- 1Department of Agronomy, Faculty of Agriculture, University of Kafrelsheikh, Kafr El-Shaikh, Egypt
- 2Department of Field Crops, Faculty of Agriculture, Siirt University, Siirt, Turkey
- 3Department of Agronomy, Hajee Mohammad Danesh Science and Technology University, Dinajpur, Bangladesh
- 4Department of Botany and Plant Physiology, Faculty of Agrobiology, Food, and Natural Resources, Czech University of Life Sciences Prague, Prague, Czechia
- 5College of Agronomy, Sichuan Agricultural University, Chengdu, China
- 6Principal Scientist (Agronomy), Punjab Agricultural University, Regional Research Station, Faridkot, India
- 7Department of Genetics and Plant Breeding, Bangladesh Agricultural University, Mymensingh, Bangladesh
- 8Department of Agronomy, Bangladesh Wheat and Maize Research Institute, Dinajpur, Bangladesh
- 9College of Plant Science and Technology, Huazhong Agricultural University, Wuhan, China
- 10Plant Physiology Division, Nuclear Institute of Agriculture (NIA), Tandojam, Pakistan
- 11Department of Agronomy, Faculty of Agriculture, University of Poonch Rawalakot (AJK), Rawalakot, Pakistan
- 12Department of Agricultural Biology, Faculty of Agriculture, University of Ruhuna, Matara, Sri Lanka
- 13ICAR-Indian Grassland and Fodder Research Institute, Jhansi, India
- 14International Rice Research Institute, Bangladesh Office, Dhaka, Bangladesh
- 15Department of Botanical and Environmental Sciences, Guru Nanak Dev University, Amritsar, India
- 16College of Agriculture, Bahauddin Zakariya University, Bahadur Sub-Campus Layyah-Pakistan, Layyah, Pakistan
- 17Department of Plant Physiology, Slovak University of Agriculture, Nitra, Slovakia
- 18Institute of Crop Science and Resource Conservation (INRES), Crop Science Group, University of Bonn, Bonn, Germany
- 19Faculty of Science, Plant Breeding Institute, Sydney Institute of Agriculture, School of Life and Environmental Sciences, University of Sydney, Sydney, NSW, Australia
- 20College of Resources and Environmental Sciences, China Agricultural University, Beijing, China
Wheat constitutes pivotal position for ensuring food and nutritional security; however, rapidly rising soil and water salinity pose a serious threat to its production globally. Salinity stress negatively affects the growth and development of wheat leading to diminished grain yield and quality. Wheat plants utilize a range of physiological biochemical and molecular mechanisms to adapt under salinity stress at the cell, tissue as well as whole plant levels to optimize the growth, and yield by off-setting the adverse effects of saline environment. Recently, various adaptation and management strategies have been developed to reduce the deleterious effects of salinity stress to maximize the production and nutritional quality of wheat. This review emphasizes and synthesizes the deleterious effects of salinity stress on wheat yield and quality along with highlighting the adaptation and mitigation strategies for sustainable wheat production to ensure food security of skyrocketing population under changing climate.
Introduction
Recently, climate change and global warming have directly affected the crops yield and quality by intensifying the frequency and extent of numerous stresses. Wheat, rice, and maize are the most important staple crops globally and contribute a significant part of daily calories and protein intake (Kizilgeci et al., 2021). Among these major cereals, wheat is ranked at the first position due to its domestication and contribution as the primary staple food crop globally (Iqbal et al., 2021). Currently, it is dominating the most of arable land (38.8%), with relatively higher grain protein (12–15%) than other cereals, but the productivity remains low [Food and Agriculture Organization (FAO) of the United Nations, 2016]. It can further decrease owing to climate change that has given to rise a variety of abiotic stresses. Various climate models projected that wheat production could decrease by 6% due to stressful environments (Asseng et al., 2015).
Salt stress affects 20% of global cultivable land and is increasing continuously owing to the change in climate and anthropogenic activities (Arora, 2019). Environmental stress including salinity can cause about 50% of production losses (Acquaah, 2007). Furthermore, the continuous increase in the human population put pressure on global food security as the world's food supply needs to be increased by up to 70% by 2050 (FAO, 2009). Wheat (Triticum aestivum) is considered the most significant grain crop among all the cereals and ranked 1st globally among grain-producing crops, especially for human consumption (Giraldo et al., 2019). About 36% of the world's population is dependent on wheat as a staple food. About 20% of calories and 55% of carbohydrates are being provided by wheat across the globe. Both growth and yield of wheat are negatively influenced by salinity (Royo and Abió, 2003). Salinity stress causes osmotic stress and ion toxicity, through increasing the assimilation of Na+ ion and decreasing the Na+/K+ ratio due to lower osmotic potential within the plant roots. Further, these ionic imbalance affects the uptake, and transport of other important essential ions in target cells and hamper the crucial plant processes and functions (Arif et al., 2020). Salinity impairs the seedling establishment, stunted plant growth, poor reproductive development, and ultimately declines the crop yield (Turan et al., 2009). Salinity also alters the ultrastructural cell components, disturbs the photosynthesis machinery, damages the membranous structure, increases the reactive oxygen species production, reduces the enzymatic activity, which limit the growth and yield of crops (Hasanuzzaman et al., 2014). Tolerancey of plants to salinity is a polygenic character that is governed by many genetic factors (Arzani and Ashraf, 2016). Crop growth is improved under salinity by the increase in K+, elimination of Na+ or by optimizing the ratio of both Na+ and K+ ions, improving transpiration efficiency, regulation of osmotic potential, and by the antioxidant, immune system of plants (Rahman et al., 2005).
Among various field crops, generally, wheat is more sensitive to salinity that hampers the growth and development of plant, leads to low productivity or even complete crop failure under extreme severity of salinity. The knowledge of stress tolerance in plants regarding the physiological basis is important for selection and breeding programs (Chaves et al., 2003). Thus, understanding of morphoanatomical, physiological, biochemical, and molecular mechanisms of wheat responses to salinity stress at each phase of growth is essential to improve breeding techniques and to develop salt-tolerant varieties with genetic modifications. The recent findings indicate that change in leaf and stem anatomical features in different genotypes of wheat are crucial traits to adaptation under salinity stress (Nassar et al., 2020). The research on the physiological changes that occur during leaf senescence due to some stresses has been primarily focused on the loss of photosynthetic pigments, protein degradation, and re-absorption of mineral nutrients (Zheng et al., 2008). At the same time accumulation of secondary metabolites (anthocyanins, flavones, phenolics, and specific phenolic acids) often occurs in plants subjected to stresses including various elicitors or signal molecules (Sytar et al., 2018). It was demonstrated that pigmented wheat genotypes with high anthocyanin content can maintain significantly higher dry matter production under salt stress conditions (Mbarki et al., 2018), which shown the role of phenolic compounds in salinity tolerance together with new breaded pigmented wheat genotypes.
Determination of physiological traits related to stress tolerance could be used as a selection criterion to enhance wheat adaptation to stress conditions. According to the previous investigations, there is a link between different physiological responses of crops to stress and their tolerance mechanisms, such as high relative water content and water potential (Datta et al., 2011). Moreover, it has been observed that anthesis and grain filling period are very sensitive stages under multiple environmental stresses including salinity and have been identified as major constraints to wheat production worldwide (Ghosh et al., 2016). Therefore, it is crucial to understand the effects of salt stress on wheat yield improvement while maintaining superior productivity and adopting mitigation strategies toward the long-term goal of sustainable food security. This study aimed to synthesize salinity effects on wheat germination, seedling growth, reproductive development, grain yield, and quality. Additionally, deleterious effects of salinity in relations to nutrient imbalance and water relations have been objectively described. Moreover, integrated approach for salinity mitigation through osmoprotectants, plant hormones, mineral nutrients, and signaling molecules has been elucidated.
The current review overviews the adverse effects of salinity stress on wheat and its adaptation and mitigation strategies for the sustainability of wheat productivity under the changing climate.
Adverse Effects of Salinity Stress on Wheat
Most of the agricultural lands, which are affected by different degrees of salinity, are located in semi-arid or arid regions (Liu et al., 2020). Huang et al. (2019) concluded that the damage of crops is mostly intensified by the synchronized action of xerothermic aspects, such as aridity and high temperature. Salinity is the most adversely affecting factor on productivity and quality of wheat through altering the physiological as well as biochemical activities in plants. Generation of ROS due to Na+ toxicity, which damage biomolecules (e.g., lipids, proteins, and nucleic acids) (Apel and Hirt, 2004) on the cellular level and alters redox homeostasis, is a common phenomenon under salt stress (Kundu et al., 2018). However, salt impacted soils are difficult to remediate due to the circumstances outlined by Arzani and Ashraf (2016). First, Na+ and Cl− ions are highly mobile in soils. Second, it is often an expensive and short-term solution for the chronic problem. Third, soil salinity has a dynamic nature and spatial variation in salinity is generated by the interactions among different variables of edaphic effects (soil pH, bulk density, permeability, topography, geohydrology, water table depth, and groundwater salt content), geographic factors (elevation, slope, and aspect), agronomic practices (irrigation, drainage, tillage, crop rotation, and fertilization), and climatic effects (temperature, humidity precipitation, wind, and evaporation) (Bui, 2013). Therefore, the integrated agronomical, physiological, and soil management approaches and targeting multiple traits at the same time are a crucial step to achieve salinity tolerance. Therefore, it is important to substitute Na+ with the Ca2+ followed by removal/leaching of salts derived by the reaction of the amendment from sodic soil for sustainable crop production (Sorour et al., 2019).
Salinity delays the onset of seedlings germination, decreases the seedling growth and the dispersion of germination events, seedling metabolism, causing a reduction in plant growth and crop productivity (El Sabagh et al., 2019a,b,c, 2020). One important approach is to develop an understanding of the plant response toward salinity stress. The response of plants to salinity can be described in two subsequent phases (Arzani and Ashraf, 2016), during the first phase, salinity causes osmotic stress because of a decrease in the soil water potential (James et al., 2006). The second phase develops within a few days or weeks (depend on the severity of salinity) and accumulates Na+ ions in different plant tissues, causing reduced yield and even plant death (Munns and Tester, 2008). Under salinity, Na+ is the principle of toxic ion imposing both osmotic stress and ionic toxicity (Munns and Tester, 2008). Salinity also negatively affects wheat phenological developments such as leaf number, leaf expansion rate, and root/shoot ratio (El-Hendawy et al., 2005), and biomass production (Sorour et al., 2019). The saline environment disturbs plant water relations including relative water content, leaf water potential, water uptake, transpiration rate, water retention, and water use efficiency (Nishida et al., 2009).
Salinity adversely affects the growth and yield of crop plants by decreasing the availability of soil moisture, and due to the toxicity effects of sodium and chloride ions at high concentrations to the plant (Munns and Tester, 2008). Salinity stress accelerates all phenological phases of wheat (Grieve et al., 1994), reduces the number of fertile tillers (Abbas et al., 2013), decreases the number of spikelet number spike−1 (Frank et al., 1987), kernel weight (Abbas et al., 2013), and affects grain yield adversely (Sorour et al., 2019). For instance, yield losses up to 45% have been recorded in salt-stressed wheat (Ali et al., 2009). Hasan et al. (2015) observed that saline stress (15 dSm−1) significantly decreases grains per spike, 1,000-grain weight, and seed yield in tolerant and sensitive wheat cultivars. The effect of salinity stress on root activity, germination, morphological traits, crucial plant processes, yield, and yield attributes in wheat are illustrated in Figure 1.
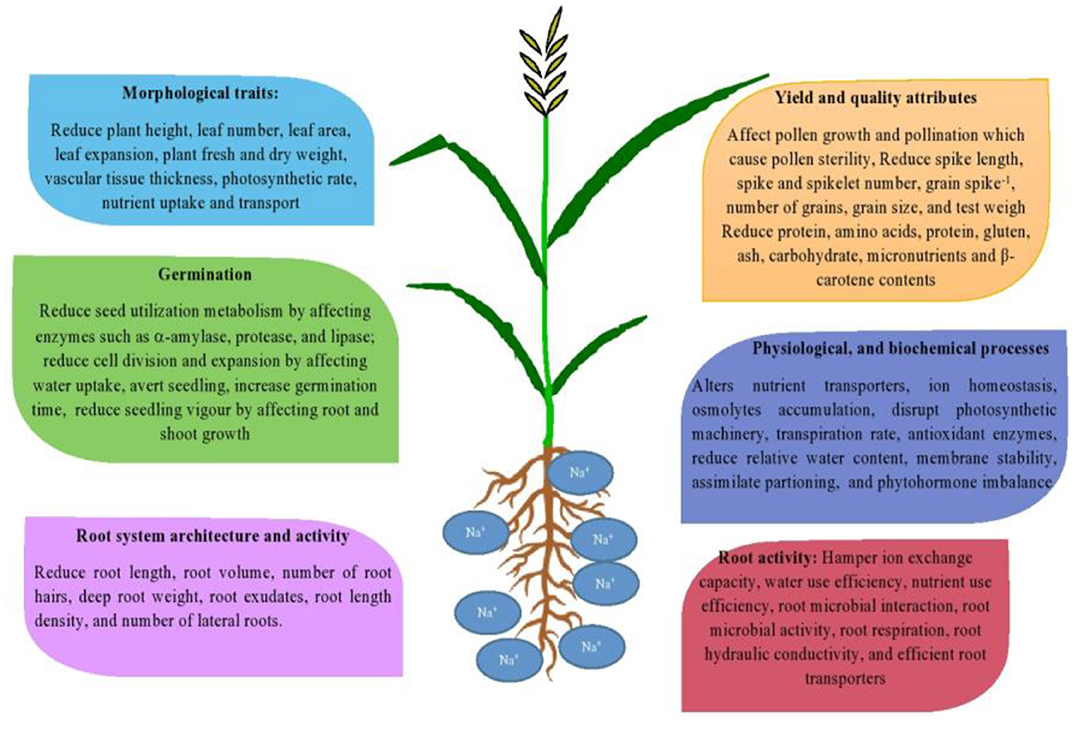
Figure 1. Illustrates the effects of salinity stress on different growth stages and crucial plant processes.
Germination and Plant Growth
Soil salinity is the second major factor responsible for land degradation after soil erosion, causing a decline in agricultural economic outputs for 10,000 years (Shahid et al., 2018). Poor salinity management can cause soil sodicity of farming soils, where sodium (Na) binds to negatively charged clay, causing clay swelling and dispersal, subsequently decreasing the crop yield. Higher levels of salinity confiscate 1.5 million hectares of land globally every year, and hence ~50% of cultivable land could be deteriorated by the mid of 21st century. During salinity, the exaggeration of most plants is apparent in the early stages, especially during seedling establishment, as it is the most responsive and critical stage that is reported to be strongly associated with successful germination and seedling development. Various factors hamper the crop yield under salinity stress, but osmotic stress, ionic imbalance, and oxidative stress are the major ones among them. In brief, the osmotic stress leads to a higher accumulation of salts in cell sap and tissues which become observable as leaf burn and wilting. These symptoms are reported to be associated directly with the accumulation of Na+ and Cl−. Thus, this ionic imbalance causes disequilibrium of nutrients that declines germination, and adversely affects the subsequent metabolic processes (Hussain et al., 2019). Furthermore, the oxidative stress exerted via accelerated ROS generation induces lipid peroxidation, disrupt nucleic acids that ultimately decreases the consistency and overall yield of the affected seed (Dehnavi et al., 2020; Kumari and Kaur, 2020).
Germination is a dynamic and critical phase in the lifecycle of a plant that pledges via the imbibition of water (Kumari and Kaur, 2018). It is a triphasic process, and during phase 1, the seeds absorb water, followed by the second phase, i.e., the “plateau phase” (stable water content), and characterized by test rupturing. In the third phase, endosperm ruptures and radicle protrusion take place, and it is also referred to as the post-germination phase (Chamorro et al., 2017). In 2007, Läuchli and Grattan proposed a general scheme for depicting the relationship of germination percentage with the time of germination under low, moderate, and high salinity levels (Figure 2).
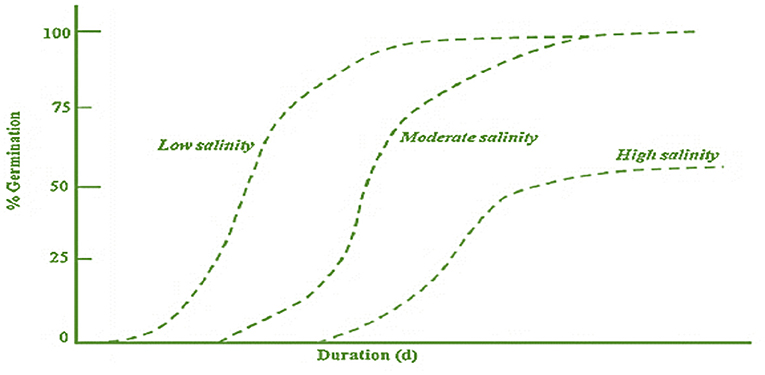
Figure 2. Association between germination rate and exposure duration after sowing at different salinity levels (low, moderate, and high) (Source: Läuchli and Grattan, 2007).
Salinity inhibits seed germination by either exerting osmotic stress that thwarts water uptake or causes ionic toxicity. These consequences collectively inhibit cell division and expansion, as well as modulates the activity of some key enzymes, thus lastly reduces the seed reserves utilization (El-Hendawy et al., 2019). Thus, it can be said that salinity negatively affected the process of germination by altering the normal germination mechanism that resulted in reduced growth and development, ultimately declined the economic yield. Moreover, the increased incidence of salt stress in the arable land indicates that there is an urgent need for a deeper understanding of the mechanisms for plant tolerance to preserve crop productivity through the optimal regulation of growing conditions of cereal crops. There is dire need to develop strategies such seed priming with natural or synthetic growth regulators etc. for boosting cereals germination in saline environment, otherwise significant decline in germination could lead food crisis in future.
Adverse Effects of Salinity Stress on Morphological Processes of Plants
Soil salinity detrimentally affects the various morphological characteristics of wheat plants including seedling growth, plant height, shoot, and root length, the number of roots, leaves, leaf area, fresh and dry weight, root/shoot ratio, and chlorophyll content. Ahmad et al. (2013a) observed that the early maturity of wheat due to salinity stress reduced the crop height and leaf area and found that plumule length was the most sensitive during early growth stages. Bacilio et al. (2004) found that root colonization pattern, leaf expansion, and flag leaf area in wheat was significantly lower under salinity stress. Furthermore, the number of leaves plant−1 and leaf size, along with leaf longevity, were shriveled because of salinity stress (El-Hendawy et al., 2009). Otu et al. (2018) reported that wheat facing salinity stress exhibited reduced stem length, stem weight, and shoot dry matter. Such detrimental effects of salinity stress on the dry weight of shoot may be due to the direct impact on photosynthesis (Parida et al., 2005). Maas (1990) stated that the salinity changes the final size of a spike and a significant reduction occurs in spike length and number, and grains spike−1. Shafi et al. (2010) found that salinity stress inhibited the shoot and root dry weight, number of root tips (lateral roots), total root length, average root diameter, and total root volume. The root is the first important organ, and a well-developed root system can contribute advantages to wheat plant to maintain plant growth for the period of early growth phases, and extracts water and micro-nutrients through the soil (Egamberdieva, 2009). The production of the well-developed root system under stress conditions is vital for above-ground biomass production (Iqbal et al., 2018). Saboora et al. (2006) observed that the root development inclined severely due to salinity stress in wheat besides reduced root length as well as area. Ammonium inhibits the root growth of many plants, including wheat, whereas NaCl stimulates the accumulation of ammonium in roots which restrains the root growth. New cultivars having improved agro-morphological traits and potential to ameliorate the adverse effect of salinity must be developed through a targeted breeding program.
Adverse Effects of Salinity Stress at the Reproductive Stage on Plant Growth and Yield
Several earlier studies illustrated that the reproductive phase of any crop is the most sensitive stage to the abiotic stresses, including salinity (Ehtaiwesh and Rashed, 2020), and cause massive yield penalty in important crops, including wheat (Kalhoro et al., 2016). However, it is well-postulated that salinity influences plant growth and yield attributes primarily due to ion toxicity and osmotic stress. Although, the intrinsic pathways and molecular mechanisms are so far not clear. Salt stress influences cell ion homeostasis by altering ion balance, such as increased Na+ and a simultaneous decreased Ca2+ and K+ content. A recent study suggested that the class I high-affinity K+ transporter (HKT) family is involved in the exclusion of Na+ from leaf blades at the reproductive stage, and this significantly influences sodium ion homeostasis under salinity stress (Suzuki et al., 2016). Likewise, the grain dry matter and K+/Na+ ratio showed a significant correlation and regulated the grain filling rate and duration under salt stress (Poustini and Siosemardeh, 2004). Similarly, an isotopic study (δ13C, δ18O, and δ15N) in wheat crop under different salinity regimes depicted that nitrogen metabolism and stomatal limitations at the anthesis stage are among the major cause of biomass reduction (Yousfi et al., 2013). Furthermore, transcriptomics study in wheat (Arg cultivar) genotype revealed that 109 genes are unique in treated plants, which are mainly associated with transcription factors gene (late embryogenic abundant [LEA] protein, dehydrin, MYB, and ERF), ion transporter (SOS1), antioxidant defense (peroxidase, glutathione S-transferase, GST) and secondary metabolites (caffeic acid 3-O-methyltransferases), and have a role in the regulation of ion homeostasis, cell redox balance, and oxidative stress (Amirbakhtiar et al., 2019).
Besides ionic imbalance, salinity stress influences available soil water, tissue water content, water use efficiency, water potential, transpiration rate, rooting depth, root respiration, root biomass, root hydraulic conductance, cell turgidity, and osmolytes accumulations (Zheng et al., 2008). Besides, it also reduces the photosynthetic rate, biomass accumulation, and source-sink activity, which hastens the reproductive organ's senescence and negatively affects the yield response factors (Khataar et al., 2018). Similarly, at the reproductive phase, alteration in water potential reduces the cell elongation, flag leaf thickness, vascular tissue thickness, mesophyll, and epidermal cell size, which are responsible for the reduction of flag leaf turgidity and leaf area, assimilates synthesis, and finally yield potential (Farouk, 2011).
Although, Ashraf and Ashraf (2016) suggested that these physiological and biochemical trait alterations are stage-specific and attribute to final yield potential. For example, salinity at different stages such as anthesis, early booting, and mid grain filling causes a reduction of grain yield by 39.1, 24.3, and 13.4%, respectively. Salt stress caused the acceleration of shoot apex development but decreased the number of spikelet primordia and also resulted in early terminal spikelet stage and anthesis. This decreased the number of spikes and kernels per spikes, which ultimately reduced the yield potential in wheat (Maas and Grieve, 1990). Likewise, use of 200 mM NaCl stress at pre-anthesis and post-anthesis stage caused reduction in aboveground biomass, ears plant−1, ear weight, number of grains plant−1, C, N, and C/N ratio in grains, and carbon use efficiency at both stages, although the reductions were higher due to imposition of stress at both stages as compared to single-stage (Eroglu et al., 2020). It has also been observed that the unavailability of sufficient photo-assimilates during the reproductive stage is the leading cause for losing yield potential in wheat, and this might be due to the changes in gene expression caused by salt stress during the pre-anthesis and grain filling stage. For example, alteration of sucrose 1-fructosyltransferase, sucrose: fructan6-fructosyltransferase, and fructanexohydrolase hampers the fructan accumulation, and remobilization of carbohydrate to grains (Sharbatkhari et al., 2016). Similarly, yield component traits such as spike length, spike weight, filled spikelet plant−1, total spikelet plant−1, and test weight were reduced to 8, 3, 37, 20, and 10%, respectively under stress condition, and resulted in 16% low total grain weight plant−1 (Tareq et al., 2011). Further, losses of grain weight under saline stress occurs due to pollen sterility, less production of assimilates, and reduced partitioning toward economic parts (grains) of plants. Likewise, the study on 151 synthetic wheat-breeding lines suggested that salt stress to be associated with Na+ toxicity, which reduced the total kernel weight, and starch content by 20 and 6%, respectively (Dadshani et al., 2019).
In spite of these changes, salinity stress has substantial impacts on grain quality traits. For example, the use of 200 mM of NaCl in wheat (cv. Shatabdi) showed an increase in Na+, K+, and Ca+2 content in grains by 155, 10, and 20%, respectively under stress (Tareq et al., 2011). Similarly, the use 0.75% NaCl salt increased the contents of high molecular weight glutenin subunit, glutenin macro-polymers (36.14%), and amino acids and improved wheat quality at certain levels but reduce grain yield (Zhang et al., 2016). Similarly, Nadeem et al. (2020) reported that salinity negatively influenced the yield (grain length, test weight, and grain yield), nutritional quality traits (moisture, fat, ash, fiber, and gluten content), and mineral nutrient content (K, Ca, Fe, P, Zn, and Mg) in wheat crop. Therefore, it can be concluded that salinity stress is a major factor for limiting yield and yield quality traits, and it affects the reproductive phage severely by altering ion homeostasis, water status, and assimilate partitioning.
Adverse Effects of Salinity on Grain Quality
Soil salinity imparts detrimental impacts on vital metabolic, biochemical, and physiological processes occurring within the plants leading to the deterioration of grain quality. The extent of changes in grain quality caused by salinity depends on the sternness of the stress. From physiological perspectives, grain quality is affected owing to the accumulation of salts in the root zone leading to osmotic stress induction, which vigorously disrupted cell ion homeostasis. Salt exposure causes osmotic stress at the beginning, while subsequently, ion toxicity hampers growth, grain development, and quality, especially if the exposure periods get prolonged. The deterioration of grain quality of cereals has also been explained in agronomic perspectives as well. The reduction in the capability of roots for water uptake owing to osmotic stress contributes to growth inhibition, declined crop productivity, and inferior grain quality (Netondo et al., 2004). Thus, grain quality is drastically affected by the devastating effects of osmotic stress, while the subsequent slower ion toxicity phase is even more detrimental.
Winter wheat constitutes a vital source of carbohydrates and protein for humans across the globe (Siddiqui et al., 2019). There have been significant achievements pertaining to boost wheat yield over the decades. However, the demand for higher-quality grain has also increased with the improvement in human lifestyle (Park et al., 2009). Plant variety, in conjunction with the prevalent environment, has also been reported to determine the wheat quality to a certain extent (Sairam et al., 2002). Previously, most researchers focused on the impacts of salinity on wheat grain yield (Zheng et al., 2009), but little is known about the relationships between salt-tolerance and grain quality. There are diverse effects of salinity levels on the grain quality of cereals. It has been inferred that salinity levels, especially beyond 150 mM of NaCl, significantly reduced the grain yield, whereby grain quality deterioration remained significant at 100 mM (Farooq and Azam, 2005). There is a varying impact of salinity on the nutritional value of grains depending upon the plant growth stage at which stress occurs. Breeding approaches to improve grain yield, especially in the salt-tolerant varieties have negatively affected the grain quality as these traits are often inversely related.
As far as grain quality of cereals under abiotic stresses especially salinity is concerned, there have been relatively limited investigations. Protein, fat, and fibers contents in grain decreased significantly due to salinity. In response to imposed salinity, protein content improved in the sensitive wheat genotypes, while it decreased in tolerant genotypes. The ash and beta-carotene contents were enhanced, while the gluten index got declined considerably (Katerji et al., 2005). At the same time, Maqsood et al. (2008) inferred that the decrease in protein and fiber contents of cereals was due to the accumulation of salts in the root zone, which deteriorated the grain quality. Additionally, higher concentration of Na+ in the external environment interferes with the absorption of nitrogen, which leads to lower protein content in wheat grains. Thus, the nutritional imbalance is reported to be the major factor behind the deteriorated grain quality of wheat under salt stress. Furthermore, salt stress interfered with prime processes, including photosynthesis, energy production, lipid metabolism, and protein synthesis. Lastly, salinity reduced phosphorus (P) concentration in plant shoots, which interfered with grain development and nutritional quality. Optimization of P foliar doses might be developed as a potent approach to alleviate the salinity effects on grain size and weights which are the vital yield attributes of cereals.
Protein Content
Protein content is the most important indicator of wheat grain quality and hence it governs and determines the end-use quality. The grain quality of wheat, especially the quality of protein as well as its quantity, is vital for dough properties and the bread-making quality of wheat flour. Under the saline condition, the protein quantity is increased, but the protein quality is decreased in wheat and triticale. The protein content is controlled by the genetic makeup of a particular cultivar or line, environmental factors, especially temperature and soil fertility status predominantly concerning N concentration in soil solution. It is significantly affected by environmental factors and their interactions. Positive correlations between environmental factors and wheat grain protein content have been reported during grain filling (Huebner et al., 1997). Protein content, along with composition, significantly modifies wheat flour quality for bread-making (Branlard et al., 2001). Nevertheless, the high protein content of the wheat grain is privileged because flour protein content has a linear relationship with bread-making quality (Schofield, 1994). Conversely, protein quality signifies the quality of bread-making. It has been established that the composition of grain protein primarily depends on wheat genotypes; however, environmental factors and their association significantly affect protein composition (Zhu and Khan, 2001). Among environmental factors, soil fertility status, especially nitrogen concentration, temperature, and moisture, influence cereals grain protein content (Rao et al., 1993). Environmental stresses also impart their influence on milling-quality as indicated by test weight. Test weight is a highly heritable character and has a direct relationship with grain quality, which can find its use for early selection of wheat lines in breeding programs (Troccoli et al., 2000). The crude protein content of cereal declined from 17.21 to 8.51% under salt stress (Fernandez-Figares et al., 2000). Similarly, Darvey et al. (2000) recorded a higher reduction of protein content in wheat compared to triticale. Contrarily, Francois et al. (1986) observed the increment of protein content in durum wheat under salt stress. Environmental factors, especially heat and salt-stressed to promote leaf senescence at grain filling, which promotes protein deposition over accumulation of starch in the grain. It is due to the fact of carbohydrate synthesis, as well as translocation to the grain, is highly prone to adverse and harsh growing conditions compared to protein synthesis and translocation (Fernandez-Figares et al., 2000). Furthermore, it is also established a fact that the protein and starch deposition rate and duration in the cereals grain are entirely independent events, which are influenced and governed by a group of environmental factors (Jenner et al., 1991). Likewise, starch deposition seemingly remains more sensitive under salt stress for shortening of grain filling period in comparison to the deposition of protein. Moreover, optimum environmental factors cause a delay in leaf senescence, which promote nitrogen absorption from the soil and subsequently translocate it to leaves. In this way, a higher grain yield with lower protein content is produced by wheat. The existence of a negative relationship between protein content and grain yield has been reported in wheat, barley, and triticale (manmade cereal developed by crossing wheat and rye) crops (Garcia del Moral et al., 1995). In response to imposed salinity, photosynthetic rate declines as feedback response due to several internal changes leading to higher demand for assimilates; triggering the rate of carbohydrate accumulation and cause a delay in leaf senescence and consequently promoting the onset of Rubisco hydrolysis. The net result is limited N availability, which tends to remobilize toward the grains. Moreover, salinity exposure results in rapid degradation of the RuBisCo enzyme, leading to the higher redistribution of N to the developing grains (Fernandez-Figares et al., 2000).
Gluten Content
The proteins for gluten storage are divided into gliadins (confers extensibility) and glutenins (causes elasticity). Similar to the protein content of the wheat grain, salinity tends to boost wet and dry gluten content in salt-tolerant wheat cultivars, while the opposite has been observed for salt-sensitive cultivars (Khan et al., 2008). Regarding the salt stress effect on the gluten content of wheat grains, it multiplied with increasing salt content in the soil (Shen et al., 2007). In contrast, Kahrizi and Sedghi (2013) inferred that salinity has a very minute impact on the gluten content of cereals grains, while Houshmand et al. (2014) observed that abiotic stresses (salt and drought) result in a significant increment of wet and dry gluten contents. Zheng et al. (2009) also found that the gluten content of wheat cultivars has a direct relationship with the salt concentration of soil. There is a dire need to conduct further in-depth studies to determine the impact of salinity levels on gluten concentration and composition in wheat grain under varying pedo-environmental conditions.
Ash Content
The Ash content of wheat grain represents the mineral constituents of grain. In comparison to saline conditions, optimal growing conditions give rise to the higher ash content of whole-grain owing to improved minerals uptake from the soil solution (Troccoli et al., 2000). Contrarily, Katerji et al. (2005) found an inverse relationship between salinity and ash content of durum wheat. Likewise, Francois et al. (1986) reported that soil salinity reduces ash content of durum as well as semi-dwarf bread wheat; thus, it may be inferred that there are disturbing effects of soil salinity pertaining to the minerals uptake, translocation as well as accumulation processes which result in a severe decline of ash content in wheat grain. Reddy et al. (2003) examined that salinity significantly reduced the grain yield as well as quality along with crop residue quality. Francois et al. (1986) concluded that soil salinity reduced the ash content and improved the flour color as well as protein content. Katerji et al. (2005) reported that excessive salt decreases ash content of wheat, and in this way, wheat grain quality gets improved. Moreover, the direct relationship between water use efficiency (WUE) and ash content of wheat grain can be a useful indicator to select wheat varieties having superior WUE under saline conditions.
Carbohydrate Content
Carbohydrate content is an important indicator of wheat grain quality, which is influenced by salinity stress, especially when wheat plants are exposed to saline environment at the grain filling stage. The synthesis and translocation of carbohydrates are more sensitive to suboptimal growing conditions compared to protein production (Rao et al., 1993). Salinity stress at the post-anthesis stage seriously shortens the accumulation duration of storage proteins leading to modification in gliadins and glutenins accumulation pathways. Moreover, the disruption effects of salinity on photosynthesis rate reduces carbohydrates synthesis at the vegetative growth stage, while it also disrupts or halts the translocation of carbohydrates toward grains at the initiation of grain filling stage, and thus it results in a significant reduction of carbohydrates concentration in wheat grain (Fernandez-Figares et al., 2000).
Beta-Carotene Content
There are rare research investigations that have focused on wheat grain quality, especially beta-carotene content under salinity stress. The beta-carotene contents varied significantly in salt-tolerant and sensitive cultivars of durum wheat under saline conditions. The beta-carotene content of grains recorded a sharp decline in wheat cultivars under salt stress environments (Katerji et al., 2005). Likewise, Francois et al. (1986) reported that both beta-carotene and ash content witnessed a sharp decline owing to salinity. Future studies need to explore the detrimental effect of saline environment on carotene contents with respect to interactive effect of varying genotypes, agro-environmental factors and agronomic management practices along with frequency and intensity of salinity.
Salinity and Nutrient Imbalance
One of the adverse effects of a saline environment, especially high salt concentration in soil solution, causes a severe reduction in the uptake of nutrients and water. Resultantly, osmotic stress intensifies ion toxicity, imbalance of nutrients under water-deficit conditions. Salinity leads to injury of photo-synthetically active leaves by causing chlorosis and triggering leaf senescence in cereals (Hanin et al., 2016). Ion imbalance is one of the most severe impacts caused by a saline environment dominated by NaCl presence, while numerous other ions also multiply the severity of the stress. The combinations of ions in the saline environment determine the types of nutrients which become either deficient or excessive (Hawkins and Lewis, 1993). The underlying mechanism of ionic imbalance has recently been elaborated. Under low-moderate salinity, the nutrient imbalance is prevented by salt ions transportation, which occurs within the vacuole that tends to off-set the ion flux into the cell from the plasma membrane (Blumwald et al., 2000). When the influx rate is higher, ionic homeostasis in cells gets compromised, and anions (Cl−) and cations (Na+, Mg2+, Ca2+) accumulate in the plasmatic compartments (cytosol, matrix, and stroma) of the cell instead of the vacuole, which results in nutrient imbalance especially K and P. To avoid ion imbalance in the short term, accumulation of ions in the apoplast continues, and resultantly no transportation of ions from the apoplast to the symplast occurs. Such ion accumulation in apoplast is usually regarded as the causal mechanism behind salinity-induced ionic imbalance (Speer and Kaiser, 1991). Accumulation of salt in the leaf apoplast disrupts cell water relations leading to wilting (Flowers et al., 1991).
Inorganic ions often perform to be competitive enzyme inhibitors, which host ionic substrates but also interfere with protein surface charges besides destabilizing molecular level interactions. The nutrient imbalance under saline conditions leads Na+ to substitute K+ from the essential binding sites. In biochemistry, the typical instances for K+ dependency are ribosomes and pyruvate kinase. It has been reported that K+ presence in optimal concentration boosts pyruvate kinase activity (Vmax) as much as 400 times (Oria-Hernández et al., 2005), while K+ substitution by Na+ causes inhibition up to 92%. In addition, peptidyl transferase activity in eukaryotic ribosomes gets regulated by K+ concentration and might reach upto 20 s−1 under optimal conditions (Ioannou and Coutsogeorgopoulos, 1997). However, metabolic imbalances occur in the case of drop-in K+ concentration and an increase in Na+ concentration, and such ionic imbalance leads to the initiation of numerous inhibitory processes that cause redox and energy metabolism.
Under normalized conditions, nutrient ion net fluxes of different cereals (maize, wheat, and barley) and legumes such as broad beans get adjusted in accordance with cellular requirements and crop development phases, leading to the establishment of ionic homeostasis (Niu et al., 1995). However, salinity severely disturbs ionic harmony as excessive salt ions (e.g., Na+, Cl− or Mg2+ and ) accumulation alters the composition of the soil solution. Excessive accumulation of the Na+ cation disturbs uptake of many cationic nutrients such as K+ (Wakeel et al., 2011) or Ca2+ (Gardner, 2016); resulting in nutrient imbalance. For instance, excessive Na+ induces Ca2+ deficiency having an appearance like lesions on aerial plant parts along with a reduction in leaf blade dry weight (Maas and Grieve, 1990). Furthermore, Na+ induces K+ uptake reduction leading to declined shoot growth. In contrast, Cl− presence in excessive concentration leads to impairment of nutrient uptake by disturbing anions uptake (Geilfus, 2018a,b). The uptake and anion-anion interactions are antagonistic in nature as the Cl− concentration multiplies by many folds in the soil solution under NaCl salt stress. It has been established that Cl− is greatly mobile in the soil, and negatively charged kaolin and clay minerals significantly repel Cl− leading to its accumulation in the soil macro-pores and soil solution (Thomas and Swoboda, 1970). On the other hand, cations, including Na+, get absorbed in very minute concentration by the negatively charged soil surface under the soil environment (Borggaard, 1984). Moreover, antagonism has been reported among Cl− and nitrate (NO3−) when external Cl− concentrations become too higher (Abdelgadir et al., 2005), which causes a reduction in the growth and yield of wheat (Hu and Schmidhalter, 1998). However, this has not been similar for corn crop (Hütsch et al., 2016). Interestingly, severe competition for anion-anion uptake has also been described for Cl− and phosphate (PO43−). In addition to cereals, such competition has also been reported for tomatoes as well as rose plants (Massa et al., 2009).
Under NaCl salinity, it seems that Cl− tends to hinder the growth and development of crops by inducing the deficiency of phosphorous and sulfur through inhibiting PO43− and SO42− uptake. However, facts remain that generalizable conclusions may not be drawn from the published research and relevant findings. For concise conclusiveness, it becomes pertinent to distinguish between Cl− and counter-cations effect under saline environment. There is a dire need to perform further experiments regarding the behavior of membrane-impermeable counter-cations of a specific salt. Besides, the underlying molecular mechanism of nutrient-nutrient antagonistic uptake remains unclear. However, one of the possible justifications can be attributed to antagonistic competition for a binding site at transport proteins of salts ions. Another justification can be leaking of Cl− from protein pores, which quantitatively displace PO43− or SO42− leading to a sharp decline in their take up. Both above-stated scenarios rely on transmembrane pores, physicochemical attributes such as their charge and size. For instance, hydrated Cl− ion radius is similar to SO42−. It seems that under salinity stress, glycophytic crops did not encounter the necessity to escape Na+ and Cl− uptake during the breeding and evolution process, which hampered the development of adaptive mechanism at the transporter site for differentiating among the requisite nutrients and undesired ions of salts. Thus, it might be inferred that two pronged strategy encompassing reduction in the uptake of salt ions and their replacement in the soil solution as well as plant need further investigations to cope with the serious challenge of salinity and impart sustainability to cereals production.
Water Relation to Salinity Stress
Wheat plants exposed to salt stress change their environmental condition. The capability of plants to tolerate salt is determined by several biochemical ways that facilitate the acquisition or maintenance of water relations, ionic homeostasis, and protect chloroplast functioning. In agriculture, salinity has been the most distressing abiotic stress having pronounced damaging effect on physiological, morphological, and biochemical characteristics of the crop plants, including uptake of water and nutrients, germination, growth, photosynthesis, enzyme actions, and yield (Cisse et al., 2019). Plant water status is hard to measure as it keeps on changing every minute-to-minute. Since stomatal conductance on which it is entirely dependent in the short term (Gil-Muñoz et al., 2020), and psychometric or pressure chamber measurements are often tricky to deliver accurate values (El-Hendawy et al., 2005). In areas where the rainfall is low and the salt remains in the subsoil, increased salt tolerance would allow plants to extract more water (Munns et al., 2006). Mostly wheat productivity is reduced when water stress occurs at heading time, and the reduction is severe when it occurs after anthesis (Barutcular et al., 2016a,b).
Accessibility of water in plants is a crucial factor for all physiological and metabolic processes of plants (Sreenivasulu et al., 2007). The higher concentration of salts causes osmotic stress to plants, which results in low water potential in wheat crop (Qamar et al., 2020). The rate at which new leaves are produced depends basically on the water potential of the soil water, in the same way as for a drought-stressed plant. According to the previous investigations, there is a link between the different physiological reaction of crops to stress and their tolerance mechanisms, viz. high relative water content and water potential (Datta et al., 2011). Singh et al. (2015) reported that salinity prevents seed germination by either exerting osmotic stress that thwarts uptake of water. Such stresses cooperatively inhibit cell expansion and division, as well as modulates the activity of some key enzymes, thus reduces the seed reserves utilization (El-Hendawy et al., 2019).
Water potential of plants at reproductive phases also reduces the cell extension, vascular tissue thickness, flag leaf thickness, mesophyll, and epidermal cell size, which are responsible for the reduction of flag leaf turgidity, flag leaf area, assimilates synthesis, and yield potential (Nassar et al., 2020). Zhang S. et al. (2016) reported that relative water content (RWC) declined by 3.5 and 6.7%, compared to their controls in the salt-tolerant, and salt-sensitive cultivars, respectively, after 6 d of 100 mM NaCl exposure. Further research resulted in a significant reduction in water use efficiency (WUE) of both tolerant and sensitive cultivars under saline field conditions (Gil-Muñoz et al., 2020). The ratio of water content decreased in the root but increased in shoot and spike of other cultivars of wheat (Tammam et al., 2008). Moreover, a direct relationship between WUE and an ash content of wheat grain can be a useful indicator to select wheat varieties having superior WUE under salinity (Zhang et al., 2020).
Consequently, from these findings, we conclude that salinity stress is a major factor for limiting yield and grain quality traits, and it affects the reproductive phase severely by altering ionic homeostasis, water status, and assimilate partitioning. Foliar application of antioxidants and growth regulators that maintain an appropriate water level in the leaves to facilitate adjustment of osmotic and stomatal activity (Arshad et al., 2020). The introduction of deep-rooted crop species is necessary to lower the water table, but salt tolerance will be required not only for the “de-watering” species but also for the annual crops that follow, as salt will be left in the soil when the water table is lowered.
Approaches to Improve Salt Stress Tolerance in Wheat
Improving the crop performance by conventional breeding methods, introducing gene markers, and selection of genetically modified genotypes are the basic approaches to produce tolerance against salinity stress in plants (Hasanuzzaman et al., 2011). In recent year development of transgenics (transfer of genes from tolerant sources) are a promising approach to developing stress-tolerant varieties. Several transgenics are also developed in wheat crop to ameliorate the adverse effects of salinity. Table 1 represents the transgenic developed in wheat crop using the osmoprotectant, ion transporters genes and their source and influenced traits to make salinity tolerant wheat.
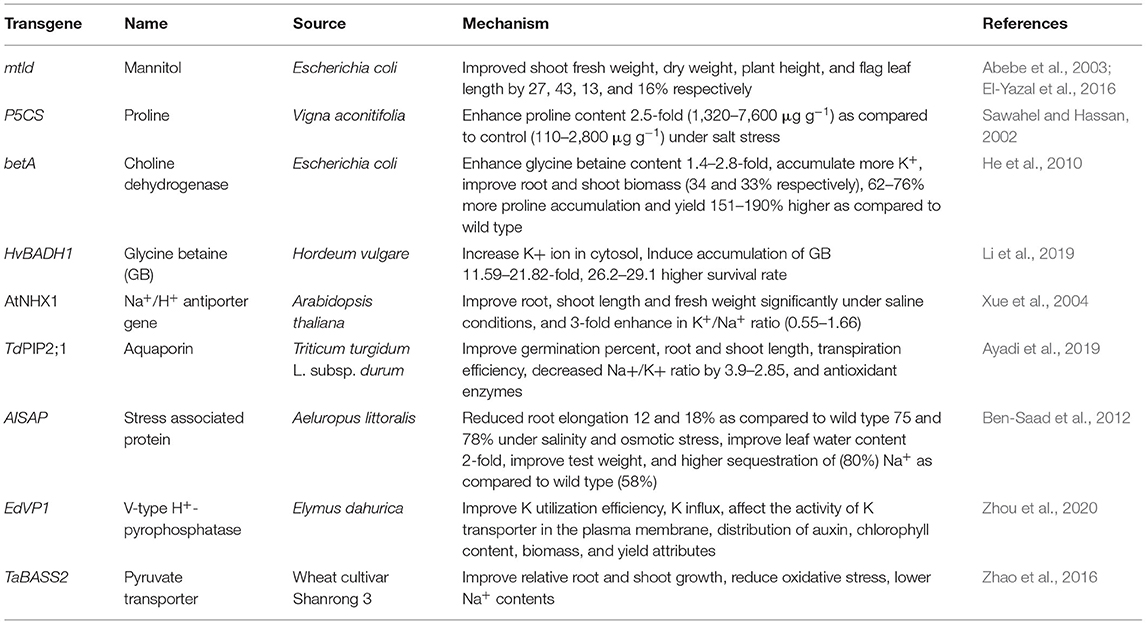
Table 1. Highlights the some transgenic developed in wheat crop and influenced traits for salinity tolerance.
Salt Tolerance Through Osmoprotectants
Various mechanisms are adopted by plants under salinity stress at the organism and tissue level to avoid adverse effects of salinity. Plants produce osmolytes and some beneficial solutes that prevent them from the impact of salinity stress by maintaining osmotic and ionic balance (Ashraf and Foolad, 2007). These compatible solutes and osmoprotectants are uncharged, less toxic soluble organic solutes that provide a -optimal environment to plants under salt stress. Proline, glycine betaine, salicylic acid (SA), and sugar alcohols like trehalose, sorbitol, and mannitol are examples of osmolytes. Membrane stabilization, protein synthesis, and maintenance of osmotic pressure are the important functions performed by these osmolytes and exogenous application of these compounds are helpful for improving salt tolerance in wheat. Plant's exposure to salt stress improves their ability to synthesize osmolytes like glycine betaine, sugar alcohols, and sorbitol that helps them to survive under salinity (Chen and Jiang, 2010). The use of osmolytes was found very vital against salt tolerance induction (Alam et al., 2014). Application of trehalose (10 mM) reduces H2O2 level, lipid peroxidation, free amino acids, proline and LOX enzyme activity, while improves photosynthetic pigments, accumulation of organic solutes and elevating the expression of AOX, NHX1, SOS1 to ion homeostasis under salinity stress (Alla et al., 2019). Further, seed priming and foliar application with plant growth regulators (PGRs), inorganic and organic ion found effective in salinity tolerance as they improve osmolytes production and strength the antioxidant system of plants (Wajid et al., 2019). Recently, the metabolic engineering of osmoprotectants elucidates the mechanisms of salinity tolerance in crops. Further, they also suggested that engineering of these metabolic compounds open a way for understanding the complex nature of salt stress and its relation with other crucial plant processes (Alzahrani, 2021). However, thorough investigations are needed to identify crop-specific osmoprotectants, their dose optimization for exogenous application, time of foliar sprays and stage of crops.
Salinity Tolerance Through Plant Hormones
Salt stress tolerance and plant growth regulation are associated with the biosynthesis of a variety of compounds in minute concentrations, which are termed asplant hormones (Ryu and Cho, 2015). Salt stress was mitigated by several types of plant growth hormones, abscisic acid (ABA), auxins (AUX), cytokinin (CK), and ethylene (ET). Auxin is an important growth regulator that enhances seedling establishment, shoot dry weight, and also balanced the ionic pressure in plants under salinity stress (Iqbal and Ashraf, 2007). Primed seeds with growth regulator auxin mitigated the salinity by improving the hormone balance, nutrients uptake leading to better yield and productivity of both salt resistant and susceptible genotypes. Crop growth rate, leaf area index, photosynthetic efficiency, grain yield, and its quality were improved by seed priming with gibberellic acid (GA) (Shaddad et al., 2013). Foliar applied -GA also enhances salinity tolerance by increasing seedling establishment, plant productivity, and antioxidant enzymatic efficiency under salt stress (Tabatabaei, 2013). Salinity stress could be mitigated by priming with cytokinin that improved seedling establishment, tillers formation, and grain weight under salt-affected soils (Iqbal et al., 2006).
Plant Nutrients
The availability of nutrients is also responsible for mitigating the salt stress effect by various physiochemical and biological mechanisms. Foliar application of potassium improves photosynthesizing efficiency, antioxidant enzymatic efficiency, potassium intake by plants, and sodium absorption and salt stress environments (El-Lethy et al., 2013). Sodium absorption, leaf area index, and biological yield were found to be improved under stressed environments by external application of phosphorous (Khan et al., 2013). Calcium sulfate is also an important nutrient that alleviates the ill effect of salt stress by improving the intake of calcium and potassium and improves crop growth in salinity stress (Zaman et al., 2005). The application of calcium nitrate decreased the peroxidation of lipids and excretion of electrolytes that enhanced the salt tolerance in plants (Tian et al., 2015). Recently the application of inorganic nutrients (Si, K, Zn), organic extract (moringa leaf extract, biochar), as seed or foliar treatments improve the salinity stress tolerance (Jan et al., 2017). Likewise, the application of nanoparticles (Zn, Si) (Mushtaq et al., 2017) showed promising results. Although, the molecular understanding regarding the application of different nutrients and transporters response can accelerate the salinity stress tolerance program in plants.
Salt Tolerance Through Various Signaling Molecules
Various signaling molecules can crosstalk with phytohormones and antioxidants within the plants that help plants to survive under salt stress. Nitric oxide (NO) is the most widely used signaling molecule to mitigate various abiotic stresses, particularly salt stress. It has interaction with other molecules by several pathways due to its signaling role besides improved crop productivity under salt-stressed environments (Hasanuzzaman et al., 2013). A valuable improvement was noticed in the wheat seedling establishment by exogenous application of NO under salt stress. Other than the NO, hydrogen sulfide (H2S), H2O2, and ROS have a significant role in salinity stress tolerance (Khan et al., 2017). These compounds have crucial roles in the regulation of salinity stress tolerance via ion, hormonal, and redox homeostasis in salt-affected cells and reduce the drastic effect by strengthening the antioxidant defense (Bhuyan et al., 2020). Moreover, these compounds have the ability to crosstalk with other signaling compounds and plant growth regulators such as melatonin, ET, ABA, and salicylic acid (Kolbert et al., 2019). Although, the last few years study of gas transmitters (gaseous signaling molecules) under plant abiotic stresses opens gate for further understanding the abiotic stress signaling mechanisms in depth. However, depth study at molecular level still required to complete understanding of abiotic stresses signaling mechanisms.
Use of Plant Growth Hormones in Mitigating Salinity-Induced Damages
Salt stress adversely affects plant growth and related metabolites, and consequently reduces crop yield (Islam et al., 2011). The reduction of plant growth and alteration of related metabolites is evident due to the decline of natural growth hormones in plant tissues (Yurekli et al., 2004). Wang et al. (2001) stated that salinity stress decreased the indole-3-acetic acid (IAA) and salicylic acid (SA), whereas increased the abscisic acid (ABA) and jasmonic acid (JA). Exogenous application of hormones, i.e., plant growth regulators (PGRs), ameliorated the negative effect of salt-induced stress and enhanced the crop yield. PGRs under the saline environment can alleviate the adverse effects of salt stress by enhancing the germination and seedling growth of wheat (Samad and Karmoker, 2012).
Gibberellic Acid (GA3)
Gibberellic acid improved the growth criteria, photosynthetic pigments, and consequently the crop yield of wheat cultivars due to better osmoregulation resulting in increased water flow and water status using the organic solutes (saccharides and proteins), which in turn increased the photosynthetic area and yield (Shaddad et al., 2013). Under saline stress conditions, GA3 stimulated the growth of wheat (Afzal et al., 2005), maize (Hassanein et al., 2009), and sorghum (Azooz et al., 2004). At the same time, GA3 modulation could support abiotic stress tolerance in wheat crop (Abhinandan et al., 2018). GA3 signaling mechanism involved in various developmental and abiotic stress conditions is mediated through an important GA3 signaling molecule called DELLA protein. DELLA protein regulates a complex network of transcription factors and genes (Schwechheimer, 2012). GA3 signaling has shown the targeted deterioration of a group of GA3-response through transcriptional repressors (Golldack et al., 2014). Such signal pathway has observed to be dominant to the success of wheat crop seedlings in the Mediterranean soil under water deficit conditions (Amram et al., 2015). It was found that the endogenous level of GA3 is inhibited under drought and salt stress (Llanes et al., 2016). Uses of GA-inhibiting compounds drought-stressed plants alleviate the negative effects of stress and increase bio weight and yield (Plaza-Wüthrich et al., 2016). It has been also found that GA and auxin (IAA) treatment in wheat reduces the negative effects of ethylene and regulate salinity tolerance in wheat crop (Abd El-Samad, 2013).
Abscisic Acid (ABA)
Abscisic acid has been found to be the main regulator of abiotic stress tolerance in wheat via regulation of protein kinases activities, which is important for phosphorylation processes (Umezawa et al., 2009). The contribution of Snf1-related protein kinases (SnRKs) has been studied extensively in Arabidopsis. Some studies of SnRKs in wheat has shown the presence of other SnRK2 homologs that appear to play a role in ABA-mediated abiotic stress signaling (Mao et al., 2010). The expression of wheat SnRK homologs is stimulated by salt stress, cold stress, drought (induced by PEG), and the application of exogenous ABA (Tian et al., 2013). Likewise, Dong et al. (2013) reported that 12-oxo-phytodienoic acid reductases (OPRs) gene associated with the synthesis of ABA and promotion of ABA-dependent and ROS related stress signaling mechanisms and confer salinity tolerance in wheat. Recently, an ABA functional analog B2 has been shown a positive response under salinity stress by enhancing the expression of ABA-responsive genes and antioxidant activity (Duan, 2020). Moreover, ABA is a crucial stress hormone that regulates the multiple abiotic stresses in the plant including salinity via crosstalk with other signaling molecules and PGRs. To date, numerous genes/transcription factor are identified (TaASN1, TabHLH1) (Yang et al., 2016), which associated with ABA signaling and stress tolerance.
Auxin and Cytokinin
The exogenous application of auxins, cytokinins mitigate the adverse effects of salt stress and consequently improved seed germination and growth (Naidu, 2001; Khan et al., 2004). Auxin and targeted cytokinin signaling is observed to be suppressed during abiotic stress signaling in wheat plants (Abhinandan et al., 2018). Furthermore, the cytokinin and auxin pathways regulate a plethora of developmental processes through their dynamic and complementary actions and their ability to crosstalk support as perfect candidates for mediating stress-adaptation responses (Bielach et al., 2017). Tryptophan derivative auxin is mostly present in the form of indole-3-acetic acid (IAA), and it was decreased in the leaves of wheat under the cold or frost stress (Kosová et al., 2012). It was shown that the cold-sensitive wheat variety was characterized by a high concentration of auxin followed by an acclimatization period connected with reduced fitness. The cytokinin level is reduced early in cold-tolerant wheat varieties as compared to the cold-sensitive ones (Kosová et al., 2012). The imposition of drought and salt stress during the reproductive stages of wheat has been shown to reduce the grain filling, which allied with reduced auxin concentration (Abid et al., 2017). Nishiyama et al. (2011) observed that drought stress decreased the cytokinin biosynthetic enzymes along with suppressed positive cytokinin signaling components, which might improve the drought-tolerance of wheat plants. The reproductive phase of wheat is more sensitive to stress, and the imposition of drought stress at this stage reduced grain filling, which is linked with low levels of auxin and cytokinin, while external cytokinin application rescued this problem (Abid et al., 2017). It has been reported that cytokinin increases the activities of antioxidant enzymes catalase (CAT) and ascorbate peroxidase (APX), prevent cellular damage by ROSs, and shore up antioxidant molecules, xanthophyll (Wilkinson et al., 2012).
Salicylic Acid
The application of salicylic acid (SA) mitigates the harmful effect of abiotic stresses (Abhinandan et al., 2018). External application of SA in wheat has been shown to be a signaling molecule to stimulate the internal radical detoxification system (Noreen et al., 2017; Fardus et al., 2018). Exogenous application of SA in wheat supports antioxidants production via utilizing ROS to be a second messenger (Agarwal et al., 2005a,b). Such responses can reduce oxidative damage under drought, heat, and saline conditions (Fardus et al., 2018). Salicylic acid also induces the accumulation of auxin and ABA, which provides tolerance against salinity stress (Shakirova et al., 2003). Likewise, the seed priming with SA, ABA and ascorbic acid improved the seedling performance by enhancing seedling fresh weight, length, and decrease electrolyte leakage. Although, the authors suggested that the priming with ABA is not so effective (Afzal et al., 2006).
Ethylene
The promotion of abiotic stress tolerance in wheat has been shown by ethylene inhibitors (Abhinandan et al., 2018). Ethylene modulates salinity stress responses largely via maintaining the homeostasis of Na+/K+, nutrients, and ROS by inducing antioxidant defense in addition to elevating the assimilation of nitrates and sulfates. Moreover, a cross-talk of ethylene signaling with other phytohormones has also been observed, which collectively regulate the salinity stress responses in plants (Riyazuddin et al., 2020). Ethylene participation in the later phase of plant growth and development relates to leaf abscission, ripening of fruit, maturation of seeds, and plant drying (Wilkinson et al., 2012). It was shown the role of glycine betaine (GB) and ethylene in salt tolerance variation of different wheat cultivars. The salt-tolerant cultivar exhibited GB content, which was found correlative with ethylene (Khan et al., 2012).
The activation and expression of some ethylene-responsive factors, proteins like TaERF1 and TaERF3, which support resistance to multiple stresses, can be induced by the perception of abscisic acid or ethylene (Rong et al., 2014). The study with isolation and molecular characterization of TdERF1, an ERF gene from durum wheat (Triticum turgidum L. subsp. durum) different varieties showed that TdERF1 gene may provide a discriminating marker between tolerant and sensitive wheat varieties (Makhloufi et al., 2014). The severity as well as stress period dictates the level of ethylene evolution and its response levels.
Salt stress declines the metabolic activity of plant cells, which inevitably reflects the inhibition of plant growth (Çiçek and Çakirlar, 2002). Application of ethephon and zeatin reverse the growth-inhibiting effect of salt stress and increase the shoot and root growth of wheat (Egamberdieva, 2009). Gul et al. (2000) stated that the plant growth stimulating compounds such zeatin, and ethephon alleviate the negative effect of salt stress and increase the germination and growth of Ceratoideslanata, Halogetonglomeratus (Khan et al., 2004).
Brassinosteroids (BRs)
The application of brassinosteroids (BRs) exogenously increases plant tolerance against abiotic stress (Abhinandan et al., 2018). Though the effect of BRs on growth and development of wheat has been studied extensively but has not been much studied at the molecular level. Although a detailed study regarding the BR receptor and other signaling components has been performed in the case of Arabidopsis, there are only limited studies of BRI1 orthologs availability in other taxa. Navarro et al. (2015) reported the presence of a single copy of BRI1 (TaBRI1) in each of three wheat genomes on the long arm of chromosome 3 with in silico analysis. It is very difficult to prove directly that BRs can improve abiotic stress tolerance due to lacking in vivo genetic data. But the exogenous application of BRsis significantly able to improve abiotic stress tolerance in wheat crop. The growing of wheat seedlings under 0.4 μM 24-epibrassinolide treatment has shown high levels of cytokinin in roots and shoots as compared to control plants (Yuldashev et al., 2012). The BRs acts as a defense agent under cadmium (Cd), lead (Pb), and salinity stress-induced Triticum aestivum. Wheat plants grown in cadmium and saline conditions normally have decreased photosynthetic activity and growth, but simulated antioxidant enzymatic activity and increased Pro concentration (Hayat et al., 2014). The manipulation with strigolactone, which is engaged in symbiotic relations in the rhizosphere, provides new ways for generating stress tolerant wheat germplasm (Abhinandan et al., 2018). Plants treated with synthetic SL analog GR24 exogenously under drought stress conditions significantly increased the thousand-grain weight as compare to non-treated drought-stressed two winter wheat cultivars (Sedaghat et al., 2017). Future research need to focus the vital role of synthetic and natural hormones for alleviating salinity stress as various hormones hold potential to modulate and regulate vital metabolic processes under stressful environment.
Plant Growth-Promoting Bacteria (PGPB)
Plant growth-promoting bacteria directly or indirectly enhanced the growth of plants (Ahmad et al., 2013b). It has been reported by Kotuby-Amazher et al. (2000) that beneficial microorganisms could reduce salt stress by ~50% in wheat. PGPB produce some beneficial compounds like indole acetic acid, siderophore, HCN, etc., solubilize minerals and break down organic matters for easy uptake by plants, fix atmospheric nitrogen, and produce siderophores that enhance the bioavailability of iron, and synthesize phytohormones such as cytokinin's, auxins and gibberellins which have beneficial roles in various stages of plant growth (Richardson and Simpson, 2011). PGPB also decreases or inhibits the detrimental effects of pathogenic organisms by enhancing the host resistance to pathogenic organisms (Van Loon, 2007). Orhan (2016) reported that halotolerant and halophilic bacterial strains significantly increased the root and shoot length, and total fresh weight of wheat under severe salt stress (200 mM NaCl). IAA is declined due to the negative effect of salt stress (Wang et al., 2001), and bacterial strains P. aureantiaca TSAU22, P. extremorientalis TSAU6, and P. extremorientalis TSAU20 produce IAA, which alleviate the reductive effect of salt stress and increase the germination percentage (up to 79%) of wheat (Egamberdieva, 2009).
The cross talks of signaling molecules with other PGRs compounds in wheat crop under salinity are highlighted in Table 2. In this table different signaling molecules and PGRs are participating in common signaling pathways and regulates the multiple stresses tolerance.
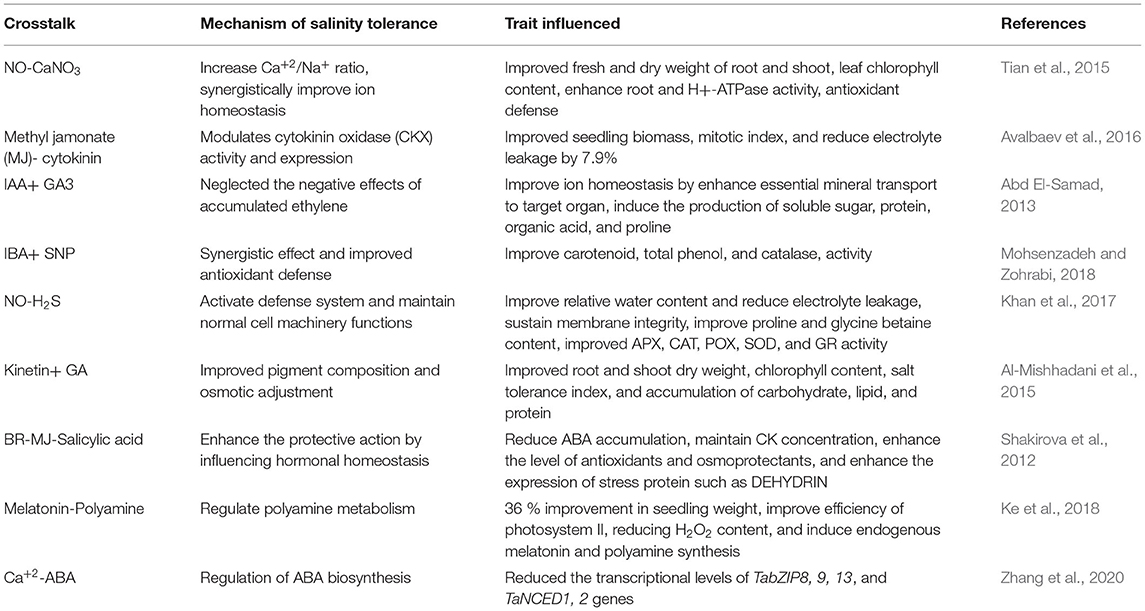
Table 2. Highlights the crosstalk between plant growth regulators and signaling compounds to ameliorate the salinity stress in wheat crop.
Antioxidant Defense in Response to Salinity-Induced Oxidative Stress
Salinity stress hampers the antioxidant strength via altering antioxidant enzyme activity viz. biosynthesis of ascorbate peroxidase (APX), sodium dismutase (SOD), and glutathione reductase (GR), and non-enzyme antioxidant (ascorbic acid, glycine betaine, and proline). These changes in antioxidants cause the accumulation of harmful ROS, malondialdehyde (MDA), and elevation of lipid peroxidation, ion leakage, membrane stability, and ultimately weakening of antioxidant system (Sairam et al., 2002).
Oxidative stress is caused by production and accumulation of ROS in cells and tissues owing to irregularities in the electron transport chain (ETC) that cause lipid peroxidation, protein oxidation, nucleic acid damage, enzyme inhibition, activation of programmed cell death pathway, and ultimately causing cell death (Hossain et al., 2021). In the biological system, the plants can generally detoxify these reactive products. Under normal growth conditions of the plant, toxic oxygen metabolites are produced in low volume, and there is desired balance between production and ROS quenching; however, this balance may be disturbed by several adverse conditions, including salinity stress. The common ROS are superoxide radicals (O2∙−), hydrogen peroxide (H2O2), hydroxyl radicals (∙OH), and singlet oxygen (1O2) (Navarro-Yepes et al., 2014). All ROS are extremely deleterious to plants at higher concentration. To understand salt adaptation strategies of plants, it is an important task of plant biologists to find out suitable mechanisms for developing salt-tolerant wheat that can produce sufficient yield under stress (Kaur et al., 2017). Priming and exogenous use of different eco-friendly compounds had been exploited to tolerate the salt stress-induced oxidative stress in different crops, including wheat (Kaur et al., 2017).
Several antioxidants have been exploited in recent years, which have a beneficial effect against oxidative stress, and to avoid oxidative damage higher plants usually raise the concentration of the endogenous antioxidant system comprising of enzymatic and non-enzymatic components to scavenge ROS (Sharma et al., 2012). In-plant cells, specific ROS producing, and scavenging systems have been established in different organelles viz., mitochondria, chloroplast, and peroxisomes etc. The enzymatic components of the antioxidative defense system (ADS) are comprised of several antioxidant enzymes like superoxide dismutase (SOD), catalase (CAT), peroxidase (POX), guaiacol peroxidase (GPX), ascorbate peroxidase (APX), monodehydroascorbate reductase (MDHAR), dehydroascorbate reductase (DHAR), and glutathione reductase (GR) (Hossain et al., 2021). The non-enzymic components of the ADS include the major cellular redox buffers, ascorbate (AsA) and glutathione (GSH), as well as tocopherol, carotenoids, and phenolic compounds (Mandhania et al., 2006). They interact with numerous cellular components and, in addition to crucial roles in defense and as enzyme cofactors (Rakhmankulova et al., 2015). Ascorbate has remained the most abundant, low molecular weight antioxidant playing a vital role in defense against oxidative stress caused by increased ROS level by scavenging and H2O2 (Karuppanapandian et al., 2011). Mandhania et al. (2006) found the NaCl induced enhancement of peroxidase activity in salinized cells of wheat by decomposition of H2O2 generated by antioxidant SOD. Catalase is another enzyme involved in H2O2 detoxification and its conversion into water and oxygen. Similar results were also found by Sairam et al. (2002), who reported enhancement in CAT activity in both salt-sensitive as well as salt-tolerant wheat cultivars. Mandhania et al. (2006) also reported that stimulation of APX and GR activity under salt stress was much higher in the salt-tolerant cultivar. Kaur et al. (2017) found that exogenous application of phenolic acids (caffeic and sinapic acids) upregulated the stressed shoots of a salt-tolerant wheat cultivar by increasing the activity of CAT and peroxidase. In comparison to stress plants, without the application of phenolic acids, APX activity was upregulated in stressed seedlings, and GB was increased in the stressed roots. Phenolic acids being major non-enzymatic components of the defense system can be used to enhance endogenous levels of these antioxidants in wheat seedlings grown under salt stress conditions. In contrast, wheat crop crucial plant processes are affected by the salinity stress. Therefore, soil and agronomical (drainage system management, leaching of salt, nutrient managements), physiological (osmotic adjustment, seed priming, improve photosynthesis efficiency, and water relation), biochemical (redox, ion, and hormonal homeostasis), and molecular (development of transgenic, genetical engineering, identification of gene) are the crucial step in wheat salinity stress tolerant program (Figure 3).
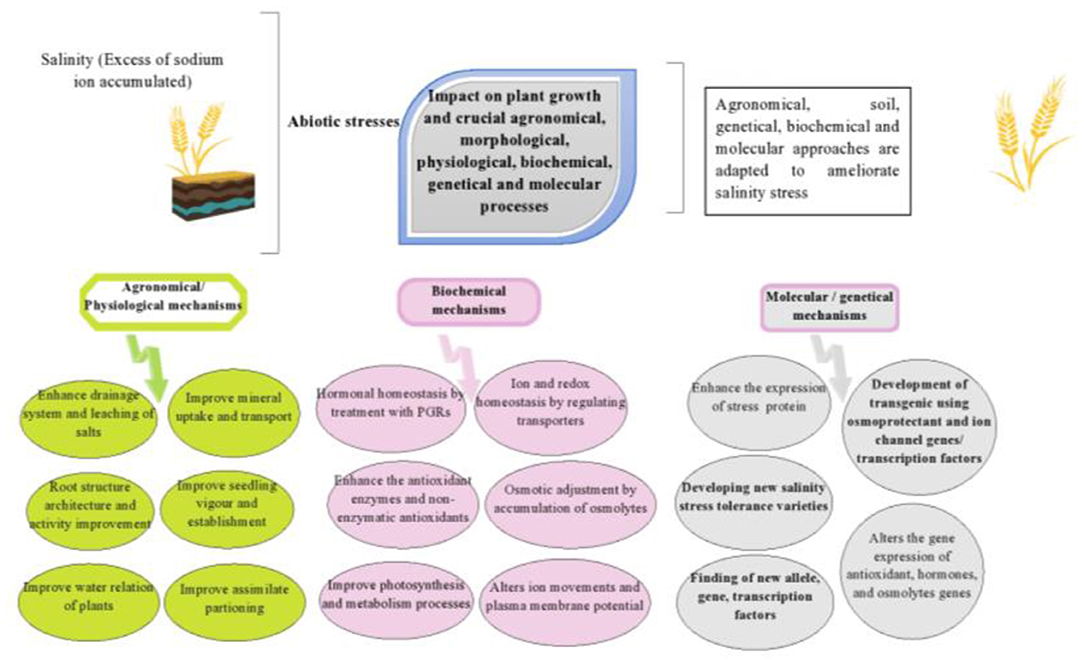
Figure 3. Illustrate the integrated approaches (physiological, biochemical and molecular) of salinity stress tolerance in wheat crop.
Conclusions
Among abiotic stresses, salinity stress especially in the arid and semi-arid regions of the world is one of has emerged as one of the most important threats to the sustainability of wheat production. It reduces germination, seedling growth as well as reproductive growth by disrupting numerous vital physiological and metabolic processes which lead to sharp decline in yield and quality depending on frequency and extent of saline environment. Although salinity tolerant plants employ several physiological and biochemical mechanisms to adapt under salinity stress, there is a lack of robust salinity tolerant wheat cultivars globally. Therefore, plant physiologists, breeders, and agronomists need to develop an integrated and sustainable strategy to enhance salt tolerance in wheat. Among these mitigation strategies, soil management practices, crop establishment, as well as the foliar application of antioxidants and growth regulators through maintaining an appropriate water level in the leaves to facilitate adjustment of osmotic and stomatal performance could be explored further to mitigate the adverse effect of salinity on wheat yield and grain quality. However, breeding strategies especially gene pyramiding should be undertaken to develop salt-tolerant varieties by exploring halotolerant gene homologs from wheat germplasm. However, an integrated approach involving soil and agronomic practices (drainage system management, salt leaching, nutrients managements for salt ions replacement), physiological strategies (osmotic adjustment, seed priming, improve photosynthesis efficiency, and water relation), biochemical (redox, ion, and hormonal homeostasis), and molecular tools (development of transgenic, genetic engineering, identification of gene, genes insertion, editing, or slicing) needs to be developed to ameliorate salinity effects and boost cereal production on sustainable basis.
Author Contributions
All authors listed have made a substantial, direct and intellectual contribution to the work, and approved it for publication.
Conflict of Interest
The authors declare that the research was conducted in the absence of any commercial or financial relationships that could be construed as a potential conflict of interest.
References
Abbas, G., Saqib, M., Rafique, Qur-Rahman, M. A., Akhtar, J., ul- Haq, M. A., and Nasim, M. (2013). Effect of salinity on grain yield and grain quality of wheat (Triticum aestivum L.). Pakistan J. Agric. Res.. 50, 185–189.
Abd El-Samad, H. M. (2013). The physiological response of wheat plants to exogenous application of gibberellic acid (GA3) or indole-3-acetic acid (IAA) with endogenous ethylene under salt stress conditions. Int. J. Plant Physiol. Biochem. 5, 58–64. doi: 10.5897/IJPPB12.016
Abdelgadir, E. M., Oka, M., and Fujiyama, H. (2005). Characteristics of nitrate uptake by plants under salinity. J. Plant Nutr. 28, 33–46. doi: 10.1081/PLN-200042156
Abebe, T., Guenzi, A. C., Martin, B., and Cushman, J. C. (2003). Tolerance of mannitol-accumulating transgenic wheat to water stress and salinity. Plant Physiol. 131, 1748–1755. doi: 10.1104/pp.102.003616
Abhinandan, K., Skori, L., Stanic, M., Hickerson, N., Jamshed, M., and Samuel, M. A. (2018). Abiotic stress signaling in wheat–an inclusive overview of hormonal interactions during abiotic stress responses in wheat. Front. Plant Sci. 9:734. doi: 10.3389/fpls.2018.00734
Abid, M., Shao, Y., Liu, S., Wang, F., Gao, J., and Jiang, D. (2017). Pre-drought priming sustains grain development under post-anthesis drought stress by regulating the growth hormones in winter wheat (Triticum aestivum L.). Planta 246, 509–524. doi: 10.1007/s00425-017-2698-4
Afzal, I., Basra, S. A., and Iqbal, A. (2005). The effects of seed soaking with plant growth regulators on seedling vigor of wheat under salinity stress. J. Stress Physiol. Biochem. 1, 6–14.
Afzal, I., Basra, S. M., Farooq, M., and Nawaz, A. (2006). Alleviation of salinity stress in spring wheat by hormonal priming with ABA, salicylic acid and ascorbic acid. Int. J. Agric. Biol. 8, 23–28.
Agarwal, S., Sairam, R. K., Srivastava, G. C., and Meena, R. C. (2005a). Changes in antioxidant enzymes activity and oxidative stress by abscisic acid and salicylic acid in wheat genotypes. Biol. Plant. 49, 541–550. doi: 10.1007/s10535-005-0048-z
Agarwal, S., Sairam, R. K., Srivastava, G. C., Tyagi, A., and Meena, R. C. (2005b). Role of ABA, salicylic acid, calcium and hydrogen peroxide on antioxidant enzymes induction in wheat seedlings. Plant Sci. 169, 559–570. doi: 10.1016/j.plantsci.2005.05.004
Ahmad, M., Shahzad, A., Iqbal, M., Asif, M., and Hirani, A. H. (2013a). Morphological and molecular genetic variation in wheat for salinity tolerance at germination and early seedling stage. Austral. J. Crop Sci. 7:66.
Ahmad, M., Zahir, Z. A., Nazli, F., Akram, F., Arshad, M., and Khalid, M. (2013b). Effectiveness of halo-tolerant, auxin producing Pseudomonas and Rhizobium strains to improve osmotic stress tolerance in mung bean (Vigna radiata L.). Brazil. J. Microbiol. 44, 1341–1348. doi: 10.1590/S1517-83822013000400045
Alam, M. M., Nahar, K., Hasanuzzaman, M., and Fujita, M. (2014). Trehalose-induced drought stress tolerance: a comparative study among different Brassica species. Plant Omics 7:271.
Ali, A., Basra, S. M. A., Ahmad, R., and Wahid, A. (2009). Optimizing silicon application to improve salinity tolerance in wheat. Soil Environ. 28, 136–144.
Alla, M. N., Badran, E., and Mohammed, F. (2019). Exogenous trehalose alleviates the adverse effects of salinity stress in wheat. Turkish J. Bot. 43, 48–57. doi: 10.3906/bot-1803-36
Al-Mishhadani, I. I., Ismail, E. N., Jaddoa, K. A., Majeed, D. M., and Mohammed, O. A. (2015). Estimation of the interaction effect between salinity and growth regulators on salt tolerance of two bread wheat cultivars. Int. J. Appl. Agric. Sci. 1, 95–101. doi: 10.11648/j.ijaas.20150104.12
Alzahrani, F. O. (2021). Metabolic engineering of osmoprotectants to elucidate the mechanism (s) of salt stress tolerance in crop plants. Planta 253, 1–17. doi: 10.1007/s00425-020-03550-8
Amirbakhtiar, N., Ismaili, A., Ghaffari, M. R., Nazarian Firouzabadi, F., and Shobbar, Z. S. (2019). Transcriptome response of roots to salt stress in a salinity-tolerant bread wheat cultivar. PLoS ONE 14:e0213305. doi: 10.1371/journal.pone.0213305
Amram, A., Fadida-Myers, A., Golan, G., Nashef, K., Ben-David, R., and Peleg, Z. (2015). Effect of GA-sensitivity on wheat early vigor and yield components under deep sowing. Front. Plant Sci. 6:487. doi: 10.3389/fpls.2015.00487
Apel, K., and Hirt, H. (2004). Reactive oxygen species: metabolism, oxidative stress, and signal transduction. Annu. Rev. Plant Biol. 55, 373–399. doi: 10.1146/annurev.arplant.55.031903.141701
Arif, Y., Singh, P., Siddiqui, H., Bajguz, A., and Hayat, S. (2020). Salinity induced physiological and biochemical changes in plants: an omic approach towards salt stress tolerance. Plant Physiol. Biochem. 156, 64–77. doi: 10.1016/j.plaphy.2020.08.042
Arora, N. K. (2019). Impact of climate change on agriculture production and its sustainable solutions. Environ. Sustain. 2, 95–96. doi: 10.1007/s42398-019-00078-w
Arshad, A., Qamar, H., Siti-Sundari, R., Zhang, Y., Zubair, M., Raza, M. A., et al. (2020). Phenotypic plasticity of spineless safflower (Carthamus tinctorius L.) cultivars in response to exogenous application of salicylic acid under rainfed climate conditions. Pakistan J. Agric. Res. 33, 729–743. doi: 10.17582/journal.pjar/2020/33.4.729.743
Arzani, A., and Ashraf, M. (2016). Smart engineering of genetic resources for enhanced salinity tolerance in crop plants. Critic. Rev. Plant Sci. 35, 146–189. doi: 10.1080/07352689.2016.1245056
Ashraf, M., and Foolad, M. R. (2007). Roles of glycine betaine and proline in improving plant abiotic stress resistance. Environ. Exp. Bot. 59, 206–216. doi: 10.1016/j.envexpbot.2005.12.006
Ashraf, M. A., and Ashraf, M. (2016). Growth stage-based modulation in physiological and biochemical attributes of two genetically diverse wheat (Triticum aestivum L.) cultivars grown in salinized hydroponic culture. Environ. Sci. Pollut. Res. 23, 6227–6243. doi: 10.1007/s11356-015-5840-5
Asseng, S., Ewert, F., Martre, P., Rötter, R. P., Lobell, D. B., Cammarano, D., et al. (2015). Rising temperatures reduce global wheat production. Nat. Clim. Change 5, 143–147. doi: 10.1038/nclimate2470
Avalbaev, A., Yuldashev, R., Fedorova, K., Somov, K., Vysotskaya, L., Allagulova, C., et al. (2016). Exogenous methyl jasmonate regulates cytokinin content by modulating cytokinin oxidase activity in wheat seedlings under salinity. J. Plant Physiol. 191, 101–110. doi: 10.1016/j.jplph.2015.11.013
Ayadi, M., Brini, F., and Masmoudi, K. (2019). Overexpression of a wheat aquaporin gene, TdPIP2; 1, enhances salt and drought tolerance in transgenic durum wheat cv. maali. Int. J. Mol. Sci. 20:2389. doi: 10.3390/ijms20102389
Azooz, M. M., Shaddad, M. A., and Abdel-Latef, A. A. (2004). Leaf growth and K+/Na+ ratio as an indication of the salt tolerance of three sorghum cultivars grown under salinity stress and IAA treatment. Acta Agron. Hungarica 52, 287–296. doi: 10.1556/AAgr.52.2004.3.10
Bacilio, M., Rodriguez, H., Moreno, M., Hernandez, J. P., and Bashan, Y. (2004). Mitigation of salt stress in wheat seedlings by a gfp-tagged Azospirillum lipoferum. Biol. Fert. Soils 40, 188–193. doi: 10.1007/s00374-004-0757-z
Barutcular, C., Yildirim, M., Koc, M., Akinc,i, C., Tanrikulu, A., El Sabagh, A., et al. (2016b). Quality traits performance of bread wheat genotypes under drought and heat stress conditions. Fresenius Environ. Bull. 25, 6159–6165.
Barutcular, C., Yildirim, M., Koc, M., Akinci, C., Toptaş, I., Albayrak, O., et al. (2016a). Evaluation of SPAD chlorophyll in spring wheat genotypes under different environments. Fresenius Environ. Bull. 25, 1258–1266.
Ben-Saad, R., Ben-Ramdhan, W., Zouari, N., Azaza, J., Mieulet, D., Guiderdoni, E., et al. (2012). Marker-free transgenic durum wheat cv. Karim expressing the AlSAP gene exhibits a high level of tolerance to salinity and dehydration stresses. Mol. Breeding 30, 521–533. doi: 10.1007/s11032-011-9641-3
Bhuyan, M. B., Hasanuzzaman, M., Parvin, K., Mohsin, S. M., Al Mahmud, J., Nahar, K., et al. (2020). Nitric oxide and hydrogen sulfide: two intimate collaborators regulating plant defense against abiotic stress. Plant Growth Regul. 90, 409–424. doi: 10.1007/s10725-020-00594-4
Bielach, A., Hrtyan, M., and Tognetti, V. B. (2017). Plants under stress: Involvement of auxin and cytokinin. Int. J. Mol. Sci. 18:1427. doi: 10.3390/ijms18071427
Blumwald, E., Aharon, G. S., and Apse, M. P. (2000). Sodium transport in plant cells. Biochim. Biophys. Acta 1465, 140–151. doi: 10.1016/S0005-2736(00)00135-8
Borggaard, O. K. (1984). Influence of iron oxides on the non-specific anion (chloride) adsorption by soil. J. Soil Sci. 35, 71–78. doi: 10.1111/j.1365-2389.1984.tb00261.x
Branlard, G., Dardevet, M., Saccomano, R., Lagoutte, F., and Gourdon, J. (2001). Genetic diversity of wheat storage proteins and bread wheat quality. Euphytica 119, 59–67. doi: 10.1007/978-94-017-3674-9_18
Bui, E. N. (2013). Soil salinity: a neglected factor in plant ecology and biogeography. J. Arid Environ. 92, 14–25. doi: 10.1016/j.jaridenv.2012.12.014
Chamorro, D., Luna, B., Ourcival, J. M., Kavgac,i, A., Sirca, C., Mouillot, F., et al. (2017). Germination sensitivity to water stress in four shrubby species across the Mediterranean Basin. Plant Biol. 19, 23–31. doi: 10.1111/plb.12450
Chaves, M. M., Maroco, J. P., and Pereira, J. S. (2003). Understanding plant responses to drought-from genes to the whole plant. Funct. Plant Biol. 30, 239–264 doi: 10.1071/FP02076
Chen, H., and Jiang, J. G. (2010). Osmotic adjustment and plant adaptation to environmental changes related to drought and salinity. Environ. Rev. 18, 309–319. doi: 10.1139/A10-014
Çiçek, N., and Çakirlar, H. (2002). The effect of salinity on some physiological parameters in two maize cultivars. Bulgarian J. Plant Physiol. 28, 66–74.
Cisse, A., Arshad, A., Wang, X., Yattara, F., and Hu, Y. (2019). Contrasting impacts of long-term application of biofertilizers and organic manure on grain yield of winter wheat in North China Plain. Agronomy 9:312. doi: 10.3390/agronomy9060312
Dadshani, S., Sharma, R. C., Baum, M., Ogbonnaya, F. C., Léon, J., and Ballvora, A. (2019). Multi-dimensional evaluation of response to salt stress in wheat. PLoS ONE 14:e0222659. doi: 10.1371/journal.pone.0222659
Darvey, N. L., Naeem, H., and Gustafson, J. P. (2000). “Triticale: production and utilization,” in Handbook of Cereal Science and Technology, eds K. Klup and J. Ponte J. 2nd edn (New York, NY: Marcel Dekker).
Datta, J. K., Mondal, T., Banerjee, A., and Mondal, N. K. (2011). Assessment of drought tolerance of selected wheat cultivars under laboratory condition. J. Agric. Technol. 7, 383–393.
Dehnavi, A. R., Zahedi, M., Ludwiczak, A., Cardenas Perez, S., and Piernik, A. (2020). Effect of salinity on seed germination and seedling development of sorghum (Sorghum bicolor (L.) Moench) genotypes. Agronomy 10:859. doi: 10.3390/agronomy10060859
Dong, W., Wang, M., Xu, F., Quan, T., Peng, K., Xiao, L., et al. (2013). Wheat oxophytodienoate reductase gene TaOPR1 confers salinity tolerance via enhancement of abscisic acid signaling and reactive oxygen species scavenging. Plant Physiol. 161, 1217–1228. doi: 10.1104/pp.112.211854
Duan, l. (2020). A novel aba functional analogue b2 enhances salinity tolerance in wheat. Appl. Ecol. Environ. Res. 18, 7139–7157. doi: 10.15666/aeer/1805_71397157
Egamberdieva, D. (2009). Alleviation of salt stress by plant growth regulators and IAA producing bacteria in wheat. Acta Physiol. Plant. 31, 861–864. doi: 10.1007/s11738-009-0297-0
Ehtaiwesh, F. A., and Rashed, H. F. (2020). Growth and yield responses of libyan hard wheat (Triticum durum Desf) genotypes to salinity stress. Zawia Univ. Bull. 22, 33–58.
El Sabagh, A., Hossain, A., Barutçular, C., Iqbal, M. A., Islam, M. S., Fahad, S., et al. (2020). “Consequences of salinity stress on the quality of crops and its mitigation strategies for sustainable crop production: an outlook of arid and semi-arid regions,” in Environment, Climate, Plant and Vegetation Growth, eds A. Fahad, M. Hasanuzzaman, M. Alam, H. Ullah, M. Saeed, I. A. Khan, and M. Adnan (Cham: Springer), 503–533. doi: 10.1007/978-3-030-49732-3_20
El Sabagh, A., Hossain, A., Barutçular, C., Islam, M. S., Ratnasekera, D., Kumar, N., et al. (2019b). Drought and salinity stress management for higher and sustainable canola (Brassica napus L.) production: a critical review. Austral. J. Crop Sci. 13, 88–97. doi: 10.21475/ajcs.19.13.01.p1284
El Sabagh, A., Hossain, A., Islam, M. S., Barutcular, C., Hussain, S., Hasanuzzaman, M., et al. (2019a). Drought and salinity stresses in barley: consequences and mitigation strategies. Austral. J. Crop Sci. 13:810. doi: 10.21475/ajcs.19.13.06.p1286
El Sabagh, A., Hossain, A., Islam, M. S., Barutçular, C., Ratnasekera, D., Kumar, N., et al. (2019c). Sustainable soybean production and abiotic stress management in saline environments: a critical review. Austr. J. Crop Sci. 13, 228–236. doi: 10.21475/ajcs.19.13.02.p1285
El-Hendawy, S., Elshafei, A., Al-Suhaibani, N., Alotabi, M., Hassan, W., Dewir, Y. H., et al. (2019). Assessment of the salt tolerance of wheat genotypes during the germination stage based on germination ability parameters and associated SSR markers. J. Plant Interact. 14, 151–163. doi: 10.1080/17429145.2019.1603406
El-Hendawy, S. E., Hu, Y., and Schmidhalter, U. (2005). Growth, ion content, gas exchange, and water relations of wheat genotypes differing in salt tolerances. Austral. J. Agric. Res. 56, 123–134. doi: 10.1071/AR04019
El-Hendawy, S. E., Ruan, Y., Hu, Y., and Schmidhalter, U. (2009). A comparison of screening criteria for salt tolerance in wheat under field and controlled environmental conditions. J. Agron. Crop Sci. 195, 356–367. doi: 10.1111/j.1439-037X.2009.00372.x
El-Lethy, S. R., Abdelhamid, M. T., and Reda, F. (2013). Effect of potassium application on wheat (Triticum aestivum L.) cultivars grown under salinity stress. World Appl. Sci. J. 26, 840–850. doi: 10.5829/idosi.wasj.2013.26.07.13527
El-Yazal, M. A. S., Eissa, H. F., Ahmed, S. M. A. E., Howladar, S. M., Zaki, S. N. S., and Rady, M. M. (2016). The mtlD gene-overexpressed transgenic wheat tolerates salt stress through accumulation of mannitol and sugars. Plant 4, 78–90. doi: 10.11648/j.plant.20160406.15
Eroglu, Ç., Cabral, C., Ravnskov, S., Topbjerg, H., and Wollenweber, B. (2020). Arbuscular mycorrhiza influences carbon-use efficiency and grain yield of wheat grown under pre- and post-anthesis salinity stress. Plant Biol. 22, 863–871. doi: 10.1111/plb.13123
FAO (2009). High Level Expert Forum-How to Feed the World in 2050. Economic and Social Development. Rome: Food and Agricultural Organization of the United Nations.
Fardus, J., Matin, M. A., Hasanuzzaman, M., Hossain, M. A., and Hasanuzzaman, M. (2018). Salicylic acid-induced improvement in germination and growth parameters of wheat under salinity stress. J. Anim. Plant Sci. 28, 197–207.
Farooq, S., and Azam, F. (2005). The use of cell membrane stability (CMS) technique to screen for salt tolerant wheat varieties. J. Plant Physiol. 163, 629–637. doi: 10.1016/j.jplph.2005.06.006
Farouk, S. (2011). Ascorbic acid and α-tocopherol minimize salt-induced wheat leaf senescence. J. Stress Physiol. Biochem. 7, 58–79.
Fernandez-Figares, I., Marinetto, J., Royo, C., Ramos, J. M., and Del Moral, L. G. (2000). Amino-acid composition and protein and carbohydrate accumulation in the grain of triticale grown under terminal water stress simulated by a senescing agent. J. Cereal Sci. 32, 249–258. doi: 10.1006/jcrs.2000.0329
Flowers, T. J., Hajibagherp, M. A., and Yeo, A. R. (1991). Ion accumulation in the cell walls of rice plants growing under saline conditions: evidence for the Oertli hypothesis. Plant Cell Environ. 14, 319–325. doi: 10.1111/j.1365-3040.1991.tb01507.x
Food and Agriculture Organization (FAO) of the United Nations (2016). Pulses: Nutritious Seeds for a Sustainable Future. Rome: Food and Agriculture Organization of the United Nations.
Francois, L. E., Maas, E. V., Donovan, T. J., and Youngs, V. L. (1986). Effect of salinity on grain yield and quality, vegetative growth, and germination of semi-dwarf and durum wheat. Agron. J. 78, 1053–1058. doi: 10.2134/agronj1986.00021962007800060023x
Frank, A. B., Bauer, A., and Black, A. I. (1987). Effects of air temperature and water stress on apex development in spring wheat. Crop Sci. 27, 113–116. doi: 10.2135/cropsci1987.0011183X002700010028x
Garcia del Moral, L. F., Boujenna, A., Yanez, J. A., and Ramos, J. M. (1995). Forage production, grain yield, and protein content in dual-purpose triticale grown for both grain and forage. Agron. J. 87, 902–908. doi: 10.2134/agronj1995.00021962008700050021x
Gardner, W. K. (2016). Sodium, calcium and magnesium ratios in soils of NW Victoria, Australia may restrict root growth and crop production. J. Plant Nutr. 39, 1205–1215. doi: 10.1080/01904167.2014.999946
Geilfus, C. M. (2018a). Chloride from nutrient to toxicant. Plant Cell Physiol. 59, 877–886. doi: 10.1093/pcp/pcy071
Geilfus, C. M. (2018b). Review on the significance of chlorine for crop yield and quality. Plant Sci. 270, 114–122. doi: 10.1016/j.plantsci.2018.02.014
Ghosh, B., Md, N. A., and Gantait, S. (2016). Response of rice under salinity stress: a review update. Rice Res. 4:167. doi: 10.4172/2375-4338.1000167
Gil-Muñoz, F., Pérez-Pérez, J. G., Quiñones, A., Primo-Capella, A., Cebolla, J., Forner-Giner, M. Á., et al. (2020). A cross population between D. kaki and D. virginiana shows high variability for saline tolerance and improved salt stress tolerance. PLoS ONE 15:e0229023. doi: 10.1371/journal.pone.0229023
Giraldo, P., Benavente, E., Manzano-Agugliaro, F., and Gimenez, E. (2019). Worldwide research trends on wheat and barley: a bibliometric comparative analysis. Agronomy 9:352. doi: 10.3390/agronomy9070352
Golldack, D., Li, C., Mohan, H., and Probst, N. (2014). Tolerance to drought and salt stress in plants: unraveling the signaling networks. Front. Plant Sci. 5:151. doi: 10.3389/fpls.2014.00151
Grieve, C. M., Francois, L. E., and Maas, E. V. (1994). Salinity affects the timing of phasic development in spring wheat. Crop Sci. 34, 1544–1549. doi: 10.2135/cropsci1994.0011183X003400060024x
Gul, B., Khan, M. A., and Weber, D. J. (2000). Alleviation of salinity and dark-enforced dormancy in Allenrolfea occidentalis seeds under various thermoperiods. Austral. J. Bot. 48, 745–752. doi: 10.1071/BT99069
Hanin, M., Ebel, C., Ngom, M., Laplaze, L., and Masmoudi, K. (2016). New insights on plant salt tolerance mechanisms and their potential use for breeding. Front. Plant Sci. 7:1787. doi: 10.3389/fpls.2016.01787
Hasan, A., Hafiz, H. R., Siddiqui, N., Khatun, M., Islam, R., and Mamun, A. A. (2015). Evaluation of wheat genotypes for salt tolerance based on some physiological traits. J. Crop Sci. Biotechnol. 18, 333–340. doi: 10.1007/s12892-015-0064-2
Hasanuzzaman, M., Alam, M., Rahman, A., Hasanuzzaman, M., Nahar, K., and Fujita, M. (2014). Exogenous proline and glycine betaine mediated upregulation of antioxidant defense and glyoxalase systems provides better protection against salt-induced oxidative stress in two rice (Oryza sativa L.) varieties. BioMed. Res. Int. 2014:757219. doi: 10.1155/2014/757219
Hasanuzzaman, M., Hossain, M. A., and Fujita, M. (2011). Selenium-induced up-regulation of the antioxidant defense and methylglyoxal detoxification system reduces salinity-induced damage in rapeseed seedlings. Biol. Trace Element Res. 143, 1704–1721. doi: 10.1007/s12011-011-8958-4
Hasanuzzaman, M., Nahar, K., and Fujita, M. (2013). “Plant responses to salt stress and role of exogenous protectants to mitigate salt-induced damages,” in Ecophysiology and Responses of Plants Under Salt Stress, eds P. Ahmad P, M. M. Azooz, and M. N. V. Prasad MNV (New York, NY: Springer), 25–87. doi: 10.1007/978-1-4614-4747-4_2
Hassanein, R. A., Hassanein, A. A., Haider, A. S., and Hashem, H. A. (2009). Improving salt tolerance of Zea mays L. plants by presoaking their grains in glycine betaine. Austral. J. Basic Appl. Sci. 3, 928–942.
Hawkins, H. J., and Lewis, O. A. M. (1993). Combination effect of NaCl salinity, nitrogen form and calcium concentration on the growth, ionic content and gaseous exchange properties of Triticum aestivum L. cv. Gamtoos. New Phytol. 124, 161–170. doi: 10.1111/j.1469-8137.1993.tb03806.x
Hayat, S., Khalique, G., Wani, A. S., Alyemeni, M. N., and Ahmad, A. (2014). Protection of growth in response to 28-homobrassinolide under the stress of cadmium and salinity in wheat. Int. J. Biol. Macromol. 64, 130–136. doi: 10.1016/j.ijbiomac.2013.11.021
He, C., Yang, A., Zhang, W., Gao, Q., and Zhang, J. (2010). Improved salt tolerance of transgenic wheat by introducing betA gene for glycine betaine synthesis. Plant Cell Tissue Organ Cult. 101, 65–78. doi: 10.1007/s11240-009-9665-0
Hossain, M. A., Hoque, T. S., Zaid, A., Wani, S. H., Mostofa, M. G., and Henry, R. (2021). “Targeting the ascorbate-glutathione pathway and the glyoxalase pathway for genetic engineering of abiotic stress-tolerance in rice,” in Molecular Breeding for Rice Abiotic Stress Tolerance and Nutritional Quality, eds M. A. Hossain, L. Hassan, K. M. Iftekharuddaula, A. Kumar A, and R. Henry R (Wiley-Blackwell), 398–427. doi: 10.1002/9781119633174.ch21
Houshmand, S., Arzani, A., and Mirmohammadi-Maibody, S. A. M. (2014). Effects of salinity and drought stress on grain quality of durum wheat. Commun. Soil Sci. Plant Anal. 45, 297–308. doi: 10.1080/00103624.2013.861911
Hu, Y., and Schmidhalter, U. (1998). Spatial distribution sand net deposition rates of mineral elements in the elongating wheat (Triticum aestivum L.) leaf under saline soil conditions. Planta 204, 212–219. doi: 10.1007/s004250050249
Huang, M., Chai, L., Jiang, D., Zhang, M., Zhao, Y., and Huang, Y. (2019). Increasing aridity affects soil archaeal communities by mediating soil niches in semi-arid regions. Sci. Total Environ. 647, 699–707. doi: 10.1016/j.scitotenv.2018.07.305
Huebner, F. R., Nelsen, T. C., Chung, O. K., and Bietz, J. A. (1997). Protein distributions among hard red winter wheat varieties as related to environment and baking quality. Cereal Chem. 74, 123–128. doi: 10.1094/CCHEM.1997.74.2.123
Hussain, S., Shaukat, M., Ashraf, M., Zhu, C., Jin, Q., and Zhang, J. (2019). Salinity stress in arid and semi-arid climates: Effects and management in field crops. Clim. Change Agric 123–145. doi: 10.5772/intechopen.87982
Hütsch, B. W., He, W., and Schubert, S. (2016). Nitrogen nutritional status of young maize plants (Zea mays) is not limited by NaCl stress. J. Plant Nutr. Soil Sci. 179, 775–783. doi: 10.1002/jpln.201500565
Ioannou, M., and Coutsogeorgopoulos, C. (1997). Kinetic studies on the activation of eukaryotic peptidyltransferase by potassium. Arch. Biochem. Biophys. 345, 325–331. doi: 10.1006/abbi.1997.0256
Iqbal, M., and Ashraf, M. (2007). Seed treatment with auxins modulates growth and ion partitioning in salt-stressed wheat plants. J. Integrat. Plant Biol. 49, 1003–1015. doi: 10.1111/j.1672-9072.2007.00488.x
Iqbal, M., Ashraf, M., and Jamil, A. (2006). Seed enhancement with cytokinins: changes in growth and grain yield in salt stressed wheat plants. Plant Growth Regulat. 50, 29–39. doi: 10.1007/s10725-006-9123-5
Iqbal, M., Irshad, S., Nadeem, M., Fatima, T., and Itrat, A. B. (2018). Salinity effects on wheat (Triticum aestivum L.) characteristics: a review article. Int. J Agric. Biol. 12, 1–15. doi: 10.12692/ijb/12.3.131-146
Iqbal, M. A., Junaid, R., Wajid, N., Sabry, H., Yassir, K., and Ayman, S. (2021). Rainfed winter wheat (Triticum aestivum L.) cultivars respond differently to integrated fertilization in Pakistan. Fresenius Environ. Bull. 30, 3115–3121.
Islam, M. S., Akhter, M. M., El Sabagh, A., Liu, L. Y., Nguyen, N. T., Ueda, A., et al. (2011). Comparative studies on growth and physiological responses to saline and alkaline stresses of Foxtail millet (Setaria italica L.) and Proso millet (Panicum miliaceum L.). Austral. J. Crop Sci. 5:1269.
James, R. A., Munns, R., Von Caemmerer, S., Trejo, C., Miller, C., and Condon, T. (2006). Photosynthetic capacity is related to the cellular and subcellular partitioning of Na+, K+ and Cl-in salt-affected barley and durum wheat. Plant Cell Environ. 29, 2185–2197. doi: 10.1111/j.1365-3040.2006.01592.x
Jan, A. U., Hadi, F., Nawaz, M. A., and Rahman, K. (2017). Potassium and zinc increase tolerance to salt stress in wheat (Triticum aestivum L.). Plant Physiol. Biochem. 116, 139–149. doi: 10.1016/j.plaphy.2017.05.008
Jenner, C. F., Ugalde, T. D., and Aspinall, D. (1991). The physiology of starch and protein deposition in the endosperm of wheat. Funct. Plant Biol. 18, 211–226. doi: 10.1071/PP9910211
Kahrizi, S., and Sedghi, M. (2013). Effect of salt stress on grain reserve composition in ten durum wheat cultivars. J. Stress Physiol. Biochem. 3, 113–121.
Kalhoro, N. A., Rajpar, I., Kalhoro, S. A., Ali, A., Raza, S., Ahmed, M., et al. (2016). Effect of salts stress on the growth and yield of wheat (Triticum aestivum L.). Am. J. Plant Sci. 7:2257. doi: 10.4236/ajps.2016.715199
Karuppanapandian, T., Moon, J. C., Kim, C., Manoharan, K., and Kim, W. (2011). Reactive oxygen species in plants: their generation, signal transduction, and scavenging mechanisms. Austral. J. Crop Sci. 5, 709–725.
Katerji, N., Van Hoorn, J. W., Fares, C., Hamdy, A., Mastrorilli, M., and Oweis, T. (2005). Salinity effect on grain quality of two durum wheat varieties differing in salt tolerance. Agric. Water Manage. 75, 85–91. doi: 10.1016/j.agwat.2004.12.005
Kaur, H., Bhardwaj, R. D., and Grewal, S. K. (2017). Mitigation of salinity-induced oxidative damage in wheat (Triticum aestivum L.) seedlings by exogenous application of phenolic acids. Acta Physiol. Plant. 39:221. doi: 10.1007/s11738-017-2521-7
Ke, Q., Ye, J., Wang, B., Ren, J., Yin, L., Deng, X., et al. (2018). Melatonin mitigates salt stress in wheat seedlings by modulating polyamine metabolism. Front. Plant Sci. 9:914. doi: 10.3389/fpls.2018.00914
Khan, A., Ahmad, I., Shah, A., Ahmad, F., Ghani, A., Nawaz, M., et al. (2013). Amelioration of salinity stress in wheat (Triticum aestivum L.) by foliar application of phosphorus. Phyton 82, 281–287. doi: 10.32604/phyton.2013.82.281
Khan, A. L., Waqas, M., Asaf, S., Kamran, M., Shahzad, R., Bilal, S., et al. (2017). Plant growth-promoting endophyte Sphingomonas sp. LK11 alleviates salinity stress in Solanum pimpinellifolium. Environ. Exp. Bot. 133, 58–69. doi: 10.1016/j.envexpbot.2016.09.009
Khan, M. A., Gul, B., and Weber, D. J. (2004). Action of plant growth regulators and salinity on seed germination of Ceratoides lanata. Canad. J. Bot. 82, 37–42. doi: 10.1139/b03-140
Khan, M. I. R., Iqbal, N., Masood, A., and Khan, N. A. (2012). Variation in salt tolerance of wheat cultivars: role of glycinebetaine and ethylene. Pedosphere 22, 746–754. doi: 10.1016/S1002-0160(12)60060-5
Khan, N. A., Shamim, M., and Shambhoo, P. (2008). Biochemical changes in wheat plants in response to salinity. Int. J. Plant Sci. 3, 11–15.
Khataar, M., Mohammadi, M. H., and Shabani, F. (2018). Soil salinity and matric potential interaction on water use, water use efficiency and yield response factor of bean and wheat. Sci. Rep. 8:2679. doi: 10.1038/s41598-018-20968-z
Kizilgeci, F., Yildirim, M., Islam, M. S., Ratnasekera, D., Iqbal, M. A., and Sabagh, A. E. (2021). Normalized difference vegetation index and chlorophyll content for precision nitrogen management in durum wheat cultivars under semi-arid conditions. Sustainability 13:3725. doi: 10.3390/su13073725
Kolbert, Z., Feigl, G., Freschi, L., and Poór, P. (2019). Gasotransmitters in action: nitric oxide-ethylene crosstalk during plant growth and abiotic stress responses. Antioxidants 8:167. doi: 10.3390/antiox8060167
Kosová, K., Prášil, I. T., Vítámvás, P., Dobrev, P., Motyka, V., Floková, K., et al. (2012). Complex phytohormone responses during the cold acclimation of two wheat cultivars differing in cold tolerance, winter Samanta and spring Sandra. J. Plant Physiol. 169, 567–576. doi: 10.1016/j.jplph.2011.12.013
Kotuby-Amazher, J., Koenig, K., and Kitchen, B. (2000). Salinity and Plant Tolerance. Available online at: https://extension.usu.edu/files/publications/publication/AG-SO-03.pdf (accessed June 20, 2020).
Kumari, A., and Kaur, R. (2018). Evaluation of benzyl-butyl phthalate induced germination and early growth vulnerability to barley seedlings (Hordeum vulgare L.). Indian J. Ecol. 45, 174–177.
Kumari, A., and Kaur, R. (2020). A review on morpho-physiological traits of plants under phthalates stress and insights into their uptake and translocation. Plant Growth Regul. 91, 327–347. doi: 10.1007/s10725-020-00625-0
Kundu, P., Gill, R., Ahlawat, S., Anjum, N. A., Sharma, K. K., Ansari, A. A., et al. (2018). “Targeting the redox regulatory mechanisms for abiotic stress tolerance in crops” in Biochemical, Physiological and Molecular Avenues for Combating Abiotic Stress Tolerance in Plants, ed S. H. Wani (Elsevier Academic Press), 151–220. doi: 10.1016/B978-0-12-813066-7.00010-3
Läuchli A, A., and Grattan, S. R. (2007). “Plant growth and development under salinity stress,” in Advances in Molecular Breeding Toward Drought and Salt Tolerant Crops, eds M. A. Jenks, P. M. Hasegawa, and S. M. Jain (Dordrecht: Springer), 1–32. doi: 10.1007/978-1-4020-5578-2_1
Li, P., Cai, J., Luo, X., Chang, T., Li, J., Zhao, Y., et al. (2019). Transformation of wheat Triticum aestivum with the HvBADH1 transgene from hulless barley improves salinity-stress tolerance. Acta. Physiol. Plant 41:155. doi: 10.1007/s11738-019-2940-8
Liu, X., Chen, D., Yang, T., Huang, F., Fu, S., and Li, L. (2020). Changes in soil labile and recalcitrant carbon pools after land-use change in a semi-arid agro-pastoral ecotone in Central Asia. Ecol. Indic. 110:105925. doi: 10.1016/j.ecolind.2019.105925
Llanes, A., Andrade, A., Alemano, S., and Luna, V. (2016). Alterations of endogenous hormonal levels in plants under drought and salinity. Am. J. Plant Sci. 7:1357. doi: 10.4236/ajps.2016.79129
Maas, E. V. (1990). “Crop salt tolerance,” in Agricultural Salinity Assessment and Management. ASCE Publications, ed K. K. Tanji (Reston, VA), 262–303.
Maas, E. V., and Grieve, C. M. (1990). Spike and leaf development of sal-stressed wheat. Crop Sci. 30, 1309–1313. doi: 10.2135/cropsci1990.0011183X003000060031x
Makhloufi, E., Yousfi, F. E., Marande, W., Mila, I., Hanana, M., Bergès, H., et al. (2014). Isolation and molecular characterization of ERF1, an ethylene response factor gene from durum wheat (Triticum turgidum L. subsp. durum), potentially involved in salt-stress responses. J. Exp. Bot. 5, 6359–6371. doi: 10.1093/jxb/eru352
Mandhania, S., Madan, S., and Sawhney, V. (2006). Antioxidant defense mechanism under salt stress in wheat seedlings. Biol. Plant. 50, 227–231. doi: 10.1007/s10535-006-0011-7
Mao, X., Zhang, H., Tian, S., Chang, X., and Jing, R. (2010). TaSnRK2. 4, an SNF1-type serine/threonine protein kinase of wheat (Triticum aestivum L.), confers enhanced multistress tolerance in Arabidopsis. J. Exp. Bot. 61, 683–696. doi: 10.1093/jxb/erp331
Maqsood, T., Akhtar, J., Farooq, M. R., Haq, M. A., and Saqib, Z. A. (2008). Biochemical attributes of salt tolerant and salt sensitive maize cultivars to salinity and potassium nutrition. Pakistan J. Agric. Sci. 45, 1–5.
Massa, D., Mattson, N. S., and Lieth, H. J. (2009). Effects of saline root environment (NaCl) on nitrate and potassium uptake kinetics for rose plants: a Michaelis–Menten modelling approach. Plant Soil 318, 101–115. doi: 10.1007/s11104-008-9821-z
Mbarki, S., Sytar, O., Zivcak, M., Abdelly, C., Cerda, A., and Brestic, M. (2018). Anthocyanins of coloured wheat genotypes in specific response to salstress. Molecules 23:1518. doi: 10.3390/molecules23071518
Mohsenzadeh, S., and Zohrabi, M. (2018). Auxin and sodium nitroprusside effects on wheat antioxidants in salinity. Russian J. Plant Physiol. 65, 651–657. doi: 10.1134/S1021443718050138
Munns, R., James, R. A., and Läuchli, A. (2006). Approaches to increasing the salt tolerance of wheat and other cereals. J. Exp. Bot. 57, 1025–1043. doi: 10.1093/jxb/erj100
Munns, R., and Tester, M. (2008). Mechanisms of salinity tolerance. Ann. Rev. Plant Biol. 59, 651–681. doi: 10.1146/annurev.arplant.59.032607.092911
Mushtaq, A., Jamil, N., Riaz, M., Hornyak, G. L., Ahmed, N., Ahmed, S. S., et al. (2017). Synthesis of silica nanoparticles and their effect on priming of wheat (Triticum aestivum L.) under salinity stress. Biol. Forum 9, 150–157.
Nadeem, M., Tariq, M. N., Amjad, M., Sajjad, M., Akram, M., Imran, M., et al. (2020). Salinity-induced changes in the nutritional quality of bread wheat (Triticum aestivum L.) genotypes. Agrivita 42, 1–12. doi: 10.17503/agrivita.v42i1.2273
Naidu, C. V. (2001). Improvement of seed germination in red sanders (Pterocarpus santalinus Linn. F.) by plant growth regulators. Indian J. Plant Physiol. 6, 205–207.
Nassar, R., Kamel, H. A., Ghoniem, A. E., Alarcón, J. J., Sekara, A., Ulrichs, C., et al. (2020). Physiological and anatomical mechanisms in wheat to cope with salt stress induced by seawater. Plants 9:237. doi: 10.3390/plants9020237
Navarro, C., Moore, J., Ott, A., Baumert, E., Mohan, A., Gill, K. S., et al. (2015). Evolutionary, comparative and functional analyses of the brassinosteroid receptor gene, BRI1, in wheat and its relation to other plant genomes. PLoS ONE 10:e0127544. doi: 10.1371/journal.pone.0127544
Navarro-Yepes, J., Burns, M., Anandhan, A., Khalimonchuk, O., Del Razo, L. M., Quintanilla-Vega, B., et al. (2014). Oxidative stress, redox signaling, and autophagy: cell death versus survival. Antioxidants Redox Signal. 21, 66–85. doi: 10.1089/ars.2014.5837
Netondo, G. W., Onyango, J. C., and Beck, E. (2004). Sorghum and salinity: II. gas exchange and chlorophyll fluorescence of sorghum under salt stress. Crop Sci. 44, 806–811. doi: 10.2135/cropsci2004.8060
Nishida, K., Khan, N. M., and Shiozawa, S. (2009). Effects of salt accumulation on the leaf water potential and transpiration rate of pot-grown wheat with a controlled saline groundwater table. Soil Sci. Plant Nutr. 55, 375–384. doi: 10.1111/j.1747-0765.2009.00368.x
Nishiyama, R., Watanabe, Y., Fujita, Y., Le, D. T., Kojima, M., Werner, T., et al. (2011). Analysis of cytokinin mutants and regulation of cytokinin metabolic genes reveals important regulatory roles of cytokinins in drought, salt and abscisic acid responses, and abscisic acid biosynthesis. Plant Cell 23, 2169–2183. doi: 10.1105/tpc.111.087395
Niu, X., Bressan, R. A., Hasegawa, P. M., and Pardo, J. M. (1995). Ion homeostasis in NaCl stress environments. Plant Physiol. 109:735. doi: 10.1104/pp.109.3.735
Noreen, S., Fatima, K., Athar, H. U. R., Ahmad, S., and Hussain, K. (2017). Enhancement of physio-biochemical parameters of wheat through exogenous application of salicylic acid under drought stress. J. Anim. Plant Sci. 27, 153–163.
Orhan, F. (2016). Alleviation of salt stress by halotolerant and halophilic plant growth-promoting bacteria in wheat (Triticum aestivum). Brazil. J. Microbiol. 47, 621–627. doi: 10.1016/j.bjm.2016.04.001
Oria-Hernández, J., Cabrera, N., Pérez-Montfort, R., and Ramírez-Silva, L. (2005). Pyruvate kinase revisited the activating effect of k+. J. Biol. Chem. 280, 37924–37929. doi: 10.1074/jbc.M508490200
Otu, H., Celiktas, V., Duzenli, S., Hossain, A., and El Sabagh, A. (2018). Germination and early seedling growth of five durum wheat cultivars (Triticum durum Desf.) is affected by different levels of salinity. Fresenius Environ. Bull. 27, 7746–7757.
Parida, A. K., Mittra, B., Das, A. B., Das, T. K., and Mohanty, P. (2005). High salinity reduces the content of a highly abundant 23-kDa protein of the mangrove Bruguiera parviflora. Planta 221, 135–140. doi: 10.1007/s00425-004-1415-2
Park, S. H., Wilson, J. D., and Seabourn, B. W. (2009). Starch granule size distribution of hard red winter and hard red spring wheat: its effects on mixing and breadmaking quality. J. Cereal Sci. 49, 98–105. doi: 10.1016/j.jcs.2008.07.011
Plaza-Wüthrich, S., Blösch, R., Rindisbacher, A., Cannarozzi, G., and Tadele, Z. (2016). Gibberellin deficiency confers both lodging and drought tolerance in small cereals. Front. Plant Sci. 7:643. doi: 10.3389/fpls.2016.00643
Poustini, K., and Siosemardeh, A. (2004). Ion distribution in wheat cultivars in response to salinity stress. Field Crops Res. 85, 125–133. doi: 10.1016/S0378-4290(03)00157-6
Qamar, H., Ilyas, M., Jan, S. A., Mustafa, H. S. B., Arshad, A., Yar, M. S., et al. (2020). Recent trends in molecular breeding and biotechnology for the genetic improvement of Brassica species against drought stress. Fresenius Environ. Bull. 29, 19–25.
Rahman, M. A., Chikushi, J., Yoshida, S., Yahata, H., and Yasunaga, E. (2005). Effect of high air temperature on grain growth and yields of wheat genotypes differing in heat tolerance. J. Agric. Meteorol. 60, 605–608. doi: 10.2480/agrmet.605
Rakhmankulova, Z. F., Shuyskaya, E. V., Shcherbakov, A. V., Fedyaev, V. V., Biktimerova, G. Y., Khafisova, R. R., et al. (2015). Content of proline and flavonoids in the shoots of halophytes inhabiting the South Urals. Russian J. Plant Physiol. 62, 71–79. doi: 10.1134/S1021443715010112
Rao, A. C. S., Smith, J. L., Jandhyala, V. K., Papendick, R. I., and Parr, J. F. (1993). Cultivar and climatic effects on the protein content of soft white winter wheat. Agron. J. 85, 1023–1028. doi: 10.2134/agronj1993.00021962008500050013x
Reddy, B. V. S., Reddy, P. S., Bidinger, F., and Blümmel, M. (2003). Crop management factors influencing yield and quality of crop residues. Field Crops Res. 84, 57–77. doi: 10.1016/S0378-4290(03)00141-2
Richardson, A. E., and Simpson, R. J. (2011). Soil microorganisms mediating phosphorus availability update on microbial phosphorus. Plant Physiol. 156, 989–996. doi: 10.1104/pp.111.175448
Riyazuddin, R., Verma, R., Singh, K., Nisha, N., Keisham, M., Bhati, K. K., et al. (2020). Ethylene: a master regulator of salinity stress tolerance in plants. Biomolecules 10:959. doi: 10.3390/biom10060959
Rong, W., Qi, L., Wang, A., Ye, X., Du, L., Liang, H., et al. (2014). The ERF transcription factor Ta ERF 3 promotes tolerance to salt and drought stresses in wheat. Plant Biotechnol. J. 12, 468–479. doi: 10.1111/pbi.12153
Royo, A., and Abió, D. (2003). Salt tolerance in durum wheat cultivars. Spanish J. Agric. Res. 1, 27–36. doi: 10.5424/sjar/2003013-32
Ryu, H., and Cho, Y. G. (2015). Plant hormones in salt stress tolerance. J. Plant Biol. 58, 147–155. doi: 10.1007/s12374-015-0103-z
Saboora, A., Kiarostami, K., Behroozbayati, F., and Hajihashemi, S. (2006). Salinity (NaCl) tolerance of wheat genotypes at germination and early seedling growth. Pakistan J. Biol. Sci. 9, 2009–2021. doi: 10.3923/pjbs.2006.2009.2021
Sairam, R. K., Rao, K. V., and Srivastava, G. C. (2002). Differential response of wheat genotypes to long term salinity stress in relation to oxidative stress, antioxidant activity and osmolyte concentration. Plant Sci. 163, 1037–1046. doi: 10.1016/S0168-9452(02)00278-9
Samad, R., and Karmoker, J. L. (2012). Effects of gibberellic acid and Kn on seed germination and accumulation of Na+ and K+ in the seedlings of triticale-I under salinity stress. Bangladesh J. Bot. 41, 123–129. doi: 10.3329/bjb.v41i2.13435
Sawahel, W. A., and Hassan, A. H. (2002). Generation of transgenic wheat plants producing high levels of the osmoprotectant proline. Biotechnol. Lett. 24, 721–725. doi: 10.1023/A:1015294319114
Schofield, J. D. (1994). “Wheat proteins: structure and functionality in milling and breadmaking,” in Wheat, eds W. Bushuk and V. F. Rasper (Boston, MA: Springer), 73–106. doi: 10.1007/978-1-4615-2672-8_7
Schwechheimer, C. (2012). Gibberellin signaling in plants–the extended version. Front. Plant Sci. 2:107. doi: 10.3389/fpls.2011.00107
Sedaghat, M., Sarvestani, Z. T., Emam, Y., and Bidgoli, A. M. (2017). Do phytohormones influence the grain quality and yield of winter wheat under drought conditions? J. Adv. Agric. Technol. 4, 151–158. doi: 10.18178/joaat.4.2.151-158
Shaddad, M. A. K., HM, A. E. S., and Mostafa, D. (2013). Role of gibberellic acid (GA3) in improving salt stress tolerance of two wheat cultivars. Int. J. Plant Physiol. Biochem. 5, 50–57. doi: 10.5897/IJPPB2011.055
Shafi, M., Zhang, G., Bakht, J., Khan, M. A., Islam, U. E., Khan, M. D., et al. (2010). Effect of cadmium and salinity stresses on root morphology of wheat. Pakistan J. Bot. 42, 2747–2754.
Shahid, S. A., Zaman, M., and Heng, L. (2018). “Introduction to soil salinity, sodicity and diagnostics techniques,” in Guideline for Salinity Assessment, Mitigation and Adaptation Using Nuclear and Related Techniques, eds M. Zaman, S. A. Shahid, and L. Heng (Cham: Springer), 1–42. doi: 10.1007/978-3-319-96190-3_1
Shakirova, F. M., Avalbaev, A. M., Bezrukova, M. V., Fatkhutdinova, R. A., Maslennikova, D. R., and Yuldashev, R. A. (2012). “Hormonal intermediates in the protective action of exogenous phytohormones in wheat plants under salinity,” in Phytohormones and Abiotic Stress Tolerance in Plants, eds N. Khan, R. Nazar, N. Iqbal, and N. Anjum (Berlin; Heidelberg: Springer), 185–228. doi: 10.1007/978-3-642-25829-9_9
Shakirova, F. M., Sakhabutdinova, A. R., Bezrukova, M. V., Fatkhutdinova, R. A., and Fatkhutdinova, D. R. (2003). Changes in the hormonal status of wheat seedlings induced by salicylic acid and salinity. Plant Sci. 164, 317–322. doi: 10.1016/S0168-9452(02)00415-6
Sharbatkhari, M., Shobbar, Z. S., Galeshi, S., and Nakhoda, B. (2016). Wheat stem reserves and salinity tolerance: molecular dissection of fructan biosynthesis and remobilization to grains. Planta 244, 191–202. doi: 10.1007/s00425-016-2497-3
Sharma, P., Jha, A. B., Dubey, R. S., and Pessarakli, M. (2012). Reactive oxygen species, oxidative damage, and antioxidative defense mechanism in plants under stressful conditions. J. Bot. 2012. doi: 10.1155/2012/217037
Shen, Y., Guo, W., Zhou, Y., Zhu, X., Feng, C., and Peng, Y. (2007). Effects of salinity stress on the dynamic changes in the accumulation of grain protein and its components in wheat. J. Triticeae Crops 26, 100–103.
Siddiqui, M. H., Iqbal, M. A., Wajid, N., Imtiaz, H., and Khaliq, A. (2019). Bio-economic viability of rainfed wheat (Triticum aestivum L.) cultivars under integrated fertilization regimes in Pakistan. Custos e Agronegocio 15, 81–96.
Singh, R. P., Jha, P., and Jha, P. N. (2015). The plant-growth-promoting bacterium Klebsiella sp. SBP-8 confers induced systemic tolerance in wheat (Triticum aestivum) under salt stress. J. Plant Physiol. 184, 57–67. doi: 10.1016/j.jplph.2015.07.002
Sorour, S. G., Aiad, M. A., Ahmed, A. A., Henash, M. I. A., Metwaly, E. M., Alharby, H., et al. (2019). Yield of wheat is increased through improving the chemical properties, nutrient availability and water productivity of salt affected soils in the north delta of Egypt. Appl. Ecol. Environ. Res. 17, 8291–8306. doi: 10.15666/aeer/1704_82918306
Speer, M., and Kaiser, W. M. (1991). Ion relations of symplastic and apoplastic space in leaves from Spinacia oleracea L. and Pisum sativum L. under salinity. Plant Physiol. 97, 990–997. doi: 10.1104/pp.97.3.990
Sreenivasulu, N., Sopory, S. K., and Kishor, P. K. (2007). Deciphering the regulatory mechanisms of abiotic stress tolerance in plants by genomic approaches. Gene 388, 1–13. doi: 10.1016/j.gene.2006.10.009
Suzuki, K., Yamaji, N., Costa, A., Okuma, E., Kobayashi, N. I., Kashiwagi, T., et al. (2016). OsHKT1; 4-mediated Na+ transport in stems contributes to Na+ exclusion from leaf blades of rice at the reproductive growth stage upon salt stress. BMC Plant Biol. 16:22. doi: 10.1186/s12870-016-0709-4
Sytar, O., Mbarki, S., Zivcak, M., and Brestic, M. (2018). “The involvement of different secon dary metabolites in salinity tolerance of crops,” in Salinity Responses and Tolerance in Plants, eds V. Kumar, S. Wani, P. Suprasanna, and L. S. Tran. Vol. 2. (Cham: Springer), 21–48. doi: 10.1007/978-3-319-90318-7_2
Tabatabaei, S. A. (2013). The effect of salicylic acid and gibberellin on enzyme activity and germination characteristics of wheat seeds under salinity stress conditions. Int. J. Agric. Crop Sci. 6, 236–240.
Tammam, A. A., Alhamd, M. F. A., and Hemeda, M. M. (2008). Study of salt tolerance in wheat (Triticum aestium L.) cultivar Banysoif 1. Austral. J. Crop Sci. 1, 115–125.
Tareq, M. Z., Hossain, M. A., Mojakkir, M. A., Ahmed, R., and Fakir, M. S. A. (2011). Effect of salinity on reproductive growth of wheat. Bangladesh J. Seed Sci. Technol. 15, 111–116.
Thomas, G. W., and Swoboda, A. R. (1970). Anion exclusion effects on chloride movement in soils. Soil Sci. 110, 163–166. doi: 10.1097/00010694-197009000-00003
Tian, S., Mao, X., Zhang, H., Chen, S., Zhai, C., Yang, S., et al. (2013). Cloning and characterization of TaSnRK2. 3, a novel SnRK2 gene in common wheat. J. Exp. Bot. 64, 2063–2080. doi: 10.1093/jxb/ert072
Tian, X., He, M., Wang, Z., Zhang, J., Song, Y., He, Z., et al. (2015). Application of nitric oxide and calcium nitrate enhances tolerance of wheat seedlings to salt stress. Plant Growth Regulat. 77, 343–356. doi: 10.1007/s10725-015-0069-3
Troccoli, A., Borrelli, G. M., De Vita, P., Fares, C., and Di Fonzo, N. (2000). Mini review: durum wheat quality: a multidisciplinary concept. J. Cereal Sci. 32, 99–113. doi: 10.1006/jcrs.2000.0322
Turan, M. A., Elkarim, A. H. A., Taban, N., and Taban, S. (2009). Effect of salt stress on growth, stomatal resistance, proline and chlorophyll concentrations on maize plant. Afr. J. Agric. Res. 4, 893–897. doi: 10.5897/AJAR.9000223
Umezawa, T., Sugiyama, N., Mizoguchi, M., Hayashi, S., Myouga, F., Yamaguchi-Shinozaki, K., et al. (2009). Type 2C protein phosphatases directly regulate abscisic acid-activated protein kinases in Arabidopsis. Proc. Natl. Acad. Sci. U.S.A. 106, 17588–17593. doi: 10.1073/pnas.0907095106
Van Loon, L. C. (2007). “Plant responses to plant growth-promoting rhizobacteria,” in New Perspectives and Approaches in Plant Growth-Promoting Rhizobacteria Research, eds P. A. H. M. Bakker, J. M. Raaijmakers, G. Bloemberg, M. Höfte, P. Lemanceau, and B. M. Cooke (Dordrecht: Springer), 243–54. doi: 10.1007/978-1-4020-6776-1_2
Wajid, M., Khan, M. A., Shirazi, M. U., and Summiya, F. (2019). Seed priming modulates germination potential, osmoprotectants accumulation and ionic uptake in wheat seedlings under salt stress. Int. J. Agric. Biol. 22, 594–600.
Wakeel, A., Farooq, M., Qadir, M., and Schubert, S. (2011). Potassium substitution by sodium in plants. Critic. Rev. Plant Sci. 30, 401–413. doi: 10.1080/07352689.2011.587728
Wang, Y., Mopper, S., and Hasenstein, K. H. (2001). Effects of salinity on endogenous ABA, IAA, JA, and SA in Iris hexagona. J. Chem. Ecol. 27, 327–342. doi: 10.1023/A:1005632506230
Wilkinson, S., Kudoyarova, G. R., Veselov, D. S., Arkhipova, T. N., and Davies, W. J. (2012). Plant hormone interactions: innovative targets for crop breeding and management. J. Exp. Bot. 63, 3499–3509. 10.1093/jxb/ers148 doi: 10.1093/jxb/ers148
Xue, Z. Y., Zhi, D. Y., Xue, G. P., Zhang, H., Zhao, Y. X., and Xia, G. M. (2004). Enhanced salt tolerance of transgenic wheat (Tritivum aestivum L.) expressing a vacuolar Na+/H+ antiporter gene with improved grain yields in saline soils in the field and a reduced level of leaf Na+. Plant Sci. 167, 849–859. doi: 10.1016/j.plantsci.2004.05.034
Yang, T., Yao, S., Hao, L., Zhao, Y., Lu, W., and Xiao, K. (2016). Wheat bHLH-type transcription factor gene TabHLH1 is crucial in mediating osmotic stresses tolerance through modulating largely the ABA-associated pathway. Plant Cell Rep. 35, 2309–2323. doi: 10.1007/s00299-016-2036-5
Yousfi, S., Serret, M. D., and Araus, J. L. (2013). Comparative response of δ13C, δ18O and δ15N in durum wheat exposed to salinity at the vegetative and reproductive stages. Plant Cell Environ. 36, 1214–1227. doi: 10.1111/pce.12055
Yuldashev, R., Avalbaev, A., Bezrukova, M., Vysotskaya, L., Khripach, V., and Shakirova, F. (2012). Cytokinin oxidase is involved in the regulation of cytokinin content by 24-epibrassinolide in wheat seedlings. Plant Physiol. Biochem. 55, 1–6. doi: 10.1016/j.plaphy.2012.03.004
Yurekli, F., Porgali, Z. B., and Turkan, I. (2004). Variations in abscisic acid, indole-3-acetic acid, gibberellic acid and zeatin concentrations in two bean species subjected to salt stress. Acta Biol. Cracoviensia Series Botanica, 46, 201–212.
Zaman, B., Niazi, B. H., Athar, M., and Ahmad, M. (2005). Response of wheat plants to sodium and calcium ion interaction under saline environment. Int. J. Environ. Sci. Technol. 2, 7–12. doi: 10.1007/BF03325852
Zhang, L., Xie, J., Wang, L., Si, L., Zheng, S., Yang, Y., et al. (2020). Wheat TabZIP8, 9, 13 participate in ABA biosynthesis in NaCl-stressed roots regulated by TaCDPK9-1. Plant Physiol. Biochem. 151, 650–658. doi: 10.1016/j.plaphy.2020.03.039
Zhang, S., Gan, Y., and Xu, B. (2016). Application of plant-growth-promoting fungi Trichoderma longibrachiatum T6 enhances tolerance of wheat to salt stress through improvement of antioxidative defense system and gene expression. Front. Plant Sci. 7:1405. doi: 10.3389/fpls.2016.01405
Zhang, X., Shi, Z., Tian, Y., Zhou, Q., Cai, J., Dai, T., et al. (2016). Salt stress increases content and size of glutenin macropolymers in wheat grain. Food Chem. 197, 516–521. doi: 10.1016/j.foodchem.2015.11.008
Zhao, Y., Ai, X., Wang, M., Xiao, L., and Xia, G. (2016). A putative pyruvate transporter TaBASS2 positively regulates salinity tolerance in wheat via modulation of ABI4 expression. BMC Plant Biol. 16:109. doi: 10.1186/s12870-016-0795-3
Zheng, Y., Wang, Z., Sun, X., Jia, A., Jiang, G., and Li, Z. (2008). Higher salinity tolerance cultivars of winter wheat relieved senescence at reproductive stage. Environ. Exp. Bot. 62, 129–138. doi: 10.1016/j.envexpbot.2007.07.011
Zheng, Y., Xu, X., Li, Z., Yang, X., Zhang, C., Li, F., et al. (2009). Differential responses of grain yield and quality to salinity between contrasting winter wheat cultivars. Seed Sci. Biotechnol. 3, 40–43.
Zhou, Y., Li, Y., Qi, X., Liu, R., Dong, J., Jing, W., et al. (2020). Overexpression of V-type H+ pyrophosphatase gene EdVP1 from Elymus dahuricus increases yield and potassium uptake of transgenic wheat under low potassium conditions. Sci. Rep. 10:5020. doi: 10.1038/s41598-020-62052-5
Keywords: salinity stress, antioxidant defense, wheat, stress tolerance, physiological and biochemical mechanisms
Citation: EL Sabagh A, Islam MS, Skalicky M, Ali Raza M, Singh K, Anwar Hossain M, Hossain A, Mahboob W, Iqbal MA, Ratnasekera D, Singhal RK, Ahmed S, Kumari A, Wasaya A, Sytar O, Brestic M, ÇIG F, Erman M, Habib Ur Rahman M, Ullah N and Arshad A (2021) Salinity Stress in Wheat (Triticum aestivum L.) in the Changing Climate: Adaptation and Management Strategies. Front. Agron. 3:661932. doi: 10.3389/fagro.2021.661932
Received: 31 January 2021; Accepted: 27 May 2021;
Published: 08 July 2021.
Edited by:
Hamid Khazaei, University of Saskatchewan, CanadaReviewed by:
Ahmad Arzani, Isfahan University of Technology, IranFarid Shekari, University of Zanjan, Iran
Copyright © 2021 EL Sabagh, Islam, Skalicky, Ali Raza, Singh, Anwar Hossain, Hossain, Mahboob, Iqbal, Ratnasekera, Singhal, Ahmed, Kumari, Wasaya, Sytar, Brestic, ÇIG, Erman, Habib Ur Rahman, Ullah and Arshad. This is an open-access article distributed under the terms of the Creative Commons Attribution License (CC BY). The use, distribution or reproduction in other forums is permitted, provided the original author(s) and the copyright owner(s) are credited and that the original publication in this journal is cited, in accordance with accepted academic practice. No use, distribution or reproduction is permitted which does not comply with these terms.
*Correspondence: Ayman EL Sabagh, YXltYW4uZWxzYWJhZ2gmI3gwMDA0MDthZ3Iua2ZzLmVkdS5lZw==