- 1Department of Plant Pathology and Weed Research, Agricultural Research Organization, Newe Ya'ar Research Center, Ramat Yishay, Israel
- 2The Robert H. Smith Institute of Plant Sciences and Genetics in Agriculture, Faculty of Agriculture, Food and Environment, The Hebrew University of Jerusalem, Rehovot, Israel
Parthenium weed (Parthenium hysterophorus L.) (Asteraceae; Heliantheae) is an invasive allergenic species that has invaded many countries worldwide, probably through an imported pasture, grain seeds, and livestock feed. In recent years, there has been increasing concern about the spread of the invasive P. hysterophorus in agricultural and non-agricultural habitats across Israel. In addition, as P. hysterophorus is a quarantine plant; any contaminated produce exported will be rejected by the European market. The current study aims to document the current distribution and invasiveness status of P. hysterophorus in Israel. Moreover, we aimed to study the life cycle and biology of P. hysterophorus. In this research, we detected invasion reports to new areas and habitats in the Jezreel valley, the Jordan valley, and the Mediterranean coastal plain. Studying the biology of the weed, we found that optimal temperatures for seed germination are between 15 and 25°C. We observed that P. hysterophorus seeds are able to emerge from a depth of 0–3 cm only. P. hysterophorus thrives under high light intensities. Our results show that under induced shading of 60 and 90%, a significant reduction in biomass, height, and a number of flowers per plant were observed. Studying the biology and phenology of P. hysterophorus is a crucial step in the path to develop an integrated management program aimed to reduce the further spread and negative impacts by P. hysterophorus.
Introduction
Invasive species present major economic and ecological threats to agricultural and natural areas. In recent years, we have been experiencing a rise in reports of invasive weed species due to a significant man-made global change. Among the leading causes for this trend are import–export trade (Levine et al., 2003; Shimono et al., 2010) and climate change (Peters et al., 2014; Hulme, 2016). In the United States alone, annual losses caused only by crop-related invasive weeds are estimated at more than $27 billion (Pimentel et al., 2005). The damage of invasive weeds is not restricted to yield losses; they may increase the spread of fire fuel (Mound, 2002; Setterfield et al., 2013; Büyüktahtakin et al., 2014), and health hazards-mainly allergies (Wopfner et al., 2005; Kaur et al., 2014; Hamaoui-Laguel et al., 2015). In Israel, several invasive species, such as Parthenium hysterophorus L., Verbesina encelioides (Cav.), Ambrosia confertiflora DC., and Amaranthus tuberculatus (Moq.) Sauer, apparently entered the country along with seed shipments imported for animal feed (Rubin and Matzrafi, 2015). P. hysterophorus was introduced into Israel in a wide range of ecosystems, the first one documented in 1980 at a fish farm in northern Israel (Yaacoby, 2013). In recent years, there has been a growing concern due to the spread of P. hysterophorus into the central part of the country and its increasing establishments in agricultural lands, such as orchards, as well as vegetable and field crops.
P. hysterophorus is an invasive weed species in more than 50 countries. It is considered as one of the worst weeds in the world due to its high fecundity (~20,000 seeds/plant), rapid germination, fast growth rate, and allelopathic nature (Adkins and Shabbir, 2014). P. hysterophorus seeds can germinate in a wide temperature range, and germination is mainly limited by soil moisture content (Navie et al., 1998a; Tamado et al., 2002b; Bajwa et al., 2018). This species is generally unpalatable, but, in the absence of other food sources, livestock will feed on it. Allergic effects of P. hysterophorus for both animals and humans include severe dermatitis, hay fever, and other symptoms (Kaur et al., 2014). P. hysterophorus thrives under high light intensities (Navie et al., 1998a) and elevated nitrogen levels (Singh, 2014). P. hysterophorus can cause 40–97% yield reductions in agricultural crops and acts as a secondary host for many crop plant diseases (Adkins and Shabbir, 2014). Effective P. hysterophorus management can be achieved via chemical (Reddy et al., 2007) and biological control (Navie et al., 1998b; Javaid and Adrees, 2009). In addition, the growth of highly competitive crops has been found to be very effective in suppressing both the emergence and initial growth of P. hysterophorus (Khan et al., 2013; Shabbir et al., 2013).
Hence, the objectives of the present study were the following: (1) to document the current distribution of P. hysterophorus throughout Israel and (2) to study the life cycle of P. hysterophorus by examining biological and phenological parameters, such as seed germination, emergence from soil depth, and the effect of shading on height, biomass production, and flowering of P. hysterophorus.
Materials and Methods
Distribution of P. hysterophorus
In order to better understand the distribution and invasiveness of P. hysterophorus throughout Israel, a detailed mapping of the infested sites and estimation of the level of infestation at each location was performed in 2018.
The mapping was done using ArcGIS (ArcGIS 9 Desktop. version 9.3.1, ESRI, Redlands, CA), with the application “Collector for ArcGis.” The main mapping of P. hysterophorus was focused on the northern part of Israel, as the majority of the reports came from this region. The mapping was performed using two methods: first, scouting for P. hysterophorus from a vehicle on the main roads, from the southern border of its known distribution in “Hefer Valley,” all the way to its northern border of distribution in the “Golan Heights.” Second, brochures and social media directed to local communities, farmers, agronomists, park rangers, and others who have sighted P. hysterophorus were distributed. These individuals were asked to access a link and update P. hysterophorus location in a designated map. After a reasonable number of sites were reported by human resources, we visited each location, confirmed the identification, analyzed the level of distribution for each site as well as the distribution of P. hysterophorus in a one-kilometer radius around each site. If a P. hysterophorus plant was sighted, an additional kilometer would be surveyed and so on, until no P. hysterophorus plants were detected. For each site, the adjacent farms and villages were surveyed in what we concluded to be “prime locations,” such as livestock farms, tool shades, and their surrounding perimeter. Data analysis and layer mapping were conducted using QGIS Desktop 2.8.1 (QGIS Delopment Team, 2018).
Plant Material
Seeds of P. hysterophorus were collected at the Newe Ya'ar Research Center (32.7115458716″N, 35.1777440341″E) in November 2015. To ensure appropriate representation of field population, mature inflorescence from 30 to 40 randomly selected plants were collected and pooled. Collected seeds were air dried and stored in the dark and at 4°C until used.
Effect of Temperature on Seed Germination
Seed germination was tested under constant and alternating temperatures. For this purpose, seeds were placed in a 9 cm Petri dish on a thin layer of Newe-Ya'ar soil collected at the same site as the seeds (57% clay, 28.2% silt, 8.1% sand, and 1.63% organic matter). Petri dishes were randomly placed in an incubator (Conviron®, plant growth chamber A1000, Winnipeg, Canada). To determine the effect of temperature on seed germination, 20 seeds were incubated in Petri dishes under eight different constant temperatures (5, 10, 15, 20, 25, 30, 35, and 40°C), 12 h photoperiod and watered as needed. Seed germination was recorded every day for 60 days. Experiments were replicated four times and arranged in a randomized complete block design.
Models for Predicting P. hysterophorus Development Dynamics
Linear Model
In order to develop thermal-time-based germination and development prediction models for P. hysterophorus, a calculation of accumulated growing degree days (GDDs) was used. Temperatures were converted to GDD units using Equation (1):
Accumulated GDDs are calculated using Tmax as the maximum daily temperature, Tmin as the minimum daily temperature, and Tbase as the minimum temperature for plant development (Mcmaster and Wilhelm, 1997).
Beta-Function Model
A four-parameter beta-function model was used to calculate the effect of temperature on P. hysterophorus germination dynamics using Equation (2):
where β is the calculated partial development rate, T is the hourly measured temperature, Tb is the minimal temperature for development, T0 is the optimal temperature for development, Tm is the maximal temperature for development, and a denotes the shape of the slope.
The β-value was multiplied by the measured soil temperature and summed to estimate the effect of temperature on P. hysterophorus dynamics, where Ri is the accumulated GDDs using Equation (3):
At this point, the β-value may be used to validate the GDD calculation using Equation (4) (Cochavi et al., 2016):
Effect of Burial Depth on Seed Emergence
Following seed germination, emergence from different burial depths was tested. This experiment was conducted in a net-house during a natural season for P. hysterophorus emergence, using the same soil used for seed germination studies. Seeds were sown at different burial depths (0, 1, 2, 3, 4, and 5 cm below soil surface) in 250 ml pots; experiments were arranged in a randomized complete block design with five seeds sown in each pot with five replicates for each treatment. Emergence was recorded for 30 days; A data logger (HOBO® data logger, Onset, USA) was used to collect temperature data during the course of the experiment. Emergence was recorded for each pot every day, and the emergence rate was calculated as a percentage of the total for each treatment.
The Effect of Shading on Vegetative and Reproductive Growth
To assess the effect of different shading levels (radiation intensities) on plant productivity, plant vegetative and reproductive parameters were tested under different shading levels; 0 (full natural light, 1,000–1,100 μmol m−2 s−1), 30, 60, and 90% shading. Experiments were performed in a net house during the natural growing season for P. hysterophorus in Israel (May–July). Pre-germinated seedlings at the stage of 2–3 true leaves were sown in 2.5 L pots filled with the soil of Newe-Ya'ar. Different shading levels were achieved using a black-shading net. For each treatment, 50 plants were used. Plant height, number of flowers, and aboveground biomass were recorded each week using five plants. Plants were irrigated and fertilized (Ecogan®, NPK 20:20:20, Israel) as needed. A data logger (HOBO®) was used for temperature recording during the course of the experiment.
Statistical Analyses
Data were analyzed and visualized using SigmaPlot (ver. 12) software (Systat Software Inc., San Jose, CA, USA). Parameters were optimized using the Solver function (Excel software, Microsoft Office Professional Plus 2016). The dynamics of seed germination were described using both the sigmoidal three parameters model as described in Equation (5):
and log-logistic three parameters model as described in Equation (6):
For both equations, X0 is the inflection point, b is the slope in the inflection point, and a is the upper asymptote.
Curve fitting was estimated according to the root mean square error values (RMSE), where an RMSE-value represents the error between the observed and expected value, as described in Equation (7):
Lower RMSE-values indicate a better-fitted model (Lati et al., 2011a).
Results
Distribution of P. hysterophorus
P. hysterophorus distribution across the northern region of Israel is shown in Figure 1. Populations were clustered in three main hot spot areas (Figure 1A). The first reported invasion sites were in the Beit Shean Valley, and two more major areas of infestation were reported in the Jordan valley and the Jezreel valley. In addition, several scattered populations were recorded in the Mediterranean coastal plain and in the upper Galili region in the Hula valley. It is important to state that no reports of P. hysterophorus populations were found south of the reported area.
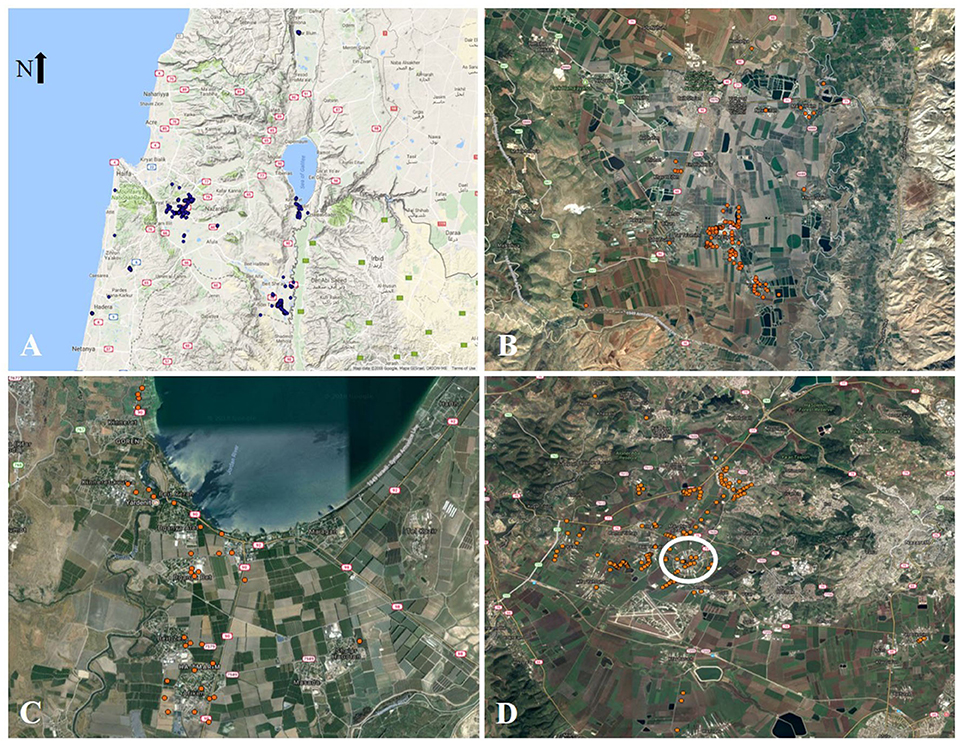
Figure 1. Distribution of Parthenium hysterophorus across Israel. (A) Overview of the current P. hysterophorus distribution region. (B) Specific distribution in the Beit Shean Valley, (C) the Jordan Valley, and (D) the Jezreel Valley.
As previously described, Beit Shean Valley is assumed to be the first introduction area for P. hysterophorus in Israel (Yaacoby, 2011). In our survey, plants from this region were detected in both cultivated and non-arable habitats, including roadsides, lawns, private gardens, field margins, and orchards (Figure 1B). For the Jordan valley hot spot, plants were detected alongside route 90 and were found to be scattered mainly in agricultural areas along the road (Figure 1C). However, in several communities such as Kibbutz Degania Bet and Kvutzat Kinneret, a high infestation level was recorded in close proximity to neighborhoods and public gardens. The distribution of P. hysterophorus in the Jezreel valley has several moderated infestation spots (Figure 1D). These spots are present in both cultivated fields and private gardens. Interestingly, one of the major hot spots of P. hysterophorus plants found in this area was detected near a livestock feed distribution industry located near Nahalal (circled in white in Figure 1D).
Seed Germination
P. hysterophorus germination was tested under eight constant temperatures for 60 days. Our findings suggest a high association between germination and incubation temperature (Table 1). For the lowest temperature of 5°C, maximum germination was only 8%, recorded after a long incubation period of 54 days (Figure 2A). The highest temperature for P. hysterophorus seed germination was 35°C. The time required for maximum germination at this temperature was 21 days; however, the final germination rate was still lower than 10% (Figure 2G). The optimal temperatures for seed germination of P. hysterophorus were 20–25°C. For both temperatures, high germination rates of 92 and 99% were achieved after 10 and 14 days, respectively (Figures 2D,E). The time required to achieve 50% germination at these two constant temperatures was the lowest compared with all other incubation temperatures (Table 1). For seeds incubated at 15°C, the germination rate reached a total of 78% after 21 days (Figure 2C). No germination was recorded when seeds were incubated at a temperature of 40°C (Figure 2H).
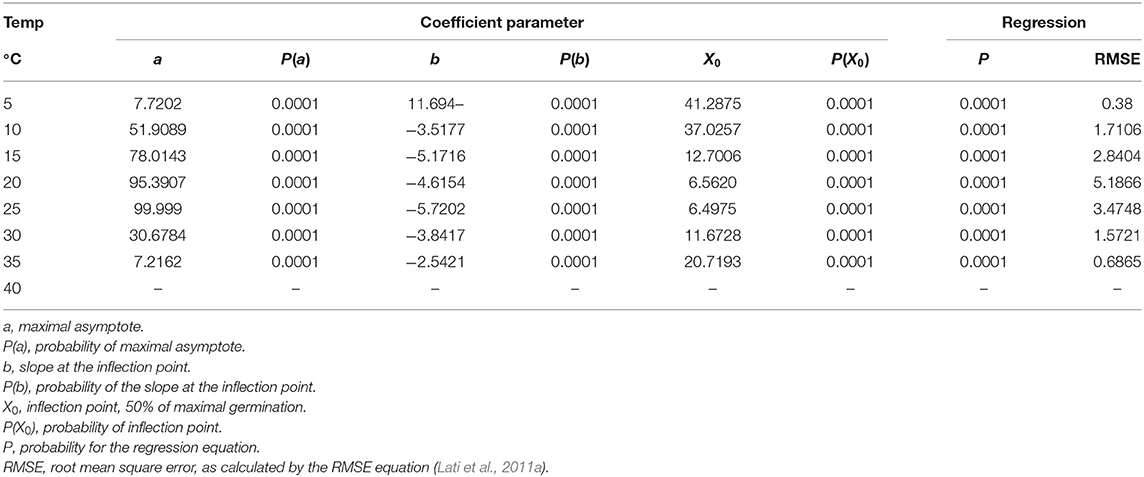
Table 1. The coefficient of three parameters log-logistic equation fitted to describe the germination of Parthenium hysterophorus seeds under constant temperatures.
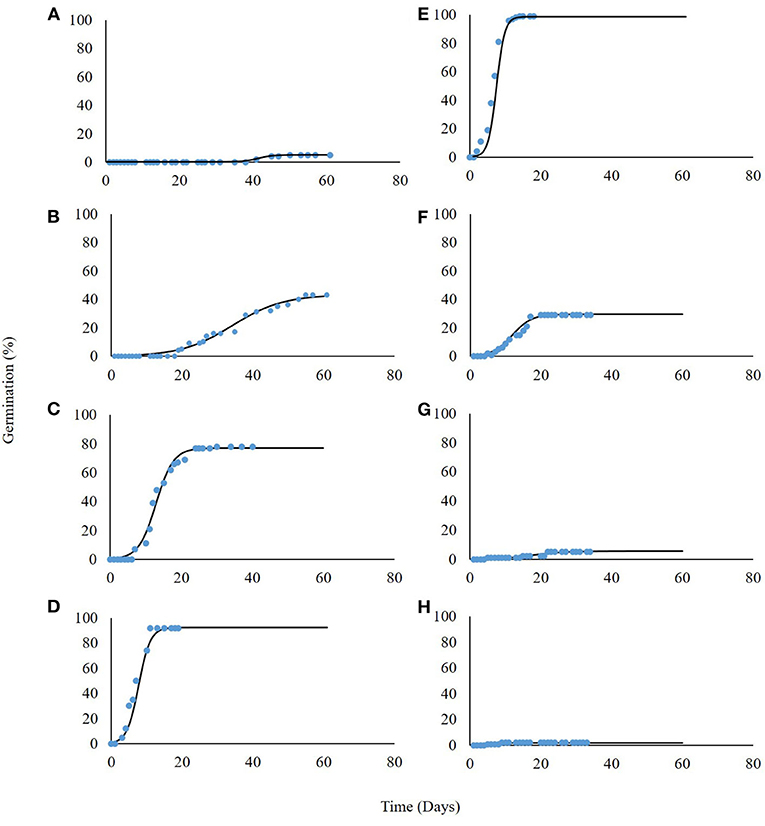
Figure 2. Germination of P. hysterophorus seeds under constant temperatures: 5, 10, 15, 20, 25, 30, 35, and 40°C (A–H, respectively). The coefficient for non-linear regressions are given in Table 1.
P. hysterophorus Germination Dynamics
Data collected in germination tests under constant temperature regimes were used for the development of a beta-function model to better fit P. hysterophorus seed germination dynamics to temperature (Figure 3A). This model is in agreement with previous studies related to seed germination (Xinyou and Kropff, 1996; Cochavi et al., 2016; Goldwasser et al., 2016). According to the model, the P. hysterophorus seed germination rate increases as the temperature rises from a minimal value (5°C) to the optimal value (25°C). At supraoptimal temperature, the germination rate decreases until the ceased temperature are reached (40°C) (Figure 3A).
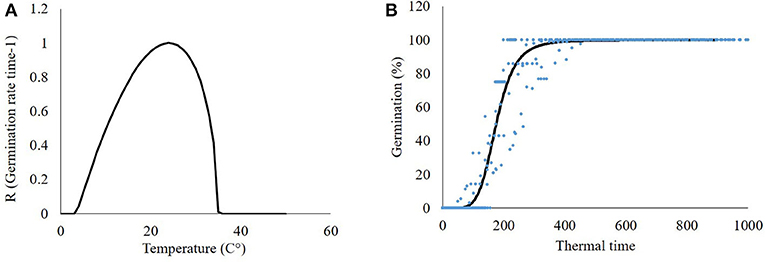
Figure 3. Mathematical models describing the germination dynamics of Parthenium hysterophorus seeds. (A) Beta-function model describing the rate and maximum temperature-dependent germination and (B) seed germination dynamics according to a log-logistic model.
Accumulated seed germination is estimated by GDD. Based on the beta-function equation and parameters that were developed in the previous stage, a GDD accumulation of the seed germination model was developed (Figure 3B). P. hysterophorus seed germination started after 130 GDD, and 50% germination was achieved after 175 GDD. Maximum germination was recorded at 350 GDD. Reviewing both models, an equation predicting seed germination was developed Equation (8):
Seed Emergence
Seedling emergence was assessed from soil depths of one to five cm. Both the emergence rate and total emergence percentage were in negative correlation with the planting depth, i.e., P. hysterophorus emergence decreased dramatically with increasing planting depth (Figure 4). Seeds that were placed on the soil surface (0 cm) showed 76% emergence; the rate of emergence as described by parameter b (Equation 6) was the highest as compared with seeds emergence from other tested planting depths (Table 2). Seeds sown at both 1- and 2-cm-soil depths showed emergence of 64 and 62% and an emergence rate of 2.1 and 2.5%, respectively (Figures 4B,C; Table 2). Only 28% emergence was recorded for seeds sown at 3 cm soil depth, whereas seeds sown at 4- and 5 cm depth did not emerge at all (Table 2).
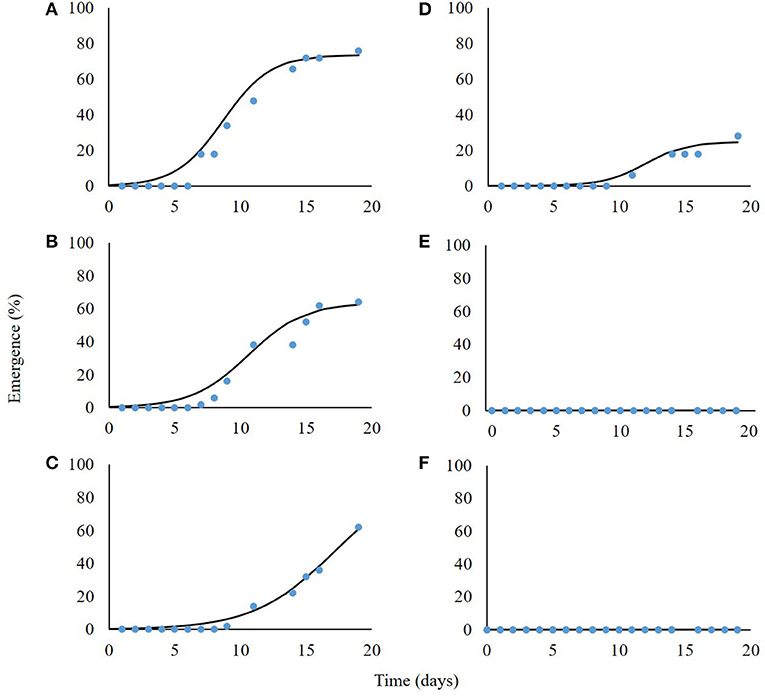
Figure 4. Seedlings emergence of Parthenium hysterophorus seeds sown in different soil depths: 0, 1, 2, 3, 4, and 5 cm (A–F, respectively). Coefficient data are given in Table 2.
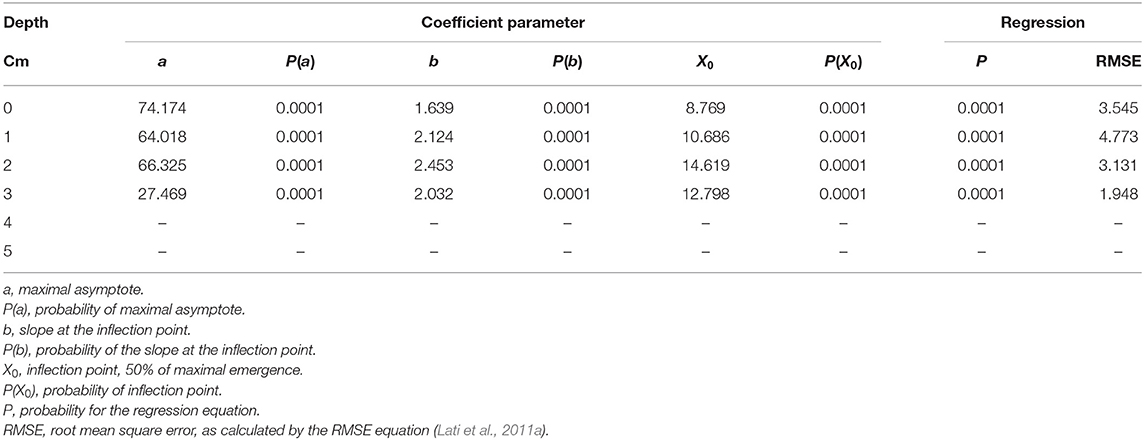
Table 2. The coefficient of three parameters log-logistic equation fitted to describe the emergence dynamics of Parthenium hysterophorus seeds sown in different soil depths.
The Effect of Shading on Vegetative and Reproductive Growth
Plant Biomass
The shading level had a negative effect on plant biomass, reduced light intensity resulted in shoot biomass reduction, and significant differences were found among shading levels (Table 3). For the non-shading control treatment (0% shading), the maximal asymptote that represents maximal accumulation of shoot weight was 28 g (Figure 5A). At the 30% shading level, maximal biomass accumulation was 24.2 g (Figure 5B). A higher reduction in biomass was recorded at the 60% shading level when the maximal accumulated plant weight reached only 16.1 g (Figure 5C). The highest effect of the radiation level on plant biomass accumulation was recorded for the 90% shading level. Accumulated shoot biomass was only 2.3 g. Moreover, for the 90% shading level, biomass accumulation peaked at 350 GDD, and no significant increase in plant biomass was shown for the rest of the experiment (Figure 5D).
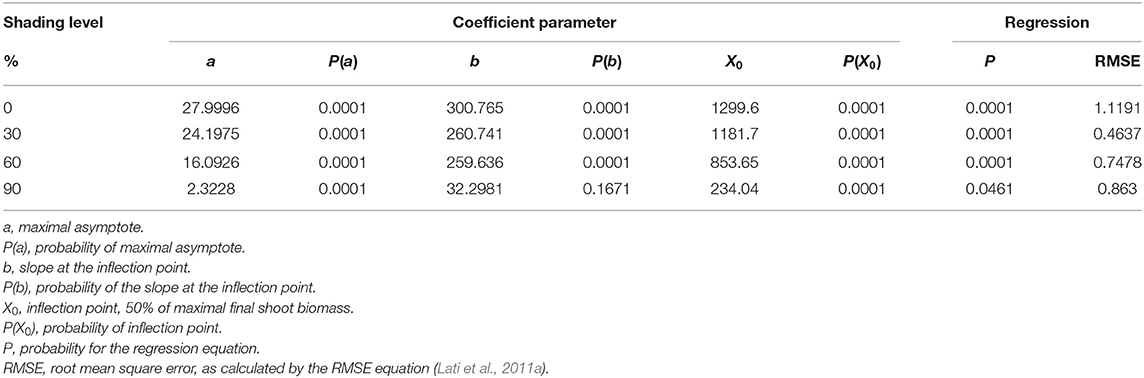
Table 3. The coefficient of three parameters log-logistic equation fitted to describe the effect of shade levels on Parthenium hysterophorus final shoot biomass.
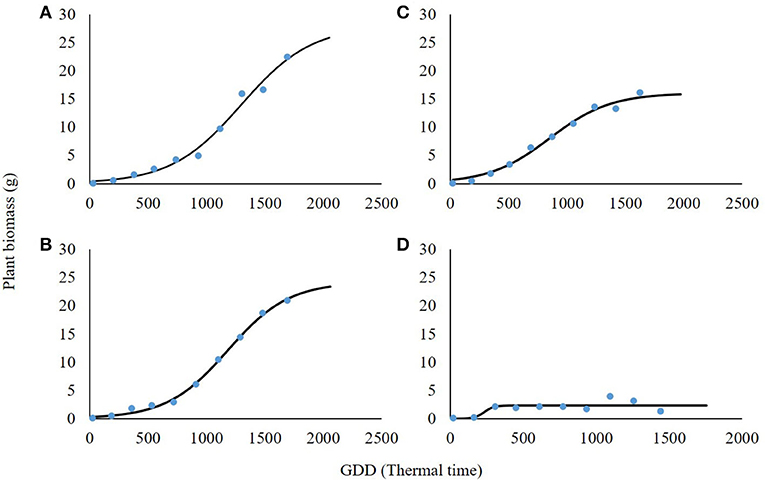
Figure 5. Effect of shade levels (0, 30, 60, and 90%; A–D, respectively) on final shoot biomass of Parthenium hysterophorus. The coefficient for non-linear regressions is given in Table 3.
Plant Height
As in the case of plant biomass, plant height was also in a negative correlation with shading levels (Figure 6; Table 4). At 0% shading, the accumulated maximal plant height was 128.9 cm. Shading levels of 30 and 60% resulted in maximal plant heights of 88.8 and 77.2 cm accumulated, respectively. At the 90% shading level treatment, plants were not able to complete their life cycle and transition from the rosette stage to the bolting stage. Plant height at the 90% shading level ranged from 2.6 to 6 cm accumulated over 1,000 GDD with no further change until the end of the experiment (Figure 6D). For all other treatments (0, 30, and 60% shading levels), the transition from rosette to the bolting stage occurred at approximately 1,000 GDD.
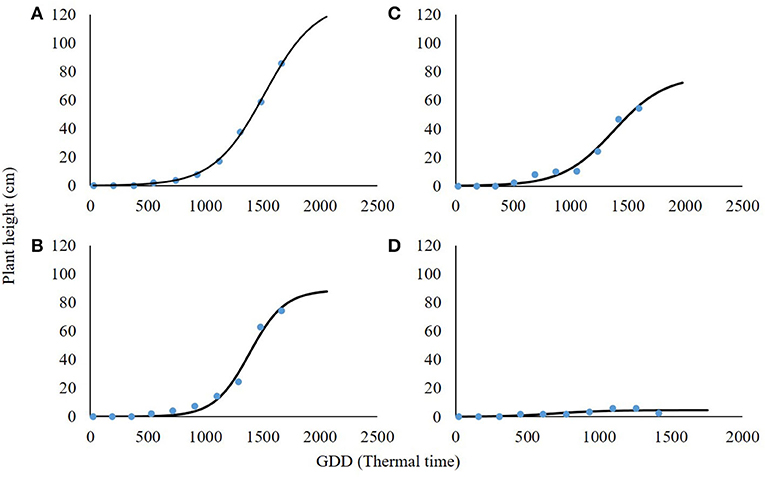
Figure 6. Effect of shade levels (0, 30, 60, and 90%; A–D, respectively) on final plant height of Parthenium hysterophorus. The coefficient for non-linear regressions is given in Table 4.
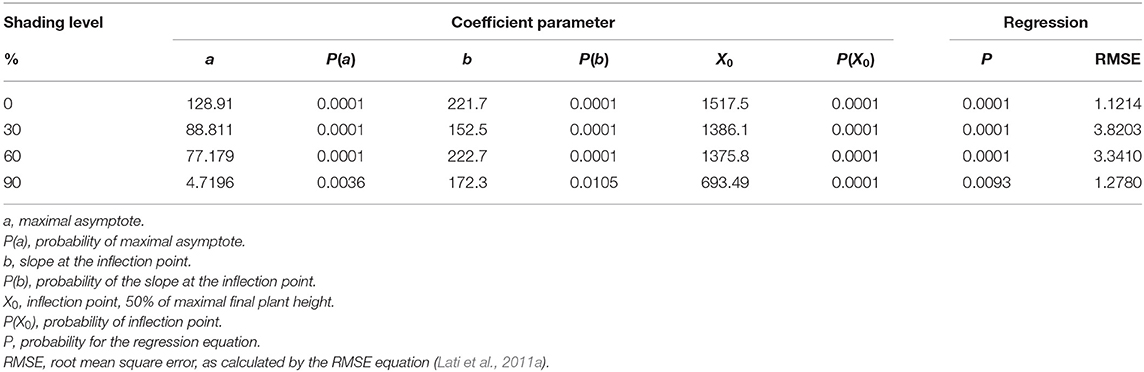
Table 4. The coefficient of three parameters log-logistic equation fitted to describe the effect of shade levels of Parthenium hysterophorus on final plant height.
Inflorescence Development
High-radiation levels accelerated the development of plant inflorescence (Figure 7). For both 0 and 30% shading, the maximal amount of flowers per plant was 71.1 and 81.5, respectively (Table 5). Total flowering was higher by 13% for the 30% shading in comparison with the 0% shading level as presented by the a parameter (maximum flowering) in Table 5. At the 60% shading level, the maximal number of flowers per plant was 42.1, and, for the 90% shading level, flower initiation did not occur. The transition from the vegetative to reproductive stage occurred under all shading treatments, except for the 90% shading level at approximately 1,000 GDD and corresponding to the changeover from rosette to the bolting stage described above.
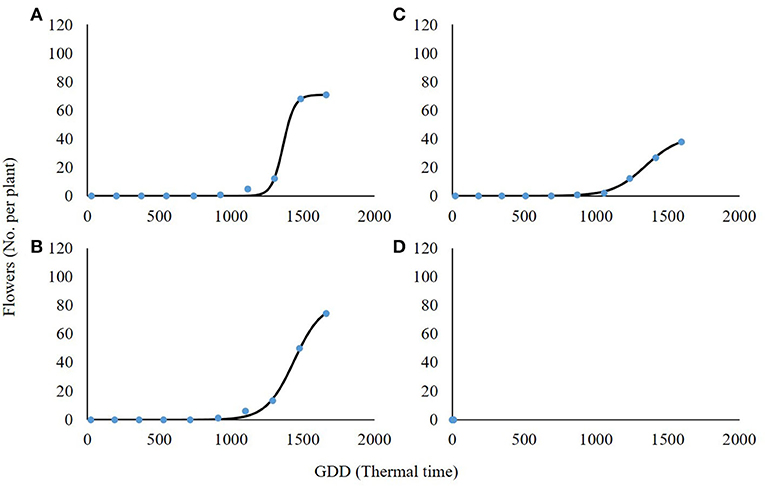
Figure 7. Effect of shade levels (0, 30, 60, and 90%; A–D, respectively) on the final number of flowers per plant of Parthenium hysterophorus. The coefficient for non-linear regressions is given in Table 5.
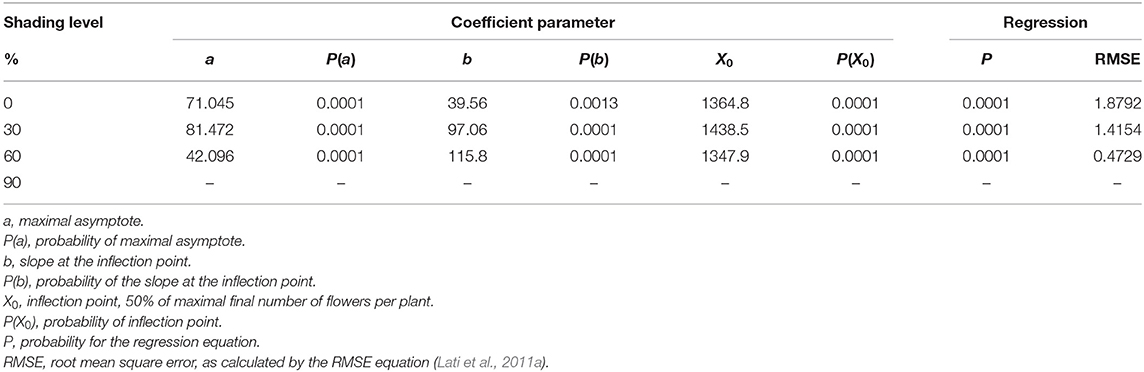
Table 5. The coefficient of three parameters log-logistic equation fitted to describe the effect of shade levels on Parthenium hysterophorus final number of flowers per plant.
Discussion
Colonization of invasive species can be characterized in two main stages: the lag phase between initial introduction and subsequent rapid population growth, and the exponential phase when explosive range expansion occurs (Radosevich et al., 2007). In Israel, these two main stages can be detected. P. hysterophorus populations found at Beit Shean Valley, Jezreel Valley, and Jordan Valley are showing vast distribution, aggressive expansion, and high competitiveness as compared with the local vegetation. In addition, the proximity of hot spots to human-populated areas indicates significant anthropogenic influence on the spread of the weed. In populations found at specific habitats such as the one found along the coastline of the Mediterranean coastal plain and in the Hula Valley, plants are located in dense clusters with minimal expansion. The distribution pattern of an invasive weed in a specific area is a key factor in the control efforts. Limited distribution across a specific site may increase the success of chemical and non-chemical weed management methods.
Previous studies have hypothesized that the invasion of weeds such as P. hysterophorus into the Israeli flora is facilitated by grain shipments imported for animal feed (Rubin and Matzrafi, 2015). This is in agreement with previous studies, describing P. hysterophorus invasion pathways (Vertak, 1968; Haseler, 1976; Tamado et al., 2002a). Our results show a high correlation between the presence of P. hysterophorus and close proximity to dairy barns, animal farms, and agricultural tool sheds. These patterns have mostly been found in new introduction areas such as the Mediterranean coastal plain (Figure 1A). However, further and more thorough work should be done to better understand these pathways of spread.
P. hysterophorus seed germination was found to be correlated with temperature increase (Figure 2). Overall, temperature increase resulted in higher seed germination; however, over the optimal temperature of 24°C, the germination rate declined. Previous work exploring the effect of temperature on P. hysterophorus seed germination has shown that different biotypes may germinate over a wide range of constant temperatures (8–32°C) under dark conditions (Bajwa et al., 2018). Tamado et al. (2002b) showed that germination occurred at a minimum of 10°C and a maximum of 25°C. Williams and Groves (1980) concluded that only temperatures higher than 35°C or lower than 5°C limit the germination of P. hysterophorus. In agreement with other studies, the optimal range for P. hysterophorus germination found in our study is 20–25°C. The GDD model based on beta-function was found to be very precise in describing P. hysterophorus seed germination dynamics (Figure 3). Good understanding of the germination dynamics may be harnessed to the process of developing new integrated weed control approaches suitable for P. hysterophorus biology as proposed for other weed species (Lati et al., 2011b; Eizenberg et al., 2012; Cochavi et al., 2015, 2016).
Examining the effect of burial depth on seed emergence showed a negative relation between burial depth and the emergence rate (Figure 3). Tamado et al. (2002b) showed that shallowly buried (0–1 cm) seeds had a high emergence rate (~60%) compared with seeds buried at 2–3 cm (10–20%). This negative correlation was also found for several other weed species such as Amaranthus palmeri (Sosnoskie et al., 2013), Rumex obtusifolius (Benvenuti et al., 2001), and Datura stramonium (Benvenuti and Macchia, 1995). Germination inhibition caused by burial depth might be due to secondary dormancy linked to interactions between seed metabolism and the soil gas environment, or depletion of seed energy reserves (Benvenuti and Macchia, 1995, 1997). From a practical point of view, the deep burial of seeds using mechanical cultivation methods may reduce the emergence rate and thus increase the depletion of a viable seed bank as a weed-control approach.
Studying the effect of different shading levels on plant development, three different parameters were examined: shoot biomass, plant height, and flowering time. Our results show that high-light intensity conditions resulted in increased shoot biomass and taller plants as compared with plants grown under low-light intensities (Figures 5, 6). The same trend was found in A. tuberculatus var. rudis when plant biomass and seed production were found to be lower as radiation levels decreased to 60, 32, and 1% light intensity (Steckel et al., 2003). For Echinochloa crus-galli, the shading level of 75% resulted in a 22% reduction in plant height; however, this was not the case for two other Echinochloa species (E. colona and E. glabrescens) (Chauhan, 2013). The same study showed that seed production for all Echinochloa species declined under reduced radiation levels. In Abutilon theophrasti, no differences were observed for plant height, leaves, or branches per plant when plants were grown under a shading level of 30%; however, for the 76% shading, reduced height, leaf number, stem branches, and plant biomass were observed (Bello et al., 1995). The effect of shading can be translated to weed/crop competition. As P. hysterophorus thrives under high-light intensities (Navie et al., 1998a), using strong competitive crops such as corn, cotton, and others may impair the growth of the weed, thus reducing its overall competitive ability. Studying the biology, phenology, and weed-crop competition aspects of P. hysterophorus is crucial in order to identify key traits that can improve its management.
Data Availability Statement
The raw data supporting the conclusions of this article will be made available by the authors, without undue reservation.
Author Contributions
HR, BR, TY, and HE conceived the study and advised on the methodology. HR and MM conducted the experiments and statistical analyses. MM, HR, and HE wrote the manuscript, with contributions from BR and TY. All authors contributed to the article and approved the submitted version.
Conflict of Interest
The authors declare that the research was conducted in the absence of any commercial or financial relationships that could be construed as a potential conflict of interest.
Acknowledgments
The authors wish to thank the Chief Scientist of the Ministry of Agriculture of Israel for funding this project.
References
Adkins, S., and Shabbir, A. (2014). Biology, ecology and management of the invasive parthenium weed (Parthenium hysterophorus L.). Pest Manag. Sci. 70, 1023–1029. doi: 10.1002/ps.3708
Bajwa, A. A., Chauhan, B. S., and Adkins, S. W. (2018). Germination ecology of two Australian biotypes of ragweed parthenium (Parthenium hysterophorus) relates to their invasiveness. Weed Sci. 66, 62–70. doi: 10.1017/wsc.2017.61
Bello, I. A., Owen, M. D. K., and Hatterman-Valenti, H. M. (1995). Effect of shade on velvetleaf (Abutilon theophrasti) growth, seed production, and dormancy. Weed Technol. 9, 452–455. doi: 10.1017/S0890037X00023678
Benvenuti, S., and Macchia, M. (1995). Effect of hypoxia on buried weed seed germination. Weed Res. 35, 343–351. doi: 10.1111/j.1365-3180.1995.tb01629.x
Benvenuti, S., and Macchia, M. (1997). Germination ecophysiology of bur beggarticks (Bidens tripartita) as affected by light and oxygen. Weed Sci. 45, 696–700.
Benvenuti, S., Macchia, M., and Miele, S. (2001). Light, temperature and burial depth effects on Rumex obtusifolius seed germination and emergence. Weed Res. 41, 177–186. doi: 10.1046/j.1365-3180.2001.00230.x
Büyüktahtakin, I. E., Feng, Z., Olsson, A. D., Frisvold, G., and Szidarovszky, F. (2014). Invasive species control optimization as a dynamic spatial process: an application to buffelgrass (Pennisetumciliare) in Arizona. Invasive Plant Sci. Manag. 7, 132–146. doi: 10.1614/IPSM-D-13-00057.1
Chauhan, B. S. (2013). Shade reduces growth and seed production of Echinochloacolona, Echinochloa crus-galli, and Echinochloa glabrescens. Crop Prot. 43, 241–245. doi: 10.1016/j.cropro.2012.10.009
Cochavi, A., Achdari, G., Smirnov, E., Rubin, B., and Eizenberg, H. (2015). Egyptian broomrape (Phelipanche aegyptiaca) management in carrot under field conditions. Weed Technol. 29, 519–528. doi: 10.1614/WT-D-14-00140.1
Cochavi, A., Rubin, B., Achdari, G., and Eizenberg, H. (2016). Thermal time model for egyptian broomrape (Phelipanche aegyptiaca) parasitism dynamics in carrot (Daucus carota L.): Field validation. Front. Plant Sci. 7, 1–11. doi: 10.3389/fpls.2016.01807
Eizenberg, H., Hershenhorn, J., Achdari, G., and Ephrath, J. E. (2012). A thermal time model for predicting parasitism of Orobanche cumana in sunflower: five years of field validation. Field Crops Res. 137, 49–55. doi: 10.1016/j.fcr.2012.07.020
Goldwasser, Y., Miryamchik, H., Rubin, B., and Eizenberg, H. (2016). Field Dodder (Cuscuta campestris)—A new model describing temperature-dependent seed germination. Weed Sci. 64, 53–60. doi: 10.1614/WS-D-15-00050.1
Hamaoui-Laguel, L., Vautard, R., Liu, L., Solmon, F., Viovy, N., Khvorosthyanov, D., et al. (2015). Effects of climate change and seed dispersal on airborne ragweed pollen loads in Europe. Nat. Clim. Change 5, 766–771. doi: 10.1038/nclimate2652
Haseler, W. H. (1976). Parthenium hysterophorus L. in Australia. Int. J. Pest Manag. 22, 515–517. doi: 10.1080/09670877609414342
Hulme, P. E. (2016). Climate change and biological invasions: evidence, expectations, and response options. Biol. Rev. 1299, 1297–1313. doi: 10.1111/brv.12282
Javaid, A., and Adrees, H. (2009). Parthenium management by cultural filtrates of phytopathogenic fungi. Nat. Prod. Res. 23, 1541–1551. doi: 10.1080/14786410902726167
Kaur, M., Aggarwal, N. K., Kumar, V., and Dhiman, R. (2014). Effects and management of Parthenium hysterophorus: a weed of global significance. Int. Sch. Res. Not. 2014:368647. doi: 10.1155/2014/368647
Khan, N., O'Donnell, C., George, D., and Adkins, S. W. (2013). Suppressive ability of selected fodder plants on the growth of Parthenium hysterophorus. Weed Res. 53, 61–68. doi: 10.1111/j.1365-3180.2012.00953.x
Lati, R. N., Filin, S., and Eizenberg, H. (2011a). Robust methods for measurement of leaf-cover areaand biomass from image data. Weed Sci. 59, 276–284. doi: 10.1614/WS-D-10-00054.1
Lati, R. N., Filin, S., and Eizenberg, H. (2011b). Temperature- and radiation-based models for predicting spatial growth of purple nutsedge (Cyperus rotundus). Weed Sci. 59, 476–482. doi: 10.1614/WS-D-11-00007.1
Levine, J. M., Vilà, M., D'Antonio, C. M., Dukes, J. S., Grigulis, K., and Lavorel, S. (2003). Mechanisms underlying the impacts of exotic plant invasions. Proc. R. Soc. B Biol. Sci. 270, 775–781. doi: 10.1098/rspb.2003.2327
Mcmaster, G. S., and Wilhelm, W. W. (1997). Growing degree-days: one equation, two interpretations Gregory. Agric. For. Meteorol. 87, 291–300. doi: 10.1016/S0168-1923(97)00027-0
Mound, L. A. (2002). Octothrips lygodii sp. n. (Thysanoptera:Thripidae) damaging weedy Lygodium ferns in south-eastern Asia, with notes on other Thripidae reported from ferns. Aust. J. Entomol. 41, 216–220. doi: 10.1046/j.1440-6055.2002.00297.x
Navie, S. C., Panetta, F. D., McFadyen, R. E., and Adkins, S. W. (1998a). Behaviour of buried and surface-sown seeds of Parthenium hysterophorus. Weed Res. 38, 335–341. doi: 10.1046/j.1365-3180.1998.00104.x
Navie, S. C., Priest, T. E., McFadyen, R. E., and Adkins, S. W. (1998b). Efficacy of the stem-galling moth Epiblema strenuana Walk. (Lepidoptera: Tortricidae) as a biological control agent for ragweed parthenium (Parthenium hysterophorus L.). Biol. Control 13, 1–8. doi: 10.1006/bcon.1998.0638
Peters, K., Breitsameter, L., and Gerowitt, B. (2014). Impact of climate change on weeds in agriculture: a review. Agron. Sustain. Dev. 34, 707–721. doi: 10.1007/s13593-014-0245-2
Pimentel, D., Zuniga, R., and Morrison, D. (2005). Update on the environmental and economic costs associated with alien-invasive species in the United States. Ecol. Econ. 52, 273–288. doi: 10.1016/j.ecolecon.2004.10.002
QGIS Delopment Team (2018). QGIS Geographic Information System: Open Source Geospatial Foundation Project. Retrieved from: http://qgis.osgeo.org
Radosevich, S. R., Holt, J. S., and Ghersa, C. M. (2007). Ecology of Weeds and Invasive Plants: Relationship to Agriculture and Natural Resource Management, 3rd Edn. Hoboken, NJ: Wiley & Sons, Inc.
Reddy, K. N., Bryson, C. T., and Burke, I. C. (2007). Ragweed parthenium (Parthenium hysterophorus) control with preemergence and postemergence herbicides. Weed Technol. 21, 982–986. doi: 10.1614/WT-07-053.1
Rubin, B., and Matzrafi, M. (2015). “Weed management in israel-challenges and approaches,” in Weed Science in the Asian-Pacific Region, eds V. S. Rao, N. T. Yaduraju, N. R. Chandrasena, G. Hassan, and A. R. Sharma (Jabalpur: Indian Society of Weed Science), 253–270.
Setterfield, S. A., Rossiter-Rachor, N. A., Douglas, M. M., Wainger, L., Petty, A. M., Barrow, P., et al. (2013). Adding fuel to the fire: the impacts of non-native grass invasion on fire management at a regional scale. PLoS ONE 8:e59144. doi: 10.1371/journal.pone.0059144
Shabbir, A., Dhileepan, K., O'Donnell, C., and Adkins, S. W. (2013). Complementing biological control with plant suppression: implications for improved management of parthenium weed (Parthenium hysterophorus L.). Biol. Control 64, 270–275. doi: 10.1016/j.biocontrol.2012.11.014
Shimono, Y., Takiguchi, Y., and Konuma, A. (2010). Contamination of internationally traded wheat by herbicide-resistant Lolium rigidum. Weed Biol. Manag. 10, 219–228. doi: 10.1111/j.1445-6664.2010.00387.x
Singh, V. (2014). Effect of nitrogen addition on the architecture and biomass allocation of two invasive plant species (Ageratum conyzoides L. and Parthenium hysterophorus L). Proc. Nat. Acad. Sci. India. Sect. B. 81, 348–358.
Sosnoskie, L. M., Webster, T. M., and Culpepper, A. S. (2013). Glyphosate resistance does not affect palmer amaranth (Amaranthuspalmeri) seedbank longevity. Weed Sci. 61, 283–288. doi: 10.1614/WS-D-12-00111.1
Steckel, L. E., Sprague, C. L., Hager, A. G., Simmons, F. W., and Bollero, G. A. (2003). Effects of shading on common waterhemp (Amaranthus rudis) growth and development. Weed Sci. 51, 898–903. doi: 10.1614/P2002-139
Tamado, T., Ohlander, L., and Milberg, P. (2002a). Interference by the weed Parthenium hysterophorus L. with grain sorghum: influence of weed density and duration of competition. Int. J. Pest Manag. 48, 183–188. doi: 10.1080/09670870110101739
Tamado, T., Schutz, W., and Milberg, P. (2002b). Germination ecology of the weed Parthenium hysterophorus in eastern Ethiopia. Ann. Appl. Biol. 140, 263–270. doi: 10.1111/j.1744-7348.2002.tb00180.x
Williams, J. D., and Groves, R. H. (1980). The influence of temperature and photoperiod on growth and development of Parthenium hysterophorus L. Weed Res. 20, 47–52. doi: 10.1111/j.1365-3180.1980.tb00040.x
Wopfner, N., Gadermaier, G., Egger, M., Asero, R., Ebner, C., Jahn-Schmid, B., et al. (2005). The spectrum of allergens in ragweed and mugwort pollen. Int. Arch. Allergy Immunol. 138, 337–346. doi: 10.1159/000089188
Keywords: emergence, seed bank, seed germination, weed mapping, radiation levels
Citation: Matzrafi M, Raz H, Rubin B, Yaacoby T and Eizenberg H (2021) Distribution and Biology of the Invasive Weed Parthenium hysterophorus L. in Israel. Front. Agron. 3:639991. doi: 10.3389/fagro.2021.639991
Received: 10 December 2020; Accepted: 14 May 2021;
Published: 14 June 2021.
Edited by:
Buddhi Marambe, University of Peradeniya, Sri LankaReviewed by:
Asad Shabbir, The University of Sydney, AustraliaPramod Kumar Jha, Tribhuvan University, Nepal
Copyright © 2021 Matzrafi, Raz, Rubin, Yaacoby and Eizenberg. This is an open-access article distributed under the terms of the Creative Commons Attribution License (CC BY). The use, distribution or reproduction in other forums is permitted, provided the original author(s) and the copyright owner(s) are credited and that the original publication in this journal is cited, in accordance with accepted academic practice. No use, distribution or reproduction is permitted which does not comply with these terms.
*Correspondence: Maor Matzrafi, bWFvcm1Adm9sY2FuaS5hZ3JpLmdvdi5pbA==