- Austrian Space Forum, Spacesuit Laboratory, Innsbruck, Austria
Mars and Moon analog field missions are established tools to investigate the potential of instruments, workflows, materials, and human factors for characterizing the astrobiological potential and geoscientific context of planetary surfaces. Historically, there is a broad spectrum on both the scientific focus and the performance parameters for analog missions. This applies specifically where performance parameters of coordinated deployment of mission assets (e.g., rovers, human crewmembers, or scientific instruments) are studied. We argue that scientific priorities and workflows shall be consolidated at an early planning stage of deep space missions such as during phase-0 or phase-A studies, while they can still impact the mission architecture design process. It is to be expected that a human-robotic mission to Mars or the Moon will include multiple field assets such as human explorers, robotic vehicles including aerial reconnaissance, mobility assets, habitat modules, stationary instruments, and engineering elements for power, communication, and in-situ resource utilization. These require more complex asset coordination compared to single-rover planetary missions. Therefore, we advocate an “Exploration Cascade,” which helps to manage these multiple assets to optimize the scientific return of planetary surface missions, to search for extinct and/or extant traces of life, and to characterize the geoscientific context of the sites of interest.
Introduction
Analog Missions as a Training Tool
Human-robotic Mars missions are likely to be launched within the next 2–3 decades. Some of these missions will include surface sojourns with at least 1 month in duration (Davila et al., 2010; Drake et al., 2010). Analog field campaigns have supported all previous planetary surface missions so far (Preston and Dartnell, 2014) and contributed to planetary missions such as NASA-MSL's Curiosity Rover (e.g., Grotzinger et al., 2015; Kah and MSL Science Team, 2015) and ESA's ExoMars (Vago et al., 2018). It is generally understood that astrobiology constitutes one of the primary scientific drivers for such missions (e.g., Belz et al., 2014; Domagal-Goldman et al., 2016; Fairén et al., 2019). Analog studies are generally considered an efficient tool to prepare for future Mars missions, complementing instrument validation campaigns. Various Mars and lunar analogs on Earth are used to train for scientific operations on planetary surfaces, or study extraterrestrial processes (e.g., Preston and Dartnell, 2014, also see Figure 1). Additionally, they help to enlighten the logistics and workflow-related aspects, focusing on human factors, engineering (e.g., human-robotic interaction) constraints, as well as safety considerations.
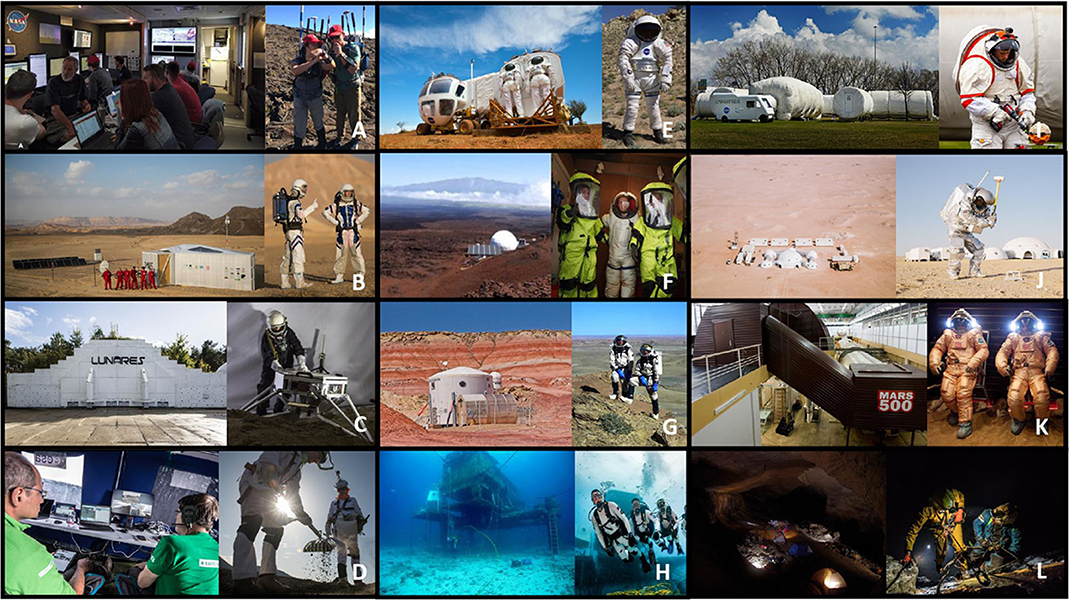
Figure 1. Examples of analog missions. (A) NASA BASALT (USA), (B) D-MARS (Israel), (C) LUNARES (Poland), (D) ESA/PANGEA (Spain), (E) NASA/D-RATS (USA), (F) HI-SEAS (USA), (G) Mars Desert Research Station (USA), (H) NASA/NEEMO underwater station (USA), (I) NDU Habitat Demonstrator (USA), (J) OeWF/AMADEE-program (Austria, Oman), (K) Mars-500 (Russia), and (L) ESA/CAVES (Spain/Italy). Image credits: (A) photos with permission from Zara Mirmalek, (B) photos with permission from Hilel Rubinstein/D-MARS, (C) photos with permission from Anna B. Gregorczyk, (D) photos from permission from ESA/S. Sirios, (E) photos NASA, (F) photos with permission from Ross Lockwood, (G) photos with permissions from OeWF, (H) photos: NASA/Florida International University, (I) photos with permission from Pablo de Leon, University of North Dakota, (J) photos with permission from OeWF, (K) photos: Roskosmos, (L) photos: European Space Agency.
Notably, a representative metasearch using the Google-Scholar database on the number of publications focusing on the keyword “Mars analog research” yields an increase from 611 results in 1997, to 1,890 in 2007, to 3,150 in 2017 (similar increases were also observed on bibliographic databases NASA ADS and Pubmed), indicating the emergence of a new scientific field. Some examples of past analog campaigns include the NASA DESERT-RATS field campaigns conducted between 1997 and 2010 (Abercromby et al., 2013), the MOONWALK project (Imhof et al., 2015, 2017), the ESA CAVES missions (Bessone et al., 2015), the NASA HI-SEAS long-duration missions (Häuplik-Meusburger et al., 2017), an initiative by the UK Centre for Astrobiology: the subsurface analog research MINAR (Payler et al., 2017), ESA PANGEA (Bessone et al., 2018), the NASA BASALT campaigns (Lim et al., 2019), or numerous stand-alone expeditions studying highly specific astrobiological questions (e.g., Schulze-Makuch et al., 2018) and others. Notably, in 2011, the European Space Agency (ESA) created a topical team to investigate recent analog activities (Martins et al., 2017) by using the Earth as a tool for studying astrobiology, and to formulate inputs and scientific needs for the improvement of ground-based astrobiological research. The outcomes and lessons learned from these and other analog missions constitute the building blocks for OeWF's field campaigns.
Besides these professional programs, there are relevant studies performed at grassroots, mixed professional/citizen-science or outreach-focused analog sites, such as the Mars Desert Research Station MDRS in Utah (Kobrick et al., 2018), or NASA's Spaceward Bound Program (Allner et al., 2010; Rask et al., 2011).
Until now, the Austrian Space Forum (OeWF, German: Österreichisches Weltraum Forum) has conducted 12 Mars analog field campaigns, as part of the PolAres (2006–2017) (Groemer, 2009) and the subsequent AMADEE Program (since 2018). These missions included more than 750 h of simulated EVA (extravehicular activity) operations and the performance of more than 100 peer-review selected experiments. The aim of these initiatives is to identify research gaps in the exploration roadmaps such as the Global Exploration Roadmap (GER) (Crawford, 2014) of the International Space Exploration Coordination Group (ISECG), the NASA Mars Reference Architecture (DRM 5.0) (Drake, 2009), the Mars Exploration Robotic Program (MREP) of ESA (Geelen et al., 2013), as well as NASA's upcoming ARTEMIS program (Chavers et al., 2019). These programmatic roadmaps facilitated the AMADEE program assumptions in terms of projected crew composition, primary scientific objectives, and mission architectural considerations.
Human Element Controversies and Science as an Early Mission Design Driver
It could be argued that robotic exploration is a more efficient tool than human explorers to study planetary surfaces concerning the scientific aspect (as opposed to policy-driven programs like Apollo). However, by looking into the exploration efficiency of current and planned robotic missions, it becomes evident that a human component adds significantly to the throughput of, e.g., sample selection, in-situ analysis, and procurement workflows (Crawford, 2012). Glass and Briggs (2003) demonstrated in a field test at Devon Island that humans can be up to 25 times more scientifically productive than a rover under certain conditions. Another concern is the planetary protection aspect, as addressed by the respective COSPAR recommendations (Kminek et al., 2010), which requires a high level of cleanliness at the target site or even access denial to the restricted areas. However, this argument applies also to robotic elements, whereas the amount of contamination (despite proper cleaning efforts on Earth) increases with the mass of the rovers. Hence—besides ethical and societal arguments (e.g., Dunér et al., 2018; Szocik, 2019)—we argue that the expected benefits of including human explorers outweigh the risks from a primarily scientific perspective.
The bandwidth of analog missions ranges from non-scientific initiatives with a focus on education, like the Chinese Plan-C station in the northwestern Gobi desert, NASA's Spaceward Bound Program which aims to train the next generation of space explorers by hosting students and teachers within some analogs (see above for the references), and also highly focused projects like the NASA D-RATS (Abercromby et al., 2013) or BASALT missions (Lim et al., 2019), complemented by laboratory-type analog studies (e.g., the Russian MARS-500 study; Ushakov et al., 2014). In addition to that, analog missions vary in the complexity of their scope. On the one end of the spectrum there are direct instrument field validations and field data acquisitions pertinent to geoscientific workflows such as the AMASE expeditions verifying the performance of the ExoMars PanCam (Amundsen et al., 2010).
On the other side it features more complex surface-sojourn suites of experiments, including human-factors and science tactical decision making, such as the D-RATS studies (Litaker and Howard, 2013). Notably, one of the underground campaigns, MINAR 2017's main focus is to carry out not only geoscientific investigations and instrumental operations under planetary deep subsurface conditions but also to develop technologies for the mining industry (Payler et al., 2017). Therefore, due to the variety in campaign goals, analog missions do not always represent a realistic projection of planetary surface activities. For instance, only a few of them (such as D-RATS, BASALT, or AMADEE) include operational remote science support teams mimicking a major design driver for surface operations (e.g., Groemer et al., 2014, 2016): the modality of the decision-making process, including constraints such as time-delay, bandwidth-limitation, and segregated expertise. Hence, many operational lessons learned of analog missions might be challenging to implement in future flight missions.
We argue that the planetary surface operations—once mission safety criteria have been met—focusing on the astrobiological and geoscientific performance indicators, should be represented as an early-stage design driver for mission architectures. Current exploration frameworks, for instance, the NASA ARTEMIS program, at first define the engineering border conditions, including spacesuit designs, the Deep Space Gateway infrastructure, etc., and then the science objectives are identified. Long lead times in developing deep space infrastructure tend to be the first step in developing architecture and traditionally, science is involved at a later point. So, for industrial policy cycle considerations, capacity building needs to start prior to the surface mission science being consolidated.
However, we suggest that in contrast to the Apollo missions, a science-first principle will ultimately lead to a more effective mission architecture. For instance, the Mars Sample Return project IMOST exemplifies how significant it is to involve detailed science work combined with engineering (Beaty et al., 2018, 2019a,b). Hence, we favor a scientific consensus-building before committing to specific mission architectures. Having multiple disciplines represented over multiple missions, testing various instrumentation, facilitates the establishment of “common trunk infrastructures.” Those provide the required technological and operational baselines that provide high flexibility for accommodating experiments and technologies.
Research Gaps in Tactical Decision Making: Applications of Lessons Learned
Managing Multiple Field Assets During Analog Missions
Balancing the needs and cultures of operational and scientific teams during a mission can be crucial. For instance, during the D-RATS missions (Abercromby et al., 2013), a discrepancy between the space operations community (which had heritage from operational branches of NASA with marginal experience in geoscience field activities) and the scientific teams (stemming from the academic community with less spaceflight operational experience) was observed. This led to flight planning friction losses resulting in both communities feeling under-served. Following the D-RATS lessons learned (Eppler et al., 2013; Rader et al., 2013), as a best-practice example, the 2017 BASALT missions included a Science Traceability Matrix (STM) and operational concepts (“ConOps”; Lim et al., 2019) defining the missions' science objective. Additionally, they implemented a near-real-time interaction between field personnel and the science back rooms at Mission Control (Brady et al., 2019), as well as selected operational considerations (Beaton et al., 2019).
Although there was a lack of assets expected during an actual mission, such as rovers, drones, or a physiological load, and consumables modeling of astronauts, the BASALT field activities were supported by the Minerva software suite (Marquez et al., 2019), optimizing a traverse planning, timeline generation and display (via the PLAYBOOK software, Marquez et al., 2017), procedure management, execution monitoring, data archiving, and visualization (Deans et al., 2017) and included a set of codified flight rules, safety rules, and troubleshooting routines. Similarly, the Austrian Space Forum has established codified standard operating procedures (SOPs), including workflows analogous to Minerva, but also including physiological modeling and monitoring and taking into account the limitations of operating in high-fidelity spacesuit simulators. These procedures are constantly trained and have evolved into an operational toolkit independent of the technical framework deployed (Groemer et al., 2016).
It is to be expected that a human-robotic mission to Mars will include multiple field assets ranging from human explorers, aerial and surface robotic vehicles, human mobility, habitat modules, stationary instruments and engineering elements for power, communication, and in-situ resource utilization. Notably, this variety will require a significantly more complex asset coordination compared to single-rover planetary missions, including the need for delay-tolerant networking and in-situ high-performance computing (Geist et al., 2019). The NASA MOSAIC initiative points out this challenge in hindsight of multiple robotic assets on the surface (Hook et al., 2018) but is not designed to address the scale and complexity of a human-robotic mission.
The Exploration Cascade
The “Exploration Cascade” (EC) is an OeWF-coined term for tactically optimizing the sequence of measurements for pursuing a pre-defined scientific question. Although the strategic aims may be set well prior to the flight mission architecture development, environmental dynamics (e.g., the Martian weather or solar activity), infrastructure limitations (e.g., communication ranges, safety rules), instrument anomalies (e.g., dust-induced degradation), human factors (e.g., reduced productivity due to isolation), and even (ground-based) data processing pipeline limitations will have a significant impact on the modalities of when and where to deploy which instruments. Building upon established workflows and SOPs, the Austrian Space Forum has devised the “Exploration Cascade” as an evolving algorithm, taking into account the aforementioned border conditions. Generally, science operations in multidisciplinary campaigns with a wide range of both requirements and expected data products may present challenges in the coordination workflows, but also offer synergistic effects, if properly applying the EC as an operational tool. To exemplify this, under certain circumstances, the high-res imagery obtained by an orbiting telescope may need certain orbital parameters to be met before the target of interest can be surveyed. Although aerial vehicles may need significantly longer time for surveying a site, they might still be the better choice as they would be readily available. This workflow defines when and where to deploy instruments, when their data are to be expected by the Mission Support Center on Earth and how fast the data processing can lead to knowledge influencing the decision making of the flight planners. The exploration cascade was first demonstrated in an early exploratory investigation during the AMADEE-18 field campaign in Oman in February 2018, bringing together 16 experiments (Garnitschnig, 2018), and will be furthered during the AMADEE-20 field campaign in Israel in late 2020.
The EC visualizes and optimizes instrument workflows and their required resources, environmental, and flight planning border conditions, as well as the ground segment data processing pipeline. In comparison to the established and well-tested PLAYBOOK software, the EC is also used as one of the selection criteria for experiment proposals at pre-mission phase, and it allows for an inclusion of the ground segments' remote science support. Initially, it can be considered an empty roadmap that is filled with mission aims, operational requirements (e.g., safety rules for astronauts), scientific priorities (e.g., prioritizing of biomarker detection over media activities), and finally with selected experiments during the mission preparation. Subsequently, after several Dress Rehearsals and map exercises, it evolves into a web of dependencies identifying critical pathways (aka sequence of experiment stages determining the minimum duration for an operation) and susceptibilities to external changes in the workflow as well as their potential alternatives by providing strong networking between experiment PIs, mission leaders, and team leaders, before and during the mission. Finally, it offers lessons learned for future missions, e.g., identifying the need for faster data processing pipelines or increasing instrument robustness for critical pathways.
Analog missions are tools to test permutations and the decision-making trees of the EC. We argue that if the field trials include representative scientific environments, such as a time-delayed remote science support teams and realistic data processing pipelines, multiple assets to be coordinated, and a plausible rule set for the field operations, then uncertainties and weaknesses in the flight mission planning can be substantially reduced. Especially given the cost-benefit ratio of analog missions, variants of the EC can be continuously field-tested with a moderate effort along the mission planning up to the landing of the actual flight missions.
Conclusions
The proper implementation of the Exploration Cascade is yet to be demonstrated, in particular, the robustness of the workflows needs to be tested in representative environments. As such, the AMADEE-20 mission in Israel in late 2020 will be a proving ground for applying the EC.
Mission teams have strived for a realistic projection of the workflows involving both flight crews on “Mars” and the remote science support teams on Earth. However, the multitude and peculiarities of instruments and technologies available to mission architects and researchers requires a plethora of planning decisions, as in contrast to, e.g., rover missions, the range of decision options rises exponentially with the number of field assets deployed. Also, the bandwidth of scientific priorities makes it challenging to identify patterns (e.g., perceived optimal sequence of workflows) and preferably strategies beyond anecdotal evidence. Therefore, we advocate for a deeper understanding of the scaling effects of an increasing number of field assets, considering long-duration surface sojourns with engineering constraints along with low bandwidth and time-delayed communication, as well as human factors.
The contribution of analog missions to flight mission architectures is strengthened by a clear definition of scientific priorities, awareness about mission architecture assumptions, and a well-structured workflow that allows for an in-depth analysis of the mission performance. Besides, a structured lessons-learned process and emerging well-maintained science data archives, that are open to the scientific community beyond individually funded missions, are key to optimizing the science return for future flight missions.
Author Contributions
All authors listed have made a substantial, direct and intellectual contribution to the work, and approved it for publication.
Conflict of Interest
The authors declare that the research was conducted in the absence of any commercial or financial relationships that could be construed as a potential conflict of interest.
References
Abercromby, A. F. J., Chappell, S. P., and Gernhardt, M. L. (2013). Desert RATS 2011: human and robotic exploration of near-Earth asteroids. Acta Astronaut. 91, 34–48. doi: 10.1016/j.actaastro.2013.05.002
Allner, M., McKay, C., Coe, L., Rask, J., Paradise, J., and Wynne, J. J. (2010). NASA's explorer school and spaceward bound programs: insights into two education programs designed to heighten public support for space science initiatives. Acta Astronaut. 66, 1280–1284. doi: 10.1016/j.actaastro.2009.09.019
Amundsen, H. E. F., Westall, F., Steele, A., Vago, J., Schmitz, N., Bauer, A., et al. (2010). “Integrated ExoMars PanCam, Raman, and close-up imaging field tests on AMASE 2009,” in EGU General Assembly 2010. Abstract No:12, 8757. Available online at: http://meetingorganizer.copernicus.org/EGU2010/EGU2010-8757.pdf (accessed July 5, 2020).
Beaton, K. H., Chappell, S. P., Abercromby, A. F. J., Miller, M. J., Kobs Nawotniak, S. E., Brady, A. L., et al. (2019). Using science-driven analog research to investigate extravehicular activity science operations concepts and capabilities for human planetary exploration. Astrobiology 19, 300–320. doi: 10.1089/ast.2018.1861
Beaty, D. W., Grady, M. M., McSween, H. Y., Sefton-Nash, E., Carrier, B. L., Altieri, F., et al (2018). iMOST, The Potential Science and Engineering Value of Samples Delivered to Earth by Mars Sample Return, Final Report August 14, 2018 (MEPAG), 186. Available online at: https://mepag.jpl.nasa.gov/reports/iMOST_Final_Report_180814.pdf (accessed July 5, 2020).
Beaty, D. W., Grady, M. M., McSween, H. Y., Sefton-Nash, E., Carrier, B. L., Altieri, F., et al. (2019a). The potential science and engineering value of samples delivered to Earth by Mars sample return, Final Report. Meteoritics Planet Sci. 54, S3–S152. doi: 10.1111/maps.13242
Beaty, D. W., Grady, M. M., McSween, H. Y., Sefton-Nash, E., Carrier, B. L., Altieri, F., et al. (2019b). The potential science and engineering value of samples delivered to Earth by Mars sample return. Meteoritics Planet Sci. 54, 667–671. doi: 10.1111/maps.13232
Belz, S., Buchert, M., Bretschneider, J., Nathason, E., and Fasoulas, S. (2014). Physicochemical and biological technologies for future exploration missions. Acta Astronaut. 101, 170–179. doi: 10.1016/j.actaastro.2014.04.023
Bessone, L., Sauro, F., Maurer, M., and Piens, M. (2018). “Testing technologies and operational concepts for field geology exploration of the Moon and beyond: the ESA PANGAEA-X Campaign,” in 20th EGU General Assembly, EGU. Abstract No: 4013. Available online at: https://meetingorganizer.copernicus.org/EGU2018/EGU2018-4013.pdf (accessed July 5, 2020).
Bessone, L., Sauro, F., and Stevenin, H. (2015). “Training safe and effective spaceflight operations using terrestrial analogues,” in Space Safety Is No Accident, eds T. Sgobba and I. Rongier (Cham: Springer), 313–318. doi: 10.1007/978-3-319-15982-9_37
Brady, A. L., Kobs Nawotniak, S. E., Hughes, S. S., Payler, S. J., Stevens, A. H., Cockell, C. S., et al. (2019). Strategic planning insights for future science-driven extravehicular activity on Mars. Astrobiology 19, 347–368. doi: 10.1089/ast.2018.1850
Chavers, G., Suzuki, N., Smith, M., Watson-Morgan, L., Clarke, S. W., Engelund, W. C., et al. (2019). “Nasa's human lunar landing strategy,” in 70th International Astronautical Congress, IAF Human Spaceflight Symposium, Conference Paper: IAC-19-B3.1.8, 1–6. Available online at: https://ntrs.nasa.gov/archive/nasa/casi.ntrs.nasa.gov/20190032452.pdf (accessed July 5, 2020).
Crawford, I. A. (2012). Dispelling the myth of robotic efficiency. Astron. Geophys. 53, 2.22–2.26. doi: 10.1111/j.1468-4004.2012.53222.x
Crawford, I. A. (2014). Science enabled by the global exploration roadmap. Astron. Geophysics 55, 3.20–3.23. doi: 10.1093/astrogeo/atu124
Davila, A. F, Skidmore, M., Fairén, A. G., Cockell, C., and Schulze-Makuch, D. (2010). New priorities in the robotic exploration of Mars: the case for in situ search for extant life. Astrobiology 10, 705–710. doi: 10.1089/ast.2010.0538
Deans, M., Marquez, J. J., Cohen, T., Miller, M., Deliz, I., Hillenius, S., et al. (2017). “Minerva: User-centered science operations software capability for future human exploration,” in 2017 IEEE Aerospace Conference (Big Sky, MT), 1–13. doi: 10.1109/AERO.2017.7943609
Domagal-Goldman, S. D., Wright, K. E., Adamala, K., Arina de la Rubia, L., Bond, J., Dartnell, L. R., et al. (2016). The astrobiology primer v2.0. Astrobiology 16, 561–653. doi: 10.1089/ast.2015.1460
Drake, B. G., (ed.). (2009). Human Exploration of Mars Design Reference Architecture 5.0. NASA-SP-2009-566. Available online at: https://www.nasa.gov/pdf/373665main_NASA-SP-2009-566.pdf (accessed July 5, 2020).
Drake, B. G., Hoffman, S. J., and Watts, K. D. (2010). “Human exploration of Mars design reference architecture 5.0.,” in 2010 IEEE Aerospace Conference (Big Sky, MT), 1–24. doi: 10.1109/AERO.2010.5446736
Dunér, D., Capova, K., Gargaud, M., Geppert, W., Kereszturi, A., and Persson, E. (2018). “Astrobiology and the Society in Europe,” in Astrobiology and Society in Europe Today. SpringerBriefs in Astronomy, eds K. Capova, E. Persson, T. Milligan, and D. Dunér (Cham: Springer), 7–10. doi: 10.1007/978-3-319-96265-8_2
Eppler, D., Adams, B., Archer, D., Baiden, G., Brown, A., Carey, W., et al. (2013). Desert Research and Technology Studies (DRATS) 2010 science operations: operational approaches and lessons learned for managing science during human planetary surface missions. Acta Astronaut. 90, 224–241. doi: 10.1016/j.actaastro.2012.03.009
Fairén, A. G., Schulze-Makuch, D., Whyte, L., Parro, V., Pavlov, A., Gómez-Elvira, J., et al. (2019). Planetary protection and the astrobiological exploration of Mars: proactive steps in moving forward. Adv. Space Res. 63, 1491–1497. doi: 10.1016/j.asr.2019.01.011
Garnitschnig, S. (2018). Development of a supportive method for the detection of biomarkers during future human-robotic Mars missions–A case study to optimize the deployment modalities based on the results of the AMADEE-18 analog mission in the Dhofar region in the Sultanate of Oman. (Bachelor thesis). University of Innsbruck, Innsbruck, Austria, 47.
Geelen, K., Vijendran, S., Rebuffat, D., Larranaga, J., and Falkner, P. (2013). The Mars Robotic Exploration Preparation (MREP) Programme: Missions and Related Technology Developments. London: EPSC, EPSC2013-1057. Available online at: https://meetingorganizer.copernicus.org/EPSC2013/EPSC2013-1057.pdf (accessed July 5, 2020).
Geist, A., Brewer, C., Davis, M., Franconi, N., Heyward, S., Wise, T., et al. (2019). “SpaceCube v3.0 NASA next-generation high-performance processor for science applications,” in 33rd Annual AIAA/USU Conference on Small Satellites. Conference Paper: SSC19-XII-02 (Logan), 1–9.
Glass, B., and Briggs, G. (2003). “Evaluation of human vs. teleoperated robotic performance in field geology tasks at a Mars analog site,” in 7th International Symposium on Artificial Intelligence, Robotics and Automation in Space (i-SAIRAS 2003) (Nara), 8. Available online at: https://ntrs.nasa.gov/archive/nasa/casi.ntrs.nasa.gov/20030032439.pdf (accessed July 5, 2020).
Groemer, G. E. (2009). AustroMars and PolAres: measuring forward contamination during Mars-Analogue Missions. Planetary Space Sci. 57, 660–663. doi: 10.1016/j.pss.2008.07.021
Groemer, G. E., Losiak, A., Soucek, A., Plank, C., Zanardini, L., Sejkora, N., et al. (2016). The AMADEE-15 Mars simulation. Acta Astronaut. 129, 277–290. doi: 10.1016/j.actaastro.2016.09.022
Groemer, G. E., Soucek, A., Frischauf, N., Stumptner, W., Ragonig, C., Sams, S., et al. (2014). The MARS2013 Mars Analog Mission. Astrobiology 14, 360–376. doi: 10.1089/ast.2013.1062
Grotzinger J. P. Crisp J. A. Vasavada A. R. the MSL Science Team (2015). Curiosity's mission of exploration at Gale Crater, Mars. Elements 11, 19–26. doi: 10.2113/gselements.11.1.19
Häuplik-Meusburger, S., Binsted, K., Bassingthwaighte, T., and Petrov, G. (2017). “Habitability studies and full-scale simulation research: preliminary themes following HISEAS Mission IV,” in 47th International Conference of Environmental Systems 2017, ICES-2017-138.
Hook, J. V., Vaquero, T., Troesch, M., de la Croix, J., Schoolcraft, J., Bandyopadhyay, S., et al. (2018). “Dynamic shared computing resources for multi-robot Mars exploration,” in International Symposium on Artificial Intelligence, Robotics, and Automation in Space (i-SAIRAS 2018) (Madrid). Also appears at the 28th International Conference on Automated Planning and Scheduling (ICAPS) 2018 Workshop on Planning and Robotics (PlanRob) (Charlston, SC: Delft). Available online at: https://robotics.estec.esa.int/i-SAIRAS/isairas2018/Papers/Session%205c/1_URS-v2.1-127-74-Vaquero-Tiago.pdf (accessed July 5, 2020).
Imhof, B., Hogle, M., Davenport, B., Weiss, P., Urbina, D., Royrvik, J., et al. (2017). “Project Moonwalk: lessons learnt from testing human robot collaboration scenarios in a lunar and Martian simulation,” in 69th International Astronautical Congress (IAC) (Adelaide, SA: IAC-17-F1.2.3), 12.
Imhof, B., Hoheneder, W., Ransom, S., Waclavicek, R., Davenport, B., Schwendner, J., et al. (2015). “Moonwalk - human robot collaboration mission scenarios and simulations,” in AIAA SPACE 2015 Conference and Exposition: Conference Paper (AIAA 2015-453) (Pasadena, CA). doi: 10.2514/6.2015-4531
Kah, L. C., and MSL Science Team. (2015). Images from curiosity: a new look at Mars. Elements 11, 27–32. doi: 10.2113/gselements.11.1.27
Kminek, G., Rummel, J. D., Cockell, C. S., Atlas, R., Barlow, N., Beaty, D., et al. (2010). Report of the COSPAR Mars special regions colloquium. Adv. Space Res. 46, 811–829. doi: 10.1016/j.asr.2010.04.039
Kobrick, R., Lopac, N., Schuman, J., French, J., and Tomiyama, T. (2018). “Increasing spaceflight analogue mission fidelity by standardization of extravehicular activity metrics tracking and analysis,” in 48th International Conference on Environmental Systems, ICES-2008-191, 1–12. Available online at: https://ttu-ir.tdl.org/handle/2346/74160 (accessed July 5, 2020).
Lim, D. S. S., Abercromby, A. F. J., Kobs Nawotniak, S. E., Lees, D. S., Miller, M. J., Brady, A. L., et al. (2019). The BASALT research program: designing and developing mission elements in support of human scientific exploration of Mars. Astrobiology 19, 245–259. doi: 10.1089/ast.2018.1869
Litaker, H. L., and Howard, R. L. (2013). Social network analysis and dual rover communications. Acta Astronaut. 90, 367–377. doi: 10.1016/j.actaastro.2012.05.013
Marquez, J. J., Hilenius, S., Kanefsky, B., Zheng, J., Deliz, I., and Reagan, M. (2017). “Increasing Crew Autonomy for Long Duration Exploration Missions: Self-Scheduling,” in 2017 IEEE Aerospace Conference (Big Sky, MT), 1–10. doi: 10.1109/AERO.2017.7943838
Marquez, J. J., Miller, M. J., Cohen, T., Deliz, I., Lees, D. S., Zheng, J., et al. (2019). Future needs for science-driven geospatial and temporal extravehicular activity planning and execution. Astrobiology 19, 440–461. doi: 10.1089/ast.2018.1838
Martins, Z., Cottin, H., Kotler, J. M., Carrasco, N., Cockell, C. S., de la Torre Noetzel, R., et al. (2017). Earth as a tool for astrobiology - a European perspective. Space Sci. Rev. 209, 43–81. doi: 10.1007/s11214-017-0369-1
Payler, S., Biddle, J., Coates, A., Cousins, C., Cross, R., Cullen, D., et al. (2017). Planetary science and exploration in the deep subsurface: results from the MINAR Program, Boulby Mine, UK. Int. J. Astrobiol. 16, 114–129. doi: 10.1017/S1473550416000045
Preston, L. J., and Dartnell, R. L. (2014). Planetary Habitability: Lessons learned from terrestrial analogues. Int. J. Astrobiol. 13, 81–98. doi: 10.1017/S1473550413000396
Rader, S. N., Reagan, M. L., Janoiko, B., and Johnson, J. E. (2013). “Human-in-the-loop operations over time delay: lessons learned,” in 43rd International Conference on Environmental Systems, AIAA 2013-3520 (Vail, CO). doi: 10.2514/6.2013-3520
Rask, J., Heldmann, J., Smith, H., Battler, M., and McKay, C. (2011). “The NASA spaceward bound field training curriculum,” in GSA Special Papers: Analogs for Planetary Explorations, Vol. 483 (Boulder, CO), 157–163. doi: 10.1130/2011.2483(10)
Schulze-Makuch, D., Wagner, D., Kounaves, S. P., Mangelsdorf, K., Devine, K. G., Vera, J. P., et al. (2018). Transitory microbial habitat in the hyperarid Atacama Desert. Proc. Natl. Acad. Sci. U.S.A. 115, 2670–2675. doi: 10.1073/pnas.1714341115
Szocik, K. (2019). Should and could humans go to Mars? Yes, but not now and not in the near future. Futures 105, 54–66. doi: 10.1016/j.futures.2018.08.004
Ushakov, I. B., Vladimirovich, M. B., Bubeev, Y. A., Gushin, V. I., Vasileva, G. Y., Vinokhodova, A. G., et al. (2014). Main findings of psychophysiological studies in the Mars 500 experiment. Herald Russian Acad. Sci. 84, 106–114. doi: 10.1134/S1019331614020063
Vago, J. L., Coates, A. J., Jaumann, R., Korablev, O., Ciarletti, V., Mitrofanov, T., et al. (2018). “Searching for traces of life with the ExoMars Rover,” in From Habitability to Life on Mars, ed N. A. Cabrol and E. A. Grin (Philadelphia, PA: Elsevier), 309–347. doi: 10.1016/B978-0-12-809935-3.00011-6
Keywords: planetary exploration, astrobiology, spacesuit simulators, exploration cascade, tactical planning
Citation: Groemer G and Ozdemir S (2020) Planetary Analog Field Operations as a Learning Tool. Front. Astron. Space Sci. 7:32. doi: 10.3389/fspas.2020.00032
Received: 05 December 2019; Accepted: 22 May 2020;
Published: 30 July 2020.
Edited by:
Tetyana Milojevic, University of Vienna, AustriaReviewed by:
Felipe Gómez, Centro de Astrobiología (CSIC-INTA), SpainAkos Kereszturi, Research Centre for Astronomy and Earth Sciences, Hungary
Jesús Martínez-Frías, Consejo Superior de Investigaciones Científicas (CSIC), Spain
Copyright © 2020 Groemer and Ozdemir. This is an open-access article distributed under the terms of the Creative Commons Attribution License (CC BY). The use, distribution or reproduction in other forums is permitted, provided the original author(s) and the copyright owner(s) are credited and that the original publication in this journal is cited, in accordance with accepted academic practice. No use, distribution or reproduction is permitted which does not comply with these terms.
*Correspondence: Gernot Groemer, Z2Vybm90Lmdyb2VtZXJAb2V3Zi5vcmc=