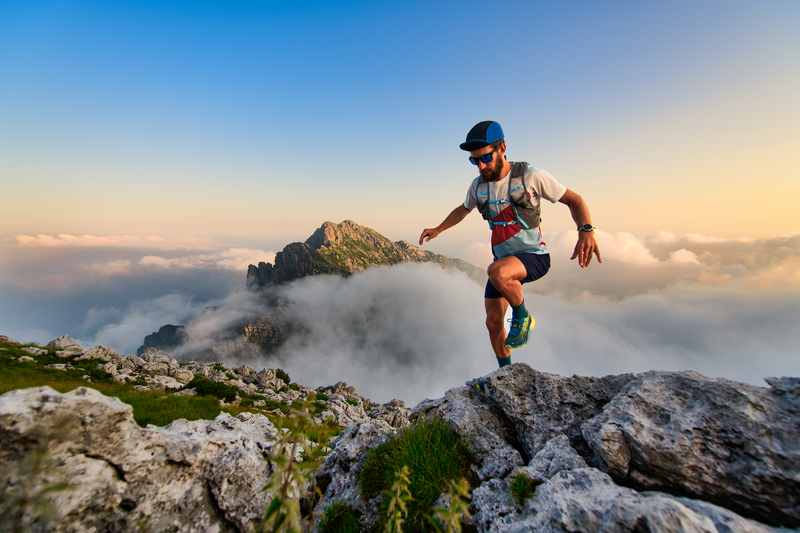
95% of researchers rate our articles as excellent or good
Learn more about the work of our research integrity team to safeguard the quality of each article we publish.
Find out more
ORIGINAL RESEARCH article
Front. Vet. Sci. , 22 April 2020
Sec. Veterinary Infectious Diseases
Volume 7 - 2020 | https://doi.org/10.3389/fvets.2020.00208
This article is part of the Research Topic Antimicrobial Use, Antimicrobial Resistance, and the Microbiome in Food Animals View all 13 articles
Rapid and accurate diagnosis of bovine respiratory disease (BRD) presents a substantial challenge to the North American cattle industry. Here we utilize recombinase polymerase amplification (RPA), a fast and sensitive isothermal DNA-based technology for the detection of four BRD pathogens (Mannheimia haemolytica, Pasteurella multocida, Histophilus somni, Mycoplasma bovis), genes coding antimicrobial resistance (AMR) and integrative conjugative elements (ICE) which can harbor AMR genes. Eleven RPA assays were designed and validated including: a) one conventional species-specific multiplex assay targeting the 4 BRD pathogens, b) two species-specific real-time multiplex RPA assays targeting M. haemolytica/M. bovis and P. multocida/H. somni, respectively with a novel competitive internal amplification control, c) seven conventional assays targeting AMR genes (tetH, tetR, msrE, mphE, sul2, floR, erm42), and d) one real-time assay targeting ICE. Each real-time RPA assay was tested on 100 deep nasopharyngeal swabs (DNPS) collected from feedlot cattle previously assessed for targets using either culture methods and/or polymerase chain reaction (PCR) verification (TC-PCR). The developed RPA assays enabled sensitive and accurate identification of BRD agents and AMR/ICE genes directly from DNPS, in a shorter period than TC-PCR, showing considerable promise as a tool for point-of-care identification of BRD pathogens and antimicrobial resistance genes.
Bovine respiratory disease (BRD) remains the most common and economically important disease affecting feedlot cattle, veal calves, weaned dairy heifers and beef calves (1, 2). Approximately 15% of cattle in North America are treated for BRD, accounting for 70% of morbidities and 40% of all cattle mortalities in feedlots (3, 4). Economic losses to the United States feedlot industry have been reported to be as high as 1 billion dollars annually, due to losses in production, increased labor expenses, drug costs, and death (5, 6). As the clinical symptoms associated with BRD may be non-specific, subtle and exhibit a rapid onset, fast and accurate diagnosis of BRD presents a significant challenge (2). Often, cattle with BRD are detected late in the disease process or not at all (2).
BRD is characterized by complex interactions between the host's immune system, bacterial (i.e., Mannheimia haemolytica, Pasteurella multocida, Histophilus somni, and Mycoplasma bovis) and viral (i.e., Bovine Herpes Virus-1, Parainfluenza-3, Bovine Viral Diarrhea Virus, Bovine Respiratory Syncytial Virus) pathogens and management practices that increase stress such as weaning and transportation (4, 6–8). Although M. haemolytica is considered to be the predominant BRD agent (9), many of the bacterial species involved are ubiquitous and considered to be commensals of the bovine respiratory tract of healthy animals (4). However, suppression of the host immune system as a result of stress or viral infection can allow these pathogens to proliferate within the upper respiratory tract, spreading to the lower respiratory tract, resulting in lesions and acute pleuropneumonia (4, 6).
Controlling BRD is the primary reason for the use of antimicrobials in feedlot cattle (4). Often, metaphylactic administration of macrolides to asymptomatic animals in the presence of diseased animals is used to improve the welfare of cattle and to decrease financial losses as a result of morbidities and mortalities (4, 10). However, antimicrobial use selects for antimicrobial-resistant (AMR) bacteria, including pathogens as well as harmless bacteria that can potentially act as a genetic reservoir of AMR gene determinants (4, 11). Excluding M. bovis, the genomes of BRD pathogens often contain integrative conjugative elements (ICE), mobile genetic elements that can harbor multiple AMR genes and encode the conjugation machinery required for transfer of ICE between BRD pathogens and to other bacteria (4, 9). The resulting multi-drug resistance (MDR) among some BRD pathogens containing ICE presents a significant challenge for the efficacy of antimicrobial therapy as a treatment for BRDClawson et al. (12) found that the gene tet(H), which confers tetracycline resistance was present in all AMR M. haemolytica strains isolated from confirmed BRD cases, and was also frequently found in P. multocida (13) and H. somni ICE (14). Furthermore, tet(H) was adjacent to the transposase gene tnpA, a core ICE gene associated with increased minimum inhibitory antimicrobial concentrations in M. haemolytica, H. somni, and P. multocida (15).
Isolation of BRD pathogens by traditional culture methods and PCR verification of bacterial isolates (TC-PCR) has long been used to confirm disease outbreaks, but with several limitations (16). Traditional culture methods are time-consuming, requiring several days to obtain bacterial isolates, and some species such as M. bovis and H. somni grow poorly, a characteristic that may result in an under representation of the role of these pathogens in BRD (16–18). Therefore, new technologies continue to be evaluated to improve the diagnosis, early detection, and prognosis of BRD (2). In this study, recombinase polymerase amplification (RPA) is proposed as an alternative diagnostic application for BRD because of its simplicity, flexibility, multiplexing capabilities and rapidity (19). Originally developed by Piepenburg (20), RPA is a sensitive, isothermal DNA-based technology which utilizes primers and recombination proteins to generate DNA amplicons, that can either be visualized by gel electrophoresis or evaluated in real-time using fluorescent probes.
The aim of this study was to utilize RPA for detection of the four main bacterial pathogens associated with BRD, as well as AMR genes and ICE, and to develop multiple real-time RPA assays containing a competitive internal amplification control (IAC) to identify false negatives (21–23). Real-time RPA assays were tested on bovine deep nasopharyngeal swabs (DNPS) collected from cattle at feedlot arrival, to determine accuracy and sensitivity of RPA in comparison to TC-PCR for detection of BRD pathogens, and to its suitability for field-based detection.
The strains used in this study are listed in Table 1. M. haemolytica and P. multocida strains were streaked onto tryptic soy agar containing sheep blood (TSA blood agar; Dalynn Biologicals, Calgary, AB, Canada) and incubated for 24 h at 37°C. H. somni strains were streaked onto TSA blood and incubated for 48 h at 37°C with 5% CO2. M. bovis was cultured by inoculating 1.5 ml pleuropneumonia-like organism broth (PPLO; brain heart infusion broth at 17.5 g per l, yeast extract at 25 g per l, and heat inactivated fetal horse serum at 200 mL per l) with a loop of glycerol stock culture. This starter culture was incubated at 37°C with 5% CO2 for 72–96 h. The entire 1.5 ml starter culture was then added to 30 ml PPLO broth and incubated for an additional 48 h.
DNA was extracted from cultured cells using the DNeasy Blood & Tissue Kit (Qiagen, Toronto, ON, Canada) using the animal tissues spin-column protocol. For M. haemolytica, P. multocida, and H. somni, lysis of the cells was completed in Qiagen tissue lysis (ATL) buffer with proteinase K at 56°C for 3 h, followed by storage at 4°C overnight. The following day the protocol was resumed according to kit instructions with an additional wash buffer 2 (AW2) wash step. For M. bovis, the lysis step was reduced to 2 min and the full protocol was completed without overnight incubation.
Extracted DNA was quantified using PicoGreen on the NanoDrop 3300 Fluorospectrometer (ThermoFisher Scientific, Ottawa, ON, Canada). The DNA was normalized to 10 ng/μl, and then to a 50,000 genome copies/μl stock and stored at −80°C. Calculation of DNA copy numbers per μl was based on the following formula: amount (copies/μl) = [DNA concentration (g/μl)/(bacterial genome length in base pairs × 660)] × 6.02 × 1023. The following genome sizes were used: M. haemolytica 2.6 Mbp, P. multocida 2.3 Mbp, H. somni 2.3 Mbp, and M. bovis 1 Mbp.
Primers and probes were designed using Geneious 8.1.9 (Biomatters Ltd., Newark, NJ, USA) and verified using the NCBI BLAST nucleotide collection (nt/rt) reference sequence database (Table 2). The primers for M. haemolytica (nmaA) were designed for specificity to serotypes A1 and A6 because of their role as causative agents of BRD, while excluding serotype A2, a commensal of the bovine upper respiratory tract (12). Reference sequences used for primer design of each species-specific RPA include: M. haemolytica M42548 nmaA (GenBank: NC_021082.1), H. somni 2336 HS_0116 (GenBank: CP000947.1), P. multocida Kmt1 (GenBank: FJ986389.1), and M. bovis uvrC (GenBank: AF003959.1).
The genomes of five MDR M. haemolytica (MH25, MH30, MH64, MH69, MH76) and one H. somni (HS31) from our collection, as well as the published sequences of P. multocida 36950 ICEPmu1 (GenBank: CP003022.1), M. haemolytica M42548 ICEMh1 (GenBank: NC_021082.1), and H. somni USDA-ARS-USMARC 63374 (GenBank: CP018808.1) were utilized during the design of the ICE RPA assay (Figure 1). While ICEs differ among strains, the presence of tet(H) (conferring tetracycline resistance) was found in 100% of AMR M. haemolytica strains associated with BRD (12). While the tet(H) gene itself is prevalent among genomes of numerous bacterial species, within ICE, tet(H) is located adjacent to a transposase (tnpA) with a conserved sequence among ICE-containing strains of M. haemolytica, P. multocida, and H. somni. Therefore, the ICE RPA was designed to span a region of both tet(H) and tnpA, allowing for specific detection of AMR ICE-containing strains of all three important BRD pathogens (Figure 1).
Figure 1. (A) Genomic comparison of the integrative conjugative element (ICE) regions of H. somni USDA-ARS-USMARC 63374, ICEMh1, ICEPmu1, M. haemolytica MH64, M. haemolytica MH25, and the putative ICE region of M. haemolytica USDA-ARS-USMARC-183. Genes are represented as arrows, with the arrowhead indicating the direction of transcription. Areas between ICEs shaded in light gray indicate regions of ≥67% sequence identity, while areas shaded in dark gray indicate ≥99% sequence identity. (B) Comparison of resistance gene regions 1 and 2 in representative bovine respiratory disease species with alignments to cassettes found in other bacterial species.
RPA reactions were performed in a total volume of 50 μl using the TwistAmp™ Basic Kit (TwistDX, Cambridge, UK). The reaction mixture included 420 nM each primer, 14 mM magnesium acetate, 29.5 μl rehydration buffer, 11.2 μl nuclease-free water, and 2 μl of bacterial DNA. A master mix was prepared containing all reagents except the DNA template and magnesium acetate, and then dispensed into 0.2 ml reaction tubes containing a dry enzyme pellet. Two microliters of DNA was added to each tube, followed by magnesium acetate into the tube lids and the lids were carefully closed. Reaction tubes were then vortexed and briefly centrifuged. Immediately thereafter, the reaction tubes were placed in an Eppendorf PCR thermocycler at 37°C to initiate the reaction. After 2 min the tubes were removed, briefly vortexed, centrifuged and then placed back into the thermocycler for another 28 min. Amplified RPA reactions were purified using the QIAquick PCR Purification Kit (Qiagen, Toronto, ON, Canada) automated on the QIAcube (Qiagen, Toronto, ON, Canada). Following purification, RPA products were electrophoresed on 2% (w/v) agarose gels containing ethidium bromide, and visualized using a fluorescence imager (FluorChem FC2; Alpha Innotech, San Leandro, CA, USA).
Each species-specific RPA assay, including multiplex and real-time assays were screened for inclusivity against 36 representative isolates of each of the four target species (n = 144). The M. haemolytica isolates represented both serotypes A1 and A6 and encompassed 35 different pulsed field gel electrophoresis (PFGE) profiles. Isolates were obtained from lung tissues of BRD mortalities, collected in both Canada and the USA (9, 15, 24). Arising from the same studies, P. multocida and H. somni isolates belonged to 31 and 21 PFGE types, respectively. The M. bovis isolates were collected from the Stanford et al. (15) study and consisted of 27 different PFGE profiles.
A total of 66 bacterial strains (Table 3) belonging to BRD pathogens, closely related species, or other species known to be present in the upper and lower bovine respiratory tract were used to test the specificity of the BRD target RPA assays using the basic kit (Table 2). Bacterial strains were purchased from the American Type Culture Collection (ATCC), Culture Collection University of Gothenburg (CCUG), or obtained from a collaborating laboratory (25). DNA was extracted using the DNeasy Blood & Tissue Kit (Qiagen, Toronto, ON, Canada) with appropriate protocols for Gram positive and Gram negative bacteria.
Similarly for the ICE RPA, reactions were prepared as described above. Specificity of the ICE target was evaluated using the three ICE control strains from our collection (Table 1) as well as an additional 22 sequenced strains (belonging to M. haemolytica, P. multocida and H. somni), 11 with and 11 without ICE.
A multiplex RPA using the TwistAmp™ Basic Kit (TwistDX, Cambridge, UK) was developed for the simultaneous amplification of all four of the BRD pathogens. Reactions were prepared as described (section Species-Specific RPA Assays for BRD Pathogens & ICE) with each of the 8 primers included at 120 nM.
Seven RPA assays were designed for AMR genes (tetH, tetR, msrE, mphE, sul2, floR, erm42). Primers are listed in Table 2 and reactions were prepared using the TwistAmp™ Basic Kit (TwistDX, Cambridge, UK) as described in section Species-Specific RPA Assays for BRD Pathogens & ICE. AMR gene RPA assays were verified using the sequenced strains listed in Table 1 (9).
A competitive internal amplification control (IAC) was designed for use in multiplex real-time RPA and ICE RPA assays so that target primers also amplified the IAC, eliminating the need for additional primers specific for an internal control (Figure 2). Note that only one set of the target primers amplified the IAC, and therefore a positive control is still required as a verification for the other target primer set. The IAC template consisted of a sequenced region unique to Bacillus atrophaeus subsp. globigii (26, 27) containing a binding site for the IAC probe, and flanked by the primer sequences for H. somni, M. haemolytica, and ICE. The IAC was synthesized and inserted into a plasmid vector (pCR2.1) by Eurofins Genomics (Toronto, ON, Canada). The IAC plasmids were transformed into E. coli DH5α cells (Invitrogen, Thermo Fisher Scientific, Waltham, MA, USA). Following plasmid purification using the QIAprep Spin Miniprep Kit (Qiagen, Toronto, ON, Canada), plasmid DNA was quantified by PicoGreen (Invitrogen, Thermo Fisher Scientific, Waltham, MA, USA), normalized to 1 × 108 copies/μl and serially diluted to 5 × 102 copies/μl for use in real-time RPA assays.
Figure 2. (A) Nucleotide sequence of the internal amplification control (IAC), including primer binding sites for H. somni (blue), M. haemolytica (orange), and ICE (green), and the internal control probe binding site (red). Backbone sequence belonging to B. atrophaeus subsp. globigii indicated in black. (B) Schematic representation of the oligonucleotide primer and probe locations on the IAC. (C) Schematic representation of each real-time RPA assay to be used with the IAC.
Three real-time RPA assays were developed: (i) P. multocida and H. somni multiplex, (ii) M. haemolytica and M. bovis multiplex, and (iii) ICE RPA assay (Figure 2C). Real-time RPA was completed using the TwistAmp™ Exo Kit (TwistDX, Cambridge, UK). Reactions for ICE contained 420 nM of each ICE primer, 78 nM ICE probe, 24 nM internal control probe, 14 mM magnesium acetate, 29.5 μl rehydration buffer, 11.3 μl nuclease-free water, 1 × 103 genome copies per reaction internal control plasmid, and 2 μl of bacterial or sample DNA. Multiplex RPA reactions for M. haemolytica and M. bovis were prepared in the same way with the following modifications: 210 nM each primer, 45 nM each of M. haemolytica and M. bovis probe, and 30 nM internal control probe. Finally, for the P. multocida and H. somni multiplex RPA, reactions contained 190 nM P. multocida primers, 230 nM H. somni primers, 42.75 nM P. multocida probe, 52.25 nM H. somni probe, and 25 nM internal control probe, with all other reaction components being the same as for the ICE real-time assay. Reactions were prepared as described in section Species-Specific RPA Assays for BRD Pathogens & ICE with the following modifications: a magnetic bead was dispensed into each reaction tube immediately following the addition of master mix, and reaction tubes were placed in a T16-ISO instrument (TwistDX, Cambridge, UK) at 37°C for 33 min. Positive amplification was asserted when the fluorescence measured over 200 mV for 60 s.
The limit of detection (LOD) was determined for each real-time RPA using dilutions of genomic DNA (ranging from 1 to 1000 genome copies/reaction). Five reactions were prepared per DNA template concentration, with each run repeated 4 times, for a total of 20 reactions per dilution.
The ICE-specific real-time RPA assay, M. haemolytica/M. bovis, and P. multocida/H. somni multiplex real-time assays were tested using 100 DNPS collected from feedlot cattle, which were also screened for BRD pathogens using TC-PCR. Samples were obtained under the supervision of a trained veterinarian and the protocol was reviewed and approved by the Lethbridge Research Center Animal Care Committees in accordance with guidelines of the Canadian Council on Animal Care (28). Consent for sampling of the cattle was also obtained from the owners.
Swabs for RPA testing were selected based on PCR-verified culture data, including those positive for any combination of the four bacterial pathogens as well as samples which were culture negative for all four pathogens. Briefly, DNPS were placed into 1 ml brain heart infusion broth containing 20% glycerol (Dalynn Biologicals, Calgary, AB) and vortexed for 1 min. Methods for TC-PCR detection of M. haemolytica, P. multocida, and H. somni were identical to those described by Stanford et al. (15) with the following modifications: 100 μl of DNPS suspension was plated for M. haemolytica and P. multocida, 50 μl each of undiluted DNPS suspension and 10−1 dilution were plated for H. somni and incubated for 48 h. Methods for TC-PCR detection of M. bovis were completed as described by Andrés-Lasheras et al. (29). DNA was obtained from a 300 μl aliquot of DNPS suspension using the DNeasy Blood and Tissue kit (Qiagen, Toronto, ON, Canada). RPA reaction mixtures contained primers and probes at concentrations described in section Real-time RPA Assays, with 10 μl DNA sample, and 1.3 μl nuclease-free water.
The LOD values for each RPA at a probability of detection of 95% were estimated by Probit regression analysis using Microsoft Excel (2016). Results of real-time, multiplex RPA and TC-PCR were compared by measuring the degree of agreement and kappa coefficient (k) (Table 4).
Table 4. Comparison of traditional culture - PCR (T-PCR) and recombinanse polymerase amplificaton (RPA) for detection of bovine respiratory disease pathogens in deep nasopharyngeal swab samples.
Using the TwistAmp™ Basic kit, RPA assays were optimized for ICE and each BRD species individually (M. haemolytica, P. multocida, H. somni, and M. bovis), as well as being used in a conventional multiplex containing all four BRD targets (Figure 3). RPA assays demonstrated 100% inclusivity and analytical specificity, as all 36 strains of each species were successfully identified in each species-specific RPA assay, and the 5 target strains were successfully detected (Table 3), while none of the 61 non-target strains were detected. Additionally, seven single-plex RPA assays were developed for AMR genes (tetH, tetR, msrE, mphE, sul2, floR, erm42). Positive and negative amplification was verified for each AMR gene assay using sequenced AMR strains (data not shown).
Figure 3. Amplification by multiplex recombinase polymerase amplification for all 4 bovine respiratory disease pathogens, H. somni, M. haemolytica, M. bovis, and P. multocida at 5 × 102 genome copies. NTC, no template control.
The real-time multiplex RPA assays are shown in Figures 4A,B, for P. multocida/H. somni and M. haemolytica/M. bovis, respectively. Each assay contained the IAC and the LOD was 161 and 40 genome copies, respectively, for P. multocida/H. somni and M. haemolytica/M. bovis assays. As few as 103 and 7 genome copies, could be detected in 50% of cases for P. multocida/H. somni and M. haemolytica/M. bovis, respectively.
Figure 4. Graphs depicting recombinase polymerase amplification (RPA) over time of 2 × 102 genome copies for (A) P. multocida and H. somni multiplex, (B) M. haemolytica and M. bovis multiplex, and (C) ICE RPA. Each assay included the internal amplification control (1 × 103 genome copies) designed in this study.
Figure 4C shows the real-time RPA assay for a region of the ICE specific to M. haemolytica, P. multocida, and H. somni, along with the IAC. The LOD for the ICE RPA was 134 genome copies per reaction (95% confidence interval). In 50% of cases, as few as 97 genome copies per reaction could be detected. Figure 5A illustrates the real-time RPA amplification of ICE using decreasing concentrations of genomic DNA template (1 × 104 to 1 × 102 copies/reaction).
Figure 5. (A) The recombinase polymerase amplification over time of ICE at decreasing genome copies (M. haemolytica MH44). (B) Example of ICE amplification from bovine nasal swabs, and (C) amplification of internal amplification control (1 × 103 genome copies) in bovine nasal swabs.
Bovine DNPS samples (n = 100) were screened for ICEs and BRD pathogens using the ICE RPA and real-time multiplex RPA assays for BRD pathogens. RPA results were compared to data collected by TC-PCR for each BRD species. Figure 5B shows an example of the amplification results of the ICE RPA using DNPS samples collected from individual cattle upon arrival at the feedlot. The IAC successfully amplified in DNPS reactions (Figure 5C). Based on TC-PCR data, among the 100 bovine DNPS swabs selected for this study, each contained 0 to 4 of the selected members of the bacterial BRD complex, denoting a total of 131 instances of BRD pathogens. RPA exhibited 81% agreement (kappa coefficient, k = 0.611) with the TC-PCR data, while in an additional 36 instances, pathogens were detected by RPA, and in 40 instances detected by TC-PCR only (Table 4). The results showed that RPA had a positive rate that was similar to that of TC-PCR (Table 4), with detection of M. bovis and H. somni being higher by RPA, and M. haemolytica and P. multocida lower by RPA than as result of culture from DNPS. Positive rates were as follows, for TC-PCR vs. RPA, respectively: 43 vs. 34% for M. haemolytica, 46 vs. 58% for M. bovis, 47 vs. 35% for P. multocida, and 35 vs. 40% for H. somni. Agreement of RPA with culture data for P. multocida was 74% (k = 0.470), H. somni was 79% (k = 0.553), M. bovis was 84% (k = 0.684), and M. haemolytica was 87% (k = 0.728). Results in which RPA either agreed with or exceeded pathogen detection over culture methods accounted for 81, 89, 92, and 98% of cases for P. multocida, M. haemolytica, H. somni, and M. bovis, respectively. ICE was detected in 55% (n = 55) of the bovine nasal swabs tested. Of the swabs positive for ICE, 91% (n = 50) were also positive for one or more of the BRD-associated pathogens by RPA and/or TC-PCR.
In this study, RPA assays were developed to detect four bacterial BRD pathogens (M. haemolytica, M. bovis, H. somni, and P. multocida), seven AMR genes, and a region of ICE associated with BRD pathogens. Furthermore, detection of M. haemolytica was specific to serotypes A1 and A6, those most commonly associated with disease, while excluding all other serotypes, including A2 a common bovine commensal (30, 31). Beker et al. (13) developed a multiplex PCR assay targeting four conserved core genes required for integration and maintenance of ICE structures within the Pasteurellaceae family and demonstrated relevance of this assay to detecting these elements in P. multocida and M. haemolytica (13). Furthermore, RPA has recently been utilized for detection of P. multocida in cattle (32). However, to our knowledge, this is the first study to develop and apply RPA for detecting four major bacterial BRD pathogen species in multiplex and real-time formats, and BRD pathogen-associated with ICEs in bovine DNPS.
A conventional multiplex RPA assay was designed using the TwistAmp™ basic kit for simultaneous amplification of the four major BRD bacterial species. While this assay is useful for verification of presumptive positive isolates identified from culture methods in a laboratory setting, all RPA assays using the TwistAmp™ basic kit require post-amplification clean up to remove excess proteins, and gel electrophoresis for visualization of amplified products, a procedure not easily achieved outside of a laboratory (33). In an effort to develop RPA assays for use in the field, RPA assays were modified for real-time detection using the TwistAmp™ Exo kit and T16-ISO instrument (TwistDX, Cambridge, UK). In comparison to real-time PCR, the RPA instrument cannot run as many reactions at a time, nor are the results quantitative. However, results are achieved within 20–30 min vs. 1.5–2 h with real-time PCR. The procedure exhibits similar sensitivity, and the instrument is substantially smaller and less expensive than a real-time PCR machine making it more suitable for a field application (33).
A real-time RPA assay for ICEs and two multiplex real-time RPAs were developed, each containing a competitive IAC. The addition of an IAC has been shown to avoid false-negatives (22, 23, 34). As opposed to a non-competitive IAC, a competitive IAC is co-amplified simultaneously with the target by the same primer set (23). By using a competitive IAC, the target and IAC are amplified by the same primers under the same conditions, reducing the need for an additional primer set, maximizing the quantity of the target primer. A competitive IAC also reduces the risk of undesirable interactions among the target primers and an additional control primer set (23). A limitation of this approach is the requirement for exogenous synthetic DNA.
The LOD was 161 and 40 genome copies per reaction for P. multocida/H. somni and M. haemolytica/M. bovis assays, respectively, and 134 genome copies for ICE. Limits of detection were similar to other published RPA and multiplex RPA assays (27, 32, 35, 36). Sensitivity of RPA depends greatly on primer and probe design, but design software and recommendations are currently lacking (19). As a result, several RPA primer and probe sets must be screened in order to determine the optimal combination (19). Multiplexing offers additional challenges, as competition among primer sets for recombinase proteins can result one target preventing the amplification of another (37).
The real-time RPA assay for ICEs amplified a region conserved among three of the four BRD pathogens targeted (M. haemolytica, H. somni, and P. multocida). An ICE is a mobile genetic element, transferred via conjugation between bacteria of the same or different species (9). ICEs may differ among species as well as within strains of the same species, containing as few as 1 to as many as 12 or more AMR genes (12). The gene tet(H), responsible for resistance to tetracycline has been associated with plasmids and chromosomal DNA, and also on a transposon-like element of P. multocida known as Tn5706 (38). The presence of tet(H) in ICEs is frequent among AMR M. haemolytica, H. somni, and P. multocida strains (12, 14, 39, 40). Within the ICE, tet(H) is located directly next to a transposase (tnpA) with a conserved sequence among ICE-containing strains of M. haemolytica, P. multocida and H. somni. Furthermore, tet(H) has only been reported in members of the Pasteurellaceae (39). Therefore, the ICE RPA was designed to span a region of both tet(H) and tnpA allowing for specific detection of three of the bacterial BRD bacterial pathogen that can potentially harbor AMR-ICE.
The bovine DNPS used in this study were collected from cattle upon arrival at the feedlot. Arrival at the feedlot is a particularly stressful period for cattle, which often involves transportation over long distances, and comingling of cattle, increasing transmission of BRD agents among members of the herd (6). While traditional culture methods are the standard for confirmation of BRD infection, they are not without limitations. Bovine nasal swabs inoculated onto agar plates can easily become overgrown by non-target bacteria, making it difficult to visually identify and isolate target species. Of the four bacterial BRD pathogens, P. multocida and M. haemolytica are most easily identified on the basis of morphology, however this approach is highly subjective. While H. somni also has a distinct morphology, it is difficult to culture and is easily overgrown as it requires twice the incubation period of P. multocida and M. haemolytica (16). M. bovis is even more challenging to culture as it requires a significantly longer to grow than other BRD pathogens, and must be cultured under humidified, microaerophilic conditions (16).
Detection of BRD species using multiplex real-time RPA showed a strong correlation with TC-PCR (90%). A greater number of swabs containing M. bovis and H. somni were detected by RPA than by TC-PCR, likely due to the aforementioned challenges associated with culturing these species in the laboratory. In contrast, fewer swabs were identified containing M. haemolytica and P. multocida by RPA than by TC-PCR. Likely, this is due to the ease with which these two species are cultured, and their distinct morphologies on laboratory media, aiding identification even when cell numbers are low. Culture-positive results for serotype A2 during TC-PCR were excluded as a positive result for M. haemolytica during data interpretation, and therefore is not a reason for the lower detection by RPA. However, RPA identified the presence of ~10% more bacterial pathogens (36 instances) in swabs than TC-PCR, reflecting the greater sensitivity of RPA over traditional culture methods.
The ICE RPA assay was utilized to screen DNPS, because unlike the AMR gene RPA assays, this particular target is specific to all three BRD bacterial species, while also serving as an indicator of AMR and potential MDR. ICE was detected among 55% (n = 55) of the nasal swabs tested in this study. No BRD pathogens were detected in 9% of ICE-positive DNPS samples. Due to the transmissible nature of ICE, this suggests that BRD pathogens may be transferring ICE to other bacterial species (13, 41). A closely related species, Bibersteinia trehalosi, as well as Moraxella and Acinetobacter may also contain ICE (9, 31).
In this study, RPA was demonstrated to be a useful technology for detection of BRD pathogens and ICE from bovine nasal swabs. Advantages of RPA over polymerase chain reaction (PCR) and other isothermal technologies include simplified instrumentation amenable for field-based studies and reduced costs (19). Furthermore, detection by RPA is sensitive, and results can be obtained in real-time in <30 min (19). Similar to other molecular based techniques, detecting the AMR profile of BRD agents by RPA does not eliminate the need for culture methods. However, conventionally, it takes 2–5 days to confirm identity of BRD agents in a laboratory setting whereas RPA can accomplish this same feat in 1–2 h. Furthermore, RPA is more tolerant to inhibitors and background DNA than PCR (33). The robustness of RPA in the presence of traditional inhibitors facilitates amplification from crude extracts, which is not achievable using PCR (37).
Diagnosis of BRD in live cattle remains difficult, since there is no gold standard to define a BRD infection (2). Because many of the BRD pathogens are also commensals, their presence alone is not necessarily an indicator of disease without other predisposing environmental factors, physiologic stressors, or concurrent (viral) infections (6, 42). This affects the ability to accurately evaluate methods or technologies for diagnosis of BRD (2). A greater understanding of the virulence mechanisms of the infecting bacteria and pathogenesis is needed (6).
Further research is required to optimize RPA technology for BRD detection in the feedlot. Specifically, a method for obtaining a high yield and quality of nucleic acids from bovine nasal swabs without the use of a commercial kit will be required. Further refinement of RPA assays to enhance sensitivity and multiplexing capability would also be beneficial. Finally, a deeper understanding of the gene mechanisms associated with virulence and antimicrobial resistance of BRD pathogens may lead to identification of additional signature genes to further improve the utility of RPA.
RPA is a sensitive, specific and accurate method which detected 4 major BRD bacterial agents in deed nasal swabs collected from feedlot cattle. Furthermore, RPA was capable of detecting ICE from MDR M. haemolytica, P. multocida, and H. somni strains, which may contribute to dissemination of AMR and virulence genes among BRD pathogens. As compared to conventional approaches for detecting BRD pathogens, RPA is affordable, fast, and easily modified for real-time field-based detection. Further studies are required to evaluate performance of RPA in field settings. Additional study linking detected pathogens to clinical BRD as well as signature genes responsible for AMR profiles would enable RPA-guided selection of effective antimicrobial treatments by the beef industry, reducing antimicrobial usage by minimizing the need for repeated treatments due to AMR.
All datasets generated for this study are included in the article/supplementary material.
The animal study was reviewed and approved by Lethbridge Research Centre Animal Care Committees in accordance with guidelines of the Canadian Council on Animal Care (2009). Written informed consent was obtained from the owners for the participation of their animals in this study.
YN, KS, TA, SC, BR, and TM conceived and designed the study. RD, MB, MGB, and KA provided expertise in RPA technology and assisted in design and development of RPA assays. CC performed laboratory activities. RZ and CC worked on analysis of the sequencing data. CC performed other statistical analyses and wrote the first draft of the manuscript. All authors revised the manuscript and gave approval for the final version to be published.
This project was funded by the Alberta Beef Producers (ANH.06.16), Alberta Livestock and Meat Agency. Funders had no role in the design, analysis or interpretation of the experiments or in the development of the assays.
The authors declare that the research was conducted in the absence of any commercial or financial relationships that could be construed as a potential conflict of interest.
The authors would like to thank Michele Anholt and the veterinary practices involved in providing the bovine nasal swabs, Tim Janzen at the Animal Diseases Research Institute for technical assistance, Dr. Cassidy Klima for expertise and advice, and Dr. Andrew Cameron for support with figure design software. The authors would also like to thank the laboratory team at the Lethbridge Research and Development Center: Reuben Ha, Dr. Sara Andres-Lasheras, Ruth Barbieri, Catrione Lee, and Brendon DeGroot for culturing BRD pathogens from DNPS.
1. Lubbers BV, Turnidge J. Antimicrobial susceptibility testing for bovine respiratory disease: Getting more from diagnostic results. Vet J. (2015) 203:149–54. doi: 10.1016/j.tvjl.2014.12.009
2. Wolfger B, Timsit E, White BJ, Orsel K. A systematic review of bovine respiratory disease diagnosis focused on diagnostic confirmation, early detection, and prediction of unfavorable outcomes in feedlot cattle. Vet Clin N Am- Food A. (2015) 31:351–65. doi: 10.1016/j.cvfa.2015.05.005
3. Hilton WM. BRD in 2014: where have we been, where are we now, and where do we want to go? Anim Health Res Rev. (2014) 15:120–2. doi: 10.1017/S1466252314000115
4. Cameron A, Mcallister TA. Antimicrobial usage and resistance in beef production. J Anim Sci Biotechnol. (2016) 7:68. doi: 10.1186/s40104-016-0127-3
5. Whiteley LO, Maheswaran SK, Weiss DJ, Ames TR, Kannan MS. Pasteurella haemolytica A1 and bovine respiratory disease: pathogenesis. J Vet Intern Med. (1992) 6:11–22. doi: 10.1111/j.1939-1676.1992.tb00980.x
6. Griffin D, Chengappa MM, Kuszak J, Mcvey DS. Bacterial pathogens of the bovine respiratory disease complex. Vet Clin N Am-Food A. (2010) 26:381–94. doi: 10.1016/j.cvfa.2010.04.004
7. Grissett GP, White BJ, Larson RL. Structured literature review of responses of cattle to viral and bacterial pathogens causing bovine respiratory disease complex. J Vet Intern Med. (2015) 29:770–80. doi: 10.1111/jvim.12597
8. Murray GM, O'neill RG, More SJ, Mcelroy MC, Earley B, Cassidy JP. Evolving views on bovine respiratory disease: an appraisal of selected key pathogens – Part 1. Vet J. (2016) 217:95–102. doi: 10.1016/j.tvjl.2016.09.012
9. Klima CL, Zaheer R, Cook SR, Booker CW, Hendrick S, Alexander TW, et al. Pathogens of bovine respiratory disease in North American feedlots conferring multidrug resistance via integrative conjugative elements. J Clin Microbiol. (2014) 52:438–48. doi: 10.1128/JCM.02485-13
10. Tennant TC, Ives SE, Harper LB, Renter DG, Lawrence TE. Comparison of tulathromycin and tilmicosin on the prevalence and severity of bovine respiratory disease in feedlot cattle in association with feedlot performance, carcass characteristics, and economic factors. J Anim Sci. (2014) 92:5203–13. doi: 10.2527/jas.2014-7814
11. Murray GM, O'neill RG, More SJ, Mcelroy MC, Earley B, Cassidy JP. Evolving views on bovine respiratory disease: an appraisal of selected control measures – Part 2. Vet J. (2016) 217, 78–82. doi: 10.1016/j.tvjl.2016.09.013
12. Clawson ML, Murray RW, Sweeney MT, Apley MD, Dedonder KD, Capik SF, et al. Genomic signatures of Mannheimia haemolytica that associate with the lungs of cattle with respiratory disease, an integrative conjugative element, and antibiotic resistance genes. BMC Genomics. (2016) 17:982. doi: 10.1186/s12864-016-3316-8
13. Beker M, Rose S, Lykkebo CA, Douthwaite S. Integrative and conjugative elements (ICEs) in Pasteurellaceae species and their detection by multiplex PCR. Front Microbiol. (2018) 9:1329. doi: 10.3389/fmicb.2018.01329
14. Bhatt K, Timsit E, Rawlyk N, Potter A, Liljebjelke K. Integrative conjugative element ICEHs1 encodes for antimicrobial resistance and metal tolerance in Histophilus somni. Front Vet Sci. (2018) 5:153. doi: 10.3389/fvets.2018.00153
15. Stanford K, Zaheer R, Klima C, McAllister TA, Peters D, Niu D, et al. Antimicrobial resistance in members of the bacterial bovine resipiratory disease complex isolated from the lung tissue of cattle mortlities manged with or without the use of antimicrobials. Microorganisms. (2019) 8:288. doi: 10.3390/microorganisms8020288
16. Nicholas R, Baker S. Recovery of mycoplasmas from animals. In: Miles R and Nicholas R, editors. Mycoplasma Protocols. Totowa, NJ: Humana Press (1998). p. 37–43. doi: 10.1385/0-89603-525-5:37
17. Angen Ø, Ahrens P, Tegtmeier C. Development of a PCR test for identification of Haemophilus somnus in pure and mixed cultures. Vet Microbiol. (1998) 63:39–48. doi: 10.1016/S0378-1135(98)00222-3
18. Cusack P, Mcmeniman N, Lean I. The medicine and epidemiology of bovine respiratory disease in feedlots. Aust Vet J. (2003) 81:480–7. doi: 10.1111/j.1751-0813.2003.tb13367.x
19. Daher RK, Stewart G, Boissinot M, Bergeron MG. Recombinase polymerase amplification for diagnostic applications. Clin Chem. (2016) 62:947–58. doi: 10.1373/clinchem.2015.245829
20. Piepenburg O, Williams CH, Stemple DL, Armes NA. DNA detection using recombination proteins. PLOS Biol. (2006) 4:e204. doi: 10.1371/journal.pbio.0040204
21. Hoorfar J, Cook N, Malorny B, Wagner M, De Medici D, Abdulmawjood A, et al. Making internal amplification control mandatory for diagnostic PCR. J Clin Microbiol. (2003) 41:5835–5835. doi: 10.1128/JCM.41.12.5835.2003
22. Higgins O, Clancy E, Forrest MS, Piepenburg O, Cormican M, Boo TW, et al. Duplex recombinase polymerase amplification assays incorporating competitive internal controls for bacterial meningitis detection. Anal Biochem. (2018) 546:10–6. doi: 10.1016/j.ab.2018.01.016
23. Yang H-L, Wei S, Gooneratne R, Mutukumira AN, Ma X-J, Tang S-Z, et al. Development of a recombinase polymerase amplification assay for Vibrio parahaemolyticus detection with an internal amplification control. Can J Microbiol. (2018) 64:223–30. doi: 10.1139/cjm-2017-0504
24. Anholt RM, Klima C, Allan N, Matheson-Bird H, Schatz C, Ajitkumar P, et al. Antimicrobial susceptibility of bacteria that cause bovine respiratory disease complex in Alberta, Canada. Front Vet Sci. (2017) 4:207. doi: 10.3389/fvets.2017.00207
25. Timsit E, Workentine M, Van Der Meer F, Alexander T. Distinct bacterial metacommunities inhabit the upper and lower respiratory tracts of healthy feedlot cattle and those diagnosed with bronchopneumonia. Vet Microbiol. (2018) 221:105–13. doi: 10.1016/j.vetmic.2018.06.007
26. Picard FJ, Gagnon M, Bernier MR, Parham NJ, Bastien M, Boissinot M, et al. Internal control for nucleic acid testing based on the use of purified Bacillus atrophaeus subsp. globigii spores. J Clin Microbiol. (2009) 47:751–7. doi: 10.1128/JCM.01746-08
27. Daher RK, Stewart G, Boissinot M, Bergeron MG. Isothermal recombinase polymerase amplification assay applied to the detection of group B Streptococci in vaginal/anal samples. Clin Chem. (2014) 60:660–6. doi: 10.1373/clinchem.2013.213504
28. Canadian Council on Animal Care. Guide to the Care and Use of Farm Animals in Research, Teaching and Testing (2009). Available online at: https://www.ccac.ca/en/training/modules/farm-animals-stream.html
29. Andrés-Lasheras S, Zaheer R, Ha R, Lee C, McAllister TA. A direct qPCR screening approach to improve the efficiency of Mycoplasma bovis isolation in the frame of a broad surveillance study. J. Microbiol. Methods. (2019) 169:105805. doi: 10.1016/j.mimet.2019.105805
30. Rice JA, Carrasco-Medina L, Hodgins DC, Shewen PE. Mannheimia haemolytica and bovine respiratory disease. Anim Health Res Rev. (2007) 8:117–28. doi: 10.1017/S1466252307001375
31. Klima CL, Cook SR, Zaheer R, Laing C, Gannon VP, Xu Y, et al. Comparative genomic analysis of Mannheimia haemolytica from bovine sources. PLoS ONE. (2016) 11:e0149520. doi: 10.1371/journal.pone.0149520
32. Zhao G, He H, Wang H. Use of a recombinase polymerase amplification commercial kit for rapid visual detection of Pasteurella multocida. BMC Vet Res. (2019) 15:154. doi: 10.1186/s12917-019-1889-6
33. Li J, Macdonald J, Von Stetten F. Review: a comprehensive summary of a decade development of the recombinase polymerase amplification. Analyst. (2019) 144:31–67. doi: 10.1039/C8AN01621F
34. Daher RK, Stewart G, Boissinot M, Boudreau DK, Bergeron MG. Influence of sequence mismatches on the specificity of recombinase polymerase amplification technology. Mol Cell Probes. (2015) 29:116–21. doi: 10.1016/j.mcp.2014.11.005
35. Kersting S, Rausch V, Bier FF, Von Nickisch-Rosenegk M. Multiplex isothermal solid-phase recombinase polymerase amplification for the specific and fast DNA-based detection of three bacterial pathogens. Mikrochim Acta. (2014) 181:1715–23. doi: 10.1007/s00604-014-1198-5
36. Crannell Z, Castellanos-Gonzalez A, Nair G, Mejia R, White AC, Richards-Kortum R. Multiplexed recombinase polymerase amplification assay to detect intestinal protozoa. Anal Chem. (2016) 88:1610–6. doi: 10.1021/acs.analchem.5b03267
37. Lobato IM, O'sullivan CK. Recombinase polymerase amplification: basics, applications and recent advances. Trends Anal Chem. (2018) 98:19–35. doi: 10.1016/j.trac.2017.10.015
38. Kehrenberg C, Werckenthin C, Schwarz S. Tn5706, a transposon-like element from Pasteurella multocida mediating tetracycline resistance. Antimicrob Agents Chemother. (1998) 42:2116–8. doi: 10.1128/AAC.42.8.2116
39. D'amours GH, Ward TI, Mulvey MR, Read RR, Morck DW. Genetic diversity and tetracycline resistance genes of Histophilus somni. Vet Microbiol. (2011) 150:362–72. doi: 10.1016/j.vetmic.2011.02.051
40. Michael GB, Kadlec K, Sweeney MT, Brzuszkiewicz E, Liesegang H, Daniel R, et al. ICEPmu1, an integrative conjugative element (ICE) of Pasteurella multocida: analysis of the regions that comprise 12 antimicrobial resistance genes. J Antimicrob Chem. (2012) 67:84–90. doi: 10.1093/jac/dkr406
41. Dedonder KD, Apley MD. A literature review of antimicrobial resistance in Pathogens associated with bovine respiratory disease. Anim Health Res Rev. (2015) 16:125–34. doi: 10.1017/S146625231500016X
Keywords: recombinase polymerase amplification, bovine respiratory disease, antimicrobial resistance, integrative conjugative element, competitive internal amplification
Citation: Conrad CC, Daher RK, Stanford K, Amoako KK, Boissinot M, Bergeron MG, Alexander T, Cook S, Ralston B, Zaheer R, Niu YD and McAllister T (2020) A Sensitive and Accurate Recombinase Polymerase Amplification Assay for Detection of the Primary Bacterial Pathogens Causing Bovine Respiratory Disease. Front. Vet. Sci. 7:208. doi: 10.3389/fvets.2020.00208
Received: 21 January 2020; Accepted: 30 March 2020;
Published: 22 April 2020.
Edited by:
Xin Zhao, McGill University, CanadaReviewed by:
Faten Abdelaal Okda, St. Jude Children's Research Hospital, United StatesCopyright © 2020 Conrad, Daher, Stanford, Amoako, Boissinot, Bergeron, Alexander, Cook, Ralston, Zaheer, Niu and McAllister. This is an open-access article distributed under the terms of the Creative Commons Attribution License (CC BY). The use, distribution or reproduction in other forums is permitted, provided the original author(s) and the copyright owner(s) are credited and that the original publication in this journal is cited, in accordance with accepted academic practice. No use, distribution or reproduction is permitted which does not comply with these terms.
*Correspondence: Yan D. Niu, ZG9uZ3lhbi5uaXVAdWNhbGdhcnkuY2E=; Tim McAllister, dGltLm1jYWxsaXN0ZXJAY2FuYWRhLmNh
Disclaimer: All claims expressed in this article are solely those of the authors and do not necessarily represent those of their affiliated organizations, or those of the publisher, the editors and the reviewers. Any product that may be evaluated in this article or claim that may be made by its manufacturer is not guaranteed or endorsed by the publisher.
Research integrity at Frontiers
Learn more about the work of our research integrity team to safeguard the quality of each article we publish.