in crops under saline stress (Palacio-Rodríguez et al., 2017; Mukhtar et al., 2019; Xiong et al., 2019). Moreover, out of the 13 selected microorganisms, eight were Poaceae-associated bacteria, while the other five (Pseudomonas strains and Pseudarthrobacter sp.) were all Asteraceae-associated microorganisms from the rhizosphere samples. The lack of microorganisms from Fabaceae could be related to the isolation methods used. While Fabaceae plants can associate with symbiotic (the members of Rhizobiae in legumes) and free-living rhizobacteria (Pseudomonas, Bacillus, and Azospirillum in grasses and other non-legumes) (Reginato et al., 2012), most studies focus on the isolation of endophytic bacteria from legumes (Reginato et al., 2012; Turan et al., 2017; Dubey et al., 2020). Also, differences in root architecture and structure could have influenced the isolation efficiency (Vacheron et al., 2013). In the case of Fabaceae, the rhizobial colonization process starts from the root tip upward, leading to the formation of root nodules. On the contrary, in Poaceae, PGPR prefers to colonize root hairs and lateral roots to express their plant beneficial properties (Vacheron et al., 2013). Therefore, since there was no root-macerate isolation, the isolated strains came from rhizosphere of the plants and rhizoplane, neglecting the possible collection of endophytic bacteria from the inner root of Fabaceae. Strikingly, some culture media behaved in a rather selective manner for isolating a particular genus: TSA 100 for Kushneria strains and NFb 1 M for Pseudomonas strains. However, in PCA 25, there was higher isolation diversity, providing all Halomonas sp. 3R strains and Planomicrobium. There was only one strain isolated from SEA, and two Halomonas sp. AR were isolated from ANF 2 M.
When considering the PGP-screening, each genus displayed a particular type of activity in a wide range of salinity conditions and, therefore, showed its application potential in different scenarios of salt-affected or salt-degraded soils. Kushneria strains stood out in phosphates solubilization (with and without salt and with both phosphorus sources). Such was the case of Kushneria sp. YCWA18, high phosphorus-solubilizing halotolerant bacteria that performed under a wide range of salinity conditions [from 0 to 20% (w/v) of NaCl] (Zhu et al., 2011). All of the studied strains of Halomonas produced siderophores in all salinity conditions and showed the highest biomass production in a wide range of salinity concentrations, from less-stressed conditions (50 mM) to 40 times higher NaCl concentrations. In line with our results, different species in the genus Halomonas were reported to have multiple PGP traits such as IAA production, ACC deaminase activity, phosphate solubilization, and high production of exopolysaccharides and siderophores, showing a particular enhanced siderophores production under multiple stresses in salt-supplemented media (Etesami and Beattie, 2018; Mukherjee et al., 2019). The studied Pseudomonas strains held the highest number of PGP activities, being able to fix nitrogen, produce siderophores, and solubilize phosphates without salts and with 1 M NaCl. Similar results showed that most Pseudomonas strains were able to simultaneously perform a wide range of PGP features (Fang et al., 2019; Costa-Gutierrez et al., 2020; Lami et al., 2020). Moreover, besides showing PGP activities with and without salt, their optimal salinity ranges located around the highest NaCl concentrations (from 1,000 to 1,750 mM NaCl). Finally, Planomicrobium sp. 3S.31 was able to solubilize phosphates in both tested media and salinity conditions, an activity reported for another strain of Planomicrobium sp. as intermediate solubilization (Khan et al., 2018, 2019) and also found among many other PGP features in Planomicrobium okeanokoites (Verma et al., 2016).
Considering chia plant-bacteria interaction, the strains Halomonas sp.3R.12, Kushneria sp. T3.7, and Pseudomonas sp. AN23 showed a positive effect on the plants. Halomonas sp. 3R.12, closely related to Halomonas sulfidaeris, increased plant growth parameters in chia grown under 50 mM NaCl. Although there are no previous reports of the strain H. sulfidaeris as a plant growth promoter, in particular, some studies showed that Halomonas sp. increased total biomass in fresh and dry weights (by expanding roots and shoots) in alfalfa and rice seedlings (Kearl et al., 2019; Mukherjee et al., 2019). The strain Kushneria sp. T3.7, which also showed significant increments in growth parameters under saline stress, was closely related to Kushneria marisflavi. However, there is little consensus about the plant growth-promoting traits of this strain. While some authors described it as beneficial to plants because of its germination acceleration (Navarro-Torre et al., 2016), others described its stimulatory effect on plant growth as slight compared with other strains (Kearl et al., 2019). Pseudomonas sp. AN23 showed a greater positive effect on chia growth under saline stress since stressed inoculated seedlings presented a similar morphological response to non-stressed plants. This strain was closely related to Pseudomonas zhaodongensis, a strain reported to increase fresh weight in Arabidopsis sp. (Del Mora-Ruiz et al., 2018). However, few reports mentioned the effect of this particular Pseudomonas strain on plant growth. The positive effect of the three strains was also seen on the proportions between roots and shoot length, which was more balanced than in other treatments. Since plants can alter their phenotype to maximize fitness according to the external environmental conditions, such as nutrients availability, they can allocate more biomass to the organ that captures the most limiting resources (Sugiura and Tateno, 2011). In this sense, a balanced relation could mean that the strains Halomonas sp.3R.12, Kushneria sp. T3.7, and Pseudomonas sp. AN23 were efficient enough in making nutrients available for the seedlings (Sugiura and Tateno, 2011), unlike Pseudomonas sp. AN21, which showed an unequal root/shoot length ratio in the more stressful condition.
Chia seedlings displayed negative responses when inoculated with Planomicrobium sp. 3S.31 and Pseudarthrobacter sp. ER25. Planomicrobium sp. 3S.31, which showed small seedlings of long roots and short shoots, was closely related to Planomicrobium flavidum and P. mcmeekinii. Although some Planomicrobium strains were described with PGP features under no saline conditions (Khan et al., 2019), P. flavidum, in particular, was mostly reported as a biodegradation agent of soil contaminants (Nagar et al., 2018; Mohamed and Mustafa, 2019). Pseudarthrobacter sp. ER25 showed an opposite adverse effect, altering the phenotype of the plants with abnormal short roots and long shoots in all salinity conditions. This negative effect on the roots could be caused by an excess of IAA (Jangu and Sindhu, 2011; Tabatabaei et al., 2016) due to the production of the phytohormone by the strain. Pseudarthrobacter sp. ER25 is closely related to P. oxydans, which showed no significant effects on maize root dry weight (Abadi et al., 2020) and produced lower germination in seeds of Salicornia ramosissima inoculated with it (Mesa-Marín et al., 2019).
Conclusion
In conclusion, this study increases the knowledge about the microorganisms from hypersaline environments and their potential as plant growth promoters for salt-sensitive crops. It shows the strains, displaying PGP features from a non-stressed to a highly saline-stressed situation, emphasizing their biotechnological potential for diverse uses in different soil degradation levels. To the best of our knowledge, this is the first study that explores the potential of bacteria isolated from bulk soil and rhizosphere samples from the Salar del Hombre Muerto in chia (Salvia hispanica L.) growth promotion. Among the studied strains, Pseudomonas sp. AN23, Kushneria sp. T3.7, and Halomonas sp. 3R.12 were the most promising for their positive effect on the growth of chia seedlings and were, therefore, selected for further research. We conclude that free-living halophile bacteria from hypersaline environments, like the Salar del Hombre Muerto, could enhance plant growth and stress tolerance of crops other than their natural partners.
Data Availability Statement
The raw data supporting the conclusions of this article will be made available by the authors, without undue reservation.
Author Contributions
MY-Y: conceptualization, methodology, visualization, formal analysis, and writing—original draft preparation. NR-A: visualization, formal analysis, data curation, and writing—reviewing and editing. VR: writing—reviewing and editing and supervision. VI: conceptualization, writing—reviewing and editing, supervision, and funding acquisition. All authors contributed to the article and approved the submitted version.
Funding
This manuscript was partially supported by the Agencia Nacional de Promoción Científica y Tecnológica (ANPCyT) (PICT 2017-1909), by the Consejo Nacional de Investigaciones Científicas y Técnicas (CONICET) (PIP 332), and by the Consejo de Investigaciones de la Universidad Nacional de Salta (CIUNSa) through the research projects N° 2070/4 program 2070, and project N° 2577. MY-Y was beneficiary of a doctoral fellowship from CONICET.
Conflict of Interest
The authors declare that the research was conducted in the absence of any commercial or financial relationships that could be construed as a potential conflict of interest.
Acknowledgments
The authors would like to thank Prof. Jerold Last for his kind help correcting the language.
Footnotes
1. ^National Center for Biotechnology Information. NCBI GenBank. Available online at: https://www.ncbi.nlm.nih.gov/genbank/
References
Abadi, A. V. M., Sepehri, M., Rahmani, H. A., and Zarei, M. (2020). Role of dominant phyllosphere bacteria with plant growth – promoting characteristics on growth and nutrition of maize (Zea mays L.). J. Soil Sci. Plant Nutr. 20, 1–16. doi: 10.1007/s42729-020-00302-1
Albdaiwi, R. N., Khyami-horani, H., Ayad, J. Y., Alananbeh, K. M., and Al-sayaydeh, R. (2019). Isolation and characterization of halotolerant plant growth promoting rhizobacteria from durum wheat (Triticum turgidum subsp. durum) Cultivated in Saline Areas of the Dead Sea Region. Front Microbiol. 10:1639. doi: 10.3389/fmicb.2019.01639
Alexander, A., Mishra, A., and Jha, B. (2019). “Halotolerant rhizobacteria: a promising probiotic for saline soil-based agriculture,” in Saline Soil-Based Agriculture by Halotolerant Microorganisms, eds M. Kumar, H. Etesami and V. Kumar (Singapore: Springer Nature Singapore Pte Ltd. 2019), 53–73. doi: 10.1007/978-981-13-8335-9_3
Alexandratos, N., and Bruinsma, J. (2012). World Agriculture Towards 2030 / 2050 The 2012 REVISION. Rome.
Banerjee, A., Sarkar, S., Cuadros-Orellana, S., and Bandopadhyay, R. (2019). “Exopolysaccharides and biofilms in mitigating salinity stress: the biotechnological potential of halophilic and soil-inhabiting PGPR microorganisms,” in Microorganisms in Saline Environments: Strategies and Functions, eds B. Giri and A. Varma (Cham: Springer Nature Switzerland AG 2019 B.), 133–153. doi: 10.1007/978-3-030-18975-4_6
Costa-Gutierrez, S. B., Raimondo, E. E., Lami, M. J., Vincent, P. A., Espinosa-Urgel, M., and de Cristóbal, R. E. (2020). Inoculation of Pseudomonas mutant strains can improve growth of soybean and corn plants in soils under salt stress. Rhizosphere 16:100255. doi: 10.1016/j.rhisph.2020.100255
Del Mora-Ruiz, M. R., Alejandre-Colomo, C., Ledger, T., González, B., Orfila, A., and Rosselló-Móra, R. (2018). Non-halophilic endophytes associated with the euhalophyte Arthrocnemum macrostachyum and their plant growth promoting activity potential. FEMS Microbiol. Lett. 365, 1–11. doi: 10.1093/femsle/fny208
Di Rienzo, J. A., Casanoves, F., Balzarini, M. G., Gonzalez, L., Tablada, M., and Robledo, C. W. (2008). InfoStat, Versión 2008. FCA, Univ. Nac. Córdoba.
Döbereiner, J., Marriel, I., and Nery, M. (1976). Ecological distribution of Spirillum lipoferum. Beijerinck. Can. J. Microbiol. 22, 1464–1473. doi: 10.1139/m76-217
Dubey, A., Kumar, A., and Mohammad Latif, K. (2020). “Role of biostimulants for enhancing abiotic stress tolerance in fabaceae plants,” in The Plant Family Fabaceae: Biology and Physiological Responses to Environmental Stresses, eds M. Hasanuzzaman, S. S. Gill, and S. Araújo (Singapore: Springer Nature Singapore Pte Ltd. 2020), 547. doi: 10.1007/978-981-15-4752-2_8
Edbeib, M. F., Wahab, R. A., and Huyop, F. (2016). Halophiles : Biology, adaptation, and their role in decontamination of hypersaline environments Halophiles : biology, adaptation, and their role in decontamination of hypersaline environments. World J. Microbiol. Biotechnol. 32, 1–23. doi: 10.1007/s11274-016-2081-9
Etesami, H., and Beattie, G. A. (2018). Mining halophytes for plant growth-promoting halotolerant bacteria to enhance the salinity tolerance of non-halophytic crops. Front. Microbiol. 9:148. doi: 10.3389/fmicb.2018.00148
Fang, K., Bao, Z., Chen, L., and Zhou, J. (2019). Growth-promoting characteristics of potential nitrogen- fixing bacteria in the root of an invasive plant Ageratina adenophora. PeerJ 7:e7099. doi: 10.7717/peerj.7099
FAO (2018). Handbook for Saline Soil Management. Food and Agriculture Organization of the United Nations and Lomonosov Moscow State University. Available onlinre at: http://www.fao.org/3/i7318en/I7318EN.pdf
Food and Agricultural Organization (2009). Feeding the World in 2050. World Agricultural Summit on Food Security. Rome.
Food and Agriculture Organization of the United Nations (2017). The Future of Food and Agriculture: Trends and Challenges. Rome. Available online at: http://www.fao.org/3/a-i6583e.pdf
Food and Agriculture Organization of the United Nations and I. T. P. on S. (2015). Status of the World's Soil Resources (SWSR) – Main Report.
Gerhardt, K. E., Macneill, G. J., Gerwing, P. D., and Greenberg, B. M. (2017). “Phytoremediation of salt-impacted soils and use of plant growth-promoting rhizobacteria (PGPR) to enhance phytoremediation,” in Phytoremediation, eds A. A. Ansari, S. Singh Gill, R. G. R. Lanza, and L. Newman (Cham: Springer International Publishing AG), 19–51. doi: 10.1007/978-3-319-52381-1_2
Glickmann, E., and Dessaux, Y. (1995). A critical examination of the specificity of the Salkowski reagent for indolic compounds produced by phytopathogenic bacteria. Appl. Environ. Microbiol. 61, 793–796. doi: 10.1128/aem.61.2.793-796.1995
Hasanuzzaman, M., Nahar, K., Alam, M., Bhowmik, P. C., Hossain, A., Rahman, M. M., et al. (2014). Potential use of halophytes to remediate saline soils. Biomed Res. Int. 2014, 1–12. doi: 10.1155/2014/589341
Ivushkin, K., Bartholomeus, H., Bregt, A. K., Pulatov, A., and Kempen, B. (2019). Remote sensing of environment global mapping of soil salinity change. Remote Sens. Environ. 231, 1–12. doi: 10.1016/j.rse.2019.111260
Ixtaina, V. Y., Nolasco, S. M., and Tomás, M. C. (2008). Physical properties of chia (Salvia hispanica L.) seeds. Ind. Crops Prod. 28, 286–293. doi: 10.1016/j.indcrop.2008.03.009
Jangu, O., and Sindhu, S. (2011). Differential response of inoculation with indole acetic acid producing Pseudomonas sp in green gram (Vigna radiata L.) and black gram (Vigna mungo L.). Microbiol. J. 1, 159–173. doi: 10.3923/mj.2011.159.173
Jardim Rosa, D. B., Soares Schultz, J., Bassi Moreno, L., Silva Michels, G., Jardim Rosa, Y. B., Soares Rosa Lemes, C., et al. (2014). Germinação de Salvia splendens L. submetida à salinidade. Ornam. Hortic. 21, 105–112. doi: 10.14295/rbho.v21i1.782
Jorquera, M. A., Maruyama, F., Ogram, A. V., Navarrete, O. U., Lagos, L. M., Inostroza, N. G., et al. (2016). Rhizobacterial community structures associated with native plants grown in chilean extreme environments. Microb. Ecol. 72, 633–646. doi: 10.1007/s00248-016-0813-x
Kearl, J., McNary, C., Lowman, J. S., Mei, C., Aanderud, Z. T., Smith, S. T., et al. (2019). Salt-tolerant halophyte rhizosphere bacteria stimulate growth of alfalfa in salty soil. Front. Microbiol. 10:1849. doi: 10.3389/fmicb.2019.01849
Khan, N., Bano, A., and Babar, A. (2019). The stimulatory effects of plant growth promoting rhizobacteria and plant growth regulators on wheat physiology grown in sandy soil. Arch. Microbiol. 201, 769–785. doi: 10.1007/s00203-019-01644-w
Khan, N., Zandi, P., Ali, S., Mehmood, A., and Shahid, M. A. (2018). Impact of salicylic acid and PGPR on the drought tolerance and phytoremediation potential of helianthus annus. Front. Microbiol. 9:2507. doi: 10.3389/fmicb.2018.02507
Kryuchkova, Y. V., Burygin, G. L., Gogoleva, N. E., Gogolev, Y. V., Chernyshova, M. P., Makarov, O. E., et al. (2014). Isolation and characterization of a glyphosate-degrading rhizosphere strain, Enterobacter cloacae K7. Microbiol. Res. 169, 99–105. doi: 10.1016/j.micres.2013.03.002
Kumawat, K. C., Sharma, P., Nagpal, S., Gupta, R. K., Sirari, A., Nair, R. M., et al. (2021). Dual microbial inoculation, a game changer? – Bacterial biostimulants with multifunctional growth promoting traits to mitigate salinity stress in spring mungbean. Front. Microbiol. 11:576. doi: 10.3389/fmicb.2020.600576
Lami, M. J., Adler, C., Caram-Di Santo, M. C., Zenoff, A. M., de Cristóbal, R. E., Espinosa-Urgel, M., et al. (2020). Pseudomonas stutzeri MJL19, a rhizosphere-colonizing bacterium that promotes plant growth under saline stress. J. Appl. Microbiol. 129, 1321–1336. doi: 10.1111/jam.14692
Lowe, B. A., Marsh, T. L., Isaacs-Cosgrove, N., Kirkwood, R. N., Kiupel, M., and Mulks, M. H. (2011). Microbial communities in the tonsils of healthy pigs. Vet. Microbiol. 147, 346–357. doi: 10.1016/j.vetmic.2010.06.025
Martínez, F., Orce, I., Rajal, V., and Irazusta, V. (2019). Salar del Hombre Muerto, source of lithium-tolerant bacteria. Environ. Geochem. Health 41, 529–543. doi: 10.1007/s10653-018-0148-2
Merritt, J. H., Kadouri, D. E., and O'Toole, G. A. (2011). Growing and analyzing static biofilms. Curr. Protoc. Microbiol., 1, 1–18. doi: 10.1002/9780471729259.mc01b01s22
Mesa-Marín, J., Pérez-Romero, J. A., Mateos-Naranjo, E., Bernabeu-Meana, M., Pajuelo, E., Rodríguez-Llorente, I. D., et al. (2019). Effect of plant growth-promoting rhizobacteria on salicornia ramosissima seed germination under salinity, CO2 and temperature stress. Agronomy 9, 1–14. doi: 10.3390/agronomy9100655
Mohamed, E. A. H., and Mustafa, F. (2019). Captan utilization by a soil bacterium Planomicrobium flavidum strain EF. Sains Malaysiana 47, 85–89. doi: 10.17576/jsm-2018-4701-10
Mukherjee, P., Mitra, A., and Roy, M. (2019). Halomonas rhizobacteria of avicennia marina of indian sundarbans promote rice growth under saline and heavy metal stresses through exopolysaccharide production. Front. Microbiol. 10:1207. doi: 10.3389/fmicb.2019.01207
Mukhtar, S., Mehnaz, S., and Kauser, A. (2019). Microbial diversity in the rhizosphere of plants growing under extreme environments and its impact on crop improvement. Environ. Sustain. 2, 329–338. doi: 10.1007/s42398-019-00061-5
Muñoz, L. A., Cobos, A., Diaz, O., and Aguilera, J. M. (2013). Chia seed (Salvia hispanica): an ancient grain and a new functional food. Food Rev. Int. 29, 394–408. doi: 10.1080/87559129.2013.818014
Murashige, T., and Skoog, F. (1962). A revised medium for rapid growth and Bio assays with Tobacco tissue cultures. Physiol. Plant. 15, 474–497. doi: 10.1111/j.1399-3054.1962.tb08052.x
Nagar, S., Kumar, A., Shalini, S., Mary, A. S., Pramod, C., and Rai, K. (2018). Aerobic biodegradation of HMX by Planomicrobium flavidum. 3 Biotech. 8:455. doi: 10.1007/s13205-018-1479-5
Navarro-Torre, S., Mateos-Naranjo, E., Caviedes, M. A., Pajuelo, E., and Rodríguez-Llorente, I. D. (2016). Isolation of plant-growth-promoting and metal-resistant cultivable bacteria from Arthrocnemum macrostachyum in the Odiel marshes with potential use in phytoremediation. Mar. Pollut. Bull. 110, 133–142. doi: 10.1016/j.marpolbul.2016.06.070
Orona-Tamayo, D., Valverde, M. E., and Paredes-López, O. (2017). “Chia — The new golden seed for the 21st century : nutraceutical properties and technological uses,” in Sustainable Protein Sources, eds S. R. Nadathur, J. P. D. Wanasundara, and L. Scanlin (Elsevier Inc.), 265–281. doi: 10.1016/B978-0-12-802778-3.00017-2
Palacio-Rodríguez, R., Coria-Arellano, J. L., López-Bucio, J., Sánchez-Salas, J., Muro-Pérez, G., Castañeda-Gaytán, G., et al. (2017). Halophilic rhizobacteria from Distichlis spicata promote growth and improve salt tolerance in heterologous plant hosts. Symbiosis 73, 179–189. doi: 10.1007/s13199-017-0481-8
Panda, A., and Parida, A. K. (2019). “Development of salt tolerance in crops employing halotolerant plant growth– promoting rhizobacteria associated with halophytic rhizosphere soils,” in Saline Soil-Based Agriculture by Halotolerant Microorganisms, eds M. Kumar, H. Etesami, and V. Kumar (Singapore: Springer Nature Singapore Pte), 75–101. doi: 10.1007/978-981-13-8335-9_4
Pérez Brandán, J., Curti, R. N., and Acreche, M. M. (2020). Developmental responses of chia (Salvia hispanica) to variations in thermo-photoperiod : impact on subcomponents of grain yield. Crop. Pasture Sci. 71, 183–189. doi: 10.1071/CP19218
Pilet, P. E., and Chollet, R. (1970). Sur le dosage colorime'trique de l'acide indolylace'tique. Comptes Rendus l'Acad. Sci. 271, 1675–1678.
Pospiech, A., and Neumann, B. (1995). A versatile quick-prep of genomic DNA from gram-positive bacteria. Trends Genet. 11, 217–218. doi: 10.1016/S0168-9525(00)89052-6
Raimondi, G., Rouphael, Y., Di Stasio, E., Napolitano, F., Clemente, G., Maiello, R., et al. (2017). Evaluation of Salvia hispanica performance under increasing salt stress conditions. Acta Hortic. 1170, 703–707. doi: 10.17660/ActaHortic.2017.1170.88
Reginato, M., Sgroy, V., Llanes, A., Cassán, F., and Luna, V. (2012). “The American halophyte prosopis strombulifera, a new potential source to confer salt tolerance to crops,” in Crop Production for Agricultural Improvement, eds M. Ashraf, M. Öztürk, M. S. Aqeel Ahmad, and A. Aksoy (Dordrecht: Springer Science+Business Media B.V), 20. doi: 10.1007/978-94-007-4116-4_5
Romano Armada, N., Doccula, F. G., Candeo, A., Valentini, G., Costa, A., and Bassi, A. (2019). In vivo light sheet fluorescence microscopy of calcium oscillations in Arabidopsis thaliana. Calcium Signalling. Methods Mol. Biol. 1925, 87–101. doi: 10.1007/978-1-4939-9018-4_8
Romano-Armada, N., Yañez Yazlle, M. F., Irazusta, V., Rajal, V. B., and Moraga, N. B. (2020). Potential of bioremediation and PGP traits in streptomyces as strategies for bio-reclamation of salt-affected soils for agriculture. Pathogens 9:117. doi: 10.3390/pathogens9020117
Sáenz-Mata, J., Palacio-Rodríguez, R., Sánchez- Galván, H., and Balagurusamy, N. (2016). “Plant growth promoting rhizobacteria associated to halophytes: potential applications in agriculture,” in Sabkha Ecosystems, eds M. Ajmal Khan, B. Boër, M. Ȫzturk, M. Clüsener-Godt, B. Gul, and S. W. Breckle (Cham: Springer International Publishing), 411–425. doi: 10.1007/978-3-319-27093-7_24
Schindelin, J., Arganda-Carreras, I., Frise, E., Kaynig, V., Longair, M., Pietzsch, T., et al. (2012). Fiji: an open-source platform for biological-image analysis. Nat. Methods 9, 676–682. doi: 10.1038/nmeth.2019
Schwyn, B., and Neilands, B. (1987). Universal chemical assay for the detection determination of siderophores. Anal. Biochem. 160, 47–56. doi: 10.1016/0003-2697(87)90612-9
Shankar, V., and Evelin, H. (2019). “Strategies for reclamation of saline soils,” in Microorganisms in Saline Environments: Strategies and Functions, eds B. Giri and A. Varma (Cham: Springer Nature Switzerland AG), 439–449. doi: 10.1007/978-3-030-18975-4_19
Shekhar Nautiyal, C. (1999). An efficient microbiological growth medium for screening phosphate solubilizing microorganisms. FEMS Microbiol. Lett. 170, 265–270. doi: 10.1111/j.1574-6968.1999.tb13383.x
Srivastava, P., Wu, Q. S., and Giri, B. (2019). “Salinity : an overview,” in Microorganisms in Saline Environments: Strategies and Functions, eds B. Giri and A. Varma (Cham: Springer Nature Switzerland AG 2019 B.), 3–18. doi: 10.1007/978-3-030-18975-4_1
Stefanello, R., Neves, L. A., Abbad, M. A., and Viana, B. B. (2015). Resposta fisiológica de sementes de chia (Salvia hispanica – Lamiales: Lamiaceae) ao estresse salino. Biotemas 28:35. doi: 10.5007/2175-7925.2015v28n4p35
Sugiura, D., and Tateno, M. (2011). Optimal leaf-to-root ratio and leaf nitrogen content determined by light and nitrogen availabilities. PLoS ONE 6:e22236. doi: 10.1371/journal.pone.0022236
Tabatabaei, S., Ehsanzadeh, P., Etesami, H., Alikhani, H. A., and Glick, B. R. (2016). Indole-3-acetic acid (IAA) producing pseudomonas isolates inhibit seed germination and α-amylase activity in durum wheat (triticum turgidum L.). Spanish J. Agric. Res. 14, 1–10. doi: 10.5424/sjar/2016141-8859
Turan, M., Kitir, N., Elkoca, E., Uras, D., Ünek, C., Nikerel, E., et al. (2017). “Nonsymbiotic and symbiotic bacteria efficiency for legume growth under different stress conditions,” in Microbes for Legume Improvement, eds A. Zaidi, M. S. Khan, and J. Musarrat (Cham: Springer International Publishing), 387–404. doi: 10.1007/978-3-319-59174-2_16
Vacheron, J., Desbrosses, G., Bouffaud, M. L., Touraine, B., Moënne-Loccoz, Y., Muller, D., et al. (2013). Plant growth-promoting rhizobacteria and root system functioning. Front. Plant Sci. 4:356. doi: 10.3389/fpls.2013.00356
Verma, P., Yadav, A. N., Khannam, K. S., Kumar, S., Saxena, A. K., and Suman, A. (2016). Molecular diversity and multifarious plant growth promoting attributes of Bacilli associated with wheat (Triticum aestivum L.) rhizosphere from six diverse agro-ecological zones of India. J. Basic Microbiol. 56, 44–58. doi: 10.1002/jobm.201500459
Wu, X., Xie, Y., Qiao, J., Chai, S., and Chen, L. (2019). Rhizobacteria strain from a hypersaline environment promotes plant growth of Kengyilia thoroldiana. Microbiology 88, 220–231. doi: 10.1134/S0026261719020127
Xiong, Y. W., Gong, Y., Li, X. W., Chen, P., Ju, X. Y., Zhang, C. M., et al. (2019). Enhancement of growth and salt tolerance of tomato seedlings by a natural halotolerant actinobacterium Glutamicibacter halophytocola KLBMP 5180 isolated from a coastal halophyte. Plant Soil 445, 307–322. doi: 10.1007/s11104-019-04310-8
Keywords: halotolerant bacteria, nitrogen fixation, phosphate solubilization, siderophores production, indole-3-acetic acid production, biofilms production, Kushneria, Halomonas
Citation: Yañez-Yazlle MF, Romano-Armada N, Rajal VB and Irazusta VP (2021) Amelioration of Saline Stress on Chia (Salvia hispanica L.) Seedlings Inoculated With Halotolerant Plant Growth-Promoting Bacteria Isolated From Hypersaline Environments. Front. Agron. 3:665798. doi: 10.3389/fagro.2021.665798
Received: 08 February 2021; Accepted: 20 May 2021;
Published: 17 June 2021.
Edited by:
Antonio Rafael Sánchez-Rodríguez, University of Cordoba, SpainReviewed by:
Sheng Qin, Jiangsu Normal University, ChinaAna María García-López, University of Seville, Spain
Copyright © 2021 Yañez-Yazlle, Romano-Armada, Rajal and Irazusta. This is an open-access article distributed under the terms of the Creative Commons Attribution License (CC BY). The use, distribution or reproduction in other forums is permitted, provided the original author(s) and the copyright owner(s) are credited and that the original publication in this journal is cited, in accordance with accepted academic practice. No use, distribution or reproduction is permitted which does not comply with these terms.
*Correspondence: Verónica Patricia Irazusta, aXJhenVzdGF2ZXJvbmljYUBnbWFpbC5jb20=
†ORCID: María Florencia Yañez-Yazlle orcid.org/0000-0002-1623-5715
Neli Romano-Armada orcid.org/0000-0003-3148-2041
Verónica Beatriz Rajal orcid.org/0000-0002-2290-8920
Verónica Patricia Irazusta orcid.org/0000-0001-7436-8543