- 1Laboratory of Astrobiology and Molecular Biology of Cyanobacteria, Department of Biology, University of Rome Tor Vergata, Rome, Italy
- 2SSPT-PROTER Division, ENEA Casaccia, Rome, Italy
- 3Department of Planetary Laboratories, Astrobiological Laboratories, German Aerospace Center (DLR), Institute of Planetary Research, Berlin, Germany
Unraveling how long life can persist under extreme dryness and what kind of environmental extremes can be faced by dried microorganisms is relevant to understand Mars habitability and to search for life on planets with transient liquid water availability. Because trehalose and sucrose stabilize dried anhydrobiotes, an in silico survey of the genome of the desert cyanobacterium Chroococcidiopsis sp. CCMEE 029 was performed to identify pathways for trehalose and sucrose biosynthesis. The expression of the identified genes was induced in response to desiccation, and trehalose and sucrose accumulation was detected in dried cells. This adaptation strategy enabled viability and biomarker permanence under extreme dryness and Mars-like UV flux. Chroococcidiopsis survivors were scored in 7-year dried biofilms mixed with phyllosilicatic Mars regolith simulant and exposed to 5.5 × 103 kJ/m2 of a Mars-like UV flux. No survivors occurred after exposure to 5.5 × 105 kJ/m2 although, in dead cells, photosynthetic pigments, and nucleic acids, both DNA and RNA, were still detectable. This suggests that dried biofilms mixed with phyllosilicatic Martian regolith simulant are suitable candidates to identify biosignatures embedded in planetary analog minerals as planned in the future BioSignatures and habitable Niches (BioSigN) space mission to be performed outside the International Space Station.
Introduction
Unraveling how long life can persist under extreme dryness and which environmental extremes can be faced in the dried state is relevant for long-term models of Mars habitability (Davila and Schulze-Makuch, 2016) and for searching for life on planets with transient availability of liquid water (Schulze-Makuch et al., 2017; Wilhelm et al., 2018). Moreover, according to the so called geogenetic latency hypothesis, subsurface microbes could survive planetary surface extinction and be re-exposed to the surface via geological processes, when conditions allow water to flow (Boston et al., 2019).
Indeed dryness is one of the main life-threatening factors; nevertheless, upon desiccation, a small group of taxonomically diverse organisms enter a reversible metabolic dormancy, a phenomenon known as anhydrobiosis (Crowe et al., 1992). Anhydrobiotes survive water removal as specialized structures, such as bacterial spores, cyanobacterial akinetes, and certain crustacean cysts and insect larvae, and a few among cyanobacteria, lichens, fungi, rotifers, nematodes, and tardigrades enter anhydrobiosis in the vegetative state (Alpert, 2006).
Hot and cold deserts are relevant Martian field analogs due to their aridity, temperatures, and/or geological features (Martins et al., 2017) and are considered of great interest to appreciate biosignature preservation under conditions similar to those of Mars (Aerts et al., 2020). So, if life ever existed on Mars' surface, it must have faced the presumed three main climatic stages of Mars: this beginning with a water-rich period, followed by a cold and semiarid one, and ending with the present-day arid and cold environment (Fairén et al., 2010). Because everything we know about biology we have learned from life on Earth (McKay, 2010), desiccation-tolerant microorganisms might be the best-case biologic scenario for understanding the habitability of Mars (Wilhelm et al., 2018) and identifying protective biomolecules to be used as a biomarker database (Jorge-Villar and Edwards, 2013).
Desert cyanobacteria of the genus Chroococcidiopsis, being metabolically active for a few hours per year, provide some evidence of their anhydrobiotic potential (Friedmann et al., 1993; Warren-Rhodes et al., 2006). Under laboratory conditions, they recovered after 4 and 7 years of air-dried storage (Billi, 2009; Fagliarone et al., 2017; Mosca et al., 2019) although new evidence will be provided by the 500-Year Microbiology Experiment aimed to investigate the desiccation longevity of dried Chroococcidiopsis and Bacillus subtilis spores, sealed in glass vials with silica gel beads (Cockell, 2015; Ulrich et al., 2018).
Insights into the environmental extremes that desert strains of Chroococcidiopsis can face have been revealed by challenging dried cells with the exposure to Mars simulations, either in the laboratory or in space. Under Mars laboratory simulations, dried biofilms survived 1.5 × 103 kJ/m2 of a Mars-like UV flux followed by 7 years of air-dried storage (Mosca et al., 2019). During the EXPOSE-R2 space mission, dried samples survived 18-month exposure to Mars simulations in low Earth orbit outside the International Space Station (Rabbow et al., 2017). Under these conditions, samples face extreme dryness induced by a space vacuum and Mars simulated pressure combined with solar UV radiation and cosmic ionizing radiation (de Vera et al., 2019). In the Biofilm Organisms Surfing Space (BOSS) space experiment, within dried biofilms, top cell layers shielded bottom cell layers against UV radiation (Billi et al., 2019a). Whereas in the BIOlogy and Mars EXperiment (BIOMEX) space experiment, dried cells were shielded by mixing with Martian regolith simulants (Billi et al., 2019b).
In the present work, by taking advantage of the BIOMEX and BOSS results, biofilms of Chroococcidiopsis sp. CCMEE 029 were obtained by growing cells mixed with phyllosilicatic Martian regolith simulant (P-MRS) and then exposed to Mars laboratory simulations. The hypothesis is that, within the biofilm structure, the mixing of cells with minerals guarantees better UV radiation shielding. Indeed, the identification of suitable biosignatures embedded in planetary analog minerals is one the aims of the future BioSignatures and habitable Niches (BioSigN) space experiment to be performed outside the International Space Station (de Vera and The Life Detection Group of BIOMEX/BIOSIGN, 2019).
No doubt, unraveling the biochemical mechanisms that stabilize dried Chroococcidiopsis cells will help identify key molecules for dryness adaptation that might enable survival and/or biomarker permanence under Mars-like conditions. In this scenario, a crucial role should be played by trehalose and sucrose, two non-reducing sugars accumulated by anhydrobiotes, which replace water molecules, prevent membrane phase transition, and allow cytoplasmic vitrification (Sun and Leopold, 1997; Crowe et al., 1998). Indeed vitrification is supposed to underlie Chroococcidiopsis survival after exposure to subfreezing temperatures in salt solutions as inferred for Europa's icy surface (Cosciotti et al., 2019). Trehalose is also proposed to act as free-radical scavenger, thus avoiding oxidative damage (Benaroudj et al., 2001). Indeed, in a radioresistant fungus, the overexpression of a trehalose–synthase gene yields an increased trehalose accumulation and enhanced resistance to gamma irradiation, UV light, and heavy metal ions (Liu et al., 2017). Notably, glucose addition to Bacillus subtilis spores increased survival after 6-year exposure to a space vacuum inside the NASA Long Duration Exposure Facility (Horneck et al., 1994).
Here an in silico analysis of the genome of Chroococcidiopsis sp. CCMEE 029 was performed in order to identify genes involved in the following trehalose and sucrose biosynthesis: (i) the TreY/TreZ pathway catalyzing the transglycosylation of maltodextrins into maltooligosyl trehalose and trehalose hydrolytic release; (ii) the TreS pathway transforming maltose into trehalose; (iii) the TPS/TPP pathway catalyzing the formation of trehalose-6-phosphate and its dephosphorylation into trehalose; (iv) the SPS/SPP pathway with a sucrose–phosphate synthase and sucrose–phosphate phosphatase; and (v) the SUS pathway catalyzing the reversible transfer of a glucosyl moiety between fructose and a nucleoside diphosphate (for a review, see Avonce et al., 2006; Kolman et al., 2015).
The role of the identified genes in the desiccation tolerance of Chroococcidiopsis was evaluated by monitoring their expression by real-time quantitative PCR (RT-qPCR) in 10- and 60-min dried cells. Trehalose and sucrose content was also determined in dried cells. After a prolonged air-dried storage (7 years), dried biofilms mixed with P-MRS and exposed to Mars laboratory simulations were investigated for viability and biomarker permanence by mean of SYTOX-Green staining and RT-qPCR, respectively.
Materials and Methods
Organism, Culture Conditions, and Desiccation
The cyanobacterium Chroococcidiopsis sp. CCMEE 029 from the Negev Desert (Israel) is maintained at the Department of Biology as part of the Culture Collection of Microorganisms from Extreme Environments (CCMEE) established by E. Imre Friedmann and Roseli Ocampo-Friedmann. The strain was grown under routine conditions at 25°C in liquid BG-11 medium under a photon flux density of 40 μmol/m2/s provided by fluorescent cool-white bulbs.
Biofilms were obtained by plating about 2 × 108 cells mixed with 0.2 g of a Phyllosilicatic Mars Regolith Simulant (P-MRS; see Baqué et al., 2016, for regolith composition) on top of BG-11 agarized medium in Petri dishes sealed with Parafilm and allowed to grow for 2 months.
Desiccation was performed as follows: (i) over silica gel: liquid-culture aliquots were immobilized on 0.2-μm polycarbonate filters (Millipore) and stored for 10 and 60 min in a glass vacuum desiccator with anhydrous silica gel at room temperature (RT) in the dark; (ii) through air-drying: liquid-culture aliquots were dried under a laminar flow hood for 24 h and stored at RT in the dark. Biofilms were allowed to air-dry for 15 days under routine conditions by removing the Parafilm from the Petri dishes (Billi et al., 2019a).
Mars Laboratory Simulations
Dried biofilms mixed with minerals were accommodated in the DLR 16-well aluminum sample carriers at the Planetary and Space Simulation facilities (Microgravity User Support Center, DLR Cologne, Germany) and exposed to Mars simulations between September 2013 and December 2013 as planned in the context of the EXPOSE-R2 space mission (de Vera et al., 2019). The top layer of the carrier samples were exposed in triplicate to 5.5 × 103 kJ/m2, 1.4 × 105 kJ/m2, 2.7 × 105 kJ/m2, and 5.5 × 105 kJ/m2 with a SOL200 lamp (1271.2 W/m2 200–400 nm) for 1 h 12 min, 30 h, 60 h, and 120 h under Earth's atmosphere, and in the bottom layer, samples were kept in the dark (see Table 3, de Vera et al., 2019). In another simulation, samples were exposed in the top layer of the carrier in triplicate to 5.7 × 105 kJ/m2 with a SOL200 lamp (1,271 W/m2 200–400 nm) for 98 h 73 min under a CO2 Mars-like atmosphere (at 1 kPa), and in the bottom-layer carrier, samples were kept in the dark under a Mars-like atmosphere (see Table 4, de Vera et al., 2019). After the Mars simulations, dried samples were kept in the dark at RT for about 7 years.
Identification of Genes Encoding Trehalose and Sucrose Biosynthetic Enzymes
The genome of Chroococcidiopsis sp. CCMEE 029 was sequenced by using Illumina/Solexa technology (CD Genomics, NY, USA), gene annotation was performed by using the PROKKA v.1.11 software (Seemann, 2014), and the interface was provided by Galaxy-based framework Orione (Cuccuru et al., 2014). Genes encoding trehalose and sucrose biosynthetic enzymes were identified by a BlastN (Nucleotide Query Searching a Nucleotide Database) search for nucleotide sequences of sequenced cyanobacterial orthologs as previously reported for DNA repair genes (Mosca et al., 2019).
RNA Extraction and RT-qPCR
Total RNA was extracted from each sample by using 1 ml of TRI Reagent® (Merck) and treatment with RQ1 RNase-Free DNase I (Promega Corporation, Madison, WI, USA) according to the manufacturer's instructions. Then, 1 μg of total RNA for each sample was retrotranscribed to single-strand cDNA by using the SensiFAST™ cDNA Synthesis Kit (Bioline Meridian Life Science, Memphis, TN, USA). Real-time reactions were performed in 12 μl, including 1 μg of cDNA template, 6 μl of iTaq™ universal SYBR® Green supermix (BioRad Laboratories, Hercules, CA, USA), and 400 nM of the appropriate primer (Table 1). Primer specificity was confirmed by melting curve analysis. 16S rRNA (GenBank accession number AF279107) was used as a reference gene (Pinto et al., 2012). PCR cycling conditions were performed in a StepOnePlus™ Real-Time PCR System (Thermo Fisher Scientific, Waltham, MA, USA) as follows: a cycle of 95°C for 10 min, then 40 cycles of 95°C for 15 s, and 60°C for 1 min, followed by a ramp from 60 to 95°C for the melting curve stage.
(i) Gene expression in response to desiccation. Real-time reactions were performed by using total RNA extracted from 10- and 60-min dried cells and liquid cultures. Relative mRNA levels were calculated by the comparative cycle threshold (Ct) method. Primer specificity was confirmed by melting curve analysis. 16S rRNA (GenBank accession number AF279107) was used as a reference gene (Pinto et al., 2012). Values obtained for liquid control were set as one, and values of dried cells were considered to be upregulated (>1) or downregulated (<1). For each gene target, n ≥ 3 qPCR reactions were conducted, each one in duplicate.
(ii) Permanence of 16S rRNA and mRNA in dried biofilms exposed to Mars laboratory simulations. RT-qPCR was performed by using total RNA extracted from 7-year-dried biofilms mixed with P-MRS and exposed to Mars laboratory simulations. Then, cycle threshold (Ct) values were acquired and used for statistical analyses. For the gene target, n ≥ 3 qPCR reactions were conducted, each one in duplicate.
HPLC Analysis
The quantitative analysis of trehalose and sucrose content was performed by reverse phase ion pair (RPIP)-HPLC48 on a Luna 5lC18 reversed phase column (150 × 4.6 mm) (Phenomenex, Torrance, CA, USA) in acetonitrile (8.5%) and tetra-n-butylammonium hydrogen sulfate (1.2 mM; Fluka, St. Louis, MO, USA) and by applying a stepwise gradient of 0.2 M NaCl from 1 to 62%. The HPLC system (Dionex, Sunnyvale, CA, USA) was run at a flow rate of 1.1 ml/min and the fluorescent labeling reaction was performed by post-column addition of 2-cyanoacetamide (0.25%; Sigma) and NaOH (0.25%) at a flow rate of 0.35 ml/min by a PC10 post-column pneumatic delivery package (Dionex, Sunnyvale, CA, USA). Signals were quantified by comparison with known amounts of standard disaccharides analyzed in parallel runs. Data were processed by using ThermoScientific Chromeleon Chromatography Data System (CDS) software.
Cell Viability
Cell viability was assessed by staining with the cell-impermeant nucleic acid dye SYTOX Green (Molecular Probes, Inc., Eugene, OR, US) at a final concentration of 50 μM for 5 min in the dark as previously reported (Billi, 2009). Images were taken with an Olympus FV1000 confocal laser-scanning microscope (CLSM) by exciting the cells with a 488-nm laser and collecting the emissions between 510 and 530 nm. Photosynthetic pigment autofluorescence was excited with a 635-nm laser and emissions collected from the 645–800 nm emission range.
Statistical Analysis
Experiments were carried out with at least three independent replicates, data are shown with standard deviation and significance assessed by using Student's t-test.
Results
Genes Encoding Trehalose Biosynthetic Enzymes
The in silico analysis identified in Chroococcidiopsis sp. CCMEE 029's genome genes for trehalose biosynthesis according to TreY/TreZ and TreS pathways (Table 2). The treY gene contained a 2,793-bp open reading frame predicted to encode a maltooligosyl trehalose synthase (TreY) and showing the highest similarity (BlastN output: query cover 99%, e-value 0.0, and total score 2,267) with the ortholog of Microcoleus sp. PCC 7113 (GenBank: CP003630.1:c4333883-4331094). The treZ gene contained an open reading frame of 1,827-bp potentially encoding a maltooligosyl trehalose trehalohydrolase (TreZ) and sharing the highest similarity (BlastN output: query cover 99%, e-value 0.0, and total score 2,547) with the ortholog of Microcoleus sp. PCC 7113 (GenBank: CP003630.1:c4339755-4337926).
Two treS genes encoding a maltose alpha-D-glucosyltransferase (TreS) were identified in Chroococcidiopsis sp. CCMEE 029's genome (Table 2). The treS1 had a 3393-bp open reading frame predicted to encode a long TreS and shared the highest similarity (BlastN output: query cover 99%, e-value 0.0, and total score 2,859) with the ortholog of Microcoleus sp. PCC 7113 (GenBank: CP003630). Although the treS2 contained a 1,641-bp open reading frame predicted to encode a short TreS, it showed the highest similarity (BlastN output: query cover 100%, e-value 0.0, and total score 1,657) with the ortholog of Scytonema sp. NIES-4073 (GenBank: AP018268.1). The in silico analysis did not identify any gene involved in the TPS/TPP pathway for trehalose biosynthesis.
Genes Encoding Sucrose Biosynthetic Enzymes
The in silico analysis of the genome of Chroococcidiopsis sp. CCMEE 029 identified genes for the sucrose synthesis according to SPS/SPP and SUS pathways (Table 3). The sps gene had a 1,281-bp open reading frame predicted to encode a sucrose–phosphate synthase (SPS) and showing the highest similarity (BlastN output: query cover 97%, e-value 0.0, and total score 135) with the gene of Chroococcidiopsis thermalis PCC 7203 encoding a glycosyltransferase (GeneBnk AF87753.1). The spp gene contained a 747-bp open reading frame encoding a putative sucrose–phosphate phosphatase (SPP) and exhibited the highest similarity (BlastN output: query cover 99%, e-value 2e-127, and total score 469) with the gene of Gloeocapsopsis sp. AAB1 encoding a sucrose-6F-phosphate phosphohydrolase (GenBank: KJ183087.1).
Two sus genes, namely susA and susB, were identified (Table 3). The susA gene contained a 2,421-bp open reading frame coding a putative sucrose synthase (SUS) and shared the highest similarity (BlastN output: query cover 100%, e-value 0.0, and total score 1,854) with the susA gene of Nostoc punctiforme PCC 73102 (GenBank: AJ316589.1). The susB gene had a 2,412-bp open reading frame coding a putative sucrose synthetase (SUS) and shared the highest similarity (BlastN output: query cover 100%, e-value 0.0, and total score 2,209) with the orthologous gene of Scytonema sp. HK-05 (GenBank: AP018194.1: c1150884-1153295) and a high similarity (BlastN output: query cover 97%, e-value 0.0, and total score 1,043) with the susB gene of Nostoc sp. PCC 7120 (GenBank: AJ316584.1).
Expression of Trehalose and Sucrose Biosynthesis Genes Upon Desiccation
The expression of trehalose biosynthetic genes was evaluated by RT-qPCR in 10- and 60-min dried Chroococcidiopsis cells over silica gel and compared to their expression in liquid controls (Figure 1A). Regarding the TreY/TreZ pathway, after 10 min of desiccation, the expression of the treY and treY genes was induced 5- and 6-fold, respectively, and after 60 min, of desiccation, the two genes resulted induced 8- and 9-fold, respectively (Figure 1A). For the TreS pathway, the treS long gene showed high mRNA levels after 10 and 60 min of desiccation, whereas the expression of the treS short gene was significantly induced at both desiccation points although at a lower level compared to the treS long gene (Figure 1A).
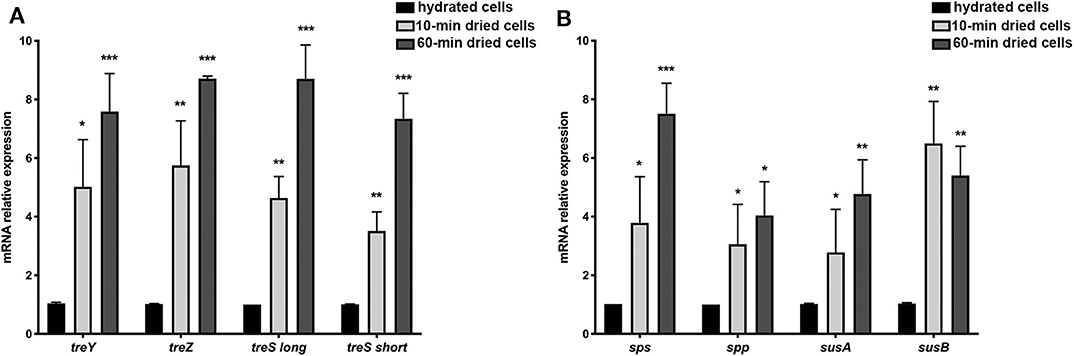
Figure 1. Expression of trehalose and sucrose biosynthetic genes in 10- and 60-min dried Chroococcidiopsis cells. Gene expression of TreY/TreZ and TreS trehalose biosynthetic pathway genes (A); gene expression of SPS/SPP and SUS sucrose biosynthetic pathways (B). Values in hydrated cells were considered as control values and set to 1. Data represent mean ± SD (n ≥ 3), ***p < 0.001, **p < 0.01, and *p < 0.05.
Genes involved in sucrose biosynthesis were induced after 10 and 60 min of desiccation compared to liquid control cultures (Figure 1B). Regarding the SPS/SPP pathway, the expression of the spp gene was induced at both desiccation points, and the mRNA levels of the sps gene increased after 10 and 60 min of desiccation. For the SUS pathway, the susB gene was more highly induced after 10 min than after 60 min of desiccation, and the mRNA levels of the susA gene increased at both desiccation points although at a lower level compared to the susB gene (Figure 1B).
Trehalose and Sucrose Content in Dried Cells
In dried Chroococcidiopsis cells, the amount of trehalose and sucrose was quantified by HLPC analysis, and liquid cultures were used as a control (Table 4). Cells desiccated over silica gel for 3 months showed a trehalose and sucrose amount of about 1.934 and 5.387 mg/g dry weight, respectively. This value was 33- and 5-fold increased from that detected in hydrated cells, which for trehalose and sucrose was about 0.059 and 1.127 mg/g dry weight, respectively. Cells air-dried for 24 h showed a trehalose and sucrose content of about 1.745 and 4.850 mg/g dry weight, respectively (Table 4).
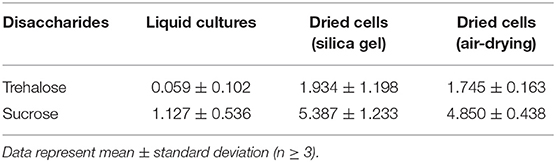
Table 4. Trehalose and sucrose content in dried cells of Chroococcidiopsis sp. CCMEE 029 (mg/g dry weight).
RNA Permanence Under Desiccation and Mars-Like UV Flux
The effects of short-term desiccation on RNA integrity was determined in 10- and 60-min dried cells Chroococcidiopsis by evaluating the cycle threshold (Ct) values in RT-qPCR assay (Figure 2), considering that a reduced RNA copy number corresponded to a high Ct value. A progressive reduction of 16S rRNA copy numbers occurred in 10- and 60-min dried cells that showed average Ct values of 23 and 25, respectively, hydrated cells had an average Ct value of 22 (Figure 2).
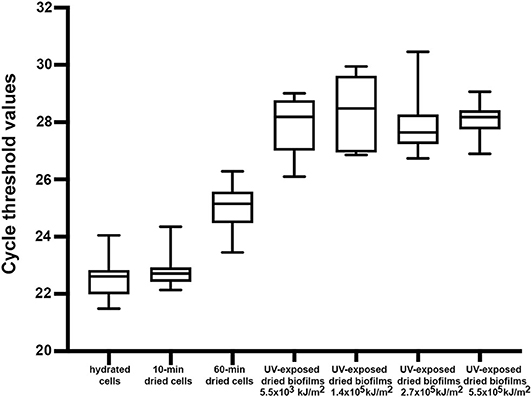
Figure 2. Permanence of 16S rRNA in 10- and 60-min dried Chroococcidiopsis cells and in 7-year dried Chroococcidiopsis biofilms mixed with P-MRS, according to Ct values in the RT-qPCR assay. Samples: hydrated control cells; 10- and 60-min dried cells; 7-year dried biofilms mixed with P-MRS and exposed to increasing Mars-like UV radiation doses. Ct values are shown as medians (lines), 25th percentile to 75th percentile (boxes), and ranges (whiskers).
The effects of long-term desiccation following exposure to Mars-like UV flux was determined in dried Chroococcidiopsis biofilms mixed with P-MRS and exposed to UV doses ranging from 5.5 × 103 kJ/m2 to 5.5 × 105 kJ/m2 (Figure 2). The absence of a reduction in the average Ct values with the increased UV doses suggests the permanence of a reduced 16S rRNA copy number (Figure 2).
RNA Permanence Under Desiccation, Mars-Like UV Flux and Mars-Like Atmosphere
The Ct value method was used to evaluate the mRNA permanence in dried Chroococcidiopsis biofilms mixed with P-MRS and exposed to a Mars-like UV flux (5.7 × 105 kJ/m2) combined with a Mars-like atmosphere and stored 7 years in the air-dried state. As shown in Figure 3, the four genes encoding sucrose biosynthetic enzyme were used as gene targets in determining Ct values in dried biofilms because they were previously reported to be induced upon desiccation (Figure 2B). Compared to hydrated cells, each gene showed reduced Ct value in 7-year dried biofilms exposed in the bottom-layer carrier, e.g., kept in the dark and exposed to a Mars-like atmosphere (Figure 3). When compared to dried biofilms exposed in the bottom-layer carrier, each gene showed slightly reduced Ct values in dried biofilms exposed in the top-layer carrier, i.e., exposed to 5.7 × 105 kJ/m2 of a Mars-like UV flux combined with a Mars-like atmosphere (Figure 3). Similar results were obtained for the four genes encoding trehalose biosynthetic enzymes (not shown).
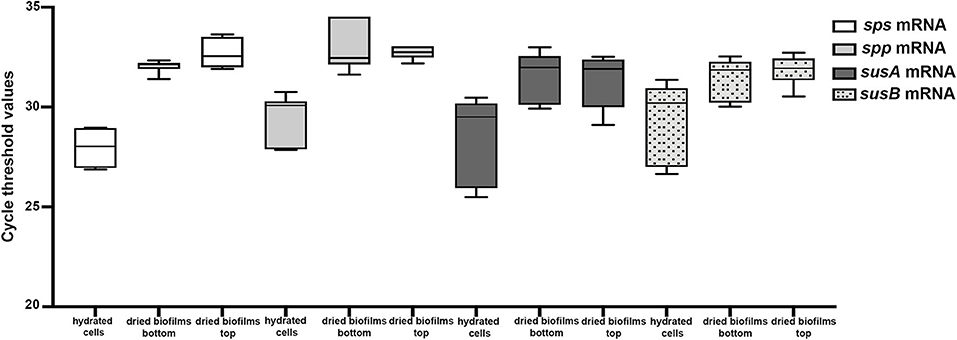
Figure 3. Permanence of mRNA in 7-year dried Chroococcidiopsis biofilms mixed with P-MRS, according to Ct values in the RT-qPCR assay. Control: hydrated cells under laboratory routine growth conditions; bottom: dried biofilms exposed to Mars-like atmosphere; top: dried biofilms exposed to Mars-like UV flux (5.7 × 105 kJ/m2) and Mars-like atmosphere.
Cell Viability of Dried Biofilms Under Mars-Like UV Flux
In hydrated Chroococcidiopsis cells, the staining with the cell-impermeant SYTOX-Green stain revealed a few dead cells with damaged cell membranes showing SYTOX-Green stained nucleoids and red photosynthetic pigment autofluorescence (Figure 4A). In 7-year dried biofilms mixed with P-MRS and exposed to 5.5 × 103 kJ/m2 of a Mars-like UV, about 20% of the alive cells were SYTOX-Green negative (Figure 4B). Only dead cells showing SYTOX-Green stained nucleoids and red photosynthetic pigment autofluorescence occurred in 7-year dried biofilms mixed with P-MRS and exposed to 1.4 × 105 kJ/m2 and 2.7 × 105 kJ/m2 (not shown) as well as to 5.5 × 105 kJ/m2 (Figure 4C).
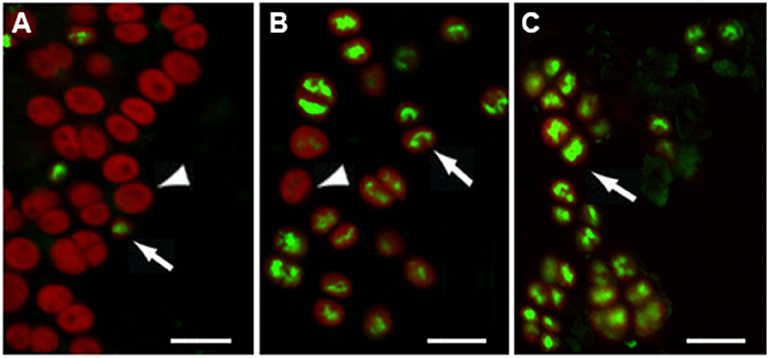
Figure 4. Viability of 7-year dried Chroococcidiopsis biofilms mixed with P-MRS exposed to increasing Mars-like UV radiation doses, according to SYTOX-Green staining. Liquid control cultures showing live, SYTOX-Green-negative cells (arrowhead) and a few dead, SYTOX-green-positive cells (arrow) (A); dried biofilms exposed to 5.5 × 103 kJ/m2 showing live, SYTOX-negative cells (arrowhead); (B); biofilms exposed to 5.5 × 105 kJ/m2 showing dead, SYTOX-green positive cells with SYTOX-green stained nucleoids (arrow) and chlorophyll autofluorescence (C). Scale bar = 10 μm.
Discussion
In order to investigate the role of trehalose and sucrose in survivability and biomarker preservation under Mars laboratory simulations of the anhydrobiotic cyanobacterium Chroococcidiopsis, an in silico analysis of the genome of the CCMEE 029 strain was performed. The genome survey identified genes for trehalose synthesis according to the TreY/TreZ and TreS pathways as well as genes for sucrose synthesis according to the SPS/SPP and SUS pathways. The presence of the treY and treZ genes provided Chroococcidiopsis with the capability of catalyzing a two-step reaction to convert maltodextrins (maltooligosaccharides, glycogen, and starch) into trehalose. But the absence of a trehalase gene (treH) might contribute to trehalose accumulation. Indeed, the treH gene absence was reported for two desiccation-tolerant Leptolyngbya strains (Shimura et al., 2015; Murik et al., 2017) although treH mutants of Anabaena sp. PCC 7120 showed an increased trehalose amount and enhanced desiccation tolerance (Higo et al., 2006). The presence of two treS genes, encoding a long and short trehalose synthase, provided Chroococcidiopsis with the capability of transforming maltose into trehalose, according to the TreS pathway (Klähn and Hagemann, 2011). The genome survey of Gloeocapsopsis sp. UTEX B3054 and Leptolyngbya ohadii (Murik et al., 2017; Urrejola et al., 2019) revealed the presence of two treS gene, a feature suggested to be unique of desiccation-tolerant cyanobacteria (Murik et al., 2017). The long treS gene of Chroococcidiopsis sp. CCMEE 029 showed motifs encoding maltogenic amylase (IPR032091), maltokinase (IPR012811), and protein kinase (IPR011009) domains, responsible for trehalose production through glycogen degradation, that occurred in the long TreS homolog of Leptolyngbya ohadii (Murik et al., 2017).
The presence the sps and sps genes encoding a sucrose–phosphate synthase and sucrose–phosphate phosphatase provided Chroococcidiopsis with the capability of using the SPS/SPP sucrose biosynthetic pathway (Kolman et al., 2015). But the presence of two sus genes encoding a sucrose synthase also suggests the presence of the SUS pathway. Remarkably, Sus-encoding genes were identified during the genome survey of a selection of heterocyst-forming cyanobacteria, and they were present in the genome of a few unicellular cyanobacteria (Kolman et al., 2012). Indeed, representatives of the Chroococcidiopsis genus and heterocyst-differentiating cyanobacteria have been reported to be each other's closest living relatives (Fewer et al., 2002).
The relevance of trehalose and sucrose accumulation in the Chroococcidiopsis adaptation to dryness was supported by the expression of the identified genes during 10 and 60 min of desiccation over silica gel. Compared to the treY gene, the expression of the treZ gene was slightly higher after 60 min of dehydration, in agreement with the trehalose hydrolytic release (Avonce et al., 2006). The expression of the TreS-short encoding gene was 2-fold higher compared to TreS-long encoding gene as previously noticed in Leptolyngbya ohadii (Murik et al., 2017). Among the identified sucrose biosynthetic, the expression of sps, sps, and susB gens was induced after 10 and 60 min of desiccation over silica, whereas the susA gene was slightly induced only in 10 min dried cells. The low expression of this sucrose degradation–encoding gene might have contributed to increased sucrose content during desiccation.
Overall, the presence of multiple biosynthetic pathways might confer the advantage of accumulating sugars relevant for dryness adaptation under changeable environmental conditions leading to limited availability of a given substrate. As a result of this genomic feature, Chroococcidiopsis cells dried over silica gel for 3 months showed a trehalose and sucrose content about 33-fold (38 nmol/mg dry weight) and 5-fold (106 nmol/mg dry weight) increased compared to hydrated cells. Cells air-dried for 24 h had a trehalose and sucrose content 30- and 4-fold increased.
Indeed, a different level of desiccation tolerance might be reflected in terms of compatible solute content. For example, Desmonostoc salinum CCM-UFV059 dried on silica gel had a trehalose and sucrose content of 40 and 15 nmol/mg dry weight, respectively (Viggiano de Alvarenga et al., 2020). Anabaena sp. PCC7120 did not recover desiccation on silica gel and showed no changes in sucrose content (Viggiano de Alvarenga et al., 2020), did not recover after 8 months of air-drying (Yoshimura et al., 2006), and showed a low trehalose amount (0.05–0.1% of dry weight) and a higher sucrose accumulation (1–2% of dry weight) (Higo et al., 2006).
In Chroococcidiopsis, the accumulation of trehalose and sucrose upon desiccation might have played a key role in cell survival and biomarker permanence in 7-year dried biofilms mixed with P-MRS and exposed to Mars laboratory simulations. Survivors were identified by SYTOX-Green staining after exposure to 5.5 × 103 kJ/m2 of a Mars-like UV flux (Figure 4). It was previously reported that dried biofilms survived 1.5 × 103 kJ/m2 of a Mars-like UV flux (Baqué et al., 2013; Mosca et al., 2019), and dried cells mixed with Mars regolith simulants died under 5.7 × 103 kJ/m2 of a Mars-like UV flux (Baqué et al., 2016). Hence, in the present work, a tight contact between cells and minerals within the biofilm structure should have guaranteed the shielding against 5.5 × 103 kJ/m2 of a Mars-like UV flux. Moreover, the occurrence of live cells in 7-year dried biofilms mixed with P-MRS exposed to 5.5 × 103 kJ/m2 of a Mars-like UV (Figure 4), corresponding to 4 days on the Martian surface (Cockell et al., 2000), further supports the possibility that, during Mars's climatic history, a biofilm-like life form mixed with minerals could have survived a few hours' exposure to Mars UV flux while being transported from one protected niche to another (Westall et al., 2013).
On the other hand, although only dead cells occurred after 5.5 × 105 kJ/m2, they showed the permanence of biomarkers: 16S RNA as revealed by RT-qPCR (Figure 2) and chlorophyll and genomic DNA as shown by CLSM analysis (Figure 4).
The drying process caused a decrease in the 16S rRNA copy number compared to hydrated cells although no further decrease occurred in 7-year dried biofilms mixed with P-MRS and exposed to increasing Mars-like UV (Figure 2). This might be due, in addition to the biofilm structure (Baqué et al., 2013), to the UV shielding provided by P-MRS and the trehalose presence acting as a free-radical scavenger (Benaroudj et al., 2001).
Moreover, the RNA stability was further reduced by 7 years of air-dried storage and, to a larger extent, by the exposure to Mars-like UV flux. In fact, 7-year dried biofilms mixed with P-MRS and exposed to a Mars-like UV flux (5.5 × 105 kJ/m2) combined with a Mars-like atmosphere, showed an overall reduced copy number of a selection of mRNAs (Figure 3), corresponding to the induced expression of the sucrose and trehalose biosynthetic genes upon desiccation (Figure 1). For example, compared to dried samples kept in the dark, i.e., in the bottom-layer carrier of the exposure facility, a reduction of the copy number of the sps mRNA occurred in 7-year dried biofilms mixed with P-MRS and exposed to 5.5 × 105 kJ/m2, i.e., in the top-layer carrier of the exposure facility (Figure 3).
These results are relevant because 5.5 × 105 kJ/m2 (200–400 nm) corresponds to the UV dose expected during 1 year of exposure in low Earth orbit, a period of time generally planned for astrobiological experiments outside the International Space Station (de Vera and The Life Detection Group of BIOMEX/BIOSIGN, 2019; de Vera et al., 2019). Moreover, because this dose corresponds to 383 sols (half-year) on the Martian surface (Cockell et al., 2000), the detectability of biomarkers, such as RNA, genomic DNA, and chlorophyll, in dead biofilms suggests that biological signals might still be preserved over a long period of time if sufficiently stabilized under dryness and protected from UV radiation.
In conclusion, this work contributes to expand our knowledge on the adaptation strategies to extremely dry conditions and suggests that sucrose and trehalose accumulation might reduce macromolecular susceptibility to chemical and physical degradation taking place after cell death (Eigenbrode, 2008). This has implications for future life detection missions on Mars; moreover, the biomarker detectability in biofilms mixed with P-MRS exposed to a Mars-like UV flux followed by 7 years of air-dried storage is of interest in the context of the future BioSigN space mission, which will be performed outside the International Space Station in order to identify suitable biosignatures embedded in planetary analog minerals (de Vera and The Life Detection Group of BIOMEX/BIOSIGN, 2019).
Data Availability Statement
The datasets presented in this study can be found in online repositories. The names of the repository/repositories and accession number(s) can be found below: https://www.ncbi.nlm.nih.gov/genbank/, MT078991, MT078990, MT078993, MT078994, MT078996, MT078989, MT078992, MT078995.
Author Contributions
DB and J-PV supervised the study. CF performed the experiments. MB prepared biofilm samples. SC performed the HPLC analysis. AN carried out the bioinformatic analyses. DB wrote the manuscript. All authors read and approved the final manuscript.
Funding
This research was supported by the Italian Space Agency (Bio-Signatures and habitable niches_Cyanobacteria - BIOSIGN_Cyano; grant 2018-15-U.0 to DB).
Conflict of Interest
The authors declare that the research was conducted in the absence of any commercial or financial relationships that could be construed as a potential conflict of interest.
Acknowledgments
The authors thank CINECA for granting computer time (Application Code HP10CKZEGT) and Elena Romano, Centre of Advanced Microscopy P. B. Albertano, University of Rome Tor Vergata, for her skillful assistance in using the confocal laser scanning microscope. The Mars-laboratory simulations were supported by the European Space Agency for the EXPOSE-R2 space mission and performed by Elke Rabbow at DLR (Cologne, Germany).
References
Aerts, J. W., Riedo, A., Melton, D. J., Martini, S., Flahaut, J., Meierhenrich, U. J., et al. (2020). Biosignature analysis of Mars soil analogs from the Atacama Desert: Challenges and implications for future missions to Mars. Astrobiology 20, 766–784. doi: 10.1089/ast.2019.2063
Alpert, P. (2006). Constraints of tolerance: why are desiccation-tolerant organisms so small or rare? J. Exp. Biol. 209, 1575–1584. doi: 10.1242/jeb.02179
Avonce, N., Mendoza-Vargas, A., Morett, E., and Iturriaga, G. (2006). Insights on the evolution of trehalose biosynthesis. BMC Evol. Biol. 6:109. doi: 10.1186/1471-2148-6-109
Baqué, M., Scalzi, G., Rabbow, E., Rettberg, P., and Billi, D. (2013). Biofilm and planktonic lifestyles differently support the resistance of the desert cyanobacterium Chroococcidiopsis under space and Martian simulations. Orig. Life Evol. Biosph. 43, 377–389. doi: 10.1007/s11084-013-9341-6
Baqué, M., Verseux, C., Böttger, U., Rabbow, E., de Vera, J.-P., and Billi, D. (2016). Preservation of biomarkers from cyanobacteria mixed with Mars-like regolith under simulated Martian atmosphere and UV Flux. Orig. Life Evol. Biosph. 46, 289–310. doi: 10.1007/s11084-015-9467-9
Benaroudj, N., Lee, D. H., and Goldberg, A. L. (2001). Trehalose accumulation during cellular stress protects cells and cellular proteins from damage by oxygen radicals. Int. J. Biol. Chem. 276, 24261–24267. doi: 10.1074/jbc.M101487200
Billi, D. (2009). Subcellular integrities in Chroococcidiopsis sp. CCMEE 029 survivors after prolonged desiccation revealed by molecular probes and genome stability assays. Extremophiles 13:49–57. doi: 10.1007/s00792-008-0196-0
Billi, D., Staibano, C., Verseux, C., Fagliarone, C., Mosca, C., Baqué, M., et al. (2019a). Dried biofilms of desert strains of Chroococcidiopsis survived prolonged exposure to space and Mars-like conditions in low Earth orbit. Astrobiology 19, 1008–1017. doi: 10.1089/ast.2018.1900
Billi, D., Verseux, C., Fagliarone, C., Napoli, A., Baqué, M., and de Vera, J.-P. (2019b). A desert cyanobacterium under simulated Mars-like conditions in low Earth orbit: implications for the habitability of Mars. Astrobiology 19, 158–169. doi: 10.1089/ast.2017.1807
Boston, P. J., Northup, D. N., and Spilde, M. N. (2019). “Long-duration natural storage of viable organisms in geological materials and structures as a model for obliquity over-wintering on Mars” in Mars Extant Life: What's Next? (Houston, TX: Lunar and Planetary Institute). Available online at: https://www.hou.usra.edu/meetings/lifeonmars2019/pdf/
Cockell, C. S. (2015). A 500-year experiment. Astron. Geophys. 56, 1.28–1.29. doi: 10.1093/astrogeo/atv028
Cockell, C. S., Catling, D. C., Davis, W. L., et al. (2000). The Ultraviolet environment of Mars: biological implications past, present, and future. Icarus 146, 343–359. doi: 10.1006/icar.2000.6393
Cosciotti, B., Balbi, B., Ceccarelli, A., Fagliarone, C., Mattei, E., Lauro, S. E., et al. (2019). Survivability of anhydrobiotic cyanobacteria in salty ice: implications for the habitability of icy worlds. Life 9:86. doi: 10.3390/life9040086
Crowe, J. H., Carpenter, J. F., and Crowe, L. M. (1998). The role of vitrification in anhydrobiosis. Annu. Rev. Physiol. 60, 73–103. doi: 10.1146/annurev.physiol.60.1.73
Crowe, J. H., Hoekstra, F. A., and Crowe, L. M. (1992). Anhydrobiosis. Annu. Rev. Physiol. 54, 579–599. doi: 10.1146/annurev.ph.54.030192.003051
Cuccuru, G., Orsini, M., Pinna, A., Sbardellati, A., Soranzo, N., Travaglione, A., et al. (2014). Orione, a web-based framework for NGS analysis in microbiology. Bioinformatics 30, 1928–1929. doi: 10.1093/bioinformatics/btu135
Davila, A. F., and Schulze-Makuch, D. (2016). The last possible outposts for life on Mars. Astrobiology 16, 159–168. doi: 10.1089/ast.2015.1380
de Vera, J. P., Alawi, M., Backhaus, T., Baqué, M., Billi, D., Böttger, U., et al. (2019). Limits of life and the habitability of Mars: the ESA space experiment BIOMEX on the ISS. Astrobiology 1, 145–157. doi: 10.1089/ast.2018.1897
de Vera, J. P., and The Life Detection Group of BIOMEX/BIOSIGN. (2019). “A systematic way to life detection: combining field, lab and space research in low Earth orbit” in Biosignatures for Astrobiology. Advances in Astrobiology and Biogeophysics, eds B. Cavalazzi and F. Westall F (Cham: Springer), 111–122.
Eigenbrode, J. L. (2008). Fossil lipids for life-detection: a case study from the early earth record. Space Sci. Rev. 135, 161–185. doi: 10.1007/s11214-007-9252-9
Fagliarone, C., Mosca, C., Ubaldi, I., Verseux, C., Baqué, M., Wilmotte, A., et al. (2017). Avoidance of protein oxidation correlates with the desiccation and radiation resistance of hot and cold desert strains of the cyanobacterium Chroococcidiopsis. Extremophiles 21, 981–991. doi: 10.1007/s00792-017-0957-8
Fairén, A. G., Davila, A. F., Lim, D., Bramall, N., Bonaccorsi, R., Zavaleta, J., et al. (2010). Astrobiology through the ages of Mars: the study of terrestrial analogues to understand the habitability of Mars. Astrobiology 10, 821–843. doi: 10.1089/ast.2009.0440
Fewer, D., Friedl, T., and Büdel, B. (2002). Chroococcidiopsis and heterocysts-differentiating cyanobacteria are each other's closest living relatives. Mol. Phylogenet. Evol. 23, 82–90. doi: 10.1006/mpev.2001.1075
Friedmann, E. I., Kappen, L., Meyer, M. A., and Nienow, J. A. (1993). Long-term productivity in the cryptoendolithic communities of the Ross Desert, Antarctica. Microb. Ecol. 25, 51–69. doi: 10.1007/BF00182129
Higo, A., Katoh, H., Ohmori, K., Ikeuchi, M., and Ohmori, M. (2006). The role of a gene cluster for trehalose metabolism in dehydration tolerance of the filamentous cyanobacterium Anabaena sp. PCC 7120. Microbiology 152, 979–987. doi: 10.1099/mic.0.28583-0
Horneck, G., Bücker, H., and Reitz, G. (1994). Long-term survival of bacterial spores in space. Adv. Space Res. 14, 41–45. doi: 10.1016/0273-1177(94)90448-0
Jorge-Villar, S. E., and Edwards, H. G. (2013). Microorganism response to stressed terrestrial environments: a Raman spectroscopic perspective of extremophilic life strategies. Life 3, 276–294. doi: 10.3390/life3010276
Klähn, S., and Hagemann, M. (2011). Compatible solute biosynthesis in cyanobacteria. Environ. Microbiol. 13, 551–562. doi: 10.1111/j.1462-2920.2010.02366.x
Kolman, M. A., Nishi, C. N., Perez-Cenci, M., and Salerno, G. L. (2015). Sucrose in Cyanobacteria: from a salt-response molecule to play a key role in nitrogen fixation. Life 5, 102–126. doi: 10.3390/life5010102
Kolman, M. A., Torres, L. L., Martin, M. L., and Salerno, G. L. (2012). Sucrose synthase in unicellular cyanobacteria and its relationship with salt and hypoxic stress. Planta 235, 955–964. doi: 10.1007/s00425-011-1542-5
Liu, T., Zhu, L., Zhang, Z., Huang, H., Zhang, Z., and Jiang, L. (2017). Protective role of trehalose during radiation and heavy metal stress in Aureobasidium subglaciale F134. Sci. Rep. 7:17586. doi: 10.1038/s41598-017-15489-0
Martins, Z., Cottin, H., Kotler, J. M., Carrasco, N., Cockell, C. S., de la Torre Noetzel, R., et al. (2017). Earth as a tool for astrobiology - a European perspective. Space Sci. Rev. 209, 43–81. doi: 10.1007/s11214-017-0369-1
McKay, C. P. (2010). An origin of life on Mars. Cold Spring Harb. Perspect. Biol. 2:a003509. doi: 10.1101/cshperspect.a003509
Mosca, C., Rothschild, L. J., Napoli, A., Ferré, F., Pietrosanto, M., Fagliarone, C., et al. (2019). Over-expression of UV-damage DNA repair genes and ribonucleic acid persistence contribute to the resilience of dried biofilms of the desert cyanobacetrium Chroococcidiopsis exposed to Mars-like UV flux and long-term desiccation. Front. Microbiol. 10:2312. doi: 10.3389/fmicb.2019.02312
Murik, O., Oren, N., Shotland, Y., Raanan, H., Treves, H., Kedem, I., et al. (2017). What distinguishes cyanobacteria able to revive after desiccation from those that cannot: the genome aspect. Environ. Microbiol. 19, 535–550. doi: 10.1111/1462-2920.13486
Pinto, F., Pacheco, C. C., Ferreira, D., Moradas-Ferreira, P., and Tamagnini, P. (2012). Selection of suitable reference genes for RT-qPCR analyses in cyanobacteria. PLoS ONE 7:e34983. doi: 10.1371/journal.pone.0034983
Rabbow, E., Rettberg, P., Parpart, A., Panitz, C., Schulte, W., Molter, F., et al. (2017). EXPOSE-R2: the astrobiological ESA mission on board of the International Space Station. Front. Microbiol. 8:1533. doi: 10.3389/fmicb.2017.01533
Schulze-Makuch, D., Airo, A., and Schirmack, J. (2017). The adaptability of fife on Earth and the diversity of planetary habitats. Front. Microbiol. 16:8. doi: 10.3389/fmicb.2017.02011
Seemann, T. (2014). Prokka: rapid prokaryotic genome annotation. Bioinformatics 30, 2068–2069. doi: 10.1093/bioinformatics/btu153
Shimura, Y., Hirose, Y., Misawa, N., Osana, Y., Katoh, H., Yamaguchi, H., et al. (2015). Comparison of the terrestrial cyanobacterium Leptolyngbya sp. NIES-2104 and the freshwater Leptolyngbya boryana PCC 6306 genomes. DNA Res. 22, 403–412. doi: 10.1093/dnares/dsv022
Sun, W. Q., and Leopold, C. A. (1997). Cytoplasmic vitrification and survival of anhydrobiotic organisms. Comp. Biochem. Phys. A 117, 327–333. doi: 10.1016/S0300-9629(96)00271-X
Ulrich, N., Nagler, K., Laue, M., Cockell, C. S., Setlow, P., and Moeller, R. (2018). Experimental studies addressing the longevity of Bacillus subtilis spores – the first data from a 500-year experiment. PLoS ONE 13:e0208425. doi: 10.1371/journal.pone.0208425
Urrejola, C., Alcorta, J., Salas, L., Vásquez, M., Polz, M. F., Vicuña, R., et al. (2019). Genomic features for desiccation tolerance and sugar biosynthesis in the extremophile Gloeocapsopsis sp. UTEX B3054. Front Microbiol. 10:950. doi: 10.3389/fmicb.2019.00950
Viggiano de Alvarenga, L., Lucius, S., Vaz, M., Araújo, W., and Hagemann, M. (2020). The novel strain Desmonostoc salinum CCM - UFV059 shows higher salt and desiccation resistance compared to the model strain Nostoc sp. PCC7120. J. Phycol. 56, 496–506. doi: 10.1111/jpy.12968
Warren-Rhodes, K. A., Rhodes, K. L., Pointing, S. B., Ewing, S. A., Lacap, D. C., Gómez-Silva, B., et al. (2006). Hypolithic cyanobacteria, dry limit of photosynthesis, and microbial ecology in the hyperarid Atacama Desert. Microb. Ecol. 52, 389–398. doi: 10.1007/s00248-006-9055-7
Westall, F., Loizeau, D., Foucher, F., Bost, N., Betrand, M., Vago, J., et al. (2013). Habitability on Mars from a microbial point of view. Astrobiology 13, 887–897. doi: 10.1089/ast.2013.1000
Wilhelm, M. B., Davila, A. F., Parenteau, M. N., Jahnke, L. L., Abate, M., Cooper, G., et al. (2018). Constraints on the metabolic activity of microorganisms in Atacama surface soils inferred from refractory biomarkers: implications for Martian habitability and biomarker detection. Astrobiology 18, 955–966. doi: 10.1089/ast.2017.1705
Keywords: biosignatures, anhydrobiosis, Mars simulation, desert cyanobacteria, life detection
Citation: Fagliarone C, Napoli A, Chiavarini S, Baqué M, de Vera J-P and Billi D (2020) Biomarker Preservation and Survivability Under Extreme Dryness and Mars-Like UV Flux of a Desert Cyanobacterium Capable of Trehalose and Sucrose Accumulation. Front. Astron. Space Sci. 7:31. doi: 10.3389/fspas.2020.00031
Received: 28 March 2020; Accepted: 22 May 2020;
Published: 14 July 2020.
Edited by:
John Robert Brucato, National Institute of Astrophysics (INAF), ItalyReviewed by:
Victor Parro, Centro de Astrobiología (CSIC-INTA), SpainOliver Strbak, Comenius University, Slovakia
Copyright © 2020 Fagliarone, Napoli, Chiavarini, Baqué, de Vera and Billi. This is an open-access article distributed under the terms of the Creative Commons Attribution License (CC BY). The use, distribution or reproduction in other forums is permitted, provided the original author(s) and the copyright owner(s) are credited and that the original publication in this journal is cited, in accordance with accepted academic practice. No use, distribution or reproduction is permitted which does not comply with these terms.
*Correspondence: Daniela Billi, YmlsbGkmI3gwMDA0MDt1bmlyb21hMi5pdA==