- 1Biology Department, Trinity Western University, Langley, BC, Canada
- 2Faculty of Land and Food Systems, University of British Columbia, Vancouver, BC, Canada
Although evolution has been often seen as a gradual process through a Darwinian lens, far more rapid evolutionary change has been observed in recent times. Recent examples documenting the potential speed of invasive plant evolution have included: latitudinal flowering clines, life history shifts, or abrupt changes in morphology. The timescales for such observations range from centuries down to <5 years. Invasive weeds provide good models for the rapid changes, partly because invasive weeds exhibit unique evolutionary mechanisms integral to their success. For example, purging of their genetic load may enable invasive plants to adapt more rapidly. Other genetic mechanisms include plasticity as an evolved trait, hybridization, polyploidy, epigenetics, and clonal division of labor. It is well-demonstrated that anthropogenic stressors such as habitat disturbance or herbicide use may work synergistically with climate change stressors in fostering rapid weed evolution. Changing temperatures, moisture regimes and extreme climate events operate universally, but invasive plant species are generally better equipped than native plants to adapt. Research on this potential for rapid evolution is critical to developing more proactive management approaches that anticipate new invasive plant ecotypes adapted to changing climatic conditions.
Introduction
Darwin's general vision for the way evolution works was via small, incremental changes, taking place over relatively long timespans (Gould and Eldredge, 1977). Although support for this Darwinian mode of evolution still pervades evolutionary theory, other models have emerged accommodating the reality that more rapid evolution than Darwin pictured frequently occurs (e.g., Alphen, 1999; Hairston et al., 2005; Pick and Heffer, 2012; Simon and Peccoud, 2018). In fact, in the past decade or two, there has been a paradigm shift where evolution occurring on a relatively short timescale is considered “ordinary” (Reznick et al., 2019).
In this review, we will look at evolutionary change in a particular group of species, invasive weeds. An invasive weed may be defined as a plant that is “either native or non-native, and may have negative effects on either natural ecosystems or agroecosystems, but must clearly be invasive in that it exhibits a tendency to rapidly colonize and spread to occupy new niches” (Clements, 2017). Some other sources define the term more narrowly, but with considerable overlap among niches among different types of plants in this general category, e.g., agricultural weeds that also invade natural areas and vice versa, we think it is useful to go with a more all-encompassing definition.
Invasive weeds provide many examples of more rapid evolutionary modes. Recent examples documenting the potential speed of invasive plant evolution have included: invasive plants establishing latitudinal flowering clines, life history shifts, or dramatic changes in root systems. The fact that many invasive plants are genetically depauperate, often due to possession of breeding systems with a high proportion of selfing (Clements et al., 2004), can work to their advantage, whereas genetic bottlenecks put endangered plants at risk of extinction (Lee et al., 2018). Even the evolutionary mechanism of purging of their genetic load may enable invasive plants adapt more rapidly (Marchini et al., 2015). The numerous other genetic mechanisms that have been attributed to rapid evolution by invasive weeds largely follow from adaptations from their roles as pioneer species for the most part, designed to respond rapidly to environmental stressors associated with disturbance.
The genetic structure of weed populations varies widely among weed species, and even varies intraspecifically among different weed populations in many cases. Outcomes of natural selection differ depending on genetic structure, so it is very important to understand the range of breeding systems among weed species. Furthermore, phenotypic plasticity is widely recognized as an extremely important trait for a large number of weed species, as first highlighted when Baker referred to weeds often possessing an “all-purpose genotype” (Baker, 1965). However, many invasive plants still rely on rapid evolutionary change to adapt to their environments, such that the “all-purpose genotype” is not a universally appropriate label for invasive weeds because many invasive weeds do have considerable genetic diversity and are capable of adapting to local conditions (Clements et al., 2004). Weed reproductive systems are diverse, and among the greatest potential influences on life-history changes during weed evolution may involve characteristics of the reproductive system itself (Barrett et al., 2008). Barrett et al. (2008) explore many possibilities for such transformations in plant reproductive systems, many of which involve clonality and asexual reproduction. For example, they cite the case of an aquatic invasive plant, Eichhornia paniculata (Spreng.) Solms (Brazilian water hyacinth) that went from being outcrossing in its native range to selfing in its invaded range, which was critical to establishment in its adventive range where its pollinators were absent (Barrett et al., 1989, 2008). Critically important is understanding how particular weedy traits, such as reproductive characteristics, are impacted by climate change stressors so that we can better predict what traits could adapt to higher temperatures, CO2 levels and other climate factors (Clements and DiTommaso, 2011).
Climate change stressors such as changing temperatures, moisture regimes and extreme climate events are operating universally, but studies have repeatedly shown that invasive plant species are generally better equipped than native plants or crops to adapt. One indication of this is the observation of niche shifts. Climate niche breadths for eight weeds were on the whole, broader in China than in their native ranges (Wan et al., 2017). Plant species like Solidago canadensis L. (Canada goldenrod) not considered a weed in its native North American range grow much taller and larger in China making the plant more competitive, and recently becoming a serious management issue (Cheng et al., 2020). Research focusing on leading invasion edges, has demonstrated the ability of some species to adapt to rapidly changing temperatures or moisture levels that come with climate change (Lustenhouwer et al., 2018; Williams et al., 2019). Some of the clearest examples of rapid evolution of invasive plants in response to changing climate are abrupt changes in flowering timing, enabling these plants to maintain or increase their reproductive output (Barrett et al., 2008; Crimmins et al., 2009; Lustenhouwer et al., 2018; de Oliveira Xavier et al., 2019). Seed dispersal adaptations are also key to the success of many invasive plants, facilitating both population spread and population growth. Local adaption to changing climatic conditions have been studied the world over for many global colonizers. For example, adaptation to climate change by Ambrosia artemisiifolia L. (common ragweed) is evident on 5 continents: its home continent North America, South America, Europe, Asia and Australia (Essl et al., 2015; Gallien et al., 2016; Sun and Roderick, 2019; van Boheemen et al., 2019).
The purpose of this review is to provide an overview of what is presently known of the dynamic interaction between invasive plants and climate change, particularly focusing on the potential for invasive plants to adapt rapidly to changes in climate. This review will also reveal the numerous gaps in our knowledge of this potentially rapid weed evolution amidst climate change, and to suggest research approaches to fill these gaps. The world is changing quickly and we likewise need to respond quickly to the forecasted rapid evolution and spread of invasive plants to minimize the negative consequences.
Recent Examples of Increased Invasion Under Climate Change
There is a broad consensus that plant invasion will increase, and is already increasing due to climate change (Clements and DiTommaso, 2011; DiTommaso et al., 2014; Varanasi and Jugulam, 2016; Ramesh et al., 2017; Waryszak et al., 2018; Ziska et al., 2019). Three prominent predictions are: (1) poleward spread due to climate warming (Clements and DiTommaso, 2011), (2) range expansion due to changing precipitation regimes (Young et al., 2017), and (3) increased dispersal and establishment due to extreme climate events (Colleran and Goodall, 2015). Below we provide examples of these three predicted consequences of climate change, as well as the potential influence of rapid weed evolution as part of the response.
In terms of poleward spread of weeds due to climate warming, many documented incidences of relatively recent changes in distribution have been reported from North American, European, and Australian temperate regions, as well as in other global regions (Clements et al., 2014). Within North America, spread of numerous weed species northward, including those reaching Canada from the United States has provided abundant evidence of this prominent trend (Clements et al., 2004; Clements and DiTommaso, 2012).
One well-studied example is Sorghum halepense (L.) Pers. (Johnsongrass). Although originally introduced as a perennial C4 grass from areas with Mediterranean climates in Eurasia and Africa (Warwick and Black, 1983), as it moved northward with crops, new ecotypes developed with rhizomes adapted to cold temperatures (Warwick et al., 1986). Additionally, some of the northern ecotypes may overwinter better via an annual life history. With climate change, S. halepense is predicted to spread further northward in North America, causing greater yield loss in Zea mays (corn) crops (McDonald et al., 2009). S. halepense exhibits many other evolutionary adaptations in addition to what we have listed here, enabling continual change of S. halepense populations at the northern edge of its range (Paterson et al., 2020).
Potential expansions in north-south ranges due to climate warming have been studied for a number of weeds that occur in both North America and Europe. For example, Impatiens glandulifera Royle. (Policeman's helmet), an invasive plant frequently infesting riparian areas has been studied in both continents (Kollmann and Bañuelos, 2004; Clements et al., 2008; Clements and DiTommaso, 2012). Presently I. glandulifera is distributed in relatively southerly regions within Canada, but the European research shows how life history traits tend to vary along a latitudinal gradient, thereby raising the possibility if rapid evolution occurred along the northern edge of its range, it could occupy the more latitudes in North America as seen in Europe. Furthermore, recent European research also points to I. glandulifera recently being able to invade forest habitats (Cuda et al., 2020; Gruntman et al., 2020) in addition to its historic spread via waterways, providing further evidence of how its adaptability might work in concert with changing climates.
As well as changes in mean temperatures in temperate regions, extensive changes in regional precipitation patterns are predicted, and have been seen trending in many areas, such as the western United States, which has seen increased frequencies of drought conditions and associated wildfires (Leung et al., 2004; Westerling et al., 2006). In the Western U.S., when modeling changes in weed distribution, precipitation variables are much more important than temperature (Bradley et al., 2009). Centaurea solstitialis L. (yellow starthistle) is a widespread invasive weed in many semi-arid parts of North America with Mediterranean-like climates, first having introduced as a seed contaminant in California in the mid-1800s (Bradley et al., 2009; Young et al., 2017). It has been referred to as California's worst wildland weed (Pitcairn et al., 2006). Summer and spring precipitation and winter and spring minimum temperatures currently constrain the range of C. solstitialis but Bradley et al. (2009) predict climate change involving more frequent droughts but higher levels of spring precipitation will lead to more widespread distribution in the western U.S. Relatively rapid genetic change seen in C. solstitialis via developing herbicide resistance (Sterling et al., 2001) and novel genotypes in different environments (Dlugosch et al., 2015) also predict its ability to take advantage of these changing precipitation regimes quickly (Young et al., 2017). Dukes et al. (2011) showed experimentally that C. solstitialis was able to increase its growth and fecundity more than other plant species with enhanced atmospheric CO2.
There are many other invasive weed species in addition to C. solstitialis poised to take advantage of these changing climate regimes in western North America, with many of them already invading new areas in the wake of increased drought frequency and changing precipitation patterns. These include, but are not limited to Bromus tectorum L. (downy brome) (Harvey et al., 2020), Ventenata dubia (Leers) Coss. (ventenata) (Harvey et al., 2020), Avena barbata Pott ex Link (slender oat) (Nguyen et al., 2016), Bromus madritensis L. (foxtail brome) (Nguyen et al., 2016), and Bassia scoparia (L.) A. J. Scott (kochia) (Chen et al., 2020). However, it should be noted there are also invasive weed species less capable of withstanding drought conditions (Young et al., 2017). Young (2015) recorded an instance when a potential invasion by Carduus nutans L. (musk thistle) in Nebraska locale was halted abruptly by drought conditions that prevented the invading plants from reproducing.
Only recently have researchers turned their attention to the response of invasive weeds to extreme climate events like storms, floods and various other powerful weather events associated with climate change (Young et al., 2017). Flooding events can greatly enhance the spread of invasive weeds already adapted for spreading via seeds or vegetative fragments such as Rosa rugosa Thunb. (Rugosa rose) and Reynoutria spp. (Rouifed et al., 2011; Colleran and Goodall, 2015). A specific instance was observed in 2011, when fragments of Reynoutria japonica Houtt. Ronse Decr. (Japanese knotweed) were distributed widely and established new populations after Tropical Storm Irene impacted Vermont, USA in 2011 (Colleran and Goodall, 2015). Similarly, after Hurricane Sandy went through the New Jersey coast in 2012, an invasive sedge Carex kobomugi Ohwi (Japanese sedge) showed a much higher establishment rate in coastal dunes than the native beach grass Ammophila breviligulata Fernald. (American beachgrass) (Charbonneau et al., 2017). Similarly, a widespread invasive wetland grass in North America, Phragmites australis (Cav.) Trin. ex Steud. (common reed), spreads much more rapidly with increased flood frequency levels, with its seed germination requiring temporary dry shoreline areas (Tougas-Tellier et al., 2015).
These examples of the potential for climate change to increase the distribution of invasive weeds reviewed here are just the tip of the iceberg of known examples, and there are no doubt many other examples of invasions and incipient plant invasions influenced by climate change that have yet to be documented and studied. In this review, we are interested in what this elevated dispersal under climate change may be accompanied by increased evolutionary potential as theorized (Clements and DiTommaso, 2011; Johnson et al., 2019), and a significant aspect of such potential evolutionary malleability are the diverse and unique genetic systems exhibited by invasive weed populations.
Clonality, Plasticity, and Epigenetics as Evolved Traits
When considering the introduction of clonal plants to novel ecosystems, one may think that such species would exhibit low diversity and therefore have low invasive success. For most plant populations, problems such as genetic bottlenecks and founder effects can be detrimental, leading to inbreeding depression and an overall decrease in evolutionary potential (Estoup et al., 2016). However, this idea is based on the understanding that the sole source of heritable variation comes from genes, based on the DNA sequence. In reality, there are other mechanisms of heritable phenotypic variation that are advantageous for invaders with relatively low levels of genetic variation. If the genome of an invasive weed is relatively plastic, and/or there are opportunities for adaptation through mechanisms like clonality or epigenetics, greater invasiveness can be arise under various types of selection pressure, including climate change (Figure 1).
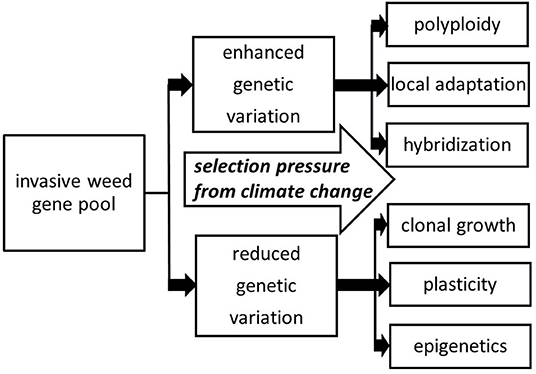
Figure 1. Various directions that selection pressure from climate change can act to build on the invasive weed gene pool, including evolutionary mechanisms involving both increased and decreased genetic variation. By and large, these mechanisms are already operating for a given species prior to encountering climate change stressors, with these mechanisms providing channels for further adaptation.
In a review of 126 invasive plant species in China, researchers were able to demonstrate a significant positive relationship between clonality and invasiveness, indicating that clonality of a species greatly contributed to its invasive success (Liu et al., 2006). A unique advantage of clonal plants is the ability to share water, nutrients, and photosynthates between the individual ramets that make up the clone; this is known as physiological integration (Wang et al., 2020). The relationship between physiological integration and fitness has been studied in both heterogeneous and homogeneous environments, as well as under many environmental stresses. Physiological integration through below-ground rhizomes or stolons allows clonal plants to divide labor, with individual ramets becoming specialized to carry out a specific function within the clone. For example, a study of Carpobrotus edulis (L.) N. E. Br. (Hottentot fig), an aggressive clonal invader, revealed that older ramets became specialized in root production, allocating more biomass below ground than younger ramets, which became specialized in capturing above ground resources, having significantly higher chlorophyll content (Roiloa et al., 2013). Researchers also found that when compared to a clone with severed stolons, an intact clonal invader, Fragaria chiolensis (L.) Duchesne (beach strawberry), had higher overall biomass, greater expansion of stolons, and higher production of new ramets (Roiloa et al., 2010). This demonstrates that clonal division of labor can be advantageous for an invading species, as it directly contributes to the lateral expansion of the clone. This adaptive capability of clonal invaders can be selected for by genotypic selection, which Pan and Price (2002) describe as the selection of genotypically based traits associated with differences in the rate of ramet production. This means that ramets with more advantageous traits can be selected for in many cases. As the climate continues to change, this competitive ability will likely become even more advantageous. It has previously been documented that physiological integration can improve fitness of clones subjected to a variety of environmental stressors, such as nutrient deficiency (D'Hertefeldt et al., 2011), shading (Alpert, 1999), submergence (Luo et al., 2014) and drought (van Kleunen and Stuefer, 1999). These types of stressors, namely drought and flooding, are predicted to become more frequent and severe under climate change; the ability of clonal species to adapt and specialize ramets to overcome these stressors will allow them to continue invasion even under unfavorable conditions.
An additional advantage for clonal and non-clonal invaders alike is high phenotypic plasticity, which can be described as when a particular genotype expresses a variety of phenotypes in response to varying environmental conditions. Having high phenotypic plasticity can be advantageous for weeds if the resulting phenotypes result in an increase in fitness of the plant (van Kleunen and Fischer, 2005). The idea that this phenomenon contributed to plant invasions was proposed by Baker (1965), who posited that when a plant arrives in a novel environment with low genetic diversity, high levels of phenotypic plasticity allow the plant to adapt and eventually become established under the new conditions. As climate change progresses, it is thought that phenotypic plasticity may play a key role in helping plant populations avoid extinction. In recent years, more studies have investigated the relationship between climate change and plastic responses. In a recent review (Franks et al., 2014), 29 studies examining plastic responses of plants to climate change were analyzed, and it was found that all 29 studies demonstrated evidence of plastic responses to climate change, indicating that these types of responses can occur. However, despite adaptations to climate change occurring, the response may not be enough to keep up with the rapidly changing climate. In a review of 12 studies that investigated this, 8 found that the observed responses to changing conditions would not be sufficient to ensure survival of the population, given the predicted future climate scenarios (Franks et al., 2014).
The types of phenotypic responses to climate change are variable, including shifting flowering times, changes in biomass, stem count, or leaf size, or changes in reproduction. For example, a study of Boechera stricta (Graham) Al-Shehbaz (Drummond's rockcress) compared recently planted individuals to historical data of flowering time of the species in the region and found that it had shifted significantly, by 0.34 days between 1973 and 2011 (Anderson et al., 2012). Through genetic analysis, it was found that directional selection favored earlier flowering due to warming temperatures, and the researchers concluded that plasticity was an important driver of this shift (Anderson et al., 2012). In a study of the global weed Alternanthera philoxeroides (Mart.) Griseb. (alligator weed), which invades both terrestrial and aquatic environments, samples were taken in the species invasive ranges, USA and China, where it relies mainly on clonal reproduction and thus has lower genetic diversity, and in its native range in Argentina (Geng et al., 2007). It was therefore predicted that phenotypic plasticity in response to different water availability would be higher in the introduced ranges where genetic diversity was thought to be lower. However, researchers observed significant phenotypic plasticity responses in all three areas; in terrestrial environments, A. philoxeroides allocated more biomass to underground root formation, while producing smaller leaves and internodes to help balance water absorption and transpiration. In aquatic environments, A. philoxeroides stem pith cavities were larger, allowing them to float on the water surface in mats. Contrary to what was expected, the plasticity responses of the invasive populations fell within the plasticity response range of the native populations, meaning some of the native clones had higher plasticity than their invasive counterparts (Geng et al., 2007). However, according to a recent meta-analysis, it is thought that invasive populations of a species generally have higher plasticity than those in the native range (Davidson et al., 2011).
In addition to phenotypic plasticity, many introduced plants have the ability to respond quickly to changes in environmental conditions through epigenetic modification, which is the alteration of chromatin without changing the DNA sequence (Jones, 2012). Epigenetic modifications usually occur in the form of histone variants, histone modifications, and DNA methylation; these change the higher order chromatin structure, resulting in a change in gene expression such as altering transcription (Asensi-Fabado et al., 2017). These epigenetic modifications are often the result of changing environmental conditions, such as those in novel environments for introduced plants, or due to climate change. Epigenetic mutations have been documented in response to a variety of environmental conditions, including flooding, drought, shading, salinity, and temperature stress (Asensi-Fabado et al., 2017). For example, after several hours of drought stress, acetylation of Arabidopsis histones resulted in a drastic increase in transcription of drought-response genes (Kim et al., 2012). The majority of research indicates that epigenetic modifications constitute an intermediate method of rapid evolution for invasive species, as most stress-related modifications are only observed in chromatin until the stress exposure ends, then reverting back to their previous state (Pecinka and Scheid, 2012). Like DNA mutations, epigenetic mutations have the potential to be selected for if they are advantageous, so long as the stressor causing the mutation persists. This is especially advantageous for clonal invaders, which generally have lower genetic diversity, as well as for populations experiencing founder effects or bottlenecks. More recently, emerging evidence suggests that some modifications can be passed down even to non-stressed progeny through a “memory” of the stressed state (Pecinka and Scheid, 2012).
With the continued change in climate, adaptation to changing environmental conditions will likely become imperative for invasive species to thrive. Populations will begin to experience novel climates without expanding their geographic range, including more extreme temperatures as well as more frequent and severe storms. Rapid evolution through epigenetic modification, phenotypic plasticity, and clonality will continue to be used by successful invading plants of the future.
Purging Genetic Material During Invasion
In general, it can be said that inbreeding reduces the fitness of populations, as it decreases heterozygosity. Inbreeding also results in the expression of genetic load, which is the accumulation of deleterious recessive alleles which can reduce growth and expansion of a population (Keller and Waller, 2002). Small edge and founding populations are especially at risk of genetic loads, as they tend to have significantly lower genetic diversity due to drift. However, it has been observed that introduced species often become aggressive invaders despite population bottlenecks, which usually lead to inbreeding depression and genetic load expression (Estoup et al., 2016). This phenomenon has been coined the “genetic paradox” of invasion, where the anticipated negative effects of inbreeding within a limited population are not observed (Allendorf and Lundquist, 2003). Previous research of wild native plant populations revealed that at range edges, the effects of inbreeding depression were decreased, indicating that purging of the genetic load had occurred (Pujol et al., 2009; Barringer et al., 2012). Genetic purging can be described as a process where genetic load of deleterious alleles is reduced as those alleles are selected against (Barringer et al., 2012).
In order to determine whether genetic purging occurs in expanding invasive populations, a study of a recent North American invader, Brachypodium sylvaticum (Huds.) P. Beauv. (slender false brome) was conducted by Marchini et al. (2015). The researchers hypothesized that intermittent gene flow to a small, isolated, inbreeding population would allow for selection of high fitness alleles at loci that had previously been fixed for deleterious mutations. Limited gene flow, occurring by outcrossing once every few generations with another population, is reflective of an invasive species slowly expanding its range (Marchini et al., 2015). The study determined that genetic purging removed deleterious mutations in populations that were primarily inbreeding with periodic gene flow, as opposed to populations that were outcrossing or inbreeding only. The level of purging was variable, depending on the size of outcrossing populations, the number of deleterious loci, and the initial diversity levels throughout the metapopulation. Because the level of genetic purging is dependent on initial genetic diversity, invasive species that have higher diversity, such as those which have had multiple introductions to the invasive region, would likely exhibit higher levels of purging. In contrast, a stable metapopulation of a native species would be more likely to have populations from single origins, in turn having lower diversity and higher relatedness, which increases the likelihood of fixation of deleterious alleles. Purging levels within populations was found to be highest when outcrossed among populations every 5 generations and the number of affected loci was low; in this scenario, 90% of loci became fixed for homozygous “normal” alleles, and no loci were fixed for deleterious alleles (Marchini et al., 2015). Interestingly, the authors also found that frequency of homozygous “normal” alleles was significantly higher in an inbreeding metapopulation with gene flow, which had a frequency of over 90% after 100 generations, compared to a single large outcrossing population, where frequency was only around 65%. This confirms the hypothesis that inbreeding with periodic outbreeding can purge genetic loads, increasing fitness of populations at range edges after relatively few generations. Continued range expansion of many invasive species is anticipated under climate change; this will likely facilitate the generation of new selfing lineages due to the purging of genetic loads.
Hybridization, Polyploidy, and Rapid Evolution
The counterintuitive result of increased invasiveness of invasive weeds, even if genetic variation is relatively low, clearly operates in ecosystems subject to changing climate, as described previously. However, enhanced genetic variation is also an effective means to fuel rapid evolutionary change as natural selection generates novel, locally adapted genotypes, a process frequently accompanied by other mechanisms like hybridization and polyploidy in invasive plants (Figure 1).
Interspecific hybridization results in new genetic combinations that can be acted upon by natural selection and is a well-used mechanism of adaptive rapid evolution, especially in invasive plants. It provides a way for genetically impoverished species, such as clonal organisms and populations experiencing bottlenecks or founder effects, to increase their genetic diversity and overall fitness. Hybridization increases fitness through the generation of “extreme” novel phenotypes, as two genetically distinct parent species reproduce, recombining their alleles (Kagawa and Takimoto, 2018). The resultant hybrid often exhibits improved biological function compared to either parent species; this concept is known as heterosis, or hybrid vigor. Hybrids will generally be larger in size, more fecund, and more display greater growth levels when compared to the parent species. In systemic review of invasive hybrids compared to their parent taxa, Hovick et al. (2014) found that the hybrids were generally larger, more fertile, and more invasive than their parents. In plants, hybridization is often associated with invasion; many invasive plant systems support the hybridization-invasion hypothesis, which states that interspecific hybridization promotes invasiveness (Hovick et al., 2014). These systems are also often associated with polyploidy, with 63% of the hybrids reviewed reported as polyploid, and of those, nearly 50% had increased ploidy compared to their parents (Hovick et al., 2014). A systemic review of 309 invasive plants and 112 rare endemics by Dar et al. (2020) revealed that invasive species were also more likely to be polyploid than native species, and also showed that the proportion of polyploids increases with more advanced invasion. In many instances, the effects of hybridization and polyploidy in invasive plants are closely linked, and it can be difficult to tell which, or both, has the most influence on invasiveness.
Polyploidy, also known as whole genome duplication (WGD), resulting in more than two sets of chromosomes in the genome, is a common process in plants, suggesting some kind of evolutionary advantage (Chen, 2007). In plants, it is usually associated with tolerance to a broad range of ecological conditions and has also been linked to higher levels of asexual reproduction, an increased resistance to pathogens, and changes to seed germination and dormancy (Dar et al., 2020). These characteristics, along with an increase in genetic diversity, can greatly influence the fitness of polyploid invaders. Polyploid organisms can further be broken down into two categories: autopolyploids and allopolyploids. An autopolyploid is the result of doubling a diploid genome, while an allopolyploid is the result of two distinct genomes combining; allopolyploidy occurs by interspecific hybridization followed by either chromosome doubling or fusing of gametes, or by interspecific hybridization of two tetraploids (Estep et al., 2014). Allopolyploids therefore have the combined positive benefits of heterosis and polyploidy, usually exhibiting higher fertility, genetic diversity, and phenotypic plasticity (Estep et al., 2014). Knowing that polyploid species have favorable characteristics such as heterosis, gene redundancy, and high genetic variability, one may hypothesize polyploids are better weeds than diploids. In general, polyploids have a higher invasive capacity than diploids; polyploids have been shown to have larger seeds that are less likely to germinate in unfavorable conditions, have higher fecundity and competitive ability, as well as being less likely to experience inbreeding depression than diploid species (Rutland et al., 2021). However, some of the worst global invaders are diploid, not polyploid; additionally, there are many weeds whose ploidy levels remain unknown, and as a result, large scale sequencing of polyploid weeds needs to be done before conclusions their fitness can be drawn (Rutland et al., 2021).
As mentioned, the processes of hybridization and polyploidy are often linked, especially in the case of allopolyploid species, as both provide benefits to expanding invasive plant populations. One of the interesting ways that polyploidy contributes to fitness of hybrid plant populations is through “pre-adaptation” to new environmental conditions, which assists plants during early establishment (te Beest et al., 2012). For example, in the case of the tetraploid Centaurea stoebe L. (spotted knapweed), widespread invasion throughout the USA is attributed to pre-adaptation of the native tetraploids Europe to a wide range of climates, including extremely dry conditions, compared to diploids in the native range. Despite both the diploid and tetraploid cytotypes being introduced to the USA, invasive populations are dominated by tetraploids (Henery et al., 2010). Additionally, polyploidy and hybridization may increase fitness by “masking” genetic load, rather than purging them, in inbreeding and outcrossing populations. Non-deleterious alleles are usually dominant and can “mask” recessive deleterious alleles. In another study of C. stoebe, it was found that due to the multiple chromosome sets and higher number of alleles per locus, tetraploid populations could mask deleterious alleles much more effectively than diploids, resulting in significantly lower levels of inbreeding depression (Rosche et al., 2017).
Hybrid and polyploid populations are also key components of range expansion, which has been well-documented in the case of the hybrid Reynoutria × bohemica Chrtek and Chrtková (Bohemian knotweed). A shift in habitat preference has been observed in R. × bohemica, as it has been found invading environments that neither parent species usually inhabit. A study by Walls (2010) found abundant populations invading beaches, coastlines, and estuaries, a very different habitat than the ditches, roadsides, and riparian zones where the parent species are usually found. It was found that high phenotypic plasticity of the hybrid allowed the populations to exhibit high drought and salinity tolerance, which is not frequently observed in either parent species. Under continued climate change, it is likely that invasive plant populations will encounter novel environmental conditions and new ecological niches. An example of this can be observed in the genus Spartina, which is comprised of around 13 species, usually occupying salt marsh habitats (Ainouche et al., 2009). This genus has a complex invasion history, with multiple hybridization and polyploidization events that have contributed to its invasive success (Ainouche et al., 2009). In a study of a Spartina allopolyploid, S. maritima × densiflora in the Mediterranean region it was found that increased temperatures during the flowering months (June–August) was positively correlated with greater lateral expansion and increased size compared to S. maritima, S. densiflora, or an S. densiflora x maritima hybrid (Gallego-Tevar et al., 2019). The increase in mean maximum monthly temperature during the study period, which is associated with an increase in flowering time in Spartina, was similar to predicted temperature increases under climate change for the region (Giannakopoulos et al., 2009). Thus, we may speculate that the conditions caused by anthropogenic climate change will increase opportunities for hybridization and polyploidization events in invasive plant populations because of the expected increase in intensity of selection pressure. Given the potential for genetic change already seen in the cases we have reviewed, novel hybrids and polyploids will likely continue to rapidly adapt to evolving climate conditions, making them aggressive competitors and invaders of native habitats.
Evidence of Rapid Evolution on Invasion Edges
Initial incursions of invasive plants are often scattered randomly across a given region. This pattern of initial invasion occurs if, as in most cases, human agency marks the beginning of the invasion. In fact, many plant invasions begin as escapes from cultivation, and may originate at a single location. However, over time an invasion front or edge becomes established, as invasive plant populations expand. For example, in North America for many invasive plant species the invasion front is on the northern edge of ranges of various species (Clements et al., 2004). If these invasive plant populations are somewhat constrained by climatic conditions, further expansion may be limited by climatic conditions at the edge of the range, and if the conditions change, invasive plant ranges predictably expand. Models may be devised to predict such range expansion utilizing data on climate change the species niches (Jiménez-Valverde et al., 2011), but if they fail to account for evolutionary processes at the edges of the range, such models may underestimate the spread of invasive plants (Clements and DiTommaso, 2011). As already described, there are particular mechanisms by which such rapid evolution at the edge of expanding ranges could occur, such as clonality, plasticity, epigenetics, hybridization, polyploidy or purging of genetic material. However, in this section of the review, we summarize case studies providing evidence for potential rapid evolution of invasive plants on invasion edges, regardless of mechanism.
It has been frequently shown that invasive species may evolve new climatic tolerance ranges in their invasive ranges by comparison to their native ranges, although this is not universally true, and in fact a recent analysis of 86 studies examining 434 invasive species concluded the invasive niches are largely conserved (Liu et al., 2020b). Still, the exceptional cases are critical species to monitor. Among the 8 weed species evaluated by Wan et al. (2017) in China, Amaranthus retroflexus L. (redroot pigweed) showed the broadest niche expansion by comparison to its niche in North America (Table 1). A. retroflexus is widespread weed of cropland and other environments throughout the world (Costea et al., 2004), and thus its apparent ability to evolve to new climatic conditions fairly rapidly (Wan et al., 2017) should be monitored closely. As mentioned previously, there is evidence for Ambrosia artemisiifolia expanding its niche breadth throughout the world (Essl et al., 2015; Gallien et al., 2016; Sun and Roderick, 2019; van Boheemen et al., 2019; Sun et al., 2020), including in China where A. artemisiifolia exhibited genetic differences in growth patterns compared to populations in North America where the species is native (Sun and Roderick, 2019; Table 1). Sun and Roderick's (2019) assessment represents evolutionary change in these Chinese populations in <100 years since the introduction of A. artemisiifolia in 1935 (Wan et al., 1993). Because A. artemisiifolia is projected to further expand its range under climate change (Qin et al., 2014), the ability to adapt quickly to different climatic conditions (Sun and Roderick, 2019) signal that this is also an important species to monitor as the climate changes, both for distributional changes and novel genotypes.
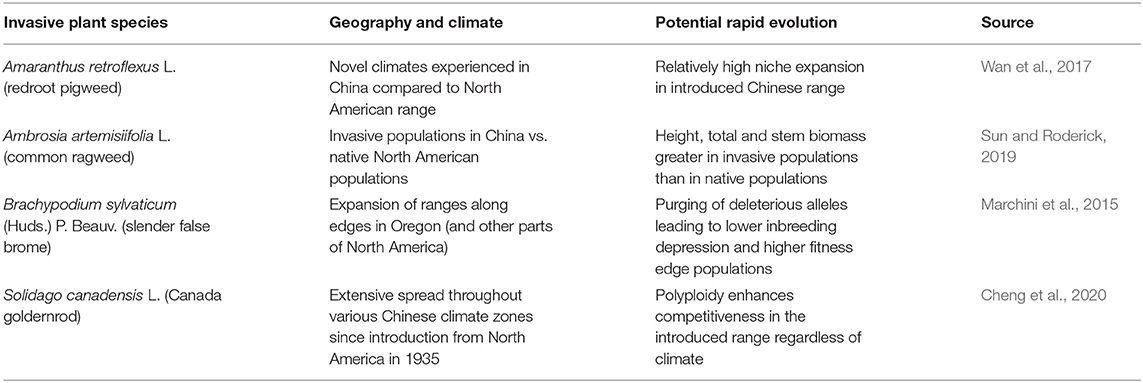
Table 1. Cases demonstrating potential rapid evolution of invasive plants under climate change for species exhibiting general niche expansion in the invaded range.
When considering the question of rapid niche expansion under climate change, as discussed previously it is important to consider unique genetic mechanisms, such as the purging of deleterious alleles seen in Brachypodium sylvaticum (Marchini et al., 2015) and polyploidy in Solidago canadensis (Cheng et al., 2020) (Table 1). The concept of purging of deleterious alleles during invasion is relatively new, and more research needs to be done to investigate the likelihood that other invasive species may employ the same strategy. The role of polyploidy in invasion is well-demonstrated (Estep et al., 2014), and S. canadensis presents a compelling case for how this might influence rapid evolutionary change in a changing climate. Cheng et al. (2020) found that diploid forms of S. canadensis tended to go extinct in their common garden experiment, whereas tetraploids and hexaploids competed well with native vegetation, particularly if they were from the introduced range (hexaploids from the native range eventually failed to outcompete native vegetation), suggesting that rapid evolution in the polyploid forms after introduction to China played a role in successful invasion. Field sampling by Wan et al. (2020) identified all populations of S. canadensis in China they sampled between 2013 and 2016 as hexaploid, in contrast to North American samples which displayed a range of ploidies. In terms of climate change, latitudinal clines in China indicate S. canadensis is adapting to particular climates, and therefore capable of adapting to future climate changes (Li et al., 2016).
As described previously, there is considerable evidence for potential future poleward spread of invasive plants, in addition to observations of latitudinal spread over the previous century due to warming occurring in temperate regions, and furthermore there is evidence for a role of rapid evolution in accelerating this movement (Table 2). Similar to trends seen for many Angiosperm species, native or non-native, Dittrichia graveolens (L.) Greuter (stinkwort) has exhibited recent genetic changes in flowering phenology across a north-south European gradient (Lustenhouwer et al., 2018). The same pattern and ability to adapt flowering phenology was seen in I. glandulifera in Europe (Kollmann and Bañuelos, 2004). Because I. glandulifera also invades over a similarly broad latitudinal range in North America (Clements et al., 2008), it likely forms a similar genetic gradient on the North American continent. Genetic variation in I. glandulifera populations is derived from outcrossing, whereas other species like S. halepense although mostly selfing derives some of its genetic diversity through introgression with the crop sorghum [Sorghum bicolor (L.) Moench] (Warwick et al., 1984; Clements and DiTommaso, 2012). Indeed, the evolutionary novelty allowing S. halepense to colonize more northerly environments in North America has been attributed to this genetic diversity. Its rhizomes have evolved cold tolerance, even though when it was introduced in the early 1800s it was a C4 grass adapted only to the warmer climates consistent with its origin in western Asia (McWhorter, 1971; Paterson et al., 2020). Furthermore, northern populations may exhibit an annual life history, allowing S. halepense to overwinter more readily along the northern edge of its range (Warwick et al., 1986). The adaptation of S. halepense to both warm and cooler climates illustrates its striking ability to adapt to a range of climates, and thus the species is poised to benefit from climatic changes across its six continent distribution.
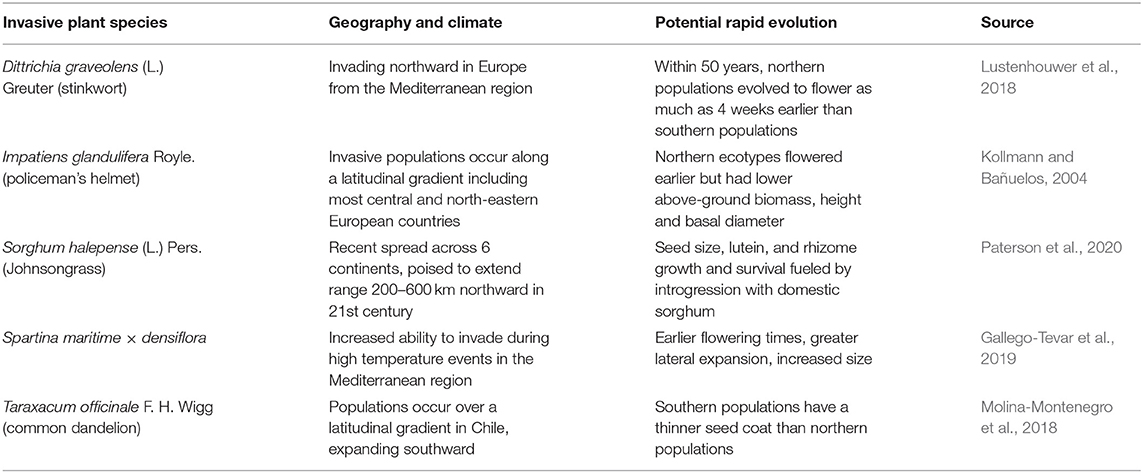
Table 2. Cases demonstrating potential rapid evolution of invasive plants under climate change for species evolving along latitudinal gradients.
The potential for adaptation by Spartina maritima × densiflora to novel environments, including warmer climates, stem from greater genetic variability achieved through hybridization (Gallego-Tevar et al., 2019; Table 2). As detailed previously, S. maritima × densiflora is part of a complex of Spartina species which hybridize extensively, providing a much greater range of genetic material than in the gene pool of a single species. Ironically, the parental species Spartina maritima (M. A. Curtis) Fernald is relatively uncommon and with increased warming in Europe, S. maritima × densiflora threatens to competitively supplant its parent (Gallego-Tevar et al., 2019).
Taraxacum officinale F. H. Wigg (common dandelion) is well-known for its adaptability the world over, invading urban and agricultural environments. A Chilean study found considerable variation in T. officinale over a north-south latitudinal gradient, with more southern populations featuring thinner seed coats (Molina-Montenegro et al., 2018; Table 2). Despite the dominant mode of asexual reproduction by T. officinale via apomixis, the Chilean study found that there was enough sexual reproduction occurring to create diverse genotypes forming the latitudinal gradient (Molina-Montenegro et al., 2018). As well as the impact of north-south temperature gradient, Molina-Montenegro et al. (2018) attributed the genetic differences in seed coat thickness to moisture patterns, in that in drier conditions, seeds with thicker coats will be tend to germinate only when rainfall is sufficient for growth and development. They pointed out that this adaptive feature may allow T. officinale to adapt to changes in moisture regimes predicted under climate change, as has been shown for many other invasive plant species (Table 3).
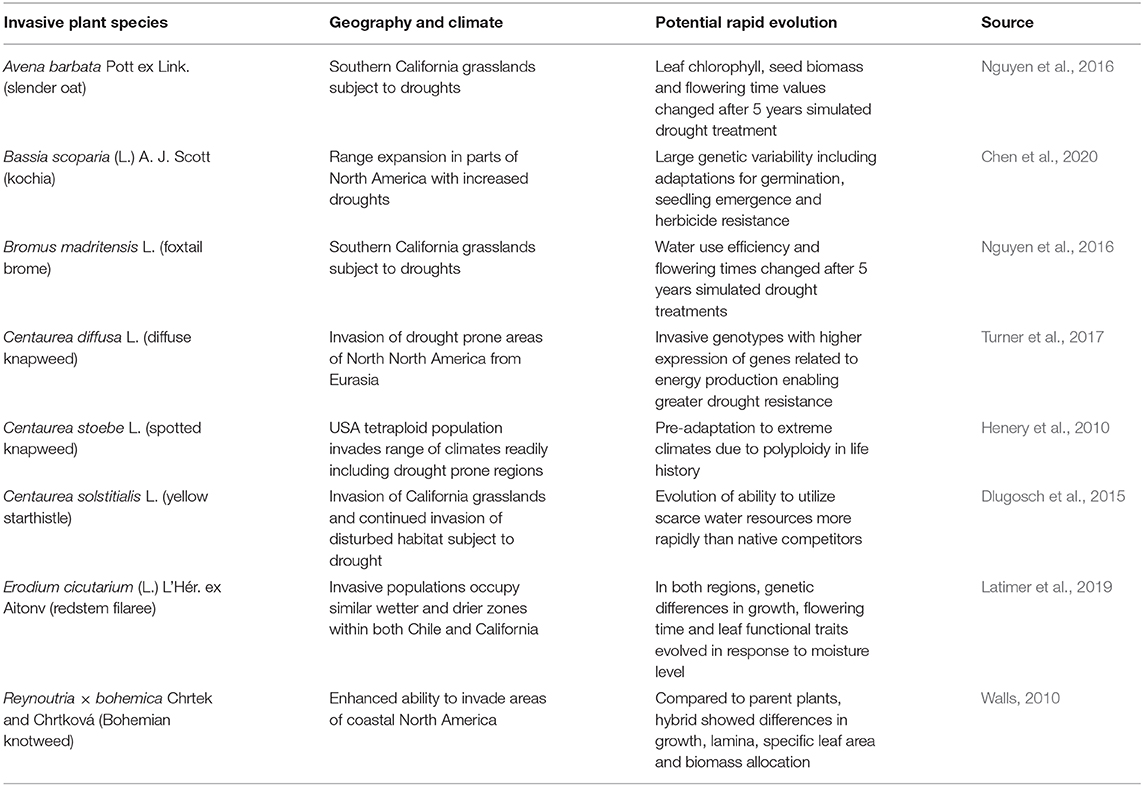
Table 3. Cases demonstrating potential rapid evolution of invasive plants under climate change for species adapting to new moisture regimes in the invaded range.
One particular region that represents changing moisture regimes subject to more frequent droughts under climate change is western USA, including California where a 5-year grassland study showed that two invasive grasses were able to evolve more drought-resistant traits with just 5 years of selection pressure (Nguyen et al., 2016; Table 3). The affected traits were slightly different for Avena barbata by comparison to the other grass species, Bromus madritensis (Table 3), but in both cases the plants subject to more frequent droughts exhibited markedly changed genotypes, illustrating that this kind of evolutionary change can occur over just a few generations (Nguyen et al., 2016).
An important consideration in the rapid evolution of invasive plants to climate change, is the interaction of anthropogenic stressors, i.e., synergism between human-caused climate change and other human habitat modifications favoring invasive species. Potential and observed changes in Bassia scoparia provide clear examples, such as the potential for this widespread invasive plant in western North America to form herbicide resistant genotypes and become more drought-resistant (Chen et al., 2020; Table 3). Similarly, various species of Centaurea introduced from Eurasia are exhibiting evolutionary change in their invaded range in western North America, where they have already been taking advantage of anthropogenic disturbances, such as overgrazing of rangeland by livestock, and now threaten to become even more abundance and widespread as drought frequency increases (Henery et al., 2010; Dlugosch et al., 2015; Turner et al., 2017; Table 3).
The annual forb Erodium cicutarium (L.) L'Hér. ex Aitonv (redstem filaree) is a global invader, having invaded all continents from its native Europe, and reaching both California and Chile some 300 years ago (Mensing and Byrne, 1998; Fiz-Palacios et al., 2010). Latimer et al. (2019) were able to observe a similar pattern of evolutionary adaptation among genotypes in both regions, with traits arising specifically adapted to local moisture conditions. This finding demonstrates the ability of global invasive plants like E. cicutarium to adapt to moisture gradients wherever they are introduced, in a relatively short period of time, presumably mirroring environmental changes expected as the climate continues to change.
An important consideration in understanding probable distribution changes of invasive plants under climate change is the ability to both adapt to novel climatic conditions and also effectively modify their dispersal and establishment abilities. Reynoutria × bohemica exemplifies this important consideration in many different ways (Walls, 2010; Gillies et al., 2016; Table 3). Walls (2010) found R. × bohemica was able to develop traits allowing it to better invade coastal areas in eastern North America. In the next session we focus on rapid evolution within this particular taxa, and implications for management.
Case Study of Rapid Weed Evolution in Reynoutria spp. (Knotweeds)
The hybridization event that has repeatedly created Reynoutria × bohemica (Bohemian knotweed) in different continents, including Europe, North America, Australia and New Zealand (Bailey and Wisskirchen, 2004), has produced a plant with a much more potential for long-distance dispersal than its largely sterile male parent, Reynoutria japonica (Japanese knotweed) (Gillies et al., 2016). Similarly, the fertile female parent, R. sachalinensis (F. Schmidt) Ronse-Decraene (giant knotweed) tends to be less problematic for management, including being less likely to spread vegetatively via rhizomes (Parepa et al., 2014). The importance of this hybridization between Reynoutria species for the invasiveness of knotweed is still unclear because the hybrids have only recently spread and been discovered (Bailey and Wisskirchen, 2004; Gaskin et al., 2014; Parepa et al., 2014; Gillies et al., 2016). It seems clear that the genetically diverse hybrid swarm constituting the R. × bohemica hybrid should enable this invasive weed to adapt more rapidly to climate change than either parent. There are concerns that the increased diversity could facilitate adaptations such as herbicide resistance (Strelau et al., 2018). The hybrid has frequently been observed forming incursions into crop fields from field edges, and thus could potentially become a serious crop pest in addition to being an invasive weed degrading natural and urban habitats. Extensive dispersal via agricultural drainage ditches is on the increase in coastal British Columbia, Canada (Clements and Jones, pers. Comm.). Parepa et al. (2014) argued that because the hybrid species was clearly the most variable among various European populations sampled from the three species, it was more competitive than its parents and similar trends have been seen in North America (Gaskin et al., 2014; Clements et al., 2016; Gillies et al., 2016). Epigenetic mechanisms are also involved in the specialized adaptations of Reynoutria spp., even the sterile R. japonica, and Parepa et al. (2014) predicted that such epigenetic mechanisms will be even more effective in facilitating local adaptations in genetically diverse R. × bohemica populations.
In their combined invasive and native ranges, Reynoutria spp. encounter a vast range of climates. Generally, Reynoutria spp. prefer a temperate or continental climate, and thrive in areas with >500 mm of annual precipitation. Beerling (1993) identified an annual threshold of 2,505 degree days and minimum temperature of −30.2°C for knotweed establishment. Young shoots are susceptible to frost in the late spring and early fall, which can cause dieback followed by new growth from the rhizome when conditions are favorable, as the rhizomes can survive temperatures well below freezing (Sołtysiak, 2020). As the global climate continues to warm, an expansion in the range of all three knotweed species is anticipated. Bourchier and Van Hezewijk (2010) modeled suitable habitat for R. japonica in British Columbia, Canada and found that 12.3% of the province was suitable based on precipitation, minimum temperature and degree day thresholds. At that time, only about half of the suitable area was inhabited with knotweed. The modeling was repeated in Ontario, Canada and found that 35% of the province was suitable habitat for R. japonica; however, when the climate thresholds were changed from normal values between 1971 and 2000 to data from 2000 to 2008, the suitable habitat increased to 53%. This indicates that northward expansion will likely occur as the climate continues to gradually warm. In fact, Reynoutria spp. populations at the northern edge of their ranges in Quebec, Canada have already been observed to exhibit increased flowering and seed germination associated with climate change (Groeneveld et al., 2014). Even the less invasive R. sachalinensis was predicted to invade the relatively cool Canadian province of Alberta under future climatic conditions (Chai et al., 2016). As previously mentioned, clonal plants like Reynoutria spp. often have high phenotypic plasticity, which will likely allow the plant to adapt over time to the temperature increases brought by climate change. The high-fitness progeny of R. × bohemica will also likely aid in producing knotweed genotypes that are well-suited to novel climates.
In their study, Groeneveld et al. (2014) examined the potential for Reynoutria spp. to extend their range northward in Quebec, Canada, through looking at genetic structure and seed production in Quebec. The ability of R. × bohemica to produce seeds 500 km north of the formerly reported limit was taken as a harbinger of northward movement, especially because in their study the more genetically diverse R. × bohemica tended to produce adventive new patches in adventive locations than R. japonica. Note R. japonica did produce viable seeds in their region, but with less genetic variability. Furthermore, the fact that R. × bohemica was rare in the region until recently was another indication that evolutionary change was pushing the boundaries for Reynoutria spp. in Eastern Canada, just as average temperatures were reaching historic highs.
Climate change involves more than simply changes in precipitation and temperature, and the increase in storm frequency and severity has already been demonstrated as a potential means to further spread the rhizomes of R. japonica (Colleran and Goodall, 2015) and by extension, the hybrid R. × bohemica. Increased weather severity will no doubt increase dispersal of the seeds of R. × bohemica as well, as the seeds are capable of surviving many days floating in water, and germinate at higher rates following periods of immersion (Rouifed et al., 2011). Given the ability of seeds to float for days and therefore be transported many kilometers down river, dispersal of seeds by hydrochory may provide powerful selection pressure for R. × bohemica to changing hydrological regimes involving more flooding, especially considering that the seeds and/or vegetative fragments must both be carried down river and deposited on shore at some point. A Quebec study of Reynoutria spp. populations distributed along the 185 km Chaudière River found widespread populations but with limited genetic variation (only one genotype each for R. japonica and for R. × bohemica) indicating that most of the spread in this case was by rhizome and stem fragments (Duquette et al., 2016). Nonetheless the massive invasion along the river with few riparian species able to outcompete the invasive weed.
Given the expected changes in climate involving increases in the growing season in temperate zones, annual temperatures, annual precipitation, and extreme climate events such as flooding it is expected that Reynoutria spp. will only continue to pose problems and extend its range in North America, Europe, Australia and Asia. Looking at the six major mechanisms promoting rapid evolution in invasive weeds (Figure 1), Reynoutria spp. exhibit all six: polyploidy, local adaptation, hybridization, clonal growth, plasticity, and epigenetics. As some of the studies reviewed here indicate, Reynoutria spp. deploy these mechanisms in different ways under different environmental circumstances often resulting in better adaptation than any neighboring native vegetation (Parepa et al., 2014; Duquette et al., 2016; Gillies et al., 2016). As the climate changes, more habitat and more pathways (e.g., by flooding and other extreme events) will become available for Reynoutria spp. to establish. Proactive management measures should include monitoring disturbed areas for knotweed plant parts, including landscapes subsequent to extreme climate events (Colleran and Goodall, 2015), and managing incipient infestations as quickly as possible, given the tremendous dispersal and persistence capabilities of this plant taxon.
Future Research Needs
Research on this potential for rapid evolution is critical to developing more proactive management approaches that anticipate new invasive plant ecotypes adapted to changing climatic conditions. Clearly, research on weed evolution in response to climate change has advanced considerably over the last several decades, but there are still many questions to be explored more fully if we are to approach the goal of devising more proactive management strategies.
Because invasive weed life histories are so extremely diverse, along with the associated reproductive characteristics and genetics (Barrett et al., 2008), there are numerous questions regarding the implications of this diverse array of life histories under climate change. Even within individual weed species, there can be multiple life history strategies at play, such as for R. × bohemica (Gillies et al., 2016) or Sorghum halepense (Paterson et al., 2020). New methods of genetic analysis, including even whole-genome characterizations offer promising possibilities for understanding weed life histories better (e.g., see Liu et al., 2020a). Yet classic evolutionary ecology approaches, as seen in many of the studies reviewed here, still offer important ways of unveiling impacts of climate change on weed life history and adaptation, such as common garden experiments, reciprocal transplants or artificial selection (Colautti and Lau, 2015).
In the face of the numerous factors at play, frameworks have been developed to help cut through the complexity. Young et al. (2017) presented a framework for studying invasive plant dynamics aimed at establishing research priorities, revealing gaps in theoretical understandings, and identifying components of invasion processes that could be used to improve management, i.e., get to the point of a more proactive approach in the case of managing invasive plants in the face of climate change. Their framework revolves around three key components: climate dynamics, invader fitness, and ecosystem resistance.
The first element of the framework, climate dynamics, potentially influences weed invasions in a variety of ways, because climate change itself has multiple aspects. Initially most studies of climate change and invasive weeds were focused on temperature changes and resultant poleward extension of invasive weed distributions (Clements et al., 2004), and there is still a need to investigate these dynamics as the mean global temperature inexorably increases. However, it is evident from the frequent focus on the response of invasive weeds to changes in drought frequency and extent (Table 3) that the impact of anticipated changes in moisture regimes under climate change on rapid weed evolution is an important research area. Furthermore, climate dynamics under climate change also include more frequent floods, fires, storms and other extreme events (Tamarin-Brodsky and Kaspi, 2017; Patricola and Wehner, 2018) and there is an urgent need for more research on such extreme events, which potentially create even greater selection pressures than more gradual changes in climate.
A challenging area of research on climate dynamics in relations to invasive weeds, is to research the problem on a global level; climate change itself is a global phenomenon and many invasive plants have likewise “gone global.” Thus, there is a need for more collaboration among invasive plant specialists from different world regions. For example, more studies like the study we reviewed on E. cicutarium which compared its invasion of both California and Chile (Latimer et al., 2019). A series on the biology of invasive plants was recently initiated in the journal Invasive Plant Science and Management which encourages the formation of multi-national author teams from far-flung world regions, in order to adequately review the biology of invasive plants in a global context (Kriticos et al., 2020). Organizations which bring together invasive plant specialists from across the globe, such as EMAPi likewise have a vital role to play in understanding whether the dynamics of invasion by the same species differ in different world regions (Pyšek et al., 2019).
The second element of the invasive plant research framework (Young et al., 2017), invader fitness, similarly involves many important research questions that have only emerged recently, especially when considering the many novel mechanisms that have recently been explored for invasive weeds such as polyploidy, local adaptation, hybridization, clonal growth, plasticity, epigenetics, and hybridization (Figure 1). Barrett et al. (2008) pointed out that little research had been conducted on potential changes in plant reproductive systems such as these during invasion. More detailed research on these mechanisms is imperative to grasping the way future invasions under climate change might look, including which conditions would favor reduced or increased genetic diversity, and whether purging genetic diversity (cf. Marchini et al., 2015) is a common element in plant invasions. Rutland et al. (2021) provide a comprehensive overview of what is known of the evolution of polyploidy in weeds, but make it clear that more research is needed. They maintain that it is impossible to definitively answer the question, “Do polyploids make better weeds?” at this juncture because of our lack of understanding of weed genomics. The evolution of clonality, including the ongoing evolution at present may be critical to understanding dispersal dynamics of species like Reynoutria × bohemica which spreads by both seed dispersal and clonal growth (Gillies et al., 2016). Williams et al. (2019) suggest ways of researching the eco-evolutionary dynamics of range expansion by systematically looking at the factors that work to generate genetic variance, to determine more clearly how processes effect the degree to which weeds evolve at the edge of their ranges. At the core of invader fitness are the particular traits that may evolve, of which there are many, but the goal should be to demonstrate the actual mechanisms involved utilizing the unique features of invasive species (Hodgins et al., 2018). To this end, van Kleunen et al. (2018) offer some useful ways forward, including synergism between molecular and phenotypic research.
The new molecular techniques referred to by van Kleunen et al. (2018) offer exciting avenues for researching the important questions we have reviewed, such as the relationship between polyploidy and hybridization, epigenetic response dynamics, and the evolution of phenotypic plasticity. In most cases we have clues from certain well-studied invasive species like Alternanthera philoxeroides, Solidago canadensis, Sorghum halepense, Reynoutria spp., and Ambrosia artemisiifolia but as indicated by these species, every invasive weed is different, and differences in life history have implications for adaptation to climate change (Clements and DiTommaso, 2012). One particular examples that illustrates the gap is that of Tragopogon hybrids in North America; although hybridization leading to the formation of new hybrid species is well-studied in Washington State (Clements et al., 1999), no research has been done in Canada on hybridization and its implications under climate change. In sum, there is much more that we do not know about invader fitness under climate change than we do know; as we speculated earlier, the conditions caused by anthropogenic climate change may increase opportunities for hybridization and polyploidization events in invasive plant populations, but this is only speculation without supporting research.
Ecosystem resistance, the third element of the invasive plant research framework (Young et al., 2017), is subject to wide variation in the face of climate change, such as the vulnerability of drought prone areas in the western USA to invasion and concomitant evolution of plant invaders taking advantage of low levels of ecosystem resistance (Table 3). Research on particular species like Sorghum halepense (Paterson et al., 2020) and Impatiens glandulfera (Cuda et al., 2020; Gruntman et al., 2020) indicates that invasive plants may invade new habitats under climate change, raising the alarm that other invasive plants may adapt to new habitats as climate changes. This presents a clear challenge to developing proactive management systems; as well as the need to understand and monitor the formation of novel invasive weed ecotypes, there is the need to manage ecosystems for greater resistance to invasion. Climate modeling approaches for invasive plants to this point have largely relied on large-scale geographic inputs (e.g., regional, national or international scales), but ecosystem resistance tends to act at local levels, and thus there is a need to research the potential for finer-scale modeling (Bradley, 2016; De Kort et al., 2020). Weed researchers conducting a horizon scan of weed research priorities identified the underlying challenges largely related to combining agroecological, socio-economic and technological approaches (Neve et al., 2018). Climate change, weed evolution and weed invasiveness were seen as important themes to pursue.
Certainly the ever clearer link between climate change and weed evolution and invasiveness calls for creative research approaches leading to creative solutions to this problem that is both on the horizon, and already upon us. As our focus on the case of Reynoutria spp. indicates, more diligent monitoring and characterization of the genetic characteristics of invasive weed populations is needed, and along with that, more attention paid to mechanisms for producing novel genotypes (Figure 1), especially at the edges of species ranges where expansion due to changes in climate are likely to occur, and are already occurring for many species in many regions.
Author Contributions
All authors contributed substantially to the literature review, analysis and writing, and approved the final version.
Conflict of Interest
The authors declare that the research was conducted in the absence of any commercial or financial relationships that could be construed as a potential conflict of interest.
References
Ainouche, M. L., Ainouche, M. L., Fortune, P. M., Grandbastien, M. A., Fukunaga, K., Ricou, M., et al. (2009). Hybridization, polyploidy and invasion: lessons from Spartina (Poaceae). Biol. Invasions 11, 1159–1173. doi: 10.1007/s10530-008-9383-2
Allendorf, F. W., and Lundquist, L. L. (2003). Introduction: population biology, evolution, and control of invasive species. Conserv. Biol. 17, 24–30. doi: 10.1046/j.1523-1739.2003.02365.x
Alpert, P. (1999). Clonal Integration in Fragaria chiloensis differs between populations: ramets from grassland are selfish. Oecologia 120, 69–76. doi: 10.1007/s004420050834
Alphen, V. (1999). Can sympatric speciation by disruptive sexual selection explain rapid evolution of cichlid diversity in Lake Victoria?. Ecol. Lett. 2, 262–271. doi: 10.1046/j.1461-0248.1999.00082.x
Anderson, J. T., Inouye, D. W., McKinney, A. M., Colautti, R. I., and Mitchell-Olds, T. (2012). Phenotypic plasticity and adaptive evolution contribute to advancing flowering phenology in response to climate change. Proc. Royal Soc. B 279, 3843–3852. doi: 10.1098/rspb.2012.1051
Asensi-Fabado, M. A., Amtmann, A., and Perrella, G. (2017). Plant responses to abiotic stress: the chromatin context of transcriptional regulation. Biochim. Biophys. Acta Gene. Regul. Mech. 1860, 106–122. doi: 10.1016/j.bbagrm.2016.07.015
Bailey, J., and Wisskirchen, R. (2004). The distribution and origins of Fallopia× bohemica (Polygonaceae) in Europe. Nord. J. Bot. 24, 173–199. doi: 10.1111/j.1756-1051.2004.tb00832.x
Baker, H. G. (1965). “Characteristics and modes of origin of weeds,” in: The Genetics of Colonizing Species, eds H. G. Baker and G. L. Stebbens (New York, NY: Academic Press Inc), 147–172.
Barrett, S. C., Colautti, R. I., and Eckert, C. G. (2008). Plant reproductive systems and evolution during biological invasion. Mol. Ecol. 17, 373–383. doi: 10.1111/j.1365-294X.2007.03503.x
Barrett, S. C. H., Morgan, M. T., and Husband, B. C. (1989). The dissolution of a complex genetic polymorphism: the evolution of self-fertilization in tristylous Eichhornia paniculata. Evolution 43, 1398–1416. doi: 10.1111/j.1558-5646.1989.tb02591.x
Barringer, B. C., Kulka, E. A., and Galloway, L. F. (2012). Reduced inbreeding depression in peripheral relative to central populations of a monocarpic herb: reduced inbreeding depression in peripheral populations. J. Evol.Biol. 25, 1200–1208. doi: 10.1111/j.1420-9101.2012.02510.x
Beerling, D. J. (1993). The impact of temperature on the northern distribution limits of the introduced species Fallopia japonica and Impatiens glandulifera in north-west Europe. J. Biogeogr. 20, 45–53. doi: 10.2307/2845738
Bourchier, R. S., and Van Hezewijk, B. H. (2010). Distribution and potential spread of Japanese knotweed (Polygonum cuspidatum) in Canada relative to climatic thresholds. Invasive Plant Sci. Manag. 3, 32–39. doi: 10.1614/IPSM-09-007.1
Bradley, B. A. (2016). Predicting abundance with presence-only models. Landsc. Ecol. 31, 19–30. doi: 10.1007/s10980-015-0303-4
Bradley, B. A., Oppenheimer, M., and Wilcove, D. S. (2009). Climate change and plant invasions: restoration opportunities ahead?. Glob. Change Biol. 15, 1511–1521. doi: 10.1111/j.1365-2486.2008.01824.x
Chai, S. L., Zhang, J., Nixon, A., and Nielsen, S. (2016). Using risk assessment and habitat suitability models to prioritise invasive species for management in a changing climate. PLoS ONE 11:e0165292. doi: 10.1371/journal.pone.0165292
Charbonneau, B. R., Wootton, L. S., Wnek, J. P., Langley, J. A., and Posner, M. A. (2017). A species effect on storm erosion: Invasive sedge stabilized dunes more than native grass during Hurricane Sandy. J. Appl. Ecol. 54, 1385–1394. doi: 10.1111/1365-2664.12846
Chen, J., Burns, E., Fleming, M., and Patterson, E. (2020). Impact of climate change on population dynamics and herbicide resistance in kochia (Bassia scoparia (L.) AJ Scott). Agron 10:1700. doi: 10.3390/agronomy10111700
Chen, Z. J. (2007). Genetic and epigenetic mechanisms for gene expression and phenotypic variation in plant polyploids. Annu. Rev. Plant Biol. 58, 377–406. doi: 10.1146/annurev.arplant.58.032806.103835
Cheng, J., Yang, X., Xue, L., Yao, B., Lu, H., Tian, Z., et al. (2020). Polyploidization contributes to evolution of competitive ability: a long term common garden study on the invasive Solidago canadensis in China. Oikos 129, 700–713. doi: 10.1111/oik.07095
Clements, D. R. (2017). “Invasive weed species and their effects.” in Integrated Weed Management for Sustainable Agriculture, ed R. Zimdahl (Cambridge, UK: Burleigh Dodds Science Publishing), 65–88.
Clements, D. R., and DiTommaso, A. (2011). Climate change and weed adaptation: can evolution of invasive plants lead to greater range expansion than forecasted? Weed Res. 51, 227–240. doi: 10.1111/j.1365-3180.2011.00850.x
Clements, D. R., and DiTommaso, A. (2012). Predicting weed invasion in Canada under climate change: evaluating evolutionary potential. Can. J. Plant Sci. 92, 1013–1020. doi: 10.4141/cjps2011-280
Clements, D. R., DiTommaso, A., and Hyvönen, T. (2014). “Ecology and management of weeds in a changing climate. Pages 13–37,” in Recent Advances in Weed Management, eds B. S. Chauhan and G. Mahajan (Berlin: Springer), 411.
Clements, D. R., DiTommaso, A., Jordan, N., Booth, B., Murphy, S. D., Cardina, J., et al. (2004). Adaptability of plants invading North American cropland. Agric. Ecosys. Environ. 104, 379–398. doi: 10.1016/j.agee.2004.03.003
Clements, D. R., Feenstra, K. R., Jones, K., and Staniforth, R. (2008). The biology of invasive plants in Canada 9. Impatiens glandulifera Royle. Can. J. Plant Sci. 88, 403–417. doi: 10.4141/CJPS06040
Clements, D. R., Larsen, T., and Grenz, J. (2016). Knotweed management strategies in North America with the advent of widespread hybrid Bohemian knotweed, regional differences, and the potential for biocontrol via the psyllid Aphalara itadori Shinji. Invasive Plant Sci. Manag. 9, 60–70. doi: 10.1614/IPSM-D-15-00047.1
Clements, D. R., Upadhyaya, M. K., and Bos, S. J. (1999). The biology of Canadian weeds. 110. Tragopogon dubius Scop., Tragopogon pratensis L., and Tragopogon porrifolius L. Can. J. Plant Sci. 79, 153–163. doi: 10.4141/P98-007
Colautti, R. I., and Lau, J. A. (2015). Contemporary evolution during invasion: evidence for differentiation, natural selection, and local adaptation. Mole. Ecol. 24, 1999–2017. doi: 10.1111/mec.13162
Colleran, B. P., and Goodall, K. E. (2015). Extending the timeframe for rapid response and best management practices of flood-dispersed Japanese knotweed (Fallopia japonica). Invasive Plant Sci. Manag. 8, 250–254. doi: 10.1614/IPSM-D-14-00046.1
Costea, M., Weaver, S. E., and Tardif, F. J. (2004). The biology of Canadian weeds. 130. Amaranthus retroflexus L., A. powellii S. Watson and A. hybridus L. Can. J. Plant Sci. 84, 631–668. doi: 10.4141/P02-183
Crimmins, T. M., Crimmins, M. A., and Bertelsen, C. D. (2009). Flowering range changes across an elevation gradient in response to warming summer temperatures. Glob. Change Biol. 15, 1141–1152. doi: 10.1111/j.1365-2486.2008.01831.x
Cuda, J., Skálová, H., and Pyšek, P. (2020). Spread of Impatiens glandulifera from riparian habitats to forests and its associated impacts: insights from a new invasion. Weed Res. 60, 8–15. doi: 10.1111/wre.12400
Dar, M. A., Wani, G. A., Reshi, Z. A., Al-Qarawi, A. A., Abd Allah, E. F., and Shah, M. A. (2020). Stage-specific ploidy level variations in invasive species in comparison to rare endemics in Kashmir Himalaya. Flora 262:151525. doi: 10.1016/j.flora.2019.151525
Davidson, A. M., Jennions, M., and Nicotra, A. B. (2011). Do invasive species show higher phenotypic plasticity than native species and, if so, is it adaptive? A meta-analysis. Ecol. Lett. 14, 419–431. doi: 10.1111/j.1461-0248.2011.01596.x
De Kort, H., Baguette, M., Lenoir, J., and Stevens, V. M. (2020). Toward reliable habitat suitability and accessibility models in an era of multiple environmental stressors. Ecol. Evol. 10, 10937–10952. doi: 10.1002/ece3.6753
de Oliveira Xavier, R., Leite, M. B., and da Silva Matos, D. M. (2019). Phenological and reproductive traits and their response to environmental variation differ among native and invasive grasses in a Neotropical savanna. Biol. Invasions 21, 2761–2779. doi: 10.1007/s10530-019-02013-w
D'Hertefeldt, T., Falkengren-Grerup, U., and Jonsdottir, I. S. (2011). Responses to mineral nutrient availability and heterogeneity in physiologically integrated sedges from contrasting habitats. Plant Biol. 13, 48–492. doi: 10.1111/j.1438-8677.2010.00393.x
DiTommaso, A., Qin, Z., and Clements, D. R. (2014). “Identifying climate change as a factor in the establishment and persistence of invasive weeds in agricultural crops,” in Invasive Species and Global Climate Change, eds L. Ziska, and J. S. Dukes (Wallingford, CT: CAB International), 353–370.
Dlugosch, K. M., Cang, F. A., Barker, B. S., Andonian, K., Swope, S. M., and Rieseberg, L. H. (2015). Evolution of invasiveness through increased resource use in a vacant niche. Nat. Plants 1, 1–5. doi: 10.1038/nplants.2015.66
Dukes, J. S., Chiariello, N. R., Loarie, S. R., and Field, C. B. (2011). Strong response of an invasive plant species (Centaurea solstitialis L.) to global environmental changes. Ecol. Appl. 21, 1887–1894. doi: 10.1890/11-0111.1
Duquette, M. C., Compérot, A., Hayes, L F., Pagola, C., Belzile, F., Dubé, J., et al. (2016). From the source to the outlet: understanding the distribution of invasive knotweeds along a North American river. River Res. Appl. 32, 958–966. doi: 10.1002/rra.2914
Essl, F., Biró, K., Brandes, D., Broennimann, O., Bullock, J. M., Chapman, D. S., et al. (2015). Biological flora of the British Isles: Ambrosia artemisiifolia. J. Ecol. 103, 1069–1098. doi: 10.1111/1365-2745.12424
Estep, M. C., McKain, M. R., Diaz, D. V., Zhong, J., Hodge, J. G., Hodkinson, T. F., et al. (2014). Allopolyploidy, diversification, and the Miocene grassland expansion. PNAS 111, 15149–15154. doi: 10.1073/pnas.1404177111
Estoup, A., Ravigné, V., Hufbauer, R., Vitalis, R., Gautier, M., and Facon, B. (2016). Is there a genetic paradox of biological invasion? Annu. Rev. Ecol. Evol. Syst. 47, 51–72. doi: 10.1146/annurev-ecolsys-121415-032116
Fiz-Palacios, O., Vargas, P., Vila, R., Papadopulos, A. S., and Aldasoro, J. J. (2010). The uneven phylogeny and biogeography of Erodium (Geraniaceae): radiations in the Mediterranean and recent recurrent intercontinental colonization. Ann. Bot. 106, 871–884. doi: 10.1093/aob/mcq184
Franks, S. J., Weber, J. J., and Aitken, S. N. (2014). Evolutionary and plastic responses to climate change in terrestrial plant populations. Evol. Appl. 7, 123–139. doi: 10.1111/eva.12112
Gallego-Tevar, B., Infante-Izquierdo, M. D., Figueroa, E., Nieva, F. J. J., Muñoz-Rodríguez, A. F., Grewell, B. J., et al. (2019). Some like it hot: maternal-switching with climate change modifies formation of invasive Spartina hybrids. Front. Plant. Sci. 10:484. doi: 10.3389/fpls.2019.00484
Gallien, L., Thuiller, W., Fort, N., Boleda, M., Alberto, F. J., Rioux, D., et al. (2016). Is there any evidence for rapid, genetically-based, climatic niche expansion in the invasive common ragweed?. PLoS ONE 11:e0152867. doi: 10.1371/journal.pone.0152867
Gaskin, J. F., Schwarzländer, M., Grevstad, F. S., Haverhals, M. A., Bourchier, R. S., and Miller, T. W. (2014). Extreme differences in population structure and genetic diversity for three invasive congeners: knotweeds in western North America. Biol. Invasions 16, 2127–2136. doi: 10.1007/s10530-014-0652-y
Geng, Y. P., Pan, X. Y., Xu, C. Y., Zhang, W. J., Li, B., Chen, J. K., et al. (2007). Phenotypic plasticity rather than locally adapted ecotypes allows the invasive alligator weed to colonize a wide range of habitats. Biol. Invasions 9, 245–256. doi: 10.1007/s10530-006-9029-1
Giannakopoulos, C., Le Sager, P., Bindi, M., Moriondo, M., Kostopoulou, E., and Goodess, C. M. (2009). Climatic changes and associated impacts in the Mediterranean resulting from a 2°C global warming. Glob. Plan. Change. 68, 209–224. doi: 10.1016/j.gloplacha.2009.06.001
Gillies, S., Clements, D. R., and Grenz, J. (2016). Knotweed (Fallopia sp.) invasion of North America utilizes hybridization, epigenetics, seed dispersal (unexpectedly) and an arsenal of physiological tactics. Invasive Plant Sci. Manag. 9, 71–80. doi: 10.1614/IPSM-D-15-00039.1
Gould, S. J., and Eldredge, N. (1977). Punctuated equilibria: the tempo and mode of evolution reconsidered. Paleobiology 3, 115–151. doi: 10.1017/S0094837300005224
Groeneveld, E., Belzile, F., and Lavoie, C. (2014). Sexual reproduction of Japanese knotweed (Fallopia japonica sl) at its northern distribution limit: new evidence of the effect of climate warming on an invasive species. Ame. J. Bot. 101, 459–466. doi: 10.3732/ajb.1300386
Gruntman, M., Segev, U., and Tielbörger, K. (2020). Shade-induced plasticity in invasive Impatiens glandulifera populations. Weed Res. 60, 16–25. doi: 10.1111/wre.12394
Hairston, N. G. Jr, Ellner, S. P., Geber, M. A., Yoshida, T., and Fox, J. A. (2005). Rapid evolution and the convergence of ecological and evolutionary time. Ecol. Lett. 8, 1114–1127. doi: 10.1111/j.1461-0248.2005.00812.x
Harvey, A. J., Rew, L. J., Prather, T. S., and Mangold, J. M. (2020). Effects of elevated temperature and CO2 concentration on seedling growth of Ventenata dubia (Leers) Coss. and Bromus tectorum L. Agron 10:1718. doi: 10.3390/agronomy10111718
Henery, M. L., Bowman, G., Mraz, P., Treier, U. A., Gex-Fabry, E., Schaffner, U., et al. (2010). Evidence for a combination of pre-adapted traits and rapid adaptive change in the invasive plant Centaurea stoebe. J. Ecol. 98, 800–813. doi: 10.1111/j.1365-2745.2010.01672.x
Hodgins, K. A., Bock, D. G., and Rieseberg, L. H. (2018). Trait evolution in invasive species. Annu. Rev. Plant Biol. 1, 459–496. doi: 10.1002/9781119312994.apr0643
Hovick, S. M., Whitney, K. D., and Gurevitch, J. (2014). Hybridisation is associated with increased fecundity and size in invasive taxa: meta-analytic support for the hybridisation-invasion hypothesis. Ecol. Lett. 17, 1464–1477. doi: 10.1111/ele.12355
Jiménez-Valverde, A., Peterson, A. T., Soberón, J., Overton, J. M., Aragón, P., and Lobo, J. M. (2011). Use of niche models in invasive species risk assessments. Biol. Invasions 13, 2785–2797. doi: 10.1007/s10530-011-9963-4
Johnson, J. S., Cantrell, R. S., Cosner, C., Hartig, F., Hastings, A., Rogers, H. S., et al. (2019). Rapid changes in seed dispersal traits may modify plant responses to global change. AoB Plants 11:plz020. doi: 10.1093/aobpla/plz020
Jones, P. A. (2012). Functions of DNA methylation: islands, start sites, gene bodies and beyond. Nat. Rev. Genet. 13, 484–492. doi: 10.1038/nrg3230
Kagawa, K., and Takimoto, G. (2018). Hybridization can promote adaptive radiation by means of transgressive segregation. Ecology Lett. 21, 264–274. doi: 10.1111/ele.12891
Keller, L. F., and Waller, D. M. (2002). Inbreeding effects in wild populations. Trends Ecol. Evol. 17, 230–241. doi: 10.1016/S0169-5347(02)02489-8
Kim, J. M, To, T. K., Ishida, J., Matsui, A., Kimura, H., and Seki, M. (2012). Transition of chromatin status during the process of recovery from drought stress in Arabidopsis thaliana. Plant Cell Phys. 53, 847–856. doi: 10.1093/pcp/pcs053
Kollmann, J., and Bañuelos, M. J. (2004). Latitudinal trends in growth and phenology of the invasive alien plant Impatiens glandulifera (Balsaminaceace). Divers. Distrib. 10, 377–385. doi: 10.1111/j.1366-9516.2004.00126.x
Kriticos, D. J., Clements, D. R., and DiTommaso, A. (2020). Biology of invasive plants: a new series within invasive plant science and management. Invasive Plant Sci. Manag. 13, 115–119. doi: 10.1017/inp.2020.25
Latimer, A. M., Jacobs, B. S., Gianoli, E., Heger, T., and Salgado-Luarte, C. (2019). Parallel functional differentiation of an invasive annual plant on two continents. AoB Plants 11:plz010. doi: 10.1093/aobpla/plz010
Lee, S. R., Choi, J. E., Lee, B. Y., Yu, J. N., and Lim, C. E. (2018). Genetic diversity and structure of an endangered medicinal herb: implications for conservation. AoB Plants 10:ply021. doi: 10.1093/aobpla/ply021
Leung, L. R., Qian, Y., Bian, X., Washington, W. M., Han, J., and Roads, J. O. (2004). Mid-century ensemble regional climate change scenarios for the western United States. Clim. Change 62, 75–113. doi: 10.1023/B:CLIM.0000013692.50640.55
Li, J., Du, L., Guan, W., Yu, F. H., and van Kleunen, M. (2016). Latitudinal and longitudinal clines of phenotypic plasticity in the invasive herb Solidago canadensis in China. Oecologia 182, 755–764. doi: 10.1007/s00442-016-3699-x
Liu, B., Yan, J., Li, W., Yin, L., Li, P., Yu, H., et al. (2020a). Mikania micrantha genome provides insights into the molecular mechanism of rapid growth. Nat. Commun. 11, 1–13. doi: 10.1038/s41467-019-13926-4
Liu, C., Wolter, C., Xian, W., and Jeschke, J. M. (2020b). Most invasive species largely conserve their climatic niche. PNAS 117, 23643–23651. doi: 10.1073/pnas.2004289117
Liu, J., Dong, M., Miao, S. L., Li, Z. Y., Song, M. H., and Wang, R. Q. (2006). Invasive alien plants in China: role of clonality and geographical origin. Biol. Invasions 8:1461. doi: 10.1007/s10530-005-5838-x
Luo, F., Chen, Y., Huang, L., Wang, A., Zhang, M., and Yu, F. (2014). Shifting effects of physiological integration on performance of a clonal plant during submergence and de-submergence. Ann. Bot. 113, 1265–1274. doi: 10.1093/aob/mcu057
Lustenhouwer, N., Wilschut, R. A., Williams, J. L., van der Putten, W. H., and Levine, J. M. (2018). Rapid evolution of phenology during range expansion with recent climate change. Glob. Change Biol. 24, e534–e544. doi: 10.1111/gcb.13947
Marchini, G. L., Ramakrishnan, A. P., Rosenthal, D. M., and Cruzan, M. B. (2015). Rapid purging of genetic load in a metapopulation and consequences for range expansion in an invasive plant. Biol. Invasions 18, 183–196. doi: 10.1007/s10530-015-1001-5
McDonald, A., Riha, S., DiTommaso, A., and Degaetano, A. (2009). Climate change and geography of weed damage: analysis of US maize systems suggests the potential for significant range transformation. Agric. Ecosys. Environ. 130, 131–140. doi: 10.1016/j.agee.2008.12.007
McWhorter, C. G. (1971). Introduction and spread of Johnsongrass in the United States. Weed Sci. 19, 496–500. doi: 10.1017/S0043174500050517
Mensing, S., and Byrne, R. (1998). Pre-mission invasion of Erodium cicutarium in California. J. Biogeogr. 25, 757–762. doi: 10.1046/j.1365-2699.1998.2540757.x
Molina-Montenegro, M. A., Acuña-Rodríguez, I. S., Flores, T. S., Hereme, R., Lafon, A., Atala, C., et al. (2018). Is the success of plant invasions the result of rapid adaptive evolution in seed traits? Evidence from a latitudinal rainfall gradient. Front. Plant Sci. 9:208. doi: 10.3389/fpls.2018.00208
Neve, P., Barney, J. N., Buckley, Y., Cousens, R. D., Graham, S., Jordan, N. R., et al. (2018). Reviewing research priorities in weed ecology, evolution and management: a horizon scan. Weed Res. 58, 250–258. doi: 10.1111/wre.12304
Nguyen, M. A., Ortega, A. E., Nguyen, K. Q., Kimball, S., Goulden, M. L., and Funk, J. L. (2016). Evolutionary responses of invasive grass species to variation in precipitation and soil nitrogen. J. Ecol. 104, 979–986. doi: 10.1111/1365-2745.12582
Pan, J. J., and Price, J. S. (2002). Fitness and evolution in clonal plants: the impact of clonal growth. Evol. Ecol. 15, 583–600. doi: 10.1023/A:1016065705539
Parepa, M., Fischer, M., Krebs, C., and Bossdorf, O. (2014). Hybridization increases invasive knotweed success. Evol. Appl. 7, 413–420. doi: 10.1111/eva.12139
Paterson, A. H., Kong, W., Johnston, R. M., Nabukalu, P., Wu, G., Poehlman, W. L., et al. (2020). The evolution of an invasive plant, Sorghum halepense L. (‘Johnsongrass’). Front. Genet. 11:317. doi: 10.3389/fgene.2020.00317
Patricola, C. M., and Wehner, M. F. (2018). Anthropogenic influences on major tropical cyclone events. Nature 563, 339–346. doi: 10.1038/s41586-018-0673-2
Pecinka, A., and Scheid, O. M. (2012). Stress-induced chromatin changes: a critical view on their heritability. Plant Cell Phys. 53, 801–808. doi: 10.1093/pcp/pcs044
Pick, L., and Heffer, A. (2012). Hox gene evolution: multiple mechanisms contributing to evolutionary novelties. Ann. N. Y. Acad. Sci. 1256, 15–32. doi: 10.1111/j.1749-6632.2011.06385.x
Pitcairn, M. J., Schoenig, S., Yacoub, R., and Gendron, J. (2006). Yellow starthistle continues its spread in California. California Agric. 60, 83–90. doi: 10.3733/ca.v060n02p83
Pujol, B., Zhou, S., Vilas, J. S., Pannell, J. R., and Boyce, M. S. (2009). Reduced inbreeding depression after species range expansion. PNAS 106, 15379–15383. doi: 10.1073/pnas.0902257106
Pyšek, P., Brundu, G., Brock, J., Child, L., and Wade, M. (2019). Twenty-five years of conferences on the ecology and management of alien plant invasions: the history of EMAPi 1992–2017. Biol. Invasions 21, 725–742. doi: 10.1007/s10530-018-1873-2
Qin, Z., DiTommaso, A., Wu, R. S., and Huang, H. Y. (2014). Potential distribution of two Ambrosia species in China under projected climate change. Weed Res. 54, 520–531. doi: 10.1111/wre.12100
Ramesh, K., Matloob, A., Aslam, F., Florentine, S. K., and Chauhan, B. S. (2017). Weeds in a changing climate: vulnerabilities, consequences, and implications for future weed management. Front. Plant Sci. 8:95. doi: 10.3389/fpls.2017.00095
Reznick, D. N., Losos, J., and Travis, J. (2019). From low to high gear: there has been a paradigm shift in our understanding of evolution. Ecol. Lett. 22, 233–244. doi: 10.1111/ele.13189
Roiloa, S., Rodríguez-Echeverría, S., Freitas, H., and Retuerto, R. (2013). Developmentally-programmed division of labour in the clonal invader Carpobrotus edulis. Biol. Invasions 15, 1895–1905. doi: 10.1007/s10530-013-0417-z
Roiloa, S. R., Rodriguez-Echeverria, S., de la Pena, E., and Freitas, H. (2010). Physiological integration increases the survival and growth of the clonal invader Carpobrotus edulis. Biol. Invasions 12, 1815–1823. doi: 10.1007/s10530-009-9592-3
Rosche, C., Hensen, I., Mráz, P., Durka, W., Hartmann, M., and Lachmuth, S. (2017). Invasion success in polyploids: the role of inbreeding in the contrasting colonization abilities of diploid versus tetraploid populations of Centaurea stoebe s.l. J. Ecol. 105, 425–435. doi: 10.1111/1365-2745.12670
Rouifed, S., Puijalon, S., Viricel, M., and Piola, F. (2011). Achene buoyancy and germinability of the terrestrial invasive Fallopia × bohemica in aquatic environment: a new vector of dispersion? Écoscience 18, 79–84. doi: 10.2980/18-1-3397
Rutland, C. A., Hall, N. D., and McElroy, J. S. (2021). The impact of polyploidization on the evolution of weed species: historical understanding and current limitations. Front. Agron. 3:5. doi: 10.3389/fagro.2021.626454
Simon, J. C., and Peccoud, J. (2018). Rapid evolution of aphid pests in agricultural environments. Curr. Opin. Insect Sci. 26, 17–24. doi: 10.1016/j.cois.2017.12.009
Sołtysiak, J. (2020). Does the urban heat island determine the distribution of Fallopia taxa in cities?–preliminary study from Wrocław (Central Europe). Ecol. Quest. 31, 1–12. doi: 10.12775/EQ.2020.010
Sterling, T. M., Norman, K. L., and Murray, L. W. (2001). Picloram-resistant and -susceptible yellow starthistle accessions have similar competitive ability. Weed Sci. 49, 42–47. doi: 10.1614/0043-1745(2001)049[0042:PRASYS]2.0.CO;2
Strelau, M., Janse van Rensburg, M., and Clements, D. R. (2018). Dose-response methodology for variant populations of Bohemian knotweed (Reynoutria × bohemica Chrtek & Chrtková). Can. J. Plant Sci. 98, 1380–1383. doi: 10.1139/cjps-2018-0043
Sun, Y., Bossdorf, O., Grados, R. D., Liao, Z., and Müller-Schärer, H. (2020). Rapid genomic and phenotypic change in response to climate warming in a widespread plant invader. Glob. Change Biol. 26, 6511–6522. doi: 10.1111/gcb.15291
Sun, Y., and Roderick, G. K. (2019). Rapid evolution of invasive traits facilitates the invasion of common ragweed, Ambrosia artemisiifolia. J. Ecol. 107, 2673–2687. doi: 10.1111/1365-2745.13198
Tamarin-Brodsky, T., and Kaspi, Y. (2017). Enhanced poleward propagation of storms under climate change. Nat. Geosci. 10, 908–913. doi: 10.1038/s41561-017-0001-8
te Beest, M., Le Roux, J. J., Richardson, D. M., Brysting, A. K., Suda, J., Kubesova, M., et al. (2012). The more the better? The role of polyploidy in facilitating plant invasions. Ann. Bot. 109, 19–45. doi: 10.1093/aob/mcr277
Tougas-Tellier, M. A., Morin, J., Hatin, D., and Lavoie, C. (2015). Freshwater wetlands: fertile grounds for the invasive Phragmites australis in a climate change context. Ecol. Evol. 5, 3421–3435. doi: 10.1002/ece3.1576
Turner, K. G., Nurkowski, K. A., and Rieseberg, L. H. (2017). Gene expression and drought response in an invasive thistle. Biol. Invasions 19, 875–893. doi: 10.1007/s10530-016-1308-x
van Boheemen, L. A., Atwater, D. Z., and Hodgins, K. A. (2019). Rapid and repeated local adaptation to climate in an invasive plant. New Phytol. 222, 614–627. doi: 10.1111/nph.15564
van Kleunen, M., Bossdorf, O., and Dawson, W. (2018). The ecology and evolution of alien plants. Ann. Rev. Ecol. Evol. Syst. 49, 25–47. doi: 10.1146/annurev-ecolsys-110617-062654
van Kleunen, M., and Fischer, M. (2005). Constraints on the evolution of adaptive phenotypic plasticity in plants. New Phytol. 166, 49–60. doi: 10.1111/j.1469-8137.2004.01296.x
van Kleunen, M., and Stuefer, J. F. (1999). Quantifying the effects of reciprocal assimilate and water translocation in a clonal plant by the use of steam-girdling. Oikos 85, 135–145. doi: 10.2307/3546799
Varanasi, A, Prasad, P. V., and Jugulam, M. (2016). Impact of climate change factors on weeds and herbicide efficacy. Adv. Agron. 135, 107–146. doi: 10.1016/bs.agron.2015.09.002
Walls, R. L. (2010). Hybridization and plasticity contribute to divergence among coastal and wetland populations of invasive hybrid Japanese knotweed s.l. (Fallopia spp.). Estuaries Coast. 33, 902–918. doi: 10.1007/s12237-009-9190-8
Wan, F. H., Guan, G. Q., and Wan, R. (1993). Ambrosia and Its Comprehensive Administration. Beijing, China: China Science and Technology Press (in Chinese).
Wan, J., Oduor, A. M., Pouteau, R., Wang, B., Chen, L., Yang, B., et al. (2020). Can polyploidy confer invasive plants with a wider climatic tolerance? A test using Solidago canadensis. Ecol. Evol. 10, 5617–5630. doi: 10.1002/ece3.6303
Wan, J. Z., Wang, C. J., Tan, J. F., and Yu, F. H. (2017). Climatic niche divergence and habitat suitability of eight alien invasive weeds in China under climate change. Ecol. Evol. 7, 1541–1552. doi: 10.1002/ece3.2684
Wang, J., Xu, T., Wang, Y., Li, G., Abdullah, I., Zhong, Z., et al. (2020). A meta-analysis of effects of physiological integration in clonal plants under homogeneous vs. heterogeneous environments. Funct. Ecol. 35, 578–589. doi: 10.1111/1365-2435.13732
Warwick, S. I., and Black, L. D. (1983). The biology of Canadian weeds. 61. Sorghum halepense (L.) Pers. Can. J. Plant Sci. 63, 997–1114. doi: 10.4141/cjps83-125
Warwick, S. I., Phillips, D., and Andrews, C. (1986). Rhizome depth: the critical factor in winter survival of Sorghum halepense (L.) Pers. (Johnsongrass). Weed Res. 26, 381–387. doi: 10.1111/j.1365-3180.1986.tb00721.x
Warwick, S. I., Thompson, B. K., and Black, L. D. (1984). Population variation in Sorghum halepense, Johnson grass, at the northern limits of its range. Can. J. Bot. 62, 1781–1790. doi: 10.1139/b84-242
Waryszak, P., Lenz, T. I., Leishman, M. R., and Downey, P. O. (2018). Herbicide effectiveness in controlling invasive plants under elevated CO2: sufficient evidence to rethink weeds management. J. Environ. Manag. 226, 400–407. doi: 10.1016/j.jenvman.2018.08.050
Westerling, A. L., Hidalgo, H. G., Cayan, D R., and Swetnam, T. W. (2006). Warming and earlier spring increase western US forest wildfire activity. Science 313, 940–943. doi: 10.1126/science.1128834
Williams, J. L., Hufbauer, R. A., and Miller, T. E. (2019). How evolution modifies the variability of range expansion. Trends Ecol. Evol. 34, 903–913. doi: 10.1016/j.tree.2019.05.012
Young, S. L. (2015). When an invasive plant fails to invade. Front. Ecol. Environ. 13, 450–451. doi: 10.1890/1540-9295-13.8.450
Young, S. L., Clements, D. R., and DiTommaso, A. (2017). Climate dynamics, invader fitness, and ecosystem resistance in an invasion-factor framework. Invasive Plant Sci. Manag. 10, 215–231. doi: 10.1017/inp.2017.28
Keywords: hybridization, climate change, polyploidy, drought, plasticity, invasion biology, rapid evolution
Citation: Clements DR and Jones VL (2021) Rapid Evolution of Invasive Weeds Under Climate Change: Present Evidence and Future Research Needs. Front. Agron. 3:664034. doi: 10.3389/fagro.2021.664034
Received: 04 February 2021; Accepted: 12 March 2021;
Published: 07 April 2021.
Edited by:
Ali Bajwa, New South Wales Department of Primary Industries, AustraliaReviewed by:
Simerjeet Kaur, Punjab Agricultural University, IndiaSteven Franks, Fordham University, United States
Copyright © 2021 Clements and Jones. This is an open-access article distributed under the terms of the Creative Commons Attribution License (CC BY). The use, distribution or reproduction in other forums is permitted, provided the original author(s) and the copyright owner(s) are credited and that the original publication in this journal is cited, in accordance with accepted academic practice. No use, distribution or reproduction is permitted which does not comply with these terms.
*Correspondence: David R. Clements, Y2xlbWVudHMmI3gwMDA0MDt0d3UuY2E=