- 1School of Natural Sciences, University of Tasmania, Hobart, TAS, Australia
- 2Genecology Research Centre, School of Science and Engineering, University of Sunshine Coast, Sippy Downs, QLD, Australia
- 3Endeavour Veterinary Ecology Pty Ltd., Toorbul, QLD, Australia
Background: Chlamydial disease is a major factor negatively affecting koala populations. Vaccination is a promising management option that would result in immune-mediated protection against disease. Measuring and assessing vaccine efficacy can be challenging owing to both direct and indirect interactions caused by vaccination. In this study, we investigate vaccine-immune-chlamydial load-disease relationships from MOMP (major outer membrane protein) vaccine trials to protect healthy free-ranging koalas against Chlamydia-related diseases.
Methods: We created a priori hypotheses based on data sources and perceived direct and indirect interactions from koalas vaccinated 6 months prior. Each hypothesis was tested as a structural equation model separately for either the urogenital or the ocular site to evaluate possible causality among measured variables. Model averaging was used as multiple models fit the data, and the strength of relationships was examined through averaged coefficients and the raw data.
Results: We found more relationships in urogenital models as compared to ocular models, particularly those with interleukin 17 (IL17) mRNA expression compared to models with interferon gamma (IFNγ) expression. In the averaged model with IL17, urogenital chlamydial load was positively associated with disease and negatively associated with IL17 expression. MOMP vaccination had a trending effect for reducing urogenital chlamydial load and also had a strong effect on increasing IL17 expression. Not surprisingly, urogenital chlamydial load was a positive predictor for the development of urogenital disease at 6 months post-vaccination.
Conclusions: Despite multiple potential sources of variation owing to the koalas in this study being free-ranging, our analyses provide unique insights into the effects of vaccinating against Chlamydia. Using structural equation modeling, this study has helped illuminate that the expression of the immune cytokine IL17 is linked to MOMP vaccination, and animals with a high urogenital chlamydial load expressed less IL17 and were more likely to develop disease, enhancing previous investigations. Going beyond univariate statistics, the methods used in this study can be applied to other preclinical vaccination experiments to identify important direct and indirect factors underpinning the effects of a vaccine.
Introduction
Koala (Phascolarctos cinereus) populations, particularly in the Australian Capital Territory and in the Australian states of New South Wales and Queensland, have suffered staggering losses over recent years (1–4) leading to their conservation status being listed as “vulnerable” per the IUCN (5). A number of factors negatively affect koala populations including: habitat loss (1, 6), climate change (7), bushfires (8), motor vehicle accidents (6), dog attacks (8), and disease (9). Deterministic age structured matrix models (9) of four of these factors (habitat loss, dog attacks, motor vehicle collisions, and disease) indicate that reducing the prevalence of disease may stabilize koala populations. The magnitude of disease-related mortality within a given population is potentially exacerbated by environmental stressors including climate change, habitat loss resulting from urbanization, and environmental disasters such as bushfires, though to our knowledge no study has investigated this in wild koala populations (10). Amid its variable magnitude, the reduction of disease has been a key management strategy of this species (2, 9, 11, 12).
Chlamydia is an obligate, intracellular bacterium that is the most prevalent disease-causing pathogen in wild koalas (12, 13). Koalas are hosts to two bacterial species of Chlamydia (C. pecorum and C. pneumoniae), however, modern vaccine candidates target the more prevalent species, C. pecorum, that typically infects epithelial cells in the ocular and urogenital mucosa (14). Sterility and disease-related mortality as a result of chlamydial infections have a direct, negative impact on koala population dynamics and are relatively common among some free-ranging koala populations (11, 15). Chlamydial infections in koalas are treatable with antibiotics, but this management strategy is potentially fatal for the specialized microflora in the koala gastrointestinal tract that is necessary for eucalyptus digestion (16). Vaccinating koalas is a promising tool for disease management that is modeled to have clear benefits, particularly at the population scale. Modeling by Craig et al. (17) suggested that chlamydial vaccination could stabilize koala populations after 5 years of using a vaccine (with protective efficacy of 75%) administered to around 10% of koalas per year. Such a vaccine does not yet exist, however, many studies within the last decade have advanced the development of a vaccine for koalas against Chlamydia.
Much of the foundational work for chlamydial vaccine development has used captive koalas under controlled veterinary conditions [reviewed by Phillips et al. (18)]. However, field trials that encompass a greater range of natural variables provide a more accurate picture of vaccine efficacy. To date, there are two published field trials testing a chlamydial vaccine using free-ranging koalas. The first study was by Waugh et al. (19). This vaccine consisted of three chlamydial major outer membrane proteins (MOMP) from three genotypes (A, F, and G) and an Immunostimulating Complex (ISC) adjuvant, that was delivered subcutaneously three times over 3 months (given at 1-month intervals). Chlamydial load and disease were compared 6 months post-vaccination between vaccinated and control koalas in the 60 koala trial. Vaccinated animals had increases in anti-Chlamydia immunoglobulin G (IgG) and lower levels of chlamydial load and prevalence of disease (at both the ocular and urogenital sites) compared to control koalas 6 months after the initial vaccination. The second field study was by Desclozeaux et al. (20). This trial tested two vaccine formulations consisting of either MOMP (three genotypes A, F, and G) or PMP (peripheral membrane protein, genotype G). Both vaccines were delivered subcutaneously alongside a tri-adjuvant (PCEP, IDR1002, and polyI:C) and vaccinated groups were compared to non-vaccinated control koalas (21 koalas in each group, 63 in total). Both MOMP and PMP vaccinated koalas had elevated interferon gamma (IFNγ) and interleukin (IL) 17 mRNA expression 6 months post-vaccination compared to pre-vaccination levels. Additionally, koalas vaccinated with MOMP had lower chlamydial loads compared to control koalas 6 months post-vaccination. Combined, these field trials have examined enough koalas that, for the first time, more detailed modeling analysis of factors involved in vaccine responses in koalas can be considered.
The koala immune response has been a focal point of research in recent years, particularly to chlamydial infection [reviewed by Madden et al. (21)]. Several important cytokines or antibodies in response to chlamydial infection in koalas have been identified: IFNγ, IL17, IL10, tumor necrosis factor alpha (TNFα), IgG, and IgA. As multiple aspects of the koala immune response are poorly understood, researchers often refer to vaccine trials against two other chlamydial species, C. trachomatis or C. muridarum, where mice are most often used as host models of infection (22, 23). Of these murine trials, the most commonly measured host cytokine in response to chlamydial infection is IFNγ (22), where the expression of IFNγ has been associated with protection against chlamydial disease (24). Increases in IFNγ concentration in vitro can lead to the degradation of tryptophan, leading to the starvation of C. trachomatis of this essential amino acid and inducing chlamydial persistence [an inactive, intracellular pathogen response to external stressors; (25)]. A recent study has shown C. pecorum to be resistant to increasing concentrations of IFNγ in vitro, suggestive of different immune-evasion mechanisms as compared to C. trachomatis (26). This difference from C. trachomatis (which is sensitive to IFNγ) could lead to a different effect of IFNγ in koalas to C. pecorum infection. Lastly, in murine hosts, elevated levels of both IFNγ and IL17 increased the production of inducible Nitric Oxide synthase (iNOS), promoting the production of microbicidal nitric oxide (NO) that correlated with the reduction of chlamydial load (27). Some studies, however, have suggested that an elevated iNOS response to infection (elevated by host cytokines) may be associated with scarring of the fallopian tubes and immunopathogenesis (28, 29). The relationships between chlamydial vaccination, the host immune response (particularly IFNγ and IL17), chlamydial load, and disease is still poorly understood in koalas.
Clearly, a key challenge in chlamydial vaccine research is understanding complex direct and indirect immune-mediated control of infection and disease. In this study, we aimed to model important direct and indirect factors surrounding the vaccination of free-ranging koalas: vaccination status, host immune parameter, chlamydial load, and disease. We used structural equation models to identify the directionality and magnitude of direct and indirect relationships when all four variables are modeled together. This statistical method has been used previously to identify both multiple environmental and individual factors that affect chlamydial disease pathology in koalas (30). We modeled vaccination status, the expression of an immune parameter (either IFNγ or IL17), chlamydial load, and disease status at two important mucosal sites (ocular and urogenital) using data collected by Waugh et al. (19) and Desclozeaux et al. (20). We tested six hypotheses to (1) identify the relationships between immune parameter and chlamydial load, and (2) identify the relationships between host immune parameter and disease.
Methods
Experimental Design
Following vaccination or non-vaccination, control and vaccinated koalas were resampled at an average of 5.95 months (± 0.33, 95% CI, referred to as 6 months post-vaccination hereafter). We adopted a cross-sectional comparison of control to vaccinated free-ranging koalas to determine if our vaccines against Chlamydia was effective, given uncontrolled background variation of individual and immune factors. The intent was to assess whether vaccination superseded background individual variation (i.e., immune history, genetics, and seasonality) in parameters, which we know does not protect koalas against Chlamydia disease. We note that koalas were sampled across seasons (Spring n = 17, Summer n = 3, Autumn n = 9, Winter n = 11), but in preliminary analyses we found no effect of season on measurements of either IL17 (Kruskal Wallis X2 = 1.060, p = 0.787) or IFNγ (Kruskal Wallis X2 = 2.185, p = 0.535).
Pooling Data From Both Field Trials
Previously collected data from two field trials of free-ranging koalas vaccinated against Chlamydia described by Waugh et al. (19) and Desclozeaux et al. (20) were used in our analysis (see Appendices 1, 2 for a summary of the methods for both studies). The two field trials (different vaccination schedules and different adjuvants) were investigated to determine if differences exist in the data for MOMP vaccinated individuals (PMP vaccinated individuals were excluded). We first used a meta-analysis to compare the effects of vaccination (control vs. vaccinated animals) on chlamydial load and disease prevalence at either the ocular or urogenital site for 6 month post-vaccination data between studies (Figure 1A). In one trial (19), all koalas (vaccinated and control; see Figure 1A) remained healthy at the ocular site (no effect size could be calculated). An effect size (or a Hedge's g) and variance were calculated to estimate the effect of MOMP vaccination on chlamydial load (at the ocular and urogenital sites) and disease (at the urogenital site) for both field trials [a total of 6 effect sizes; see Borenstein et al. (31)] (19, 20). A meta-regression was performed on the effect sizes using the MAd package (32) in the statistical program R [v3.5.3; (33)] and an I2 statistic was calculated to determine heterogeneity between studies for each measurement (as omnibus models) (31). As control koalas in the field trial by Desclozeaux et al. (20) did not have measurements of IFNγ or IL17 expression, a comparison was made for these immune measurements using univariate methods (Figure 1B). As no evidence indicated differences between the two trials, the data collected from Waugh et al. (19) and Desclozeaux et al. (20) were pooled to test hypothesized models with chlamydial load, disease, and immune response measurements taken at 6 months after the first chlamydial vaccination.
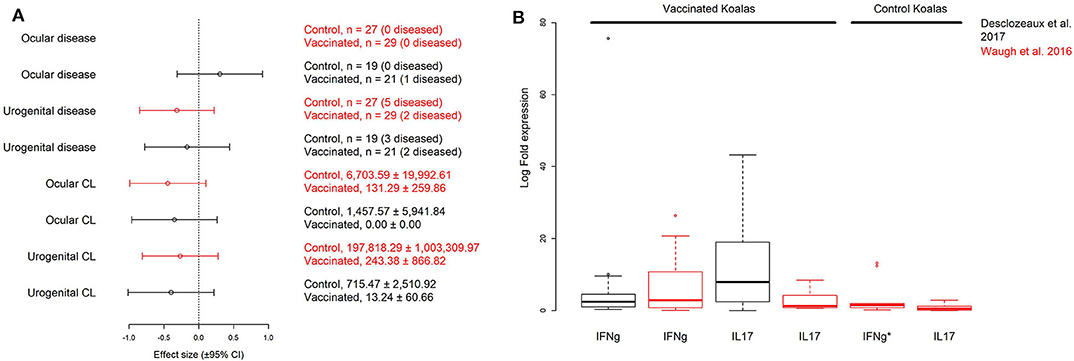
Figure 1. Comparison of hedge's g (± 95% CI) effect sizes for disease and chlamydial load (CL) data collected at the urogenital and ocular sites for vaccinated and control groups (A), and log10 transformed interferon γ (IFNg) and interleukin 17 (IL17) cytokine expression for vaccinated and control groups (B) of wild koalas in two published MOMP-vaccination trials (see Appendices 1, 2): Desclozeaux et al. (20) (black effect sizes and boxplots) and Waugh et al. (19) (red effect sizes and boxplots). All measurements were taken 6 months post-vaccination. Sample size (with n diseased) or mean (± S.D.) copies/μL are shown for each factor in this (A). Despite differences between the two trials, we found no evidence against pooling data between the two trials after performing a meta-regression comparing the effect of MOMP vaccination on disease and chlamydial load comparing treated and control koalas (meta-regression of effect size, g by field trial, p = 0.892). Immune cytokine measurements (fold gene expression relative to the housekeeping gene GAPDH) from vaccinated koalas 6 months post-vaccination were comparable between the two studies after performing a non-parametric Wilcoxon rank sum test for IFNg or IL17 (p = 0.936, and p = 0.075, respectively). To better visualize the data, the IFNg expression from one control koala (IFNg*) is excluded from the figure (2592.26 log fold expression), but included in all analyses. Only Waugh et al. (19) measured the expression of both IFNg and IL17 from non-vaccinated control koalas. Additionally, no effect size could be estimated for ocular disease in the trial by Waugh et al. (19) as all animals were clinically healthy at the ocular site.
Creation and Testing of Hypothesized Models
Six a priori structural equation models were created based on biological hypotheses proposed in the literature (Table 1; see Appendix 1 for additional background information and Appendix 2 for measurement sample sizes). We created our hypothesized models with the assumptions that vaccination affected chlamydial load and host immune parameters (black arrows between V. Status and either C.L. or I.P., see Table 1) established in the original publications by Waugh et al. (19) and Desclozeaux et al. (20). We also made our hypothesized models with the assumption that MOMP vaccination was not directly associated with disease based on previous studies that evaluated the safety of vaccinating healthy koalas against Chlamydia (34, 35). Lastly, we assumed that there was a link between chlamydial load and chlamydial disease (black arrow between C.L. and Disease, see Table 1) based on structural equation models of non-vaccinated free-ranging koalas (30).
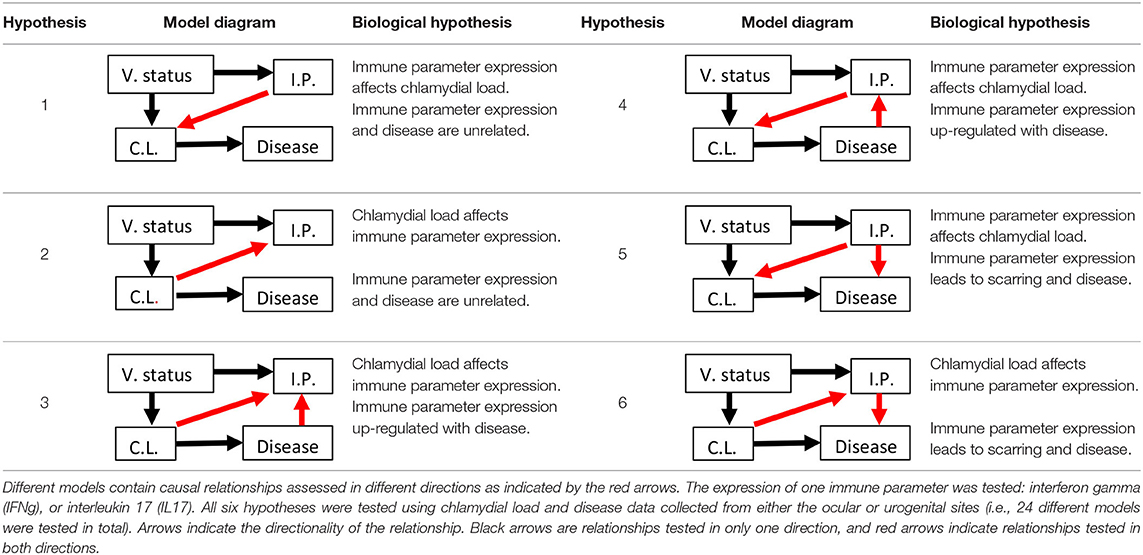
Table 1. Six hypotheses of MOMP (major outer membrane protein) vaccination status (V. status), immune parameter (I.P.) mRNA expression, chlamydial load (C.L.), and disease with explanations for each hypothesized model.
To begin, IFNγ or IL17 expression was evaluated as the immune parameter. Two models were made to determine if, (1) a causal relationship exists between these immune parameters and disease, and (2) the directionality between these immune parameters and chlamydial load (hypotheses 1 and 2). Four additional models were made to assess the directionality between (1) chlamydial load and these immune parameters, and (2) these immune parameters and disease (hypotheses 3, 4, 5, and 6). Only one immune parameter was included at a time in each of the six hypothesized models so as to maintain appropriate 10:1 sample size to variable ratios (36), resulting in 30 different models. The data were separated by either the urogenital or ocular site and tested in each hypothesized model, which resulted in a total of 60 models tested. The ocular models include ocular chlamydial load and disease status (e.g., ocular conjunctivitis), and the urogenital models include urogenital chlamydial load and disease status (e.g., cystitis or dilation of the ovarian bursae).
Prior to model testing, we chose to standardize data from all variables into a 0 to 1 scale to make all model coefficients between all variables comparable within each model. Binomial values were assigned to vaccination status (0 for non-vaccinated and 1 for vaccinated), and ocular and urogenital disease status (0 for clinical disease absent or subclinical and 1 for clinical disease present). Ocular and urogenital chlamydial load measurements were placed in one of three ordinal categories from 0 to 1 depending on an untransformed qPCR result similar to those used by Quigley et al. (30): (1) samples with no detectable qPCR result were given a value of 0, (2) samples with ≤ 100 copies·(μL of swab diluent tested)−1 were detectable but not quantifiable and given a value of 0.5, and (3) samples with > 100 copies·(μL of swab diluent tested)−1 were detectable and quantifiable and given a value of 1. Finally, values for both IFNγ and IL17 expression were transformed to a 0 to 1 scale by dividing each IFNγ or IL17 log fold expression measurement by the maximum IFNγ or IL17 log fold expression measurement, respectively, for all koalas in both studies.
Structural equation modeling (SEM) requires an individual to have all measurements for each variable tested in the model. We first tested each of the 60 models for model fit using the fit indices Root Mean Square Error of Approximation (RMSEA, absolute fit index) and Confirmatory Fit Index (CFI, relative fit index) described by Kline (37), and a Bollen-Stine Bootstrap for non-parametric model fit [100 bootstrap draws; (38, 39)]. No further analysis was performed for models that failed to fit the data using these indices [CFI > 0.9; RMSEA < 0.05; Bollen-Stine p > 0.05; (37, 38)]. Models that fit the data, however, were then compared using Akaike's information criterion corrected for small sample sizes (AICc) and model weights (wi) were calculated (40, 41). All models were tested using R statistical software [v3.5.3; (33)] using the lavaan package (42).
Model Interpretation
A coefficient of determination (R2) was obtained for disease, chlamydial load, and immune parameter expression for each of the fitting models to estimate how well the model predicts each of these variables within the model. All standardized relationship coefficients (± 95% CI) were obtained for each path tested in each of the fitting models. Standardized relationship coefficients indicate the strength of the relationship (the magnitude of the coefficient), direction of the relationship (e.g., positive relationships indicate positive effects of one variable on another), and how well each relationship is predicted (by the 95% CI) by the model. We used standardized relationships in our model interpretation so we could compare the magnitude of all relationships within each model. As some models contained different sample sizes, care was taken not to compare relationship coefficients (or R2) across unrelatable models (i.e., models that do not contain the same variables). We defined strong model relationships based on 95% CI and whether it crossed 0, the threshold of an effect of an exogenous (predictor) variable on an endogenous variable (response). As our models were similar in complexity and limited by sample size, it was not uncommon that more than one model fit the data. For multiple model interpretation, we used model averaging to calculate model averaged parameters (and variance, 95% CI) using the previously described model weights [see Burnham et al. (41) for equation].
Results
We first investigated the effects of MOMP vaccination 6 months after the initial vaccination on chlamydial abundance and disease at two important mucosal sites (ocular and urogenital) between two Chlamydia vaccination trials using free-ranging koalas (19, 20). We estimated effect sizes (as Hedge's g) for these responses [see Methods; (22)] and found no evidence for a difference in MOMP vaccination on chlamydial load (ocular and urogenital) and disease (urogenital) between field trials (meta-regression, p = 0.880; Figure 1A). A similar result was obtained when we analyzed the effect of MOMP vaccination on disease (urogenital) and transformed chlamydial load data (urogenital and ocular being placed into one of three ordinal categories, see Methods; meta-regression, p = 0.247). Estimations of heterogeneity were low for pairwise measurements of urogenital chlamydial load (I2 = 0.000), ocular chlamydial load (I2 = 0.000), and urogenital disease (I2 = 0.000). Additionally, we found measurements of IFNγ or IL17 expression in vaccinated animals to be comparable between the two experiments after performing a non-parametric Wilcoxon rank sum test (IFNγ, p = 0.936; IL17, p = 0.075; Figure 1B). As we found no evidence supporting a difference between the two trials, we pooled the data to obtain sample sizes sufficient to use in structural equation models.
The pooled data from the two trials resulted in a large collection of data for MOMP vaccinated (n = 50) or control (n = 46) wild koalas with recorded clinical disease status (control or vaccinated with ocular disease n = 1, control or vaccinated with urogenital disease n = 7; see Appendix 2) and measured chlamydial load out to 6 months post-vaccination. Of these 96 koalas, a subset of animals had measurements of either IFNγ (n = 40, n = 25 vaccinated koalas, n = 15 control koalas) or IL17 (n = 36, n = 25 vaccinated koalas, n = 11 control koalas) expression (see Appendix 2), and those that were missing these measurements were removed from the analysis (n = 51 and n = 55 for IFNγ and IL17, respectively). Of the 40 animals in the IFNγ expression subset (inclusive of all animals in the subset with IL17 expression), 13 animals had an ocular chlamydial load of ≥ 100 copies·μL−1 (n = 7 vaccinated, n = 6 control) at baseline, while 16 animals had an urogenital chlamydial load of ≥ 100 copies·μL−1 (n = 7 vaccinated, n = 9 control) at baseline. As sample size was limited for this analysis, we could not exclude animals with an infection at baseline nor could we introduce another variable into our models. We focussed on models with both immune parameters analyzed separately as structural equation modeling requires robust sample sizes [i.e., ratio of samples to variables analyzed = 10:1, (36); Appendix 3]. When we tested the 6 hypotheses for both the ocular and urogenital sites with either IFNγ or IL17 expression (Table 1), we obtained multiple models that fit the data (Appendix 4). For multiple hypothesis interpretation, we focussed on coefficients where the 95% confidence interval did not overlap 0 [a strategy used in other structural equation modeling studies such as Dorresteijn et al. (43)]. Additionally, we used a model averaging approach to determine if the direction and magnitude of these relationships were consistent across multiple models.
Models With Ocular Chlamydial Load and Disease: MOMP Vaccination Reduced Ocular Chlamydial Load
All six models with ocular chlamydial load, disease, and IFNγ expression fit the data (Appendix 4). We found that MOMP vaccination had a negative effect on chlamydial load in koalas (hypothesis 1, −0.286 ± 0.276; hyp. 2, −0.320 ± 0.300; hyp. 3, −0.320 ± 0.288; hyp. 6, −0.320 ±0.259; Table 2). We obtained the same result when all models were averaged together (model average, −0.303 ± 0.300; Table 2 and Figure 2A).
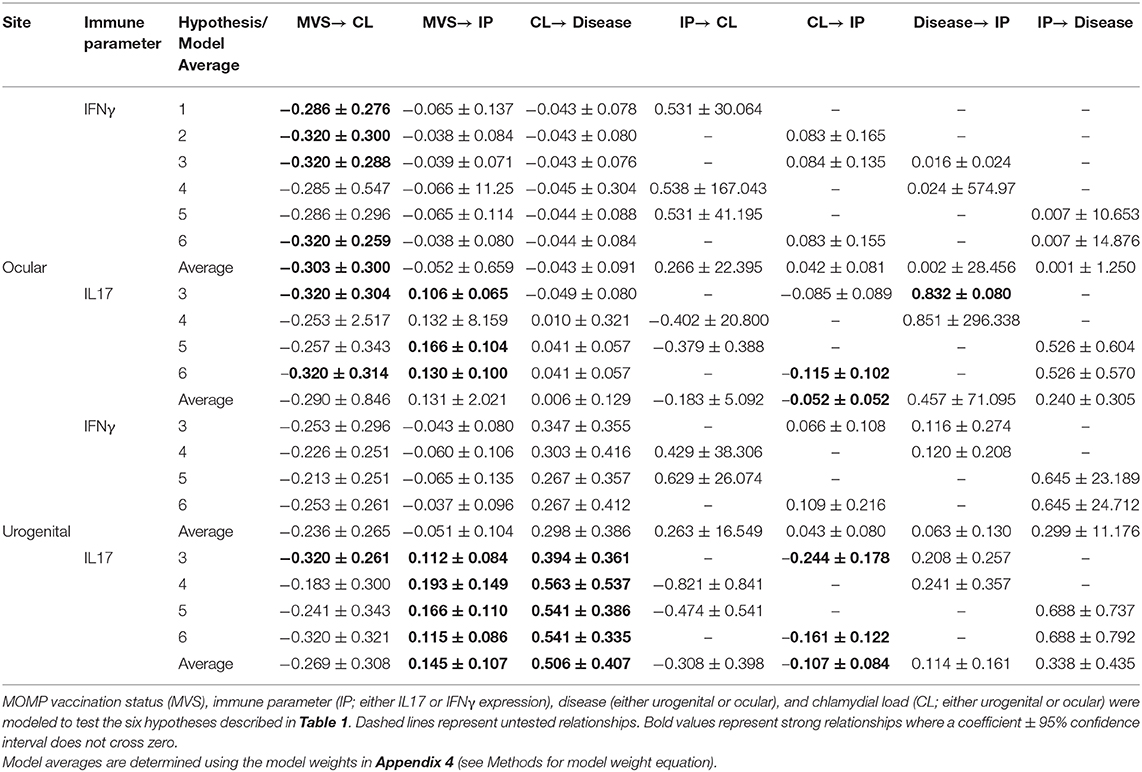
Table 2. Standardized estimates (± 95% CI) for each relationship within all best fitting models identified (see Appendix 4 for model fit and weights).
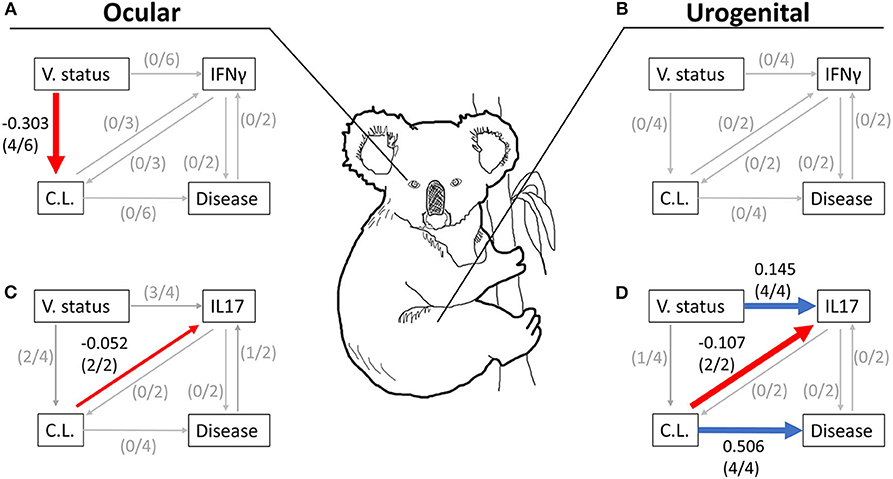
Figure 2. Summary figure of averaged models in Table 2 for ocular (A,C) and urogenital (B,D) data tested with either the expression of IFNγ (A,B) or IL17 (C,D) 6 months post-vaccination. Red and blue lines represent relationships with a negative or positive coefficient, respectively, where the coefficient and its variance (95% CI) did not cross 0 (i.e., the threshold of effect). Very small gray lines represent tested relationships in the averaged model where coefficients and its variance (95% CI) crossed 0. The number of hypotheses with a clear relationship (where the coefficient and its 95% CI did not cross 0) between two variables are listed in parentheses. Care should be made in the interpretation of this summary figure as it does not represent the results from any single model, but across all best fitting models using a model averaging approach. The directionality and magnitude of each coefficient shown in this figure are supported by significant coefficients (i.e., coefficients with 95% CIs that do not cross 0) obtained from the averaged models in Table 2. V. status, MOMP vaccination status; C.L., chlamydial load; Disease, chlamydial disease.
Four models containing ocular chlamydial load, disease, and IL17 expression fit the data (hypotheses 3, 4, 5, and 6; Appendices 4–6). Two of these hypotheses showed that koalas receiving a MOMP vaccination tended to have a lower chlamydial load (hypothesis 3, −0.320 ± 0.304; hyp. 6, −0.320 ± 0.314; Table 2 and Figure 2C), agreeing with models containing IFNγ expression. Three hypotheses indicate that MOMP vaccinated koalas produced more IL17 compared to control koalas (hypothesis 3, 0.106 ± 0.065; hyp. 5, 0.166 ± 0.104; hyp. 6, 0.130 + 0.100), and ocular chlamydial load negatively predicted IL17 expression (−0.115 ± 0.102; hypothesis 6). Lastly, one hypothesis supported a relationship between ocular disease and an increase in IL17 expression (0.832 ± 0.080, hypothesis 3; see Appendix 5 for ocular disease raw data); this however, is complicated as only one koala developed ocular disease after 6 months (IL17 fold expression compared to glyceraldehyde 3-phosphate dehydrogenase, GAPDH = 43.21, n = 1; see Appendix 5 for a comparison with other IL17 expression measurements). When all models were averaged, only one relationship had a marginal effect (ocular chlamydial load has an inverse relationship with IL17 expression; −0.052 ± 0.052; Table 2 and Figure 2C).
Models With Urogenital Chlamydial Load and Disease: MOMP Vaccinated Koalas Reduced Chlamydial Load and Had More IL17 Gene Expression Compared to Control Animals
Four models with urogenital chlamydial load, disease, and IFNγ expression that fit the data (hypotheses 3, 4, 5, and 6; Appendices 4–6). When we investigated the coefficients for each relationship across all urogenital models with IFNγ expression, we found no clear relationships due to the large variance for each coefficient. As a result, we found no clear support for directionality or magnitude between IFNγ expression and urogenital chlamydial load or IFNγ expression and urogenital disease, though some relationships within these models were trending. We found a trending relationship between MOMP vaccinated koalas and a lower urogenital chlamydial load compared to control koalas (−0.236 ± 0.265, Table 2 and Figure 2B).
There were four hypotheses with urogenital chlamydial load and disease and IL17 expression that fit the data (hypotheses 3, 4, 5, and 6; Appendix 4). There was clear evidence that MOMP vaccinated koalas had a lower urogenital chlamydial load compared to control koalas (hypothesis 3, −0.320 ± 0.261). MOMP vaccinated koalas produced more IL17 compared to control animals that was supported by all four hypotheses (hypothesis 3, 0.112 ± 0.084; hyp. 4, 0.193 ± 0.149; hyp. 5, 0.166 ± 0.110; hyp. 6, 0.115 ± 0.086; Table 2). These four hypotheses also indicate that diseased koalas typically had a high chlamydial load (hypothesis 3, 0.394 ± 0.361; hyp. 4, 0.563 ± 0.537; hyp. 5, 0.541 ± 0.386; hyp. 6, 0.541 ± 0.335; Table 2). Two hypotheses indicate that when all koalas are considered (all 36 koalas from both the control or vaccinated groups) with a high chlamydial load tended to produce less IL17 compared to koalas with a low chlamydial load (hypothesis 3, −0.244 ± 0.178; hyp. 6, −0.161 ± 0.122; Table 2). Lastly, across all hypotheses, we found no relationship between IL17 expression and urogenital disease. When all urogenital models with IL17 expression were averaged we found: (1) that MOMP vaccinated koalas produced more IL17 compared to control koalas (0.145 ± 0.107), (2) koalas with a high chlamydial load were more likely to develop urogenital disease 6 months post-vaccination (0.506 ± 0.407), and (3) koalas (both vaccinated and control) with a high urogenital chlamydial load tended to express less IL17 mRNA compared to koalas with a lower urogenital chlamydial load (−0.107 ± 0.084; Table 2 and Figure 2D). These structural equation models therefore connect relationships among MOMP vaccination, IL17 expression, urogenital chlamydial load, and disease (Figure 2D).
Discussion
There exist many unknown aspects surrounding chlamydial vaccination in koalas primarily due to the difficulty in obtaining samples and the cost for vaccine experiments. Much of the underlying chlamydial biology, particularly complex immunological responses to infections, is understood from experiments using the mouse model or from in vitro cell cultures. In the current study, data from two free-ranging koala vaccination trials (both using the major outer membrane protein, MOMP) were used to investigate the effects of MOMP vaccination both directly and indirectly on the expression of koala immune parameters, chlamydial infection, and disease. Koalas were compared from either vaccinated or control groups to evaluate vaccine efficacy given variability associated with free-ranging outbred animals (see Methods). Despite sources of variation in our data (numbers of koalas, use of outbred animals, unknown number of sexual encounters, unknown infection dates) there were clear relationships identified in our analyses that provide unique insights into the effects of vaccinating free-ranging koalas against Chlamydia, relative to unvaccinated individuals in the population. In particular, this study has helped establish one immune cytokine, IL17, may be an important factor in MOMP vaccinated koalas, however, animals with high chlamydial loads are expressing low amounts of IL17 and are more likely to develop urogenital disease.
Two measurements of the koala immune response were incorporated into the models created in this study, IFNγ and IL17 expression. IFNγ, a T-cell secreted cytokine, is currently regarded as one of most important cytokines in chlamydial biology (44). Across all pre-clinical studies investigating a chlamydial vaccine using various animal models (mice, non-human primates, cats, pigs, guinea pigs etc.), there exists a common aim of eliciting strong mucosal T-cell responses, particularly increases to IFNγ concentration (18, 22). Though IFNγ is involved in multiple immune pathways, IFNγ production leads to the enzymatic degradation of tryptophan, effectively starving Chlamydia of this essential amino acid (23). Some studies suggest that tryptophan starvation may even cause C. trachomatis to enter a persistence form (25, 45). Experiments conducted in vitro show that elevated IFNγ concentrations prevent chlamydial growth in C. trachomatis, but not C. pecorum (26). To our knowledge only one study, beside the two reported in the current study, has reported measurements of IFNγ expression and chlamydial load after MOMP vaccination in diseased koalas ex vivo. Nyari et al. (46) found that vaccination of captive koalas with MOMP (delivered along with Tri-adjuvant) did not have a clear effect on systemic IFNγ expression ex vivo despite a reduction in ocular disease among seven koalas (pre-vaccination compared to 6 weeks post-vaccination, n = 7). It is certainly possible that C. trachomatis and C. pecorum have differing sensitivities to IFNγ which may explain why measurements of koala IFNγ expression had no relationship with chlamydial load in any of our models (either ocular or urogenital; Table 2 and Figures 2A,B). These measurements of IFNγ expression contain large amounts of variance that can be seen in the raw data (Appendix 6). The average log transformed IFNγ measurement between the non-vaccinated and vaccinated groups were similar, 1.378 ± 0.918 (95% CI, n = 16) and 1.445 ± 0.430 (95% CI, n = 25), respectively. Additionally, IFNγ measurements have been shown to be dependent on the stage of chlamydial infection in mice (47) and non-human primates (48), something that is currently uncontrollable in trials using free-ranging koalas. More longitudinal studies are needed to determine if IFNγ expression is a key factor in protection against C. pecorum infection in free-ranging koalas.
In contrast, models with IL17 expression indicated that koalas that typically had higher ocular and urogenital chlamydial load tended to have low levels of IL17 expression, 6 months post-vaccination (Table 2 and red arrows in Figures 2C,D). Experiments using IL17 knockout mice have shown that IL17 is a factor in both protection (49) as well as pathogenesis (50). IL17 is a pro-inflammatory cytokine secreted mainly from T cells and has many functions in response to chlamydial infection. In the wild type mouse model and in vitro experiments, IL17 has been shown to work synergistically with IFNγ to inhibit C. muridarum growth by increasing the expression of inducible nitric oxide synthase (iNOS) and indirectly increasing antimicrobial nitric oxide [promoted by iNOS; (27)]. Indeed, iNOS has been shown to be up-regulated in human patients suffering from ectopic pregnancies associated with C. trachomatis infection (28). In koalas, one study reported the IL17a response from koala PBMCs to be higher in clinically diseased animals (n = 12) compared to subclinical animals [n = 29; (51)]. Three studies since have measured free-ranging koala IL17 response to chlamydial vaccination. In the first, Khan et al. (52) focussed on non-diseased koalas that tested negative for Chlamydia, finding that IL17 expression increased with MOMP vaccination when compared to pre-vaccination levels. The second and third, Waugh et al. (19) and Desclozeaux et al. (20), were included in our current analysis. One additional study using captive koalas with ocular disease reported comparable measurements of IL17a expression between koalas at pre-vaccination and 6 weeks post-vaccination (n = 7), even though there was improvement of ocular disease scores among the cohort at 6 weeks post-vaccination (46). In our current analysis, we found that koalas vaccinated with MOMP tended to have higher IL17 expression 6 months post-vaccination compared to non-vaccinated koalas in models based on urogenital data (Figure 2D). This was supported by three hypotheses (out of four) based on ocular data (Table 2). This increase can be seen by inspecting the raw data as the average log transformed expression of IL17 was higher in vaccinated compared to non-vaccinated groups, 1.616 ± 0.430 (95% CI, n = 25) and 0.462 ± 0.279 (95% CI, n = 12), respectively (Appendix 6). Additionally, we found no clear evidence for a direct relationship between IL17 expression and either ocular or urogenital disease that developed 6 months post-vaccination. Interestingly, the directionality of the relationship between chlamydial load and IL17, suggests that IL17 expression is dependent on chlamydial load. Perhaps animals with a high chlamydial load are unable to express IL17 or, more likely, are producing an immune response without IL17 when a high abundance of Chlamydia is present. Indeed, IL17 is one immune variable among many others that are part of a complex immune response to Chlamydia and its expression may or may not be a major factor in reducing chlamydial growth and preventing pathogenesis. More work needs to be done to determine if IL17 expression is linked to the reduction of chlamydial load and protection against disease in koalas.
Both Waugh et al. (19) and Desclozeaux et al. (20) evaluated the effect of MOMP vaccination on subsequent chlamydial disease, using univariate statistical methods. Waugh et al. (19) found that fewer koalas developed disease after 12 months in the MOMP vaccinated group (1 of 23 koalas) compared to control animals (4 of 27 koalas). This result, however, was not statistically significant (X2 = 1.512, p = 0.363) likely owing to the fact that many chlamydial infections remain subclinical and that the total sample size (n = 50) may have been too small to determine a statistically significant effect of vaccination on disease. Desclozeaux et al. (20) found that fewer koalas in the MOMP vaccinated group developed chlamydiosis (either ocular or urogenital chlamydial disease) compared to control koalas (0 of 21 and 3 of 21 for MOMP and control koalas, respectively); this comparison showed a trending (p < 0.1) effect between groups (X2 = 2.813, p = 0.093). Desclozeaux et al. (20) also found that six MOMP vaccinated koalas, with a detectable chlamydial infection recorded at pre-vaccination, reduced their chlamydial load (in either the ocular, urogenital or both sites) 6-months post-vaccination (6 of 21 koalas), and this effect was statistically significant compared to control koalas (0 of 21 koalas; p = 0.048).
By combining data from both trials, we were able to expand upon these analyses by introducing additional realistic complexity using structural equation models. Preliminary structural equation models indicated that a direct relationship between MOMP vaccination status and subsequent disease development failed our fitting criterion. Given this result, we created models where MOMP vaccination status indirectly affected disease status 6 months post-vaccination through direct relationships with either chlamydial load or an immune parameter. Ideally, we would have included both IFNγ and IL17 expression in the same models, but sample size limitations (having individual koalas in each group measured for all variables: IFNγ, IL17, infection load, disease status) prevented models with this level of complexity and, hence, we adopted a IFNγ or IL17 expression SEM approach. An additional ramification of our sample size limitation was that we were unable to exclude animals with baseline infections (animals with an ocular chlamydial load of >100 copies·μL−1, n = 7 vaccinated and n = 6 control; or urogenital chlamydial load of >100 copies·μL−1 n = 7 vaccinated and n = 9 control) nor were we able to include this as a variable within our models. Multiple models using ocular data and one model using urogenital data (Table 2) indicated that MOMP vaccination status had a direct negative effect on chlamydial load. These effects, however, were lost when we averaged all the urogenital models together. It is possible that given a larger sample size, we could have detected an effect. We performed a power analysis to determine the necessary sample size to detect a univariate effect of MOMP vaccination on urogenital chlamydial load. After estimating an effect size (Cohen's d) to estimate the effect of MOMP vaccination on urogenital chlamydial load (31), we found that a sample size of 117 koalas would be necessary to have an 80% chance of detecting an effect at a significance level of 0.05. This finding suggests that an effect might be obtained if a large-scale study included nearly three times the number of koalas that were used in our analysis.
In this study, we adopted a cross-sectional experimental design that focussed on control vs. vaccinated koalas at 6 months post-vaccination from which to implement our models. We chose this study design because research has shown koalas can become infected with C. pecorum and exhibit disease year-round. Therefore, background variation in individual parameters (immune factors, genotype, season sampled, etc.) appears insufficient to protect koalas from infection or disease, and the effect of a vaccine on immune parameters would most likely need to exceed normal background variation observed. We also acknowledge other valid study designs could be utilized. A common design for data similar to ours is to compare the change in variables over time (pre to post-vaccination) between the control and vaccinated groups. Although this is a valid approach, in our study system we chose not to adopt the change in variable approach for the reason described above, and because a vaccination effect (relative to controls) could be observed at the cohort level and still be within background variation. While not the determining reason, we do also acknowledge that utilizing a change in chlamydial load or host immune expression from baseline would further reduce the sample size for analyses (n = 3 for each model) in our study. Future research with a greater sample size of koala could consider a comparison of these designs, or the extent to which individual responses to vaccination over time are repeatable.
Chlamydial load is among one of several factors that affect disease pathogenesis in free-ranging koalas (30). Specifically, Quigley et al. (30) found that measurements of chlamydial load (separated into 3 ordinal categories of severity) are strong predictors of urogenital disease, but not ocular disease. In our current study, we also found that koalas that developed urogenital chlamydial disease had a higher chlamydial load as compared to non-diseased animals when the data were modeled with IL17 expression. We did not detect this effect in models of ocular chlamydial load and disease, though this was likely due to a low prevalence of koalas with ocular disease (n = 1). Previous studies have suggested that disease pathogenesis in koalas is complex (21, 53). Three out of four of our averaged models (Figures 2B–D) excluded Hypotheses 1 and 2, possibly suggesting that immune parameter and disease are related, however this remains unclear as only one relationship was significant (ocular chlamydial load and IL17; see Table 2). A large amount of variation in the prevalence of urogenital disease 6 months post-vaccination remains unexplained from even our best models (R2 = 0.268). Including more factors that are currently associated with disease pathogenesis, including koala retrovirus (KoRV) status, may improve the predictability of these models.
Conclusions
In this study, we used structural equation models to identify direct and indirect relationships underpinning vaccination against chlamydial disease in free-ranging koalas. The use of structural equation modeling has previously been applied to identify complex factors influencing disease pathogenesis in koalas (30). One factor, urogenital chlamydial load, was positively associated with urogenital disease prevalence, agreeing with the findings of Quigley et al. (30). We found that MOMP vaccination may indirectly affect the development of urogenital disease in koalas by inhibiting the growth of Chlamydia (i.e., lower chlamydial load compared to control koalas). IL17 expression is one immune parameter that was increased in MOMP vaccinated koalas as compared to control koalas. Chlamydial load was a negative predictor of IL17 expression, suggesting that hosts express this cytokine less in response to chlamydial growth. Collectively, the structural equation models in this study highlight the importance of considering vaccine-immune-chlamydial load-disease relationships in a single analysis that can be performed in future studies of novel correlates of protection against Chlamydia. Lastly, more studies using free-ranging animals may be needed to determine the effect of multiple immune parameters on chlamydial load and disease and may make it possible to detect a direct effect of MOMP vaccination on chlamydial load.
Data Availability Statement
Most of the data generated or analyzed during this study are included in the published articles (19, 20). Measurements of cytokine expression production for IL17 and IFNγ in the current study are available from the corresponding author on reasonable request.
Ethics Statement
Collection of koala data previously collected by Waugh et al. (19) and Desclozeaux et al. (20) were approved by the University of the Sunshine Coast (USC) Animal Ethics Committee (AN/A/13/80) and the Queensland Government (Scientific Purposes Permit, WISP11532912).
Author Contributions
DL, PT, BQ, and SC conceived the study design. PT and JH collected the data. DL performed the analysis. All authors contributed to interpreting the results and drafting of the manuscript.
Funding
This project was supported by the Australian Research Council (Linkage Project 160100138) to PT and SC. Funding for original data collection awarded to PT, A. Polkinghorne, and K. Beagley (LP1202000051) by the Australian Research Council. The overall koala work was supported by many groups including significant support by the Queensland Government (Department of Transport and Main Roads) and specifically the Moreton Bay Rail project team.
Conflict of Interest
JH was employed by the company Endeavor Veterinary Ecology.
The remaining authors declare that the research was conducted in the absence of any commercial or financial relationships that could be construed as a potential conflict of interest.
Acknowledgments
We thank C. X. Cunningham for his modeling advice and C. L. Exton for her koala illustration. We thank the many groups that supported the overall koala data collection, specifically, for collecting the data published in Waugh et al. (19) and Desclozeaux et al. (20), respectively, that were used in the current study. This manuscript was previously posted as a pre-print on ResearchSquare, Lizárraga et al. (54), and updated upon the authors request for its withdrawal, Lizárraga et al. (55).
Supplementary Material
The Supplementary Material for this article can be found online at: https://www.frontiersin.org/articles/10.3389/fvets.2020.530686/full#supplementary-material
References
1. Melzer A, Carrick F, Menkhorst P, Lunney D, John BS. Overview, critical assessment, and conservation implications of koala distribution and abundance. Conserv Biol. (2000) 14:619–28. doi: 10.1046/j.1523-1739.2000.99383.x
2. McAlpine C, Lunney D, Melzer A, Menkhorst P, Phillips S, Phalen D, et al. Conserving koalas: a review of the contrasting regional trends, outlooks and policy challenges. Biol Conserv. (2015) 192:226–36. doi: 10.1016/j.biocon.2015.09.020
3. Adams-Hosking C, McBride MF, Baxter G, Burgman M, De Villiers D, Kavanagh R, et al. Use of expert knowledge to elicit population trends for the koala (Phascolarctos cinereus). Divers Distributions. (2016) 22:249–62. doi: 10.1111/ddi.12400
4. Rhodes JR, Beyer H, Preece H, McAlpine C. South east Queensland Koala Population Modelling Study. (2015). Available online at: https://environment.des.qld.gov.au/__data/assets/pdf_file/0029/88913/seq-koala-population-modelling-study.pdf (accessed September 12, 2020).
5. Woinarski JC, Burbidge AA, Harrison PL. Ongoing unraveling of a continental fauna: decline and extinction of Australian mammals since European settlement. Proc Natl Acad Sci USA. (2015) 112:4531–40. doi: 10.1073/pnas.1417301112
6. Mcalpine CA, Bowen ME, Callaghan JG, Lunney D, Rhodes JR, Mitchell DL, et al. Testing alternative models for the conservation of koalas in fragmented rural–urban landscapes. Austral Ecol. (2006) 31:529–44. doi: 10.1111/j.1442-9993.2006.01603.x
7. Seabrook L, McAlpine C, Baxter G, Rhodes J, Bradley A, Lunney D. Drought-driven change in wildlife distribution and numbers: a case study of koalas in south west Queensland. Wildlife Res. (2011) 38:509–24. doi: 10.1071/WR11064
8. Lunney D, Gresser S, O'neill LE, Matthews A, Rhodes J. The impact of fire and dogs on koalas at port stephens, new south wales, using population viability analysis. Pac Conserv Biol. (2007) 13:189–201. doi: 10.1071/PC070189
9. Rhodes JR, Ng CF, de Villiers DL, Preece HJ, McAlpine CA, Possingham HP. Using integrated population modelling to quantify the implications of multiple threatening processes for a rapidly declining population. Biol Conserv. (2011) 144:1081–8. doi: 10.1016/j.biocon.2010.12.027
10. McCallum H, Kerlin DH, Ellis W, Carrick F. Assessing the significance of endemic disease in conservation—koalas, Chlamydia, and koala retrovirus as a case study. Conserv Lett. (2018) 11:e12425. doi: 10.1111/conl.12425
11. Beyer HL, de Villiers D, Loader J, Robbins A, Stigner M, Forbes N, et al. Management of multiple threats achieves meaningful koala conservation outcomes. J Appl Ecol. (2018) 55:1966–75. doi: 10.1111/1365-2664.13127
12. Polkinghorne A, Hanger J, Timms P. Recent advances in understanding the biology, epidemiology and control of chlamydial infections in koalas. Vet Microbiol. (2013) 165:214–23. doi: 10.1016/j.vetmic.2013.02.026
13. Jackson M, White N, Giffard P, Timms P. Epizootiology of Chlamydia infections in two free-range koala populations. Vet Microbiol. (1999) 65:255–64. doi: 10.1016/S0378-1135(98)00302-2
14. Jackson M, Giffard P, Timms P. Outer membrane protein A gene sequencing demonstrates the polyphyletic nature of koala Chlamydia pecorum isolates. Syst Appl Microbiol. (1997) 20:187–200. doi: 10.1016/S0723-2020(97)80065-3
15. Loader J. An investigation of the health of wild koala populations in South-East Queensland. In: Bachelor of Applied Science (Animal Studies) Honours Thesis. St. Lucia, QLD: School of Animal Studies, The University of Queensland (2010). p. 1–219.
16. Robbins A, Loader J, Timms P, Hanger J. Optimising the short and long-term clinical outcomes for koalas (Phascolarctos cinereus) during treatment for chlamydial infection and disease. PLoS ONE. (2018) 13:e0209679. doi: 10.1371/journal.pone.0209679
17. Craig AP, Hanger J, Loader J, Ellis WA, Callaghan J, Dexter C, et al. A 5-year Chlamydia vaccination programme could reverse disease-related koala population decline: Predictions from a mathematical model using field data. Vaccine. (2014) 32:4163–70. doi: 10.1016/j.vaccine.2014.05.049
18. Phillips S, Quigley BL, Timms P. Seventy years of Chlamydia vaccine research–limitations of the past and directions for the future. Front Microbiol. (2019) 10:70. doi: 10.3389/fmicb.2019.00070
19. Waugh C, Khan SA, Carver S, Hanger J, Loader J, Polkinghorne A, et al. A prototype recombinant-protein based Chlamydia pecorum vaccine results in reduced chlamydial burden and less clinical disease in free-ranging koalas (Phascolarctos cinereus). PLoS ONE. (2016) 11:e0146934. doi: 10.1371/journal.pone.0146934
20. Desclozeaux M, Robbins A, Jelocnik M, Khan SA, Hanger J, Gerdts V, et al. Immunization of a wild koala population with a recombinant Chlamydia pecorum major outer membrane protein (MOMP) or polymorphic membrane protein (PMP) based vaccine: new insights into immune response, protection and clearance. PLoS ONE. (2017) 126:e0178786. doi: 10.1371/journal.pone.0178786
21. Madden D, Whaite A, Jones E, Belov K, Timms P, Polkinghorne A. Koala immunology and infectious diseases: how much can the koala bear? Dev Comp Immunol. (2018) 82:177–85. doi: 10.1016/j.dci.2018.01.017
22. Lizárraga D, Carver S, Timms P. Navigating to the most promising directions amid complex fields of vaccine development: a chlamydial case study. Expert Rev Vaccines. (2019) 18:1323–37. doi: 10.1080/14760584.2019.1698954
23. Vasilevsky S, Greub G, Nardelli-Haefliger D, Baud D. Genital Chlamydia trachomatis: understanding the roles of innate and adaptive immunity in vaccine research. Clin Microbiol Rev. (2014) 27:346–70. doi: 10.1128/CMR.00105-13
24. Igietseme J, Ukwade C, Ouburg S, Omosun Y, Joseph K. Profile of anti-Chlamydia immune responses in complicated (infertile) and non-complicated (fertile) genital infections. J Clin Immunol Immunother. (2015) 2:1–5. doi: 10.24966/CIIT-8844/100006
25. Beatty WL, Belanger TA, Desai AA, Morrison RP, Byrne GI. Tryptophan depletion as a mechanism of gamma interferon-mediated chlamydial persistence. Infect Immun. (1994) 62:3705–11. doi: 10.1128/IAI.62.9.3705-3711.1994
26. Islam MM, Jelocnik M, Huston WM, Timms P, Polkinghorne A. Characterization of the in vitro Chlamydia pecorum response to gamma interferon. Infect Immun. (2018) 86:e00714–17. doi: 10.1128/IAI.00714-17
27. Zhang Y, Wang H, Ren J, Tang X, Jing Y, Xing D, et al. IL-17A synergizes with IFN-γ to upregulate iNOS and NO production and inhibit chlamydial growth. PLoS ONE. (2012) 7:e39214. doi: 10.1371/journal.pone.0039214
28. Refaat B, Al-Azemi M, Geary I, Eley A, Ledger W. Role of activins and inducible nitric oxide in the pathogenesis of ectopic pregnancy in patients with or without Chlamydia trachomatis infection. Clin Vaccine Immunol. (2009) 16:1493–503. doi: 10.1128/CVI.00221-09
29. Agrawal T, Bhengraj AR, Vats V, Salhan S, Mittal A. Expression of TLR 2, TLR 4 and iNOS in cervical monocytes of Chlamydia trachomatis-infected women and their role in host immune response. Am J Reprod Immunol. (2011) 66:534–43. doi: 10.1111/j.1600-0897.2011.01064.x
30. Quigley BL, Carver S, Hanger J, Vidgen ME, Timms P. The relative contribution of causal factors in the transition from infection to clinical chlamydial disease. Sci Rep. (2018) 8:8893. doi: 10.1038/s41598-018-27253-z
31. Borenstein M, Hedges LV, Higgens JP, Rothstein HR. Introduction to Meta-Analysis. Hoboken, NJ: John Wiley & Sons, Ltd. (2009). p. 1–421. doi: 10.1002/9780470743386
32. Viechtbauer W. Conducting meta-analyses in R with the metafor package. J Stat Softw. (2010) 36:1–48. doi: 10.18637/jss.v036.i03
33. Team RC: R: A Language and Environment for Statistical Computing. Vienna: R Foundation for Statistical Computing (2017).
34. Kollipara A, George C, Hanger J, Loader J, Polkinghorne A, Beagley K, et al. Vaccination of healthy and diseased koalas (Phascolarctos cinereus) with a Chlamydia pecorum multi-subunit vaccine: evaluation of immunity and pathology. Vaccine. (2012) 30:1875–85. doi: 10.1016/j.vaccine.2011.12.125
35. Hernández-Sánchez J, Brumm J, Timms P, Beagley KW. Vaccination of koalas with a prototype chlamydial vaccine is safe, does not increase the incidence of lymphoma-related disease and maybe associated with increased lifespan in captive koalas. Vaccine. (2015) 33:4459–63. doi: 10.1016/j.vaccine.2015.07.029
36. MacCallum RC, Widaman KF, Zhang S, Hong S. Sample size in factor analysis. Psychol Methods. (1999) 4:84–99. doi: 10.1037/1082-989X.4.1.84
37. Kline RB. Principles and Practice of Structural Equation Modeling. New York, NY: Guilford publications (2015)
38. Bollen KA, Stine RA. Bootstrapping goodness-of-fit measures in structural equation models. Sociol Methods Res. (1992) 21:205–29. doi: 10.1177/0049124192021002004
39. Kim H, Millsap R. Using the bollen-stine bootstrapping method for evaluating approximate fit indices. Multiv Behav Res. (2014) 49:581–96. doi: 10.1080/00273171.2014.947352
40. Burnham K, Anderson D. Model Selection and Multi-Model Inference. Second NY: Springer-Verlag (2004). p. 63. doi: 10.1007/b97636
41. Burnham KP, Anderson DR, Huyvaert KP. AIC model selection and multimodel inference in behavioral ecology: some background, observations, and comparisons. Behav Ecol Sociobiol. (2011) 65:23–35. doi: 10.1007/s00265-010-1029-6
42. Rosseel Y. Lavaan: an R package for structural equation modeling and more. Version 0.5–12 (BETA). J Stat Softw. (2012) 48:1–36. doi: 10.18637/jss.v048.i02
43. Dorresteijn I, Schultner J, Nimmo DG, Fischer J, Hanspach J, Kuemmerle T, et al. Incorporating anthropogenic effects into trophic ecology: predator–prey interactions in a human-dominated landscape. Proc R Soc B Biol Sci. (2015) 282:20151602. doi: 10.1098/rspb.2015.1602
44. Brunham RC, Rey-Ladino J. Immunology of Chlamydia infection: implications for a Chlamydia trachomatis vaccine. Nat Rev Immunol. (2005) 5:149–61. doi: 10.1038/nri1551
45. Beatty WL, Byrne GI, Morrison RP. Morphologic and antigenic characterization of interferon gamma-mediated persistent Chlamydia trachomatis infection in vitro. Proc Natl Acad Sci USA. (1993) 90:3998–4002. doi: 10.1073/pnas.90.9.3998
46. Nyari S, Booth R, Quigley BL, Waugh CA, Timms P. Therapeutic effect of a Chlamydia pecorum recombinant major outer membrane protein vaccine on ocular disease in koalas (Phascolarctos cinereus). PLoS ONE. (2019) 14:e0210245. doi: 10.1371/journal.pone.0210245
47. Cheng C, Pal S, Tifrea D, Jia Z, Luis M. A vaccine formulated with a combination of TLR-2 and TLR-9 adjuvants and the recombinant major outer membrane protein elicits a robust immune response and significant protection against a Chlamydia muridarum challenge. Microbes Infect. (2014) 16:244–52. doi: 10.1016/j.micinf.2013.11.009
48. Cheng C, Pal S, Bettahi I, Oxford KL, Barry PA, Luis M. Immunogenicity of a vaccine formulated with the Chlamydia trachomatis serovar F, native major outer membrane protein in a nonhuman primate model. Vaccine. (2011) 29:3456–64. doi: 10.1016/j.vaccine.2011.02.057
49. Scurlock AM, Frazer LC, Andrews CW, O'Connell CM, Foote IP, Bailey SL, et al. Interleukin-17 contributes to generation of Th1 immunity and neutrophil recruitment during Chlamydia muridarum genital tract infection but is not required for macrophage influx or normal resolution of infection. Infect Immun. (2011) 79:1349–62. doi: 10.1128/IAI.00984-10
50. Andrew DW, Cochrane M, Schripsema JH, Ramsey KH, Dando SJ, O'Meara CP, et al. The duration of Chlamydia muridarum genital tract infection and associated chronic pathological changes are reduced in IL-17 knockout mice but protection is not increased further by immunization. PLoS ONE. (2013) 8:e76664. doi: 10.1371/journal.pone.0076664
51. Mathew M, Pavasovic A, Prentis PJ, Beagley KW, Timms P, Polkinghorne A. Molecular characterisation and expression analysis of Interferon gamma in response to natural Chlamydia infection in the koala, Phascolarctos cinereus. Gene. (2013) 527:570–7. doi: 10.1016/j.gene.2013.06.019
52. Khan SA, Desclozeaux M, Waugh C, Hanger J, Loader J, Gerdts V, et al. Antibody and cytokine responses of koalas (Phascolarctos cinereus) vaccinated with recombinant chlamydial major outer membrane protein (MOMP) with two different adjuvants. PLoS ONE. (2016) 11:e0156094. doi: 10.1371/journal.pone.0156094
53. Wan C, Loader J, Hanger J, Beagley K, Timms P, Polkinghorne A. Using quantitative polymerase chain reaction to correlate Chlamydia pecorum infectious load with ocular, urinary and reproductive tract disease in the koala (Phascolarctos cinereus). Austr Vet J. (2011) 89:409–12. doi: 10.1111/j.1751-0813.2011.00827.x
54. Lizárraga D, Timms P, Quigley BL, Hanger J, Carver S. Capturing complex vaccine-immune-disease relationships for free-ranging koalas: IL17 production is an important intermediate factor against chlamydial disease. Res Square. (2020). doi: 10.21203/rs.2.21243/v1
Keywords: structural equation model, Chlamydia, vaccine, koala, cytokine
Citation: Lizárraga D, Timms P, Quigley BL, Hanger J and Carver S (2020) Capturing Complex Vaccine-Immune-Disease Relationships for Free-Ranging Koalas: Higher Chlamydial Loads Are Associated With Less IL17 Expression and More Chlamydial Disease. Front. Vet. Sci. 7:530686. doi: 10.3389/fvets.2020.530686
Received: 30 January 2020; Accepted: 21 August 2020;
Published: 25 September 2020.
Edited by:
Jasim Muhammad Uddin, Bangladesh Agricultural University, BangladeshReviewed by:
S. Khan, Chittagong Veterinary and Animal Sciences University, BangladeshJoanne Meers, The University of Queensland, Australia
Sandra Andorf, University of Cincinnati, United States
Copyright © 2020 Lizárraga, Timms, Quigley, Hanger and Carver. This is an open-access article distributed under the terms of the Creative Commons Attribution License (CC BY). The use, distribution or reproduction in other forums is permitted, provided the original author(s) and the copyright owner(s) are credited and that the original publication in this journal is cited, in accordance with accepted academic practice. No use, distribution or reproduction is permitted which does not comply with these terms.
*Correspondence: David Lizárraga, david.lizarraga@utas.edu.au