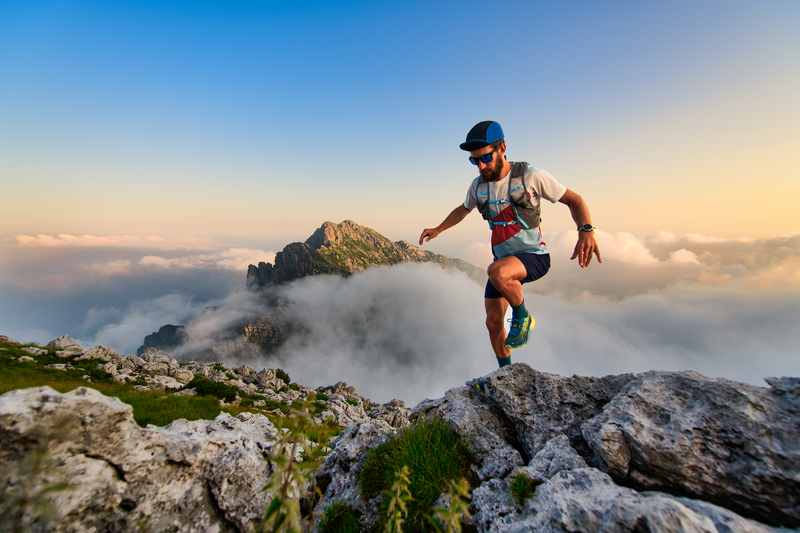
94% of researchers rate our articles as excellent or good
Learn more about the work of our research integrity team to safeguard the quality of each article we publish.
Find out more
ORIGINAL RESEARCH article
Front. Astron. Space Sci. , 22 April 2020
Sec. Astrobiology
Volume 7 - 2020 | https://doi.org/10.3389/fspas.2020.00014
This article is part of the Research Topic Astrobiology of Mars, Europa, Titan and Enceladus - Most Likely Places for Alien Life View all 7 articles
Water from the plumes of icy moons is a potential sampling target that may preserve the aqueous chemistry of the interior oceans, as well as organics and other biosignatures or prebiotic chemical precursors. Water within the plumes will be modified both as it is transported far above the moon's surface, and as samples are taken of the plume water. Plume water is found to freeze rapidly by evaporation and sublimation, increasing the concentration of solutes by a factor of 20%. Organics within samples of the plume water are also potentially subject to significant alteration as they are collected, particularly oxidation resulting from reaction with oxidants entrained on ejection in the plume or formed via breakdown of H2O on the surface as the drops are exposed in space. Mitigation of this oxidation will be critical to successful sample collection.
The discovery of a water plume at Europa (Roth et al., 2014) and at Enceladus (Hansen et al., 2006) both provide an opportunity for the first direct sampling of liquid water from the outer Solar System (with potential for sample return). Liquid water is believed to be highly important for the development of life on other worlds, and hence such a discovery—and the accompanying analyses—could identify how habitable these moons might be (e.g., McKay et al., 2014; Barge and White, 2017).
The Enceladus plume has been characterized as having an alkaline pH (Glein et al., 2015), with low redox conditions, consistent with both carbonate and ammonia present within the plume water (a redox potential of about −0.5 V, see Postberg et al., 2011). The source of the water has been postulated to be in direct contact with rock based on its salinity (Glein and Shock, 2010), and on the presence of H2, likely from serpentinization (Waite et al., 2017).
The surface of Enceladus is generally characterized as being pure water ice (Souček et al., 2016), with trace amounts of reduced compounds such as ammonia and tholins (Hendrix et al., 2010). Plume particles reach sizes of a few μm in radius (Ingersoll and Ewald, 2011) and are ejected at speeds of hundreds of m/s to km/s (Dong et al., 2011; Spencer and Nimmo, 2013).
The discovery of the Europa plume is comparatively recent, and postdates active Jovian space missions, hence little is known about the Europan plume water composition. The Europa plume appears to be transient lasting only days to months, and may be driven by tides (Roth et al., 2014; Rhoden et al., 2015; Sparks et al., 2016). The source region of the plume is likely subsurface, and may be the ocean, or beneath the icy crust from melt pockets, or some intermediate region with mixing between the two. If the source is the ocean, then the plume may provide a glimpse as to the global habitability of Europa, by constraining the oceanic conditions (pH, redox conditions, solutes and salt content), as well as organic content.
The crust of Europa consists primarily of water ice, as well as oxidants such as hydrogen peroxide (H2O2), molecular oxygen (O2), and dark material consisting of some yet-unidentified hydrated salts (Carlson et al., 1999; McCord et al., 1999). These salts may consist of sulfate minerals (Orlando et al., 2005) or hydrated sulfuric acid (e.g., Maynard-Casely et al., 2014). The surface of Europa is hence very oxidizing with up to a few percent of strong oxidants, and organic compounds at the surface should be unstable, decaying to CO2 or carbonate salts, though the timescales of such reactions may be long.
In contrast to the surface, less is known about the composition of Europa's subsurface ocean, though a variety of models have sought to determine the minerals and solutes present in the ocean, as well as possible pH and redox conditions (e.g., Kargel et al., 2000). Prior work (Pasek and Greenberg, 2012) predicted an acidic ocean if surficial oxidants react with hydrothermally-released reducing agents such as H2S on a large scale. Alternatively, the ocean may be alkaline or neutral if transport of oxidants from the surface to the subsurface does not occur, or if the rocky ocean floor can buffer acid generation by dissolving into the ocean (Vance et al., 2016).
Whatever the source region of the plume material (ocean and/or icy crust), the material will be modified during ejection. Depressurization of the material results in evaporation and sublimation of the water, which in turn is coupled to heat loss and freezing. Loss of mass results in a higher net concentration of non-volatile solutes such as salts in the frozen droplet.
Collection of this plume material by spacecraft may further modify samples, depending on the collecting method used (Leroux et al., 2008). Collecting samples will likely result in heating of the samples, possibly with melting and vaporization of water. This may modify the material, especially if oxidants have been entrained within the collected particles. Oxidants may alter organic compounds by breaking C-H and C-C bonds, ultimately forming CO2, especially if during collection the temperature increases rapidly and if the oxidants and certain oxidation-promoting catalysts are abundant.
A major oxidant-producing reaction that would be of concern for sample collection is the Fenton reaction (Walling, 1975). In the Fenton reaction, H2O2 reacts to produce free hydroxyl (OH) and peroxyl (OOH) radicals via catalytic transition metals (typically iron, but also copper):
Such a reaction follows a complicated set of reaction steps (Cohen, 1985; Kremer, 1999) that can aggressively oxidize organic compounds in situ, and if enough stoichiometric H2O2 is around, complete oxidation of organics is plausible. Effectively, each reaction of an OH or OOH radical can result in the +1 oxidation of a carbon atom, which range in oxidation state from −4 (methane, CH4) to +3 (oxalic acid, C2O4H2) for organic carbon, and complete oxidation occurs at an oxidation state of +4 (CO2 or carbonate). As an example, the oxidation of formaldehyde—the simplest sugar—requires four OH radicals:
Plume activity on Europa likely ejects a mixture of gas and solid/liquid H2O. Although returning samples of gaseous H2O would be beneficial from the perspective of understanding the history and sources of water on Europa (e.g., D/H and 18O/16O ratios), collection of larger water samples would better sample the source chemistry. If the plume source region includes the subsurface ocean, then water droplets ejected from the plume would bear compositions similar to the ocean, detailing the environmental conditions and potential habitability of Europa's ocean. In this paper I constrain the amount of water that would evaporate or sublime from liquid water droplets after ejection in the plume, and the effects this has on concentration of solutes. Additionally, I determine how much oxidation might occur as the sample is collected and heated and the effects this might have on the organic inventory of the water samples.
Thermodynamic data for water transitions, including heat capacity (CP) as a function of temperature, phase change enthalpies (ΔH), and vapor pressures at saturation (PSat) are retrieved using the thermodynamic equilibrium program HSC Chemistry (version 7.1, Outokompu Research Oy). This code uses the GIBBS energy solver (White et al., 1958) to determine equilibrium concentrations and solve for reaction mass balances, and has been used previously to understand water-rock transitions (Pirim et al., 2014; Herschy et al., 2018), and model Europa ocean chemistry (Pasek and Greenberg, 2012).
The evaporation or sublimation of water drives cooling. Water evaporates so long as the pressure released by vaporizing does not exceed the saturation vapor pressure. The saturation vapor pressure is determined by calculating the mass transfer constant K from the reaction:
Over the range of temperatures (T) of interest (75 K for Enceladus, 135 K for Europa, reaching to 273 K), the vapor pressure (in torr) can be found by:
Evaporation and sublimation cease to remove mass when condensation and deposition on grains equals the evaporation/sublimation rate, v. This occurs when the vapor pressure is equal to the surface pressure of Europa (10−11-10−12 atm, Leblanc et al., 2002), which corresponds to a temperature of about 135 K. At and below this temperature, water ice droplet sublimation is balanced by condensation.
For liquid water at 273 K, evaporative or sublimative cooling results in a phase change to ice, with an enthalpy of fusion (ΔHFus) of 80 cal/g. The mass of water that must vaporize in order to cause the rest of the drop to freeze is thus the balance between the enthalpy of fusion and the enthalpy of vaporization (677.8 cal/g, see below). To this end, mass from a drop of water will sublimate to drive cooling:
where mi is the initial mass of water, and ml is the mass lost by vaporization. After freezing, the enthalpy (ΔHVap) of reaction (4) is calculated as 660 cal/g at 75 K to 677.8 cal/g at 273 K, for an average value of 674.7 cal/g over the temperature range of 135 K and greater. This reaction enthalpy can be contrasted to the heat capacity of ice as a function of temperature to determine the amount of mass lost by sublimation that thereby drives cooling to ambient temperatures (135 K at Europa). Using a temperature-dependent heat capacity (CP) defined as:
the quantity of water (ml) that evaporates can be determined from:
The above equations are time-independent. The rate of evaporation or sublimation is calculated from the Hertz-Knudsen-Langmuir equation:
where v is the rate of evaporation in g/s, αS is the temperature-dependent sublimation coefficient of Kossacki and Leliwa-Kopystynski (2014), M is the molecular mass in g/mol, T is the temperature in K, PSat is the vapor pressure at saturation in torr or mm Hg, PSurf is the pressure above the surface of the drop in torr, and r is the radius of the droplet in question in cm. The 0.0437 corrects for units and includes the Boltzmann constant. Though this evaporation rate equation includes the empirically-derived αS, which has been shown to vary by three orders of magnitude even for water (Persad and Ward, 2016), the estimations here provide approximations of the sublimation rate of droplets that should be accurate to an order of magnitude.
The loss of water as gas thus drives cooling and determines the temperature (T) of the droplet in Equation (v) by the relationship:
where mD is the mass of the remaining droplet. Using a finite difference method to calculate temperature changes and its effect on vapor pressure, heat capacity, and evaporation rate, and mass loss with the associated surface area loss, I calculated the effect of evaporation and sublimation on droplets of various starting sizes.
Another change that may occur to water samples that are subjected to the environments of Jupiter and Saturn is the accumulation of radiation damage and formation of an oxidized crust. These oxides may react with organics to alter them or to completely oxidize them to CO2 upon collection by a spacecraft. This process would be related to the amount of entrained oxides and the exposure timescales of the droplets, followed by their thermal histories (which is a function of droplet size and capture velocity). To this thermal profile I added kinetic calculations associated with the Fenton reaction that produces highly oxidizing OH radicals, to provide an estimate of the fates of organics during collection of water samples at these locations.
The production of peroxide is considered using the H2O2 production rate of Carlson et al. (1999). H2O2 is estimated to be produced at a rate of 2 × 1011 molecules/cm2 s, and is produced primarily by high energy particle bombardment. The number of H2O2 molecules formed on a droplet's surface (NH2O2) is thus estimated as:
where τ is the timescale of exposure of these particles, and the particle bombardment is not assumed to come from Europa, hence the surface area of the particle is calculated as its cross-sectional area. In these simple calculations it is assumed that all high energy particles stop at the surface of the water ice droplets, though a more rigorous model could include variation of penetration depth with respect to particle type (Cooper et al., 2001).
The oxidation of organics by oxidants such as peroxide requires a liquid medium. Although peroxide in ice likely does not oxidize organics due to diffusion limitations and low reactivity at cold temperatures, melting of the droplets via reheating by impact with Europa's surface, or during sample collection, may cause in situ organics to oxidize to CO2 and lose information on their speciation (which is critical to identifying biosignatures). The amount of melting that occurs during collection is calculated from the conversion of kinetic energy to thermal energy via:
with V the velocity in m/s, CP having its temperature dependence as per (iii), and equal to 1 cal/g above 273 K, and ΔHFus being the enthalpy of melting/fusion (requiring 80 cal/g), needed only if temperatures reach 273 K. The velocity required to melt the frozen droplets is ~660 m/s, and to reach the boiling point is ~1,400 m/s.
After heating, the water samples should cool via thermal diffusion as they will likely be in direct contact with a cold collection chamber, on timescales of
where kD is the thermal diffusivity of water (~10−3 cm2/s, James, 1968). Using various sizes, peroxide contents, and a temperature profile that is assumed to decrease linearly over the timescale t from (ix) to reach the ambient temperature (75 or 135 K), the production of oxides from the Fenton reaction is determined by integrating reaction velocities using a kinetic rate law constant k determined as:
where the factor −9,500 is the activation energy divided by R of Lin and Lo (1997), and the frequency factor 3 × 1015 s−1M−1 is calculated from a reaction k equal to 40 at 298 K (Pignatello et al., 2006).
The rate of oxidant [Ox] production is estimated as:
where [H2O2] is defined as the molarity of peroxide from (v), and [Fe2+] is the ferrous iron molarity in solution (estimated as 10−4 M at circumneutral pH, consistent with Fanale et al., 2001). In the Fenton reaction, Fe2+ is regenerated from Fe3+ [reaction (2)] and hence it is the total molarity of iron that controls the production of OH and OOH, the oxidants capable of altering organic carbon.
The oxidation rate is integrated over the timescale from (ix) to provide the total molarity of OH generated from H2O2. This represents a measure of the oxidizing power of the peroxide as the plume samples cool. This oxidant production is then compared to the effect of the oxidants on organic carbon, assuming the carbon begins with an oxidation state of zero [consistent with sugar or formaldehyde, as per reaction (3)]. The oxidation of organics occurs rapidly at room temperature in the presence of transition metal catalysts driven by the Fenton reaction (see Walling, 1975; Pasek et al., 2008), but is otherwise slow (Debellefontaine et al., 1996). This oxidation process is a function of peak temperature, cooling rate, drop size and exposure timescale [from (vii)], and fraction of catalyst present. Oxidation states other than zero are not unreasonable for other biological molecules and range from ~ −2 for hydrocarbons, to +2/+3 for carboxylic acids.
Here I evaluate the effects of the time of exposure of liquid water to low pressure conditions and to oxidizing radiation. Low pressure and temperature conditions result in rapid evaporation and sublimation of water as it freezes and drops to ambient conditions. Drops are estimated to cool rapidly (seconds) and lose ~17% of their mass during this time. Exposure to radiation results in the buildup of oxidants that may degrade organics that were present in the original liquid droplet, especially by reheating during sample collection of these plumes. Oxidant buildup is most relevant if the drops cool slowly and have accumulated significant oxidants during their exposure time.
Water droplets ejected from the plume are frozen rapidly by evaporation of water from their surface. The water droplets lose ~10% of their mass during this process [calculated from Equation (ii)], over a timescale of μs to s, depending on the initial radius of the drop [Figure 1, calculated coupling Equation (ii) with (v)]. The fraction of mass lost is independent of size. The net volume of the drop is changed only slightly, as the loss of 10% of the mass is accompanied by an 8.5% volumetric expansion.
Figure 1. The time required to completely freeze a pure liquid water droplet at 273 K by cooling from evaporation, balancing the mass lost from Equation (ii) with the evaporation rate from Equation (v).
Subsequent sublimation at the surface of the ice droplets also occurs rapidly, and is effectively complete on the order of seconds to minutes [Figure 2, calculated from (vi)]. All droplets eventually reach a 7% mass loss at Europa from sublimation [9% at Enceladus, calculated from (iv)], independent of size, occurring as the ice droplets reach 135 K and ambient pressures (75 K at Enceladus). The net loss of mass due to sublimation and evaporation is thus between 16 and 19%, the sum of the mass lost from Equations (ii) and (iv).
Figure 2. The mass lost as calculated from Equations (v) and (vi) due to sublimation as a function of time for a frozen ice droplet with an initial radius of 10 μm, relative to its initial mass (set as 1). The change in slope at about 0.1s is due to a change in the coefficient of sublimation from 0.15 to 1.0 at 215 K (Kossacki and Leliwa-Kopystynski, 2014). The droplet asymptotically reaches 6–7% mass loss in minutes [as per Equation (iv)].
The production of oxidants such as H2O2 on ice droplets is strongly related to both the time of exposure and the drop size. The mole fraction of H2O2 is shown as Figure 3, with the amount of H2O2 dependent on different exposure timescales (from vii) and on differing droplet sizes as shown, and the molarity equivalent is this mole fraction multiplied by 55.56 (the moles per L of water). Concentrations of peroxide on the order of ~ 1 molar are feasible for smaller droplets after only a few weeks.
Figure 3. The exposure timescale [in seconds, from Equation (vii)] required to produce a specific mole fraction of H2O2 (y-axis) as a function of droplet size (diameter in cm, x-axis). Note that 1 month is about ~106.5 s.
The Fenton reaction is highly effective as an oxidant producer over relatively short timescales (<1,000 s). The production of oxidants by such a reaction is most critical for large droplets that cool slowly and that reach high temperatures during collection. For large droplets, complete breakdown of all H2O2 is expected [due to a slow cooling rate, from (ix)], whereas smaller droplets that cool much faster would have much less OH production (Figure 4). This effectively means that any H2O2 generated by bombardment in the Europa environment will break down into highly reactive OH for the largest droplets, whereas smaller droplets will have less OH generation as they cool faster and H2O2 has less time to react.
Figure 4. The percentage of H2O2 estimated to break down into OH and OOH radicals via the Fenton reaction as a function of droplet size (diameter as given) and peak temperature, as determined from Equations (x) and (xi). When the droplets are large and collected at high velocity, they heat substantially and cool slowly. As a result, the temperature-dependent Fenton reaction is capable of breaking down significant quantities of H2O2 into OH and OOH.
This generation of OH would correspond to a degradation of organics, as per reaction (3). Organics are altered when an OH reacts with a C-H bond to form a C radical (that can then react internally or with other C radicals to polymerize toward tar), and the final product of such a reaction is CO2. This occurs most readily for larger droplets that are slow to cool with a large H2O2 to organic C ratio, but may be pertinent to all droplets if the quantity of H2O2 present is high (for instance, via entrained crustal material). This effect is quantified by tying Equations (x) and (xi) with reaction (3) in Figure 5.
Figure 5. The H2O2 to C content on a mole per mole basis of a drop determines how likely it is to alter the organic inventory of a droplet irrevocably. Effectively, the complete oxidation of an organic compound such as formaldehyde requires a 4:1 or greater H2O2 to C ratio to potentially result in the complete oxidation of such a molecule. However, concentrations higher than 4:1 may still not alter all of the organics within the droplet, as the peak temperature and droplet size both contribute to the generation of reactive oxidant (OH and OOH) material capable of degrading organics. For this diagram, concentrations of H2O2 that greatly exceed the moles of organic carbon are more likely to degrade the organics for large droplets that are heated to high peak temperatures.
Both the Enceladus and Europa plumes present an opportunity to sample water from these potentially habitable moons. It is necessary to constrain possible chemical alteration that may take place after leaving the plume source region so as to determine how representative the samples are of their environment, critical to determining biosignatures and habitability. Key results of this study are that water droplets comprising part of plume are frozen rapidly, with loss of about 17% of the water by evaporation and sublimation if the water is originally liquid and at 273 K. The 17% loss of water corresponds to a 20% increase in the concentration of non-volatile solutes such as salts, if the associated water vapor is not collected. Volatiles, such as gases and clathrates (Mousis et al., 2013), will be altered during sublimation, at least as far as their concentration is concerned.
Of far greater concern are oxidants reacting with organic compounds in the droplets on collection. These oxidants may come from particle bombardment of droplet surfaces after ejection, or alternatively, may be entrained from the oxidizing icy crust as the droplets are expelled especially in the case of Europa. If there is little entrainment, then it is possible that oxidation by H2O2 should not be much of a concern. Small droplets with the highest expected H2O2 are also those that cool the quickest and hence produce little oxidant during capture. Large droplets that can produce more OH from H2O2 should generally have less oxidants if their exposure time is short.
However, if oxidants from the crust are entrained in the droplets during ejection, then organic compounds may be altered, especially in large drops. Europa's ice crust may bear up to 1–3% O2 by mass (Hand et al., 2007) and 0.13% H2O2 (Carlson et al., 1999), implying that if a subsurface droplet is contaminated with even 1% of the icy crust material, then the total organic carbon concentration would have to exceed ~10−3 M to be preserved if all of these oxidants react.
Oxidation of organics will primarily take place when the droplets are liquefied, and if there are transition metals present in the droplets, such as Fe2+ or Cu+. In the presence of transition metals, H2O2 decays to OH radicals, which promote organic breakdown to CO2. These oxidation reactions occur on timescales of tenths of seconds even at room temperature (Duesterberg and Waite, 2006) and are faster still at elevated temperature. Given that most collection methods will heat samples (Leroux et al., 2008), organic compounds may be subjected to oxidation unless the samples are quickly cooled, or the transition metals are removed during collection (perhaps by capture in a chelating gel). Either step could be a potential mitigation against this oxidation effect. However, the result of this oxidation would be an alteration or even complete removal of organic speciation as a biosignature.
Given our current state of knowledge of Europa's plume and its composition, this study is preliminary. Further estimates of plume sample compositional modifications should include better estimates on the kinetics of oxidation of organic compounds, for a variety of organics of astrobiologic interest. Furthermore, experimental investigation of organic compound oxidation rates under the worst-case scenario (high H2O2 with a large, slow-to-cool drop) could corroborate these model results. Additionally, the plume dynamics will control the sample size distribution, which has several significant effects on evaporation/sublimation, and on oxidation. Little as of yet is known about the source region for the plume, the energetics driving the plume, or interactions of the plume material with the icy crust, especially for Europa. Better constraints on these plume features will help constrain the potential modifications of samples prior to analysis.
The datasets generated for this study are available on request to the corresponding author.
The author confirms being the sole contributor of this work and has approved it for publication.
This work was jointly supported by the NSF and the NASA Astrobiology Program, under the NSF Center for Chemical Evolution (CHE-1504217), and through the NASA Emerging Worlds Program (Grant 80NSSC18K0598).
The author declares that the research was conducted in the absence of any commercial or financial relationships that could be construed as a potential conflict of interest.
Barge, L. M., and White, L. M. (2017). Experimentally testing hydrothermal vent origin of life on Enceladus and other icy/ocean worlds. Astrobiology 17, 820–833. doi: 10.1089/ast.2016.1633
Carlson, R. W., Anderson, M. S., Johnson, R. E., Smythe, W. D., Hendrix, A. R., Barth, C. A., et al. (1999). Hydrogen peroxide on the surface of Europa. Science 283, 2062–2064. doi: 10.1126/science.283.5410.2062
Cohen, G. (1985). “The fenton reaction,” in Handbook Methods Oxygen Radical Research, ed R. A. Green (Boca Raton, FL: CRC Press), 55–64.
Cooper, J. F., Johnson, R. E., Mauk, B. H., Garrett, H. B., and Gehrels, N. (2001). Energetic ion and electron irradiation of the icy Galilean satellites. Icarus 149, 133–159. doi: 10.1006/icar.2000.6498
Debellefontaine, H., Chakchouk, M., Foussard, J. N., Tissot, D., and Striolo, P. (1996). Treatment of organic aqueous wastes: wet air oxidation and wet peroxide oxidation®. Environ. Pollut. 92, 155–164. doi: 10.1016/0269-7491(95)00100-X
Dong, Y., Hill, T. W., Teolis, B. D., Magee, B. A., and Waite, J. H. (2011). The water vapor plumes of enceladus. J. Geophys. Res. Space Phys. 116:A10204. doi: 10.1029/2011JA016693
Duesterberg, C. K., and Waite, T. D. (2006). Process optimization of fenton oxidation using kinetic modeling. Environ. Sci. Technol. 40, 4189–4195. doi: 10.1021/es060311v
Fanale, F. P., Li, Y. H., De Carlo, E., Farley, C., Sharma, S. K., Horton, K., et al. (2001). An experimental estimate of Europa's “ocean” composition independent of Galileo orbital remote sensing. J. Geophys. Res. Planets 106, 14595–14600. doi: 10.1029/2000JE001385
Glein, C. R., Baross, J. A., and Waite, J. H. Jr. (2015). The pH of enceladus' ocean. Geochim. Cosmochim. Acta 162, 202–219. doi: 10.1016/j.gca.2015.04.017
Glein, C. R., and Shock, E. L. (2010). Sodium chloride as a geophysical probe of a subsurface ocean on enceladus. Geophys. Res. Lett. 37:L09204. doi: 10.1029/2010GL042446
Hand, K. P., Carlson, R. W., and Chyba, C. F. (2007). Energy, chemical disequilibrium, and geological constraints on Europa. Astrobiology 7, 1006–1022. doi: 10.1089/ast.2007.0156
Hansen, C. J., Esposito, L., Stewart, A. I. F., Colwell, J., Hendrix, A., Pryor, W., et al. (2006). Enceladus' water vapor plume. Science 311, 1422–1425. doi: 10.1126/science.1121254
Hendrix, A. R., Hansen, C. J., and Holsclaw, G. M. (2010). The ultraviolet reflectance of enceladus: implications for surface composition. Icarus 206, 608–617. doi: 10.1016/j.icarus.2009.11.007
Herschy, B., Chang, S. J., Blake, R., Lepland, A., Abbott-Lyon, H., Sampson, J., et al. (2018). Archean phosphorus liberation induced by iron redox geochemistry. Nat. Commun. 9:1346. doi: 10.1038/s41467-018-03835-3
Ingersoll, A. P., and Ewald, S. P. (2011). Total particulate mass in enceladus plumes and mass of saturn's E ring inferred from cassini ISS images. Icarus 216, 492–506. doi: 10.1016/j.icarus.2011.09.018
James, D. W. (1968). The thermal diffusivity of ice and water between– 40 and+ 60°C. J. Mater. Sci. 3, 540–543. doi: 10.1007/BF00549738
Kargel, J. S., Kaye, J. Z., Head, J. W. III., Marion, G. M., Sassen, R., et al. (2000). Europa's crust and ocean: origin, composition, and the prospects for life. Icarus 148, 226–265. doi: 10.1006/icar.2000.6471
Kossacki, K. J., and Leliwa-Kopystynski, J. (2014). Temperature dependence of the sublimation rate of water ice: influence of impurities. Icarus 233, 101–105. doi: 10.1016/j.icarus.2014.01.025
Kremer, M. (1999). Mechanism of the fenton reaction. Evid. N. Intermediat. Phys. Chem. Chem. Phys. 1, 3595–3605. doi: 10.1039/a903915e
Leblanc, F., Johnson, R. E., and Brown, M. E. (2002). Europa's sodium atmosphere: an ocean source? Icarus 159, 132–144. doi: 10.1006/icar.2002.6934
Leroux, H., Rietmeijer, F. J. M., Velbel, M. A., Brearley, A. J., Jacob, D., Langenhorst, F., et al. (2008). A TEM study of thermally modified comet 81P/Wild 2 dust particles by interactions with the aerogel matrix during the stardust capture process. Meteorit. Planet. Sci. 43, 97–120. doi: 10.1111/j.1945-5100.2008.tb00612.x
Lin, S. H., and Lo, C. C. (1997). Fenton process for treatment of desizing wastewater. Water Res. 31, 2050–2056. doi: 10.1016/S0043-1354(97)00024-9
Maynard-Casely, H. E., Brand, H. E. A., and Wallwork, K. S. (2014). Phase relations between the water-rich sulfuric acid hydrates, potential markers of thermal history on Jupiter's icy moons. Icarus 238, 59–65. doi: 10.1016/j.icarus.2014.04.012
McCord, T. B., Hansen, G. B., Shirley, J. H., and Carlson, R. W. (1999). Discussion of the 1.04-μm water ice absorption band in the Europa NIMS spectra and a new NIMS calibration. J. Geophys. Res. Planet. 104, 27157–27162. doi: 10.1029/1999JE001046
McKay, C. P., Anbar, A. D., Porco, C., and Tsou, P. (2014). Follow the plume: the habitability of enceladus. Astrobiology 14, 352–355. doi: 10.1089/ast.2014.1158
Mousis, O., Lakhlifi, A., Picaud, S., Pasek, M., and Chassefière, E. (2013). On the abundances of noble and biologically relevant gases in lake vostok, Antarctica. Astrobiology 13, 380–390. doi: 10.1089/ast.2012.0907
Orlando, T. M., McCord, T. B., and Grieves, G. A. (2005). The chemical nature of Europa surface material and the relation to a subsurface ocean. Icarus 177, 528–533. doi: 10.1016/j.icarus.2005.05.009
Pasek, M. A., and Greenberg, R. (2012). Acidification of Europa's subsurface ocean as a consequence of oxidant delivery. Astrobiology 12, 151–159. doi: 10.1089/ast.2011.0666
Pasek, M. A., Kee, T. P., Bryant, D. E., Pavlov, A. A., and Lunine, J. I. (2008). Production of potentially prebiotic condensed phosphates by phosphorus redox chemistry. Angew. Chem. Int. Ed. 47, 7918–7920. doi: 10.1002/anie.200802145
Persad, A. H., and Ward, C. A. (2016). Expressions for the evaporation and condensation coefficients in the hertz-knudsen relation. Chem. Rev. 116, 7727–7767. doi: 10.1021/acs.chemrev.5b00511
Pignatello, J. J., Oliveros, E., and MacKay, A. (2006). Advanced oxidation processes for organic contaminant destruction based on the Fenton reaction and related chemistry. Crit. Rev. Environ. Sci. Technol. 36, 1–84. doi: 10.1080/10643380500326564
Pirim, C., Pasek, M. A., Sokolov, D. A., Sidorov, A. N., Gann, R. D., and Orlando, T. M. (2014). Investigation of schreibersite and intrinsic oxidation products from sikhote-alin, seymchan, and odessa meteorites and Fe3P and Fe2NiP synthetic surrogates. Geochim. Cosmochim. Acta 140, 259–274. doi: 10.1016/j.gca.2014.05.027
Postberg, F., Schmidt, J., Hillier, J., Kempf, S., and Srama, R. (2011). A salt-water reservoir as the source of a compositionally stratified plume on enceladus. Nature 474, 620–622. doi: 10.1038/nature10175
Rhoden, A. R., Hurford, T. A., Roth, L., and Retherford, K. (2015). Linking Europa's plume activity to tides, tectonics, and liquid water. Icarus 253, 169–178. doi: 10.1016/j.icarus.2015.02.023
Roth, L., Saur, J., Retherford, K. D., Strobel, D. F., Feldman, P. D., McGrath, M. A., et al. (2014). Transient water vapor at Europa's south pole. Science 343, 171–174. doi: 10.1126/science.1247051
Souček, O., Hron, J., Běhounková, M., and Cadek, O. (2016). Effect of the tiger stripes on the deformation of saturn's moon enceladus. Geophys. Res. Lett. 43, 7417–7423. doi: 10.1002/2016GL069415
Sparks, W. B., Hand, K. P., McGrath, M. A., Bergeron, E., Cracraft, M., and Deustua, S. E. (2016). Probing for evidence of plumes on Europa with HST/STIS. Astrophys. J. 829:121. doi: 10.3847/0004-637X/829/2/121
Spencer, J. R., and Nimmo, F. (2013). Enceladus: an active ice world in the saturn system. Ann. Rev. Earth Planet. Sci. 41, 693–717. doi: 10.1146/annurev-earth-050212-124025
Vance, S. D., Hand, K. P., and Pappalardo, R. T. (2016). Geophysical controls of chemical disequilibria in Europa. Geophys. Res. Lett. 43, 4871–4879. doi: 10.1002/2016GL068547
Waite, J. H., Glein, C. R., Perryman, R. S., Teolis, B. D., Magee, B. A., Miller, G., et al. (2017). Cassini finds molecular hydrogen in the enceladus plume: evidence for hydrothermal processes. Science 356, 155–159. doi: 10.1126/science.aai8703
Keywords: plume, oxidation, astrobiology, biosignature detection, Europa - satellite (moon) of the planet Jupiter
Citation: Pasek MA (2020) Plume Sample Modification at Icy Moons: Implications for Biosignatures. Front. Astron. Space Sci. 7:14. doi: 10.3389/fspas.2020.00014
Received: 13 January 2020; Accepted: 30 March 2020;
Published: 22 April 2020.
Edited by:
Isik Kanik, NASA Jet Propulsion Laboratory (JPL), United StatesReviewed by:
Henry Sun, Desert Research Institute (DRI), United StatesCopyright © 2020 Pasek. This is an open-access article distributed under the terms of the Creative Commons Attribution License (CC BY). The use, distribution or reproduction in other forums is permitted, provided the original author(s) and the copyright owner(s) are credited and that the original publication in this journal is cited, in accordance with accepted academic practice. No use, distribution or reproduction is permitted which does not comply with these terms.
*Correspondence: Matthew A. Pasek, bXBhc2VrQHVzZi5lZHU=
Disclaimer: All claims expressed in this article are solely those of the authors and do not necessarily represent those of their affiliated organizations, or those of the publisher, the editors and the reviewers. Any product that may be evaluated in this article or claim that may be made by its manufacturer is not guaranteed or endorsed by the publisher.
Research integrity at Frontiers
Learn more about the work of our research integrity team to safeguard the quality of each article we publish.