- 1Instituto de Agrobiotecnología y Biología Molecular, CONICET - INTA, Buenos Aires, Argentina
- 2Instituto de Genética “Edwald Alfredo Favret”, INTA, Buenos Aires, Argentina
- 3Laboratorio de Agrobiotecnología, IPADS, CONICET - INTA, Balcarce, Argentina
- 4Facultad de Ciencias Agrarias, Universidad Nacional de Mar del Plata, Balcarce, Argentina
- 5Department of Plant Sciences, University of California, Davis, Davis, CA, United States
Because its ability to acquire large amounts of nitrogen by symbiosis, tetraploid alfalfa is the main source of vegetable proteins in meat and milk production systems in temperate regions. Alfalfa cultivation also adds fixed nitrogen to the soil, improving the production of non-legumes in crop rotation and reducing the use of nitrogen fertilizers derived from fossil fuel. Despite its economic and ecological relevance, alfalfa genetics remains poorly understood, limiting the development of public elite germplasm. In this brief article, we reported the high-efficiency of alfalfa mutagenesis by using the public clone C23 and the CRISPR/Cas9 system. Around half of the GUS overexpressing plants (35S-GUS under C23 genomic background) transformed with an editing plasmid containing two sgRNAs against the GUS gene and the Cas9 nuclease exhibited absence of GUS activity. Nucleotide analysis showed that the inactivation of GUS in CRISPR/Cas9-editing events were produced via different modifications in the GUS gene, including frameshift and non-sense mutations. Using the CRISPR/Cas9 system and two sgRNAs, we have also edited the alfalfa gene NOD26, generating plants with different doses of alleles at this locus, including complete gene knockout at high efficiency (11%). Finally, we discuss the potential applications of genome-editing technologies to polyploid research and to alfalfa improvement public programs.
Introduction
The production of mutant alleles has been essential for plant functional genetics. Efficient generation of stable plant mutant lines has been achieved using different methods, including ethyl methanesulfonate, ionizing radiation, RNA interference, artificial microRNAs, and the insertion of T-DNA and retrotransposons. During the last years, there has been an explosion in the production of knockout plant lines through the clustered regularly interspaced short palindromic repeats (CRISPR)-Cas9 genome-editing system of Streptococcus pyogenes, which requires the canonical PAM motif NGG (N, any nucleotide; G, guanine) in dsDNA-bound states for DNA targeting. This system contains two components: one encoding the Cas9 endonuclease and the other containing a synthetic single guide RNA (sgRNA), with the latter typically under the control of the U6 small nuclear RNA gene promoter. Cas9 is normally efficient at inducing double-strand DNA breaks in both model plants and important crops. When repaired by the error-prone non-homologous end-joining pathway, these DNA breaks eventually result in the emergence of short deletions and/or insertions (indels) that give rise to stop codons or non-sense and frameshift mutations (Bortesi and Fischer, 2015).
Alfalfa (Medicago sativa L.), commonly known as the “Queen of Forages,” is the main source of plant protein for the meat and milk global production. Because of its ability to establish effective symbiosis with nitrogen-fixing bacteria, the production of animal protein based on alfalfa feeds does not require the use of nitrogen fertilizers derived from fossil fuel, reducing our dependence on non-renewable energy sources and mitigating the emission of greenhouse gases. Although during the last years the production of stable mutant libraries has been extended from model plants (e.g., Arabidopsis thaliana, Medicago truncatula, and Brachypodium distachyon) to main crops (e.g., potato, soybean, and sorghum), public projects have not produced mutant libraries of alfalfa, limiting functional genomics studies from this important legume crop. Consequently, there is a continuing and increasing interest in the development of alternative methods for the editing of alfalfa genome, which partially depends on the regenerative genotype used during alfalfa transformation (Gao et al., 2018; Chen et al., 2020; Wolabu et al., 2020). Previously, we developed a highly effective procedure for the transformation of the public alfalfa clone C23 via Agrobacterium tumefaciens (Garcia et al., 2014; Jozefkowicz et al., 2016, 2018; Stritzler et al., 2018; Pascuan et al., 2020). In this brief research report, we used this framework for the production of CRISPR/Cas9 gene-edited alfalfa from clone C23.
Materials and Methods
For the construction of the pGUS-MUT plasmid, two gRNAs were produced using the gh51 plasmid (Narimatsu et al., 2018) as template and specific primers designed against the GUS gene (Supplementary Figure 1). The binary vector pGUS-MUT was constructed in two steps by using the Golden Gate cloning system (Weber et al., 2011), and BsaI and BbSI restriction enzymes supplemented with T4 ligase for Level 1 and Level 2 vectors, respectively. First, the Level 1 vectors pICH47751 and pICH47761 (Weber et al., 2011) were used in combination with pICSL01009::AtU6p (Nekrasov et al., 2013), to obtain sgRNAGUSMse and sgRNAGUS under the control of the A. thaliana U6 promoter. Next, Level 1 constructs (pICH47751::AtU6p::sgRNAGUSMse and pICH47761::AtU6p::sgRNAGUS), pICH47732::NOSp-NPTII-OCST (Castel et al., 2019), pICH47742::35S-Cas9-NOST (Belhaj et al., 2013) and the linker pICH41780 (Weber et al., 2011) were assembled into the Level 2 vector pAGM4723 (Weber et al., 2011). For molecular and histochemical analyses of transgenic and edited plants, genomic DNA of alfalfa plants was isolated from leaf tissue by using the DNeasy plant mini kit (Cat.#69104, Qiagen). The presence and integrity of the GUS reporter in alfalfa plants were analyzed by PCR studies against the GUS reporter and Sanger sequencing. In PCR analysis, a 1,098-bp fragment derived from the GUS reporter was amplified by using primers GUS-UP and GUS-Low (Supplementary Figure 1), and the following amplification conditions: 1 cycle at 94°C for 5 min, 34 cycles of 94°C for 1 min, 60°C for 1 min 30 s and 72°C for 1 min, and a final extension of 72°C for 8 min. We verified that the GUS gene was edited in a subset of 25 plants via Sanger sequencing. Histochemical detection of GUS activity in alfalfa plants was analyzed as previously (Kelemen et al., 2002).
For the insertion and mutation of the GUS gene in the alfalfa genome, two transgenic alfalfa events, called here 3-1 and 5-1, which show high and constitutive expression of the GUS reporter, were generated by transforming the regenerative alfalfa clone C2-3 with the binary vector pBI121 (Accession Number: AF485783.1) as described previously (Garcia et al., 2014; Jozefkowicz et al., 2018). To determine the number of T-DNA insertions in 3-1 and 5-1, these 35S-GUS-transformed alfalfa events were individually crossed manually with CW1010 wild-type alfalfa by using transgenic parental plants as pollen donors. The progenies of 3-1 and 5-1 were analyzed by histochemical detection of GUS activity, showing in both cases a Mendelian inheritance pattern of a single gene. For the editing of the GUS reporter in 3-1 and 5-1 via CRISPR/Cas9, the binary vector pGUS-MUT was introduced into Agrobacterium tumefaciens LBA4404 by electroporation, as described by Shen and Forde (1989). Petioles of 3-1 and 5-1 were transformed with pGUS-MUT via A. tumefaciens and cultured in vitro as described previously (Garcia et al., 2014), with some modifications to minimize escapes from the kanamycin selection and maximize the efficiency of genome editing in alfalfa. Petiole tissues were decontaminated by dipping in 70% ethyl alcohol for 1 min and 2% sodium hypochlorite for 20 min. The petioles were washed five times in sterile bidistilled water. Explants earlier damaged with a scalpel were inoculated with an A. tumefaciens culture for 3 min (OD600nm= 0.6), and then dried in Whatman filter paper and transferred to SHK medium (Garcia et al., 2014) supplemented with 100 μM acetosyringone and 100 mg/l cefotaxime, and incubated for 3 days at 25°C in the dark. The alfalfa explants were then washed five times with 400 mg/l cefotaxime and transferred to SHK medium containing 400 mg/l cefotaxime and 25 mg/l kanamycin, and then, routinely transferred to fresh medium every 1 week, at 25°C and 16 h light (150 μmoles/m2s). Somatic embryos were transferred to MS rooting medium, composed of MS medium (Jozefkowicz et al., 2016) diluted 1:2 with water. Around 6 months after callus formation, regenerated plantlets were transferred to growth chambers programmed for 16 h light at 23°C and 8 h dark at 20°C. CRISPR/Cas9-mediated gene-editing events were propagated by cuttings to increase the plant material available for histochemical and molecular studies. Kanamycin-tolerant plants derived from different calluses were considered independent gene-editing events.
The pNOD26-MUT plasmid was produced using the modular system and the Webtools for the Voytas Lab Plant Genome Engineering Toolkit previously described by the Voytas group (Cermak et al., 2017). To corroborate the complete conservation of the nucleotide sequence to edit in the genome of the C23 clone, the two first exons and the first intron of the NOD26 gene from Medicago sativa (MSAD_247675) was cloned into a pGEM-T easy vector (Biodynamics, Argentina) and sequenced. Two sgRNAs were designed for each target, specifically exon 1 and exon 2 of MsNOD26 (Supplementary Figure 1). PCR products containing BsaI restriction sites were digested and ligated through a Golden Gate reaction, resulting in one polycistronic element containing the CmYLCV promoter, sgRNAs, Csy4 sites and the CaMV terminator. The subsequent fragment was digested with SapI enzyme and cloned into pMOD_B2103. The pMODB vector harboring the polycistronic construct and three other modular vectors (pMOD_A0501, pMOD_C3003, and pTRANS_220d) were assembled together with AarlI digestion and ligation, resulting in the binary vector pNOD26-MUT. The transformation of the C23 clone with the pNOD26-MUT vector via Agrobacterium and the selection of transgenic plants using kanamycin were performed as described above. The presence of the Cas9 gene in regenerated plants was confirmed by PCR studies. In this analysis, a 353-bp fragment derived from the Cas9 gene was amplified by using specific primers (Supplementary Figure 1), and the following amplification conditions: 1 cycle at 94°C for 5 min, 35 cycles of 94°C for 30 s, 60°C for 30 s and 72°C for 30 s, and a final extension of 72°C for 5 min. For the analysis of NOD26-edited alleles in transgenic plants, genomic DNA was extracted from young leaves as described above. DNA fragments derived from the target region was amplified by using specific primers (Supplementary Figure 1) in a final volume of 20 L in the presence of 75 ng DNA, 1 U of Taq DNA polymerase (Platinum Taq DNA Polymerase, Invitrogen), 2.5 mM MgCl2, 0.2 mM of each dNTP, 2 L of 10 X PCR Buffer (Invitrogen) and 0.5 mM of each primer. The forward primer was labeled with 5'6-FAM (Thermo Fisher Scientific, Argentina). The PCR protocol was: 1 cycle at 94°C for 2 min, 35 cycles at 94°C for 30 s, 55 °C for 30 s, and 72°C for 40 s, and a final extension of 10 min at 72°C. The amplified fragments were detected by a Genetic Analyzer GA3500XL (Applied Biosystems), as previously (Cuyeu et al., 2013, 2014). Each PCR was performed six times.
Results and Discussion
To evaluate the rate of induced mutation frequency via CRISPR/Cas9 in alfalfa by using the C23 genotype, we produced two transgenic alfalfa events (3-1 and 5-1) with the pBI121 binary vector containing the GUS gene (Figure 1A) and expressing high GUS activities in both somatic embryos and leaves (Figure 1B), which were re-transformed with the pGUS-MUT plasmid containing two sgRNAs against the GUS gene under the control of the U6 promoter for the editing of this reporter via CRISPR/Cas9 (Figure 1A). As expected, no GUS inactivation was observed in 97 regenerated plants derived from 3-1 to 5-1, confirming the absence of spontaneous GUS inactivation under our specific regeneration condition (data not shown). Contrarily, GUS inactivation was observed in plants derived from 3-1 to 5-1 transformed with the pGUS-MUT plasmid (Figure 1B), where the frequency of GUS inactivation was 48% (37 GUS-negative and 40 GUS-positive plants) and 62% (58 GUS-negative and 35 GUS-positive plants), respectively, showing the high efficiency (an average of 55%) of CRISPR/Cas9 genome editing system in C23 genomic background. As expected, nucleotide analysis showed that GUS inactivation in CRISPR/Cas9-editing events (CRISPR/Cas9) were produced via different alterations in the GUS gene, including frameshift and non-sense mutations (Figure 1C).
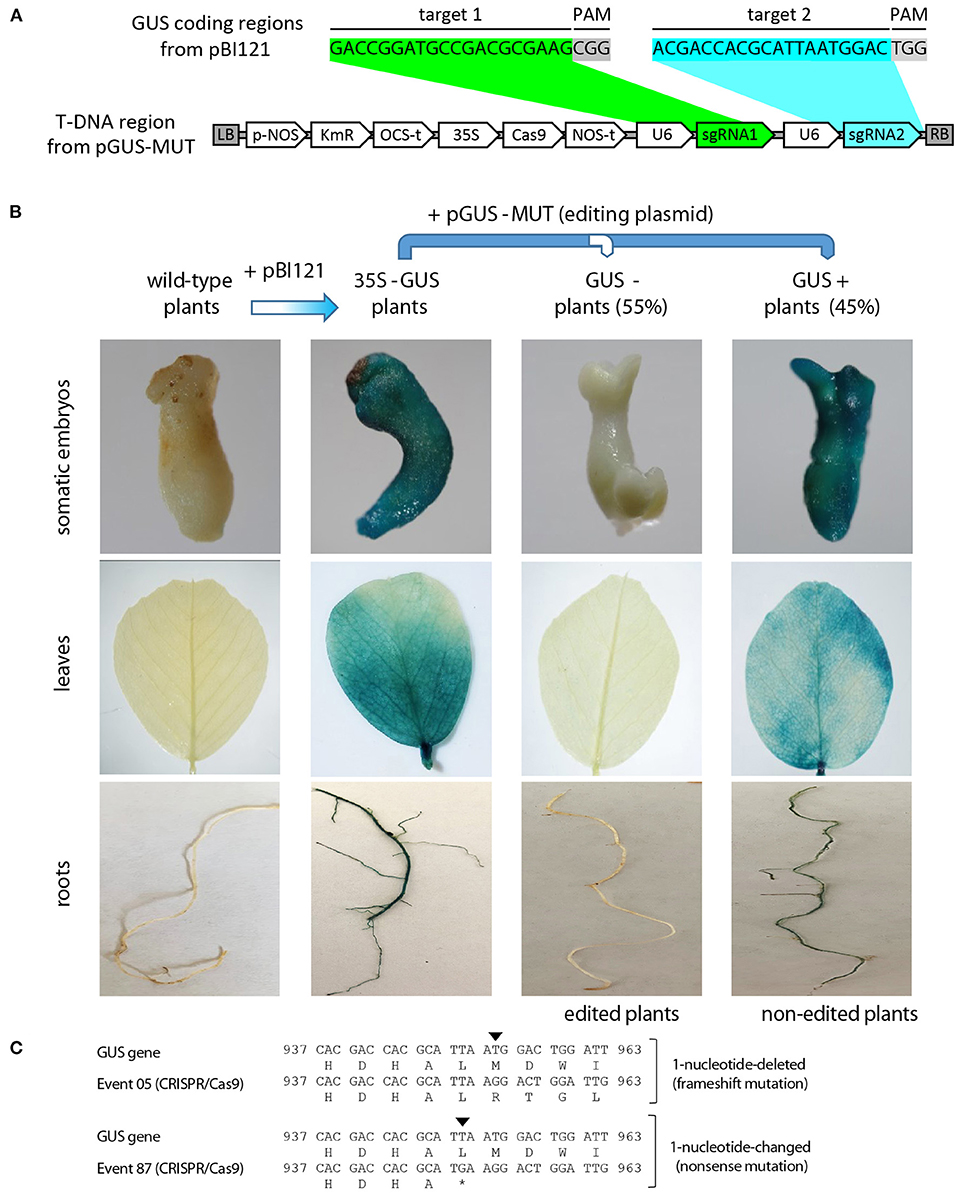
Figure 1. High-efficiency alfalfa mutagenesis by using regenerative alfalfa clone C23 and the CRISPR/Cas9 system. (A) Above: Schematic representation of a fragment of the GUS coding sequence from the binary vector pBI121 used to produce transgenic alfalfa with high GUS activities, specifically showing the sequence targeted by the sgRNA (target 1 and target 2) and the sequence recognized by the Cas9 nuclease (PAM sequences). Below: Schematic representation of the T-DNA region from the binary vector pGUS-MUT used for the CRISPR/Cas9-mediated knockout of GUS reporter. In the last vector, the nopaline synthase (pNOS), the Arabidopsis thaliana U6 (U6), and the CaMV 35S promoters were used to drive the constitutive expression of the NPTII gene for kanamycin selection in planta (KmR), a plant codon-optimized Cas9 gene for genome editing in dicots (Cas9) and two sgRNA (sgRNA1 and sgRNA2) that targeted in two regions (target 1 and target 2) of the GUS gene. The octopine synthase (OCS-t) and nopaline synthase (NOS-t) terminators within the T-DNA region are shown. (B) Representative images of GUS activity in somatic embryos and leaves of alfalfa clone C23 without synthetic elements (wild-type plants), alfalfa clone C23 containing the GUS reporter from pBI121 (35S-GUS plants), and importantly, GUS-positive (GUS+) and GUS-negative (GUS-) plants derived from 35S-GUS plants transformed with the CRISPR/Cas9 system for GUS edition from pGUS-MUT. As described in the text and summarized in this picture, around half of the 35S-GUS plants transformed with the Cas9 nuclease exhibited absence of GUS activity (GUS- plants), showing that this genome editing system (CRISPR/Cas9) is an excellent tool for the mutagenesis of this genomic background (alfalfa clone C23). (C) Sequence analysis showed different mutations within the GUS genes from edited alfalfa events (CRISPR/Cas9) that generate mutant versions of GUS proteins in these plants, such as frameshift and non-sense mutations within target 2 in CRISPR/Cas9-editing events 5 and 87.
Due to the polyploidy nature of cultivated alfalfa (Medicago sativa), it is important to analyze the efficiency of CRISPR/Cas9 to mutagenize the four alleles of an endogenous gene of the C23 genotype. In this context, we produced 35 transgenic events with the pNOD26-MUT plasmid containing CRISPR/Cas9 and two sgRNAs against the NOD26 gene (Frare et al., 2018) under the CmYLCV promoter. Specifically, these sgRNAs target to the first two exons of the MsNOD26 gene (Figure 2A). Due to the high conservation of the alleles of the MsNOD26 gene in the region selected to edit, the 499-bp amplified fragment derived from wild-type alleles can be rapidly detected by flow cytometry (Figure 2B). This amplification showed 31 alleles, with band sizes of 492–499 bp and multiple products per events (ranging from 1 to 4), at an average of 1.7 alleles per locus in the 36 transgenic events (Supplementary Table 1), supporting the high efficiency of the CRISPR/Cas9 genome editing system in the C23 genomic background (Supplementary Table 1). This approach preferentially induces small indels in edited alleles (Supplementary Table 1), and consequently, these directed mutations mimic spontaneous mutations. The occurrence of this particular type of genetic modifications improves the commercial perspectives of the edited alfalfa germplasm in major alfalfa-producing countries (i.e., USA, Canada, Australia and Argentina), where there are particular legislations regarding new plant-breeding technologies (Menz et al., 2020). Interestingly, it is possible to generate different genotypes for this simple locus (MsNOD26 gene) with a relative small set of transgenic events. These genotypes include non-edited (wild-type) plants and homozygous and heterozygous mutants for the MsNOD26 gene (Figures 2B,C). Therefore, the mutagenesis of alfalfa clone C23 via the CRISPR/Cas9 system may help to explore the effect of different doses of alleles at an individual locus, expanding our understanding of its complex genetics. Additionally, the CRISPR/Cas9 system delivers gene knockout at high efficiencies (11%) in the C23 genomic background (Supplementary Table 1), a common rate-limiting step to understand the role of a single gene in this important legume crop.
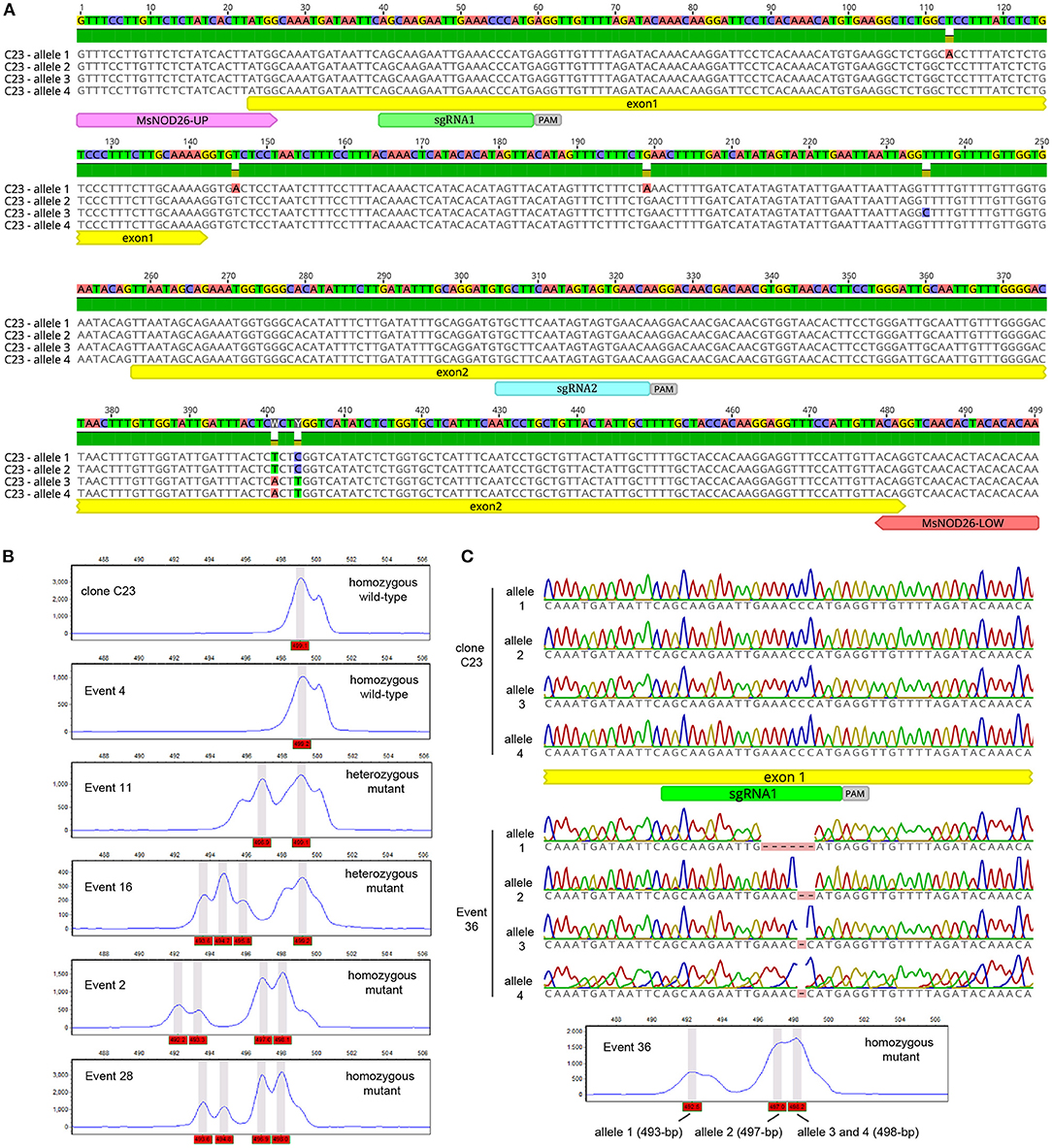
Figure 2. (A) Nucleotide sequence of wild-type alleles of MsNOD26 gene (MSAD_247675) from clone C23. In this picture, we highlighted the first two exons (exon1 and exon2), the two guides (sgRNA1 and sgRNA2) and the sequence recognized by the Cas9 nuclease (PAM sequences) and the primers (MsNOD26-up and MsNOD26-low) used for the identification of edited events using high-resolution fragment assays. (B) Flow cytometry fluorescence intensities of wild-type (499-bp) and mutant (492–498 bp) alleles of MsNOD26 gene. This figure shows representative examples of different genotypes. (C) Sequence analysis showed small deletions (1, 2, and 6-bp) within the alleles of MsNOD26 gene in CRISPR/Cas9-editing event 36.
The alfalfa-related species Medicago truncatula has been consolidated as the model plant for legume genetics and genomics, due to several important features, including its diploid genome, short generation time, small genome size, capacity to reproduce by self- and out-crossing, and the availability of its complete genome sequence and vast mutant libraries. In addition, there are clear examples that elegantly illustrate how knowledge gained from this model species can be used to improve alfalfa (Yang et al., 2008; Gou et al., 2018). However, as normally observed for any pair of related plant species, Medicago truncatula and alfalfa have numerous differences that limit the application of this model in alfalfa breeding. Since cultivated alfalfa is a tetraploid, allogamous, perennial species, species-specific factors influence the survival and high production of an alfalfa stand (e.g., plant regrowth), which depends on complex allelic variants. Besides, although alfalfa exhibits high levels of self-incompatibility, the homozygosis in an individual locus can be rapidly and inexpensively generated via alfalfa selfing by using non-emasculated hand crosses under greenhouse conditions (Jozefkowicz et al., 2018). Consequently, full allelic knock-out of an individual alfalfa gene could be obtained by autofecundation of edited plants, independently of the efficiency of the CRISPR/Cas9 system to simultaneously target multiple alleles in this crop. In genetically heterozygous tetraploid potato, high-efficacy full-allelic CRISPR/Cas9 gene editing was obtained by replacing the Arabidopsis U6 promoter (the same used in this work to control of expression of sgRNA against the GUS reporter), driving expression of the CRISPR factors, with endogenous potato U6 promoters (Johansen et al., 2019). In this line, we recently generated native-optimized vectors (e.g., binary vectors containing alfalfa H3.2 promoter) to improve the CRISPR/Cas9 system in alfalfa (Pascuan et al., 2020). Moreover, the recent release of the genome sequence of alfalfa (Chen et al., 2020) may help to improve the design of sgRNA guides for editing alfalfa genes in the next years.
While there is a legal structure for the deregulation of transgenic crops and there are several commercial transgenic cultivars in the world, practically no country envisages the release of genetically modified microbes (e.g., recombinant nitrogen-fixing rhizobia) into agroecosystems. Consequently, symbiotic nitrogen fixation improvement in legume crops (e.g., Soybean and Alfalfa) via genetic manipulation requires the engineering of the host genome, at least in the next years or few decades. Recently, we described a robust platform for the incorporation of transgenic traits into elite germplasm (Jozefkowicz et al., 2018), which can be easily adapted to the production of edited varieties (Figure 3). In this context, we propose the use of the public clone C23 and CRISPR/Cas9 system for the rapid incorporation of alfalfa mutants in public breeding programs, and the application of the genome-editing technology to guide the engineering of the nitrogen fixation system in this major legume crop.
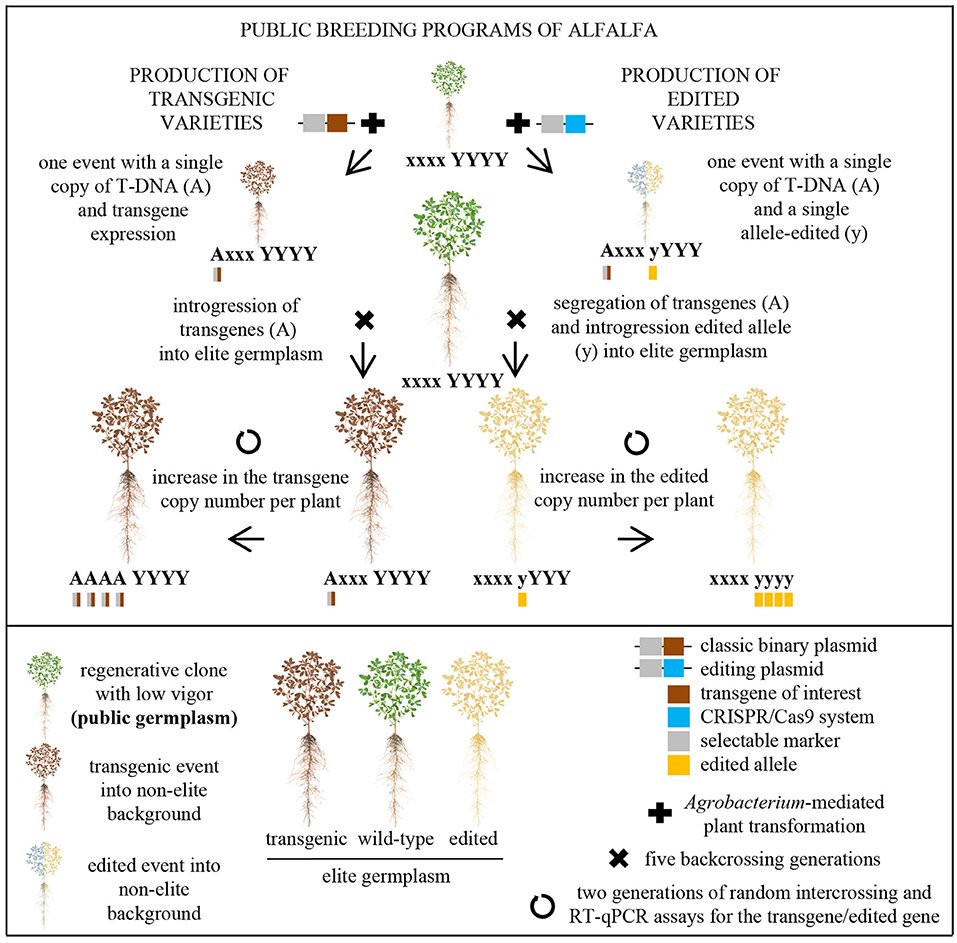
Figure 3. Schematic representation of the similarities between the production of public commercial alfalfa varieties containing only one transgenic (left) or edited (right) trait. These approaches contain the same three critical steps: the transformation of a public alfalfa germplasm (e.g., clone C23) with a binary plasmid via Agrobacterium tumefaciens, the introgression of the transgene or the edited allele into elite germplasm, and the increase in the transgenic or the edited copy number per plant.
Data Availability Statement
The datasets presented in this study can be found in online repositories. The names of the repository/repositories and accession number(s) can be found in the article/Supplementary Material.
Author Contributions
GS designed and funded the experiments. EBo, MS, RF, GM, MG, HT, and CG performed the experiments. EBo, MS, RF, GM, MG, HT, CG, SF, NA, EBl, and GS helped with the experiments and data analysis. GS, NA, SF, and EBl wrote the manuscript. All authors contributed to the article and approved the submitted version.
Funding
This work was supported by Grant PICT 2017-0484 to GS and by INTA PE I115. EBo, MG, and MS were supported by fellowships from CONICET and IUBMB.
Conflict of Interest
The authors declare that the research was conducted in the absence of any commercial or financial relationships that could be construed as a potential conflict of interest.
Acknowledgments
We thank Carmen Soria for her technical support.
Supplementary Material
The Supplementary Material for this article can be found online at: https://www.frontiersin.org/articles/10.3389/fagro.2021.661526/full#supplementary-material
References
Belhaj, K., Chaparro-Garcia, A., Kamoun, S., and Nekrasov, V. (2013). Plant genome editing made easy: targeted mutagenesis in model and crop plants using the CRISPR/Cas system. Plant Methods 9:39. doi: 10.1186/1746-4811-9-39
Bortesi, L., and Fischer, R. (2015). The CRISPR/Cas9 system for plant genome editing and beyond. Biotechnol. Adv. 33, 41–52. doi: 10.1016/j.biotechadv.2014.12.006
Castel, B., Tomlinson, L., Locci, F., Yang, Y., and Jones, J. D. G. (2019). Optimization of T-DNA architecture for Cas9-mediated mutagenesis in Arabidopsis. PLoS ONE 14:e0204778. doi: 10.1371/journal.pone.0204778
Cermak, T., Curtin, S. J., Gil-Humanes, J., Cegan, R., Kono, T. J. Y., Konecna, E., et al. (2017). A multipurpose toolkit to enable advanced genome engineering in plants. Plant Cell 29, 1196–1217. doi: 10.1105/tpc.16.00922
Chen, H., Zeng, Y., Yang, Y., Huang, L., Tang, B., Zhang, H., et al. (2020). Allele-aware chromosome-level genome assembly and efficient transgene-free genome editing for the autotetraploid cultivated alfalfa. Nat. Commun. 11:2494. doi: 10.1038/s41467-020-16338-x
Cuyeu, R., Pagano, E., Rosso, B., Soto, G., and Ayub, N. D. (2014). The genetic diversity of wild rescuegrass is associated with precipitation levels. J. Genet. 93, e29–34. doi: 10.1007/s12041-014-0349-0
Cuyeu, R., Rosso, B., Pagano, E., Soto, G., Fox, R., and Ayub, N. D. (2013). Genetic diversity in a world germplasm collection of tall fescue. Genet. Mol. Biol. 36, 237–242. doi: 10.1590/S1415-47572013005000021
Frare, R., Ayub, N., Alleva, K., and Soto, G. (2018). The ammonium channel NOD26 is the evolutionary innovation that drives the emergence, consolidation, and dissemination of nitrogen-fixing symbiosis in angiosperms. J. Mol. Evol. 86, 554–565. doi: 10.1007/s00239-018-9867-3
Gao, R., Feyissa, B. A., Croft, M., and Hannoufa, A. (2018). Gene editing by CRISPR/Cas9 in the obligatory outcrossing Medicago sativa. Planta 247, 1043–1050. doi: 10.1007/s00425-018-2866-1
Garcia, A. N., Ayub, N. D., Fox, A. R., Gomez, M. C., Dieguez, M. J., Pagano, E. M., et al. (2014). Alfalfa snakin-1 prevents fungal colonization and probably coevolved with rhizobia. BMC Plant Biol. 14:248. doi: 10.1186/s12870-014-0248-9
Gou, J., Debnath, S., Sun, L., Flanagan, A., Tang, Y., Jiang, Q., et al. (2018). From model to crop: functional characterization of SPL8 in M. truncatula led to genetic improvement of biomass yield and abiotic stress tolerance in alfalfa. Plant Biotechnol. J. 16, 951–962. doi: 10.1111/pbi.12841
Johansen, I. E., Liu, Y., Jørgensen, B., Bennett, E. P., Andreasson, E., Nielsen, K. L., et al. (2019). High efficacy full allelic CRISPR/Cas9 gene editing in tetraploid potato. Sci. Rep. 9:17715. doi: 10.1038/s41598-019-54126-w
Jozefkowicz, C., Bottero, E., Pascuan, C., Pagano, E., Ayub, N. D., and Soto, G. (2016). Minimizing the time and cost of production of transgenic alfalfa libraries using the highly efficient completely sequenced vector pPZP200BAR. Plant Cell Rep. 35, 1987–1990. doi: 10.1007/s00299-016-2026-7
Jozefkowicz, C., Frare, R., Fox, R., Odorizzi, A., Arolfo, V., Pagano, E., et al. (2018). Maximizing the expression of transgenic traits into elite alfalfa germplasm using a supertransgene configuration in heterozygous conditions. Theor. Appl. Genet. 131, 1111–1123. doi: 10.1007/s00122-018-3062-1
Kelemen, Z., Mai, A., Kapros, T., Feher, A., Gyorgyey, J., Waterborg, J. H., et al. (2002). Transformation vector based on promoter and intron sequences of a replacement histone H3 gene. A tool for high, constitutive gene expression in plants. Transgenic Res 11, 69–72. doi: 10.1023/A:1013923826979
Menz, J., Modrzejewski, D., Hartung, F., Wilhelm, R., and Sprink, T. (2020). Genome edited crops touch the market: a view on the global development and regulatory environment. Front. Plant Sci. 11:586027. doi: 10.3389/fpls.2020.586027
Narimatsu, Y., Joshi, H. J., Yang, Z., Gomes, C., Chen, Y. H., Lorenzetti, F. C., et al. (2018). A validated gRNA library for CRISPR/Cas9 targeting of the human glycosyltransferase genome. Glycobiology 28, 295–305. doi: 10.1093/glycob/cwx101
Nekrasov, V., Staskawicz, B., Weigel, D., Jones, J. D., and Kamoun, S. (2013). Targeted mutagenesis in the model plant Nicotiana benthamiana using Cas9 RNA-guided endonuclease. Nat. Biotechnol. 31, 691–693. doi: 10.1038/nbt.2655
Pascuan, C., Bottero, E., Kapros, T., Ayub, N., and Soto, G. (2020). pBAR-H3.2, a native-optimized binary vector to bypass transgene silencing in alfalfa. Plant Cell Rep. 39, 683–685. doi: 10.1007/s00299-020-02521-3
Shen, W. J., and Forde, B. G. (1989). Efficient transformation of Agrobacterium spp. by high voltage electroporation. Nucleic Acids Res. 17:8385. doi: 10.1093/nar/17.20.8385
Stritzler, M., Elba, P., Berini, C., Gomez, C., Ayub, N., and Soto, G. (2018). High-quality forage production under salinity by using a salt-tolerant AtNXH1-expressing transgenic alfalfa combined with a natural stress-resistant nitrogen-fixing bacterium. J. Biotechnol. 276–277, 42–45. doi: 10.1016/j.jbiotec.2018.04.013
Weber, E., Engler, C., Gruetzner, R., Werner, S., and Marillonnet, S. (2011). A modular cloning system for standardized assembly of multigene constructs. PLoS ONE 6:e16765. doi: 10.1371/journal.pone.0016765
Wolabu, T. W., Cong, L., Park, J. J., Bao, Q., Chen, M., Sun, J., et al. (2020). Development of a highly efficient multiplex genome editing system in outcrossing tetraploid alfalfa (Medicago sativa). Front. Plant Sci. 11:1063. doi: 10.3389/fpls.2020.01063
Keywords: alfalfa, genome editing, CRISPR/Cas9, mutants, transformation
Citation: Bottero E, Massa G, González M, Stritzler M, Tajima H, Gómez C, Frare R, Feingold S, Blumwald E, Ayub N and Soto G (2021) Efficient CRISPR/Cas9 Genome Editing in Alfalfa Using a Public Germplasm. Front. Agron. 3:661526. doi: 10.3389/fagro.2021.661526
Received: 30 January 2021; Accepted: 17 June 2021;
Published: 16 July 2021.
Edited by:
Juan Sanjuan, Consejo Superior de Investigaciones Científicas (CSIC), SpainReviewed by:
Jun Shan, Institute of Soil Science (CAS), ChinaLonglong Wang, Huazhong Agricultural University, China
Copyright © 2021 Bottero, Massa, González, Stritzler, Tajima, Gómez, Frare, Feingold, Blumwald, Ayub and Soto. This is an open-access article distributed under the terms of the Creative Commons Attribution License (CC BY). The use, distribution or reproduction in other forums is permitted, provided the original author(s) and the copyright owner(s) are credited and that the original publication in this journal is cited, in accordance with accepted academic practice. No use, distribution or reproduction is permitted which does not comply with these terms.
*Correspondence: Gabriela Soto, c290by5nYWJyaWVsYWNpbnRoaWFAaW50YS5nb2IuYXI=; orcid.org/0000-0002-3492-1268