- 1Institute for Bioengineering Research and Department of Mechanical Engineering, University of Kansas, Lawrence, KS, United States
- 2Department of Pharmaceutical Chemistry, the University of Kansas, Lawrence, KS, United States
Interventional procedures for the recanalization of blood vessels to treat deep vein thrombosis carry a high risk of vessel wall injuries or hemorrhaging. Focused ultrasound (FUS) has been used to non-invasively break down blood clots that occlude the vessels in both in vitro and in vivo studies. Previous studies have either used thrombolytic drugs or ultrasound contrast agents (e.g., microbubbles) in combination with FUS. Several studies have applied very high peak-negative-pressures (PNP) during FUS treatment to achieve successful thrombolysis without the use of contrast agents. In the current study, we demonstrated that cavitation activity could be significantly enhanced by placing a nitinol wire, whose surface was roughed by laser etching, in the focal region of a FUS field. We demonstrated in vitro in a mock thrombosis that the thrombolysis efficacy of a 500 kHz FUS transducer was significantly enhanced using a surface-etched nitinol wire as compared to an unetched nitinol wire, whereas FUS-alone at the same pressure level did not result in any thrombolysis. These results suggest that a surface modified nitinol catheter exposed to FUS can result in intense cavitation activities leading to enhanced thrombolysis without the use of additional pharmacological or contrast agents.
1 Introduction
Deep vein thrombosis (DVT), an occlusion of veins with an excessive blood clot (i.e., thrombus), is the third most common cause of cardiovascular disease (CVD) (Næss et al., 2007), with over 10 million people affected globally each year (Jha et al., 2013). Medical impairments associated with DVT include pulmonary embolism (PE) and post-thrombotic syndrome (PTS) (Augustinos and Ouriel, 2004; Di Nisio et al., 2016; Johnson et al., 1995; Kahn and Ginsberg, 2004). PE is a life-threatening complication, which is caused by detachment and transportation of thrombus debris into much smaller pulmonary blood vessels. Occlusions and loss of blood flow can result in damage to the lungs, leading to possible suffocation and death. The annual mortality due to PE in the United States in the year 2020 was over 49,000. (Farmakis et al., 2022). PTS is another common complication associated with DVT, and is often symptomatic with edema, chronic limb pain, swelling, redness of skin, and heaviness of the limb. Studies have shown that 20%–80% patients are vulnerable to PTS after the formation of distal calf DVT (Schulman et al., 1986). Overall, annual expenditures for DVT-associated complexities are estimated to be between $7 and $10 billion in the United States and as high as $69 billion globally (Grosse et al., 2016).
Existing treatment approaches for DVT include the use of anticoagulants to prevent thrombus progression; however, anticoagulants sometimes are not efficient in dissolving clots, especially in the case of chronic disease (Kearon et al., 2012). Mechanical thrombectomy (MT) is an endovascular technique useful in larger vessels, which utilizes a coil retriever, stent retriever, or an aspiration tube, to mechanically remove blood clots. Studies have demonstrated that MT can improve recanalization rates (as compared to anticoagulant and thrombolytic therapies) and produce satisfactory clinical outcomes (Berlis et al., 2004; Derex and Cho, 2017). While current MT devices can effectively remove acute blood clots, they often fail to remove chronic clots, which are hardened over time and often have a much stronger connection with the vessel wall, with an increases the risk of damaging the vessel wall, especially the venous valvular function (Kohi et al., 2016; Sharafuddin et al., 1999a).
Ultrasound-based therapies have emerged as promising thrombolytic interventions (Luo et al., 1993; Parikh et al., 2008; Tachibana and Tachibana, 1995; Ter Haar, 1999; Unger et al., 2002; Wright et al., 2012). Ultrasound based treatments fragment the blood clot non-invasively by inducing cavitation at the beam focal point targeted within the thrombus. However, treatment requires very high peak negative pressures at low frequencies (e.g., either 0.5 MHz or 1 MHz) for high efficacy (Maxwell et al., 2011). At such low frequencies, the focal spot size exceeds 10 mm, which is greater than the diameter of most veins, and can cause damage to the walls of blood vessels (Maxwell et al., 2011). Although the focal spot size can be reduced by reducing the f-numbers (Rosnitskiy et al., 2016), but this process will eventually reduce the focal depth of the FUS transducer and compromise beam propagation through deep underlying tissues. The focal spot size of FUS is smaller at higher frequencies, but the corresponding cavitation pressure thresholds will increase. To improve the safety and efficacy of ultrasound-based thrombolysis, ultrasound contrast agents (e.g., microbubbles) can be introduced (Tachibana and Tachibana, 1995; Datta et al., 2008; Feng et al., 2024; Mizushige et al., 1999). While this approach has demonstrated great success, it is not without concern. For example, higher dosages of microbubbles may cause severe tissue damage and increase the risk of emboli (Chang, 2018; Paradossi et al., 2019). In addition, the efficiency of thrombolysis is limited by the number of microbubbles that can be delivered to and retained at the site of a blood clot (Borrelli et al., 2012). Particularly, if a blood vessel is completely blocked with no blood flow, the injected agents will not be able to reach the clot. The short lifetime of the injected microbubbles also limits the temporal therapeutic window. In combination with thrombolytic drugs such as tPA (tissue plasminogen activator), rPA (recombinant tissue plasminogen activator), urokinase and tenecteplase, ultrasound-accelerated thrombolysis has been demonstrated to increase rate of lysis at reduced drug dosages through increased drug mass transport into the fibrils (Parikh et al., 2008; Blinc et al., 1993). However, complete lysis, i.e., thrombus removal rate greater than 90%, was observed only in 70% cases (Parikh et al., 2008). These observations indicate that ultrasound-accelerated thrombolysis still has a limited ability to fully resolve clots in many cases.
In this study, we present a catheter-assisted FUS technique that could potentially combine elements of MT and FUS for more efficient thrombolysis. Specifically, we surface modified nitinol wires for use with FUS; the resulting wires significantly increased cavitation and thrombolytic efficiency of FUS at low energy levels. Nitinol wires were selected because they are commonly used to make MT catheters. Physical defects (i.e., surface roughening) were produced on the surface of 0.9 mm diameter nitinol wires using a
Although catheter-assisted FUS is a cavitation bubble based approach, in comparison with other approach of using injected microbubbles, the main difference is that catheter-assisted FUS does not need the injection of external agents such as microbubbles or thrombolytic drugs during the treatment process. As a result, barriers related to the delivery and retaining of microbubbles are eliminated.
2 Materials and methods
2.1 Experimental setup
A schematic of the in vitro experimental setup has been illustrated in Figure 1. In the experimental setup, a FUS transducer of 0.5 MHz center frequency (TEQ60-0560, Siansonic Technology, Beijing, China) with a 60 mm focal length and 57.5 mm diameter was used. A function generator (33250A, Agilent Technologies, Santa Clara, CA, United States) generated sinusoidal bursts of 500 kHz. The output from the function generator was delivered to a power amplifier (2,100 L, Electronics and Innovation Ltd., Rochester, NY, United States), and then transmitted to the FUS transducer to produce the acoustic bursts. A passive cavitation detector (PCD) of 10 MHz center frequency was used to receive acoustic emissions from the focal region of the FUS transducer when needed. Prior to experiment, the PCD was made confocal to the FUS transducer so that the focal spots of the PCD and the FUS transducer overlapped on the target inside samples. The size of the FUS transducer focal zone was 3 mm × 21 mm, and the size of the PCD was 0.23 mm × 2.4 mm. As a result, the size of the confocal zone was determined by the PCD and was 0.23 mm × 2.4 mm.
During experiments, the acoustic scatterings received by the PCD were amplified using the through mode of a pulse-receiver (DPR 300, JSR Ultrasonics, Pittsford, NY, United States), and then collected by a digital oscilloscope (TBS2000B, Tektronix, Inc., Beaverton, Oregon, United States).
Tissue-mimicking agar phantoms and blood clots were used for the in-vitro studies. Samples were immersed in degassed water along with the PCD and the FUS transducer. In both the agar-based phantom and blood clot samples, nitinol wires (8320K39, Mc-MASTER, Elmhurst, IL, United States) of 0.9 mm diameter, either
Prior to the thrombolysis procedure, a sample was positioned at the confocal zone of the FUS and PCD transducers using a manual three-dimensional translational stage. Once the FUS transducer was focused on the target zone, the system was switched back to the therapy mode.
2.2 Materials
2.2.1 Tissue-mimicking agar phantom
The agar solution was prepared by stirring 2% w/v agar powder in 100 mL of distilled water, which was heated to above 85°C using a magnetic stirrer-heat plate. Then, the agar solution was poured into a mold (50.8 × 25.4 × 12.7 mm) and stored inside a refrigerator (4°C) until it solidified. Three groups of cuboid shaped agar phantom samples were created; in the first group of samples an etched catheter was inserted, and in the second group of samples an unetched catheter was inserted. The distance between the catheter and the front face (facing towards the FUS transducer) of the phantom was 25 mm. In the third group of samples, no catheter was imbedded.
2.2.2 Thrombus samples
Blood clot samples were prepared by mixing whole bovine blood (Innovative Grade US Origin Bovine Whole Blood, Innovative Research, Novi, MI, United States) with calcium chloride powder (C4901 Calcium chloride anhydrous powder, Fischer Scientific, Pittsburgh, PA, United States) inside a silicone tube of 6 mm diameter. This size was selected to match the size of deep veins in the lower extremities (Meissner, 2005). In the first step, an etched catheter was anchored at the bottom of a tube that was closed with paraffin film, and a mixture of blood and calcium chloride was poured inside the silicone tube. Similarly, samples with an unetched catheter were also prepared. Samples in the control group contained no catheter, and the mixture of blood and calcium chloride was poured directly into the silicone tube. For each group five different samples were prepared prior to the experiment. All blood clot samples were placed inside an incubator and were heated to a temperature of 37
2.2.3 Surface modification and characterization of the catheter wires
Nitinol wires (8320K39, Mc-MASTER, Elmhurst, IL, United States) of 0.9-mm diameter were used in the current study. Surface roughening of the nitinol catheter wire surface was performed by etching the wire surface under a CO2 laser beam (Epilog Fusion Edge
Scanning electron microscopy (SEM) analysis was used to evaluate the surface texture of the etched nitinol wires (Phenom ProX Desktop SEM, ThermoFisher Scientific, Waltham, MA, United States) with an accelerating voltage of 5 kV. Atomic force microscopy (AFM) was used to quantify surface roughness and obtain topography images for both etched and unetched wires (Multimode V Atomic Force Microscope, Veeco Instruments Inc., Plainview, NY, United States) at a scan rate of 1 Hz and scan size of 5
2.3 Cavitation threshold detection
Cavitation thresholds with and without catheter wires were measured first in agar phantoms to demonstrate the feasibility of the technique. The FUS transducer was focused on the metallic surface of the catheter with ultrasound bursts each containing 2,500 cycles at a central frequency of 500 kHz. The applied peak negative pressure (PNP) was increased from 0.1 MPa to 5.1 MPa at a step-size of 0.12 MPa to determine the threshold. For repeated detections, a waiting period of at least 10-s was used between ultrasound bursts to avoid any acoustic interference or thermal effects from a previous burst. The acoustic broadband emissions from the samples were collected by the PCD with the oscilloscope in peak detection mode. Similar data collection methods were repeated for agar phantoms without catheters. After the peak-to-peak values were collected, statistical computations including mean and standard deviations were performed on each group data.
The PNP calibration for the FUS transducer was carried out by using a needle hydrophone (HNR-0500 Series, ONDA Corporation, Sunnyvale, United States). During this process, the need hydrophone was placed at the focus of the FUS transducer. The FUS transducer was used to send 10-cycle bursts to the needle hydrophone while the FUS transducer was moved translationally in parallel and perpendicular directions to the experimental set-up table by using a 3D translation stage. The received signal by the needle hydrophone was recorded by a digital oscilloscope, and the voltage reading was converted to pressure based on the calibration data of the needle hydrophone.
2.4 Treatment
Treatments were carried out on blood clot samples with 2500-cycle ultrasound bursts at a 500 kHz center frequency and a 20 Hz burst repetition rate (a burst period of 50 ms), resulting in a 10% duty cycle. This duty cycle was selected to mitigate potential thermal effects of FUS. The PNP value for thrombolysis was determined by performing an optimization process, wherein the PNP value was increased within the same range as that applied to the agar samples during cavitation threshold detection. Samples then were visually inspected to determine whether blood clot material was removed during catheter-assisted FUS thrombolysis. The optimal PNP value was the minimum value needed to induce the thrombus removal. Treatment time was set to 2 min for each group.
Before each experiment, the initial weight of the blood clot sample was measured with a laboratory balance (Melter-Toledo xs205 Dual Range, Fischer Scientific, Waltham, MA, United States). Then the sample, with catheter wire (etched or unetched) or without catheter wire, was placed in the focal region of the FUS transducer in a water tank filled with degassed water. The treatment was then performed using the predetermined ultrasound parameters. The initial weight was subtracted from the final weight to quantify the amount of blood clot removal.
2.5 Thrombus removal quantification method and statistical analyses
The entire experiment was divided into 3 groups: FUS-only, FUS plus unetched wire (FUS-unetched wire), and FUS plus etched wire (FUS-etched wire) groups. All blood clots were prepared with the same amount of blood, so they had approximately the same initial weights. The difference between the initial weight and the final weight of each sample was used to compare the treatment outcomes between the three groups. To obtain statistical results for each group of data, boxplots with overlaid individual data points were plotted using MATLAB R2022b (MathWorks, Natick, MA, United States). Paired t-test analysis was performed to compute the statistical significance between groups, and each set of experiments was performed under identical conditions.
3 Results
3.1 Surface characterization of nitinol wires
SEM images of the surfaces of nitinol wires are shown in Figure 2. The exposure of nitinol wires to the CO2 laser engraver beam generated clearly uneven surfaces in the form of bulges on the treated wire surfaces, shown in Figures 2A, C, as compared to matching untreated wires, shown in Figures 2B, D.
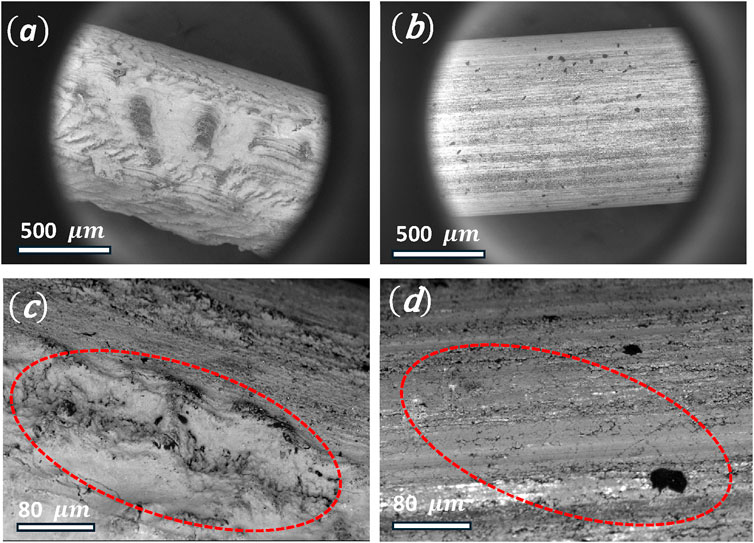
Figure 2. (A) SEM image of a CO2-laser etched nitinol wire, (B) unetched nitinol wire, (C) SEM image of an etched nitinol wire (red dotted circle indicates bulges), (D) unetched nitinol wire. Diameter of the Nitinol wire is 0.9 mm.
Surface roughness was quantified on the nitinol wire samples based on three-dimensional (3D) topography AFM images, which showed elevated terrains on the surface of etched wire, as shown in Figure 3A. In contrast, few elevated terrains were observed on the surface of the unetched wires, as shown in Figure 3B. Average surface roughness of the etched and unetched wires was 0.12
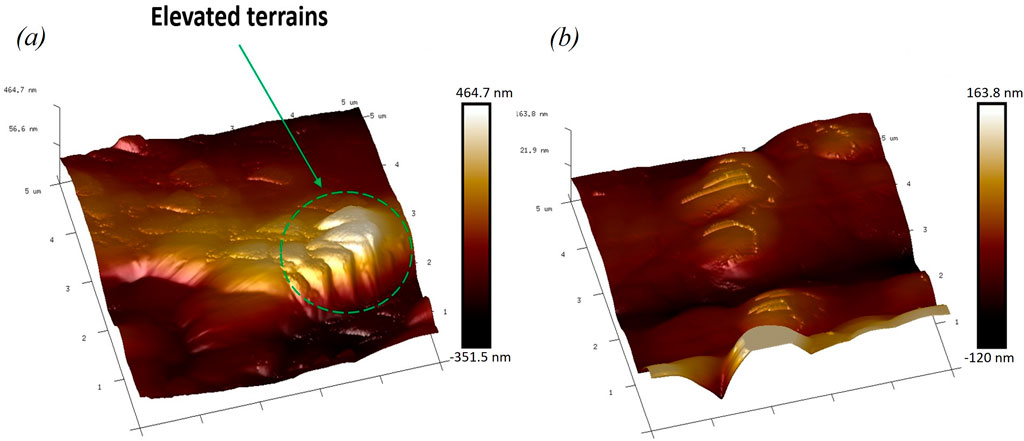
Figure 3. (A) Atomic force microscopy (AFM) image of the etched nitinol wire showing elevated terrains. (B) Image of the unetched Nitinol wire showing less elevated terrains.
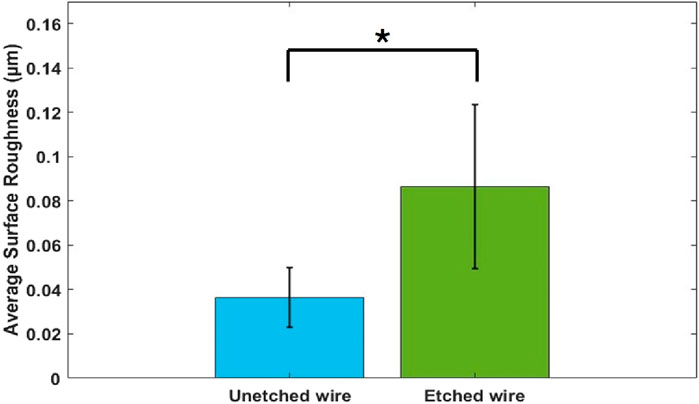
Figure 4. Quantification of average surface roughness from unetched and etched nitinol wires using AFM at a scan rate of 1 MHz and scan size of 5
3.2 Cavitation threshold measurement
The PCD was used to measure the peak values of samples’ passive cavitation signals. These values were plotted against the applied FUS peak negative pressure to determine the cavitation threshold. For the agar phantom with an unetched wire (FUS-unetched), PCD peak detection showed an inflection starting at a PNP of 1.8 MPa where significant jumps (more than twice of the expected value based on a linear profile) in the peak amplitude of received acoustic signals were measured. This inflection was used as an indicator for the initiation of cavitation, and this pressure point was defined as the cavitation threshold. In contrast, with an etched wire inside the agar phantom (FUS-etched), cavitation was initiated at a PNP of 0.8 MPa. In the agar phantoms without a wire (FUS only), no cavitation was detected at PNP less than about 4.5 MPa (Figure 5). These results demonstrated that laser etching the surface of the nitinol wire (i.e., surface roughening) can significantly reduce the FUS PNP required for the onset of inertial cavitation, indicating an enhanced cavitation effect. The typical signals detected by PCD at 1.8 MPa are shown in the complementary Figure 1.
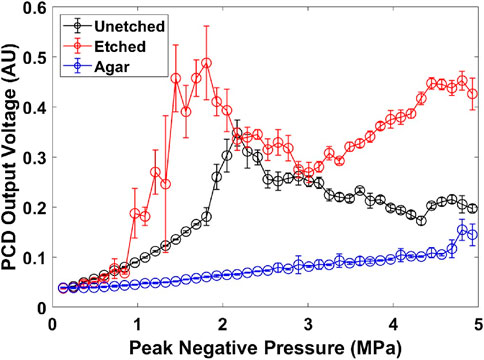
Figure 5. Cavitation threshold detection curves for agar phantoms with 0.9 mm diameter Nitinol wire. Error bars represent the standard deviations from 10 measurements. (au) stands for arbitrary unit.
The detected PCD signal decreased and fluctuated when the applied FUS pressure was above cavitation threshold. This is possibly because bubbles have formed in the pre-focal zone of the PCD and signals from the focal region of the PCD were shielded. This phenomenon has been reported in the early studies of high intensity focused ultrasound (Holt and Roy, 2001).
3.3 Treatment of blood clot samples
Based on the agar phantom study shown in Figure 5, a FUS PNP of 1.8 MPa was selected for the blood clot thrombolysis study. Figure 6 shows photographs of the blood clot samples treated at 1.8 MPa PNP and with or without nitinol wires. When treated with FUS-only, there was no thrombus removal and no visible clot debris. (Supplementary Video S3). However, when treated with FUS combined with an unetched nitinol wire, blood clot debris appeared with a light smoke-type appearance (Supplementary Video S2). In comparison, when clots were treated with FUS combined with an etched nitinol wire, clot debris particles were generated with an appearance resembling dense smoke (Supplementary Video S1). Treating blood clot samples with FUS-only resulted in negligible blood clot removal after treatment, Figure 6B. Treatment with FUS and an unetched wire resulted in partial or less removal of blood clot, Figure 6D, and treatment with FUS and an etched wire resulted in complete or maximum removal of the thrombus, Figure 6F.
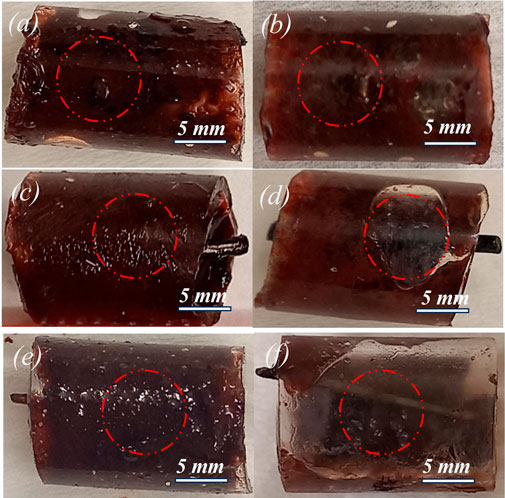
Figure 6. Photographs of thrombus samples treated with 0.5-MHz FUS bursts for 2 min at 1.8 MPa PNP. (A) before FUS-only treatment, (B) after FUS-only treatment (no removal of clot), (C) before FUS-unetched wire treatment, (D) after FUS-unetched wire treatment (partial removal of clot), (E) before FUS-etched wire treatment (F) after FUS-etched wire treatment (maximum removal of clot).
Figure 7 shows the quantification of post-treatment for blood clot samples with and without wires using 1.8 MPa PNP with 2,500 cycles, 20 Hz repetition rate, 10% duty cycle for 2 min treatment time. The FUS-only group showed negligible reduction in thrombus weight with a mean value of −3.76 mg. The FUS-unetched wire group showed an improvement in the thrombus weight reduction with a mean value of −68.99 mg. The FUS-etched wire group showed the largest reduction in clot weight with a mean value of −178.56 mg. The resulting differences between each pair of groups were statistically significant; p < 0.01 for (FUS-only, FUS-unetched wire) and (FUS-unetched wire, FUS-etched wire) respectively. The last pair (FUS-only, FUS-etched wire) showed the high level of significance (p < 0.001).
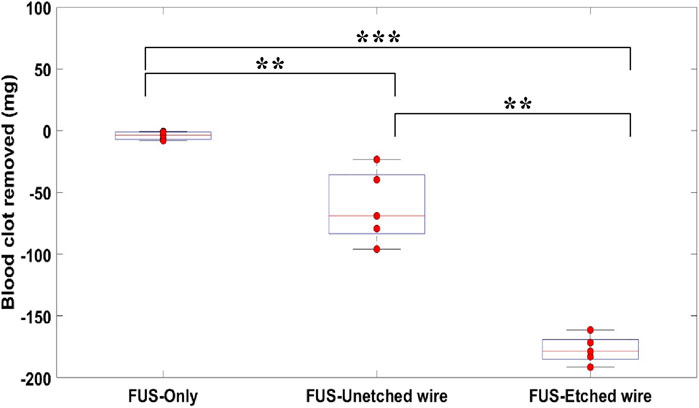
Figure 7. Quantification of thrombus removed from blood clot samples treated with 0.5-MHz FUS bursts for 2 min at 1.8 MPa PNP using FUS-only, FUS-unetched wire, FUS-etched wire. Each FUS burst contained 2,500 cycles at 10% duty cycle with a burst period of 50 ms. “***”: p < 0.001, “**”: p < 0.01, n=5.
4 Discussion
Current study presented a new engineering approach to enhance cavitation in the FUS field without the injection of microbubbles. This approach has not been explored previously. The results from this study demonstrate introduction of a wire into the field of FUS induces more effective fragmentation of blood clots as compared to FUS alone. Furthermore, applying a surface-roughening laser treatment to the nitinol wire further increases the efficiency of FUS at lower energy levels.
A potential mechanism explaining the improved thrombolysis with FUS-etched wire therapy is the initiation of multiple enhanced inertial cavitation bubble spots at the roughened wire surface. Laser etching was performed on the surface of nitinol wire to develop a rough surface profile that acted as nucleation sites for inertial cavitation. Surface roughness apart from the host liquid has a significant role in the induction of inertial cavitation bubbles (Ghorbani et al., 2018). The laser-etching procedure resulted in in bulges on the wire surface. The space between bulges can contain air-pockets, which when immersed in water, trap bubbles. When exposed to an FUS beam, an intense cavitation cloud can develop at the interface between the etched wire and thrombus tissue. In addition, cavitation enhancement may be further achieved due to the metal wire surface that acts as a hard boundary, which reflects all the incoming waves. As a result, the incident wave superposes on the reflected wave, and the pressure near the interface between metal and thrombus soft tissue can double in certain regions, i.e. 1.8 MPa PNP will become 3.6 MPa PNP near the wire-thrombus interface. This increased PNP will further enhance cavitation activity at low applied PNP energies (Samaddar et al., 2024). In the case of FUS treatment with an unetched (smooth) wire, only the pressure doubling effect, resulting from the hard boundary, takes place. The lack of surface roughening results in fewer bubble nucleation spots and less total inertia cavitation. Due to this lack of nucleation sites, unetched wire-assisted FUS therapy was less effective at depleting thrombus tissues than etched wire-assisted FUS therapy.
The synergistic effect between intense bubble generation and hard-boundary pressure doubling can result in the onset of early cavitation, which leads to a relatively low inertial cavitation threshold of 0.8 MPa PNP. When the FUS-etched wire treatment was performed at a 1.8-MPa PNP, i.e., over 200% of the cavitation threshold pressure, strong collapse of cavitation bubbles can be induced, leading to effective fragmentation of the clot (Supplementary Video S1) and thus, enhanced thrombolysis as seen in Figure 6F. In contrast, FUS exposure with the unetched wire demonstrated a delayed onset of cavitation at 1.8 MPa PNP, resulting in less fragmentation (Supplementary Video S2), and an incomplete or partial removal of the clot from blood vessels as seen in Figure 6D.
The nitinol wire used in the study was 0.9 mm in diameter. The wire was inserted into a silicone tube of 6-mm diameter, mimicking a deep vein inside body. In many clinical situations, insertion of a catheter of a size equivalent to 1.3–2 mm is safe inside blood vessel lumens, with minimum risks of injuring tissues in proximity to the vessel wall (Hamel, 2009). Cavitation activity will be affected by the size of catheter wires, and this will be investigated in the future. This phenomenon may have a significant clinical importance, since the combination of a surface roughened catheter wire with FUS results in enhanced thrombolytic cavitation at relatively low PNP, which could minimize the risk of damage to surrounding tissues. In contrast to injected microbubble contrast agents, the technique described here results in more precise control of the cavitation zone, as contrast agents may not distribute evenly within the clot or fail to penetrate complete clots, which can cause spatially distributed cavitation events within the FUS field (Heo et al., 2023).
The mechanical forces induced by cavitation only damages a thin layer of tissue that is in direct contact with it, and potentially can remove a layer much less than 100 µm in thickness (Vogel and Venugopalan, 2003; Lamminen et al., 2004) Many conventional MT procedures exert force on the inner wall of blood vessel to scrape thrombus off. However, such interventions can potentially damage the venous valvular operations, such as the prevention of blood back flow (Kohi et al., 2016; Sharafuddin et al., 1999b). The technique we have described has the potential to minimize venous valve damage as compared to conventional MT.
In comparison with thrombolysis techniques based on the injection of microbubbles, the current catheter-assisted FUS does not need the injection of microbubbles. Instead, bubbles can be produced in situ at the blood clot location. In practice, this approach may be implemented the same way as microbubble-based approach but without the injection of microbubbles. Thus, we do not expect catheter-assisted FUS will be more costly and complex than thrombolysis techniques based on the injection of microbubbles. In fact, the technique may have a relatively low barrier for future clinic translation. Endovascular approaches utilizing MT and catheter-directed thrombolysis are standards in the treatment of blood clots. In both approaches, a catheter has already been used. Our technology will only require the addition of a non-invasive FUS system. As a result, catheter-assisted FUS could serve as an adjunctive therapy to MT and catheter-directed thrombolysis when the catheter-based approaches cannot successfully remove blood clots. While the current study demonstrated the feasibility of catheter-assisted FUS for thrombolysis, its efficiency and safety need to be further evaluated in animal studies and clinical trials, particularly in the situation where MT and catheter-directed thrombolysis cannot successfully remove blood clots.
Ultrasound parameters in the current study were selected based on our previous investigations (Singh and Yang, 2023; Hu et al., 2017; Cui and Yang, 2011) so that strong mechanical effect induced by cavitation could be produced without significant thermal effect. While localized heating induced by the applied FUS is possible, we do not expect the heating effect will be significant to cause any thermal-related damage due to the use of short ultrasound bursts (5 ms) and relatively low ultrasound frequency (500 kHz). To induce significant local heating, the applied acoustic pressure has to be much higher than 5.1 MPa that was the maximum pressure used in the phantom study, as demonstrated in boiling histotripsy study (Khokhlova et al., 2023). The laser etching process was selected to produce 100 µm defects on the wire surface. This size of defect is the largest that can be produced by our device. We have tried smaller defects, but the resulting cavitation activity was relatively weak. However, all these parameters are by no means optimized. While the systematic optimization is beyond the scope of the current feasibility study, future investigation should focus on the relation between defect size and ultrasound parameters. In addition, surface defects with various shapes and patterns may also impact cavitation activity, which are worth further investigation.
This technique, however, is not without limitations. The relative location between a catheter-wire and thrombus is limited. In real world clinical applications, when the catheter-wire is within the lumen of a blood vessel, the direction of the incident FUS wave needs to be adjusted so that FUS beam focal spot overlaps at the wire-thrombus interface. One limitation of this study is that all experiments were performed in degassed water and not in real blood vessels. However, the uniaxial extensional viscosity of blood can attenuate cavitation likelihood (Brujan, 2019). Although we observed that blood clots were fragmented into very small debris, as observed with both the unetched and etched wires (Supplementary Videos S1, S2, respectively), it is important to measure the clot debris particle size in future studies. Debris particle size could be measured by particle size optometry, and if necessary, filters or aspiration tubes can be installed on the catheter to remove large debris.
We expect this technology can be further improved when it is guided by real-time imaging. Imaging technologies such CT angiography and ultrasound imaging are routinely used in the clinic and would be compatible with the thrombolytic technique. In addition, other surface modification approaches, such as surface coating treatments and optimized etching patterns, should be explored to further enhance cavitation. This technique should also be tested at higher FUS frequences, which have a smaller beam focal point and could further improve the precision of this technique in delicate tissue structures, without requiring the larger PNP energies generally required with higher frequencies.
In conclusion, this study demonstrates a technology to enhance cavitation with a surface-etched wire, which when exposed to FUS can initiate cavitation activities at a low FUS pressure. In vitro FUS experiments performed at the same PNP demonstrated complete breakdown of blood clots using an etched wire, partial breakdown of blood clots using an unetched wire, and negligible breakdown of blood clots using only FUS.
Data availability statement
The raw data supporting the conclusions of this article will be made available by the authors, without undue reservation.
Ethics statement
Ethical approval was not required for the studies on animals in accordance with the local legislation and institutional requirements because only commercially available established cell lines were used.
Author contributions
AS: Conceptualization, Data curation, Formal Analysis, Investigation, Methodology, Software, Writing–original draft, Writing–review and editing, Visualization, Validation. MF: Writing–review and editing. XY: Conceptualization, Formal Analysis, Investigation, Project administration, Resources, Supervision, Validation, Visualization, Writing–review and editing.
Funding
The author(s) declare that no financial support was received for the research, authorship, and/or publication of this article.
Conflict of interest
The authors declare that they have filed a patent application pertinent to this technology.
Publisher’s note
All claims expressed in this article are solely those of the authors and do not necessarily represent those of their affiliated organizations, or those of the publisher, the editors and the reviewers. Any product that may be evaluated in this article, or claim that may be made by its manufacturer, is not guaranteed or endorsed by the publisher.
Supplementary material
The Supplementary Material for this article can be found online at: https://www.frontiersin.org/articles/10.3389/facou.2024.1456606/full#supplementary-material
References
Augustinos, P., and Ouriel, K. (2004). Invasive approaches to treatment of venous thromboembolism. Circulation 110 (9_Suppl. l_1), I-27-I–I34. doi:10.1161/01.cir.0000140900.64198.f4
Berlis, A., Lutsep, H., Barnwell, S., Norbash, A., Wechsler, L., Jungreis, C. A., et al. (2004). Mechanical thrombolysis in acute ischemic stroke with endovascular photoacoustic recanalization. Stroke 35 (5), 1112–1116. doi:10.1161/01.str.0000124126.17508.d3
Blinc, A., Francis, C. W., Trudnowski, J. L., and Carstensen, E. L. (1993). Characterization of ultrasound-potentiated fibrinolysis in vitro. 81, 2636–2643. doi:10.1182/blood.v81.10.2636
Borrelli, M. J., O’Brien, W. D., Hamilton, E., Oelze, M. L., Wu, J., Bernock, L. J., et al. (2012). Influences of microbubble diameter and ultrasonic parameters on in vitro sonothrombolysis efficacy. J. Vasc. Interventional Radiology 23 (12), 1677–1684.e1. doi:10.1016/j.jvir.2012.08.019
Brujan, E.-A. (2019). Shock wave emission and cavitation bubble dynamics by femtosecond optical breakdown in polymer solutions. Ultrason. Sonochemistry 58, 104694. doi:10.1016/j.ultsonch.2019.104694
Chang, E. H. (2018). An introduction to contrast-enhanced ultrasound for nephrologists. Nephron 138 (3), 176–185. doi:10.1159/000484635
Cui, H., and Yang, X. (2011). Enhanced-heating effect during photoacoustic imaging-guided high-intensity focused ultrasound. Appl. Phys. Lett. 99 (23), 231113. doi:10.1063/1.3669441
Datta, S., Coussios, C.-C., Ammi, A. Y., Mast, T. D., de Courten-Myers, G. M., and Holland, C. K. (2008). Ultrasound-enhanced thrombolysis using Definity® as a cavitation nucleation agent. Ultrasound Med. and Biol. 34 (9), 1421–1433. doi:10.1016/j.ultrasmedbio.2008.01.016
Derex, L., and Cho, T.-H. (2017). Mechanical thrombectomy in acute ischemic stroke. Rev. Neurol. 173 (3), 106–113. doi:10.1016/j.neurol.2016.06.008
Di Nisio, M., van Es, N., and Büller, H. R. (2016). Deep vein thrombosis and pulmonary embolism. Lancet 388 (10063), 3060–3073. doi:10.1016/s0140-6736(16)30514-1
Farmakis, I. T., Valerio, L., Bikdeli, B., Connors, J. M., Giannakoulas, G., Goldhaber, S. Z., et al. (2022). Annual mortality related to pulmonary embolism in the US before and during the COVID-19 pandemic. J. Am. Coll. Cardiol. 80 (16), 1579–1581. doi:10.1016/j.jacc.2022.08.721
Feng, S., Wang, S., Tang, J., and Zhu, X. (2024). Ultrasound combined with continuous microbubble injection to enhance catheter-directed thrombolysis in vitro and in vivo. J. Ultrasound Med. 43 (4), 741–749. doi:10.1002/jum.16400
Ghorbani, M., Sadaghiani, A. K., Villanueva, L. G., and Koşar, A. (2018). Hydrodynamic cavitation in microfluidic devices with roughened surfaces. J. Micromechanics Microengineering 28 (7), 075016. doi:10.1088/1361-6439/aab9d0
Grosse, S. D., Nelson, R. E., Nyarko, K. A., Richardson, L. C., and Raskob, G. E. (2016). The economic burden of incident venous thromboembolism in the United States: a review of estimated attributable healthcare costs. Thrombosis Res. 137, 3–10. doi:10.1016/j.thromres.2015.11.033
Hamel, W. J. (2009). Femoral artery closure after cardiac catheterization. Crit. care nurse 29 (1), 39–46. doi:10.4037/ccn2009157
Heo, J., Park, J. H., Kim, H. J., Pahk, K., and Pahk, K. J. (2023). Sonothrombolysis with an acoustic net-assisted boiling histotripsy: a proof-of-concept study. Ultrason. Sonochemistry 96, 106435. doi:10.1016/j.ultsonch.2023.106435
Holt, R. G., and Roy, R. A. (2001). Measurements of bubble-enhanced heating from focused, MHz-frequency ultrasound in a tissue-mimicking material. Ultrasound Med. Biol. 27 (10), 1399–1412. doi:10.1016/s0301-5629(01)00438-0
Hu, Z., Zhang, H., Mordovanakis, A., Paulus, Y. M., Liu, Q., Wang, X., et al. (2017). High-precision, non-invasive anti-microvascular approach via concurrent ultrasound and laser irradiation. Sci. Rep. 7, 40243. doi:10.1038/srep40243
Jha, A. K., Larizgoitia, I., Audera-Lopez, C., Prasopa-Plaizier, N., Waters, H., and Bates, D. W. (2013). The global burden of unsafe medical care: analytic modelling of observational studies. BMJ Qual. and Saf. 22 (10), 809–815. doi:10.1136/bmjqs-2012-001748
Johnson, B. F., Manzo, R. A., Bergelin, R. O., and Strandness, D. E. (1995). Relationship between changes in the deep venous system and the development of the postthrombotic syndrome after an acute episode of lower limb deep vein thrombosis: a one-to six-year follow-up. J. Vasc. Surg. 21 (2), 307–313. doi:10.1016/s0741-5214(95)70271-7
Kahn, S. R., and Ginsberg, J. S. (2004). Relationship between deep venous thrombosis and the postthrombotic syndrome. Archives Intern. Med. 164 (1), 17–26. doi:10.1001/archinte.164.1.17
Kearon, C., Akl, E. A., Comerota, A. J., Prandoni, P., Bounameaux, H., Goldhaber, S. Z., et al. (2012). Antithrombotic therapy for VTE disease: antithrombotic therapy and prevention of thrombosis: American College of Chest Physicians evidence-based clinical practice guidelines. Chest 141 (2), e419S–e496S. doi:10.1378/chest.11-2301
Khokhlova, V. A., Rosnitskiy, P. B., Tsysar, S. A., Buravkov, S. V., Ponomarchuk, E. M., Sapozhnikov, O. A., et al. (2023). Initial assessment of boiling histotripsy for mechanical ablation of ex vivo human prostate tissue. Ultrasound Med. Biol. 49 (1), 62–71. doi:10.1016/j.ultrasmedbio.2022.07.014
Kohi, M. P., Kohlbrenner, R., Kolli, K. P., Lehrman, E., Taylor, A. G., and Fidelman, N. (2016). Catheter directed interventions for acute deep vein thrombosis. Cardiovasc. diagnosis Ther. 6 (6), 599–611. doi:10.21037/cdt.2016.11.20
Lamminen, M. O., Walker, H. W., and Weavers, L. K. (2004). Mechanisms and factors influencing the ultrasonic cleaning of particle-fouled ceramic membranes. J. Membr. Sci. 237 (1-2), 213–223. doi:10.1016/s0376-7388(04)00197-8
Luo, H., Steffen, W., Cercek, B., Arunasalam, S., Maurer, G., and Siegel, R. J. (1993). Enhancement of thrombolysis by external ultrasound. Am. Heart J. 125 (6), 1564–1569. doi:10.1016/0002-8703(93)90741-q
Maxwell, A. D., Owens, G., Gurm, H. S., Ives, K., Myers, D. D., and Xu, Z. (2011). Noninvasive treatment of deep venous thrombosis using pulsed ultrasound cavitation therapy (histotripsy) in a porcine model. J. Vasc. interventional radiology 22 (3), 369–377. doi:10.1016/j.jvir.2010.10.007
Meissner, M. H. (2005). “Lower extremity venous anatomy,” in Seminars in interventional radiology. Copyright© 2005 (New: Thieme Medical Publishers, Inc.), 333. Seventh Avenue.
Mizushige, K., Kondo, I., Ohmori, K., Hirao, K., and Matsuo, H. (1999). Enhancement of ultrasound-accelerated thrombolysis by echo contrast agents: dependence on microbubble structure. Ultrasound Med. and Biol. 25 (9), 1431–1437. doi:10.1016/s0301-5629(99)00095-2
Næss, I. A., Christiansen, S. C., Romundstad, P., Cannegieter, S. C., Rosendaal, F. R., and Hammerstrøm, J. (2007). Incidence and mortality of venous thrombosis: a population-based study. J. thrombosis haemostasis 5 (4), 692–699. doi:10.1111/j.1538-7836.2007.02450.x
Paradossi, G., Oddo, L., Cerroni, B., Ben-Harush, C., Ariel, E., Di Meco, F., et al. (2019). In vivo toxicity study of engineered lipid microbubbles in rodents. ACS omega 4 (3), 5526–5533. doi:10.1021/acsomega.8b03161
Parikh, S., Motarjeme, A., McNamara, T., Raabe, R., Hagspiel, K., Benenati, J. F., et al. (2008). Ultrasound-accelerated thrombolysis for the treatment of deep vein thrombosis: initial clinical experience. J. Vasc. Interventional Radiology 19 (4), 521–528. doi:10.1016/j.jvir.2007.11.023
Rosnitskiy, P. B., Yuldashev, P. V., Sapozhnikov, O. A., Maxwell, A. D., Kreider, W., Bailey, M. R., et al. (2016). Design of HIFU transducers for generating specified nonlinear ultrasound fields. IEEE Trans. ultrasonics, Ferroelectr. Freq. control 64 (2), 374–390. doi:10.1109/tuffc.2016.2619913
Samaddar, A., Singh, R., Yang, X., Ebersole, K. C., and Forrest, M. L. (2024). Investigating the potential of catheter-assisted pulsed focused ultrasound ablation for atherosclerotic plaques. Med. Phys. 51 (8), 5181–5189. doi:10.1002/mp.17253
Schulman, S., Granqvtst, S. A., Juhlin-Dannfelt, A., and Lockner, D. (1986). Long-term sequelae of calf vein thrombosis treated with heparin or low-dose streptokinase. Acta Medica Scand. 219 (4), 349–357. doi:10.1111/j.0954-6820.1986.tb03323.x
Sharafuddin, M. J., Gu, X., Han, Y. M., Urness, M., Gunther, R., and Amplatz, K. (1999a). Injury potential to venous valves from the Amplatz thrombectomy device. J. Vasc. Interv. Radiol. 10 (1), 64–69. doi:10.1016/s1051-0443(99)70013-8
Sharafuddin, M. J., Gu, X., Han, Y.-M., Urness, M., Gunther, R., and Amplatz, K. (1999b). Injury potential to venous valves from the Amplatz thrombectomy device. J. Vasc. interventional radiology 10 (1), 64–69. doi:10.1016/s1051-0443(99)70013-8
Singh, R., and Yang, X. (2023). A review on photo-mediated ultrasound therapy. Exp. Biol. Med. 248 (9), 775–786. doi:10.1177/15353702231181191
Tachibana, K., and Tachibana, S. (1995). Albumin microbubble echo-contrast material as an enhancer for ultrasound accelerated thrombolysis. Circulation 92 (5), 1148–1150. doi:10.1161/01.cir.92.5.1148
Ter Haar, G. (1999). Therapeutic ultrasound. Eur. J. ultrasound 9 (1), 3–9. doi:10.1016/s0929-8266(99)00013-0
Unger, E. C., Matsunaga, T. O., McCreery, T., Schumann, P., Sweitzer, R., and Quigley, R. (2002). Therapeutic applications of microbubbles. Eur. J. Radiology 42 (2), 160–168. doi:10.1016/s0720-048x(01)00455-7
Vogel, A., and Venugopalan, V. (2003). Mechanisms of pulsed laser ablation of biological tissues. Chem. Rev. 103 (2), 577–644. doi:10.1021/cr010379n
Wright, C., Hynynen, K., and Goertz, D. (2012). In vitro and in vivo high-intensity focused ultrasound thrombolysis. Investig. Radiol. 47 (4), 217–225. doi:10.1097/rli.0b013e31823cc75c
Keywords: thrombolysis, focused ultrasound, cavitation, rough surface, catheter
Citation: Samaddar A, Forrest ML and Yang X (2024) Pulsed focused ultrasound ablation assisted by a surface modified catheter for thrombolysis: a feasibility study. Front. Acoust. 2:1456606. doi: 10.3389/facou.2024.1456606
Received: 28 June 2024; Accepted: 04 September 2024;
Published: 18 September 2024.
Edited by:
Yun Jing, The Pennsylvania State University (PSU), United StatesReviewed by:
John Sharer Allen, University of Hawaii at Manoa, United StatesZhongtao Hu, Beihang University, China
Copyright © 2024 Samaddar, Forrest and Yang. This is an open-access article distributed under the terms of the Creative Commons Attribution License (CC BY). The use, distribution or reproduction in other forums is permitted, provided the original author(s) and the copyright owner(s) are credited and that the original publication in this journal is cited, in accordance with accepted academic practice. No use, distribution or reproduction is permitted which does not comply with these terms.
*Correspondence: Xinmai Yang, eG15YW5nQGt1LmVkdQ==