- 1Departamento de Física Teórica, Universidad Autónoma de Madrid, Madrid, Spain
- 2Instituto de Física Teórica UAM-CSIC, Campus de Cantoblanco, Madrid, Spain
We briefly review the general insight of the indirect searches of dark matter. We discuss the primary equation in a three-level multimessenger approach (gamma rays, neutrinos, and antiprotons), and we introduce the reader to the main topics and related uncertainties (e.g., dark matter density distribution, cosmic rays, particle physics). As an application of the general concept, we focus on the multi-TeV dark matter candidate among other weak interactive massive particles. We present the state-of-the-art on this sub-field, and we discuss open questions and experimental limitations.
1. Introduction: Dark Matter, an Open Question
More than 80 years ago, Zwicky applied the virial theorem to the Coma Cluster and determined that a large amount of non-luminous matter must be present to keep the system bound together (Zwicky, 1933); nearly 40 years later, Rubin observed similar gravitational evidence by studying the rotation curve of spiral galaxies (Rubin and Thonnard, 1980; Broeils, 1992; Persic et al., 1996). From then on, many astrophysical and cosmological evidence hints at some inconsistencies in our understanding of the Universe as a whole. Many theories have been proposed in order to account for the gravitational observations: they include both modified gravity (e.g., Milgrom, 2015) or a dark component of matter (Bradac et al., 2006; Ade et al., 2014, 2016; Lage and Farrar, 2014). In particular, the need for non-baryonic Dark Matter (DM) is favored by a variety of independent estimates of the matter density in the Universe, that points to a value larger than the value provided by baryons alone, according to nucleosynthesis (see e.g., Bergstrom, 2000 for a general overview). DM candidates cover a broad range of masses from 10−35 to 1018 GeV (Gardner and Fuller, 2013) (see e.g., Bertone et al., 2005). Among them, and beyond the Standard Model (SM) of particle physics, the Weak Interactive Massive Particle (WIMP) represents one plausible candidate, compatible with both cosmological constraints and large-scale structure (galaxies and galaxy clusters) formation and evolution models and simulations (Naab and Ostriker, 2017). In particular, TeV WIMP stands as an open possibility and one of the next frontiers for the DM community (Livio and Silk, 2014). Up to masses of 100 TeV, DM candidates still conserve cosmological properties of thermal candidates. Thermal relics were as abundant as photons in the primordial hot plasma, being freely created and destructed in pairs in the thermal bath. Due to the cooling of the Universe, their relative number density then started to be suppressed as annihilations proceeded. When the temperature dropped below their mass, the annihilation processes froze out and their final abundance would be the observed 27% of the whole content of the Universe at present (Ade et al., 2014, 2016).
WIMPs searches based on different approaches and methodologies, have been developed in order to investigate different energy scales. They are commonly classified in three main classes: experiments of direct and indirect searches of DM and colliders. DM searches at colliders, such as the Large Hadron Collider (LHC) among others (Munoz, 2004; Bertone, 2010; Penning, 2018), focus on the possibility to produce DM particles through the interaction of two SM particles and the subsequent production of unknown particles (SM-SM → DM-DM, see e.g., Figure 2.1 of Gammaldi (2015) for a schematic visualization of different processes). Due to both experimental and theoretical limitations, the highest particle mass that can be studied at this experiment is a few TeV, and they are strongly dependent on the particle physics model of interest (Hong, 2017). Therefore, the study of particle physics nature of the multi-TeV DM candidate at colliders is a challenge and represents a new frontier in physics. Similar limitations affect the experiments of direct searches (Drukier and Stodolsky, 1984; Goodman and Witten, 1985; Bertone, 2010; Baudis, 2014). The latter are underground experiments designed to investigate the SM-DM → SM-DM interaction, that is, the scattering angle between the prospective DM particles within the Milky Way halo with heavy nuclei. This kind of experiment mainly addresses the DM particle mass of 1−104 GeV depending on the spin dependence (Munoz, 2004; Bertone, 2010; Marrodan Undagoitia and Rauch, 2016). The DAMA Collaboration claimed for a periodic signal that could be explained with a DM particle mass of a few (tens) GeV (Bernabei et al., 2008, 2013, 2018; Baum et al., 2019). However, strong tension emerges between the DAMA/NaI and DAMA/LIBRA claim and the null results from several underground experiments (Savage et al., 2009), such as CDMS (Ahmed et al., 2009), XENON10 (Angle et al., 2008), CRESST I (Lang and Seidel, 2009), CoGeNT (Aalseth et al., 2008), TEXONO (Lin et al., 2009), and Super-Kamiokande (SuperK)(Desai et al., 2004).
Searches of multi-TeV DM candidates can be addressed by means of cosmic-ray experiments, that allow the energy range of a few MeV to PeV to be investigated. In particular, detectors of very high energy (VHE) cosmic rays investigate the TeV energy scale. Indirect searches of DM focus on the DM-DM → SM-SM interaction, that is the production of SM particles by DM annihilation or decay events in astrophysical targets (Gammaldi, 2015), with a process similar to that taking place in the primordial plasma before particle decoupling. The benchmark thermal annihilation cross-section is and the decay half-life is tuned to . WIMPs annihilate or decay into SM particles, which then produce secondary fluxes of cosmic rays (gamma-rays, neutrinos, antimatter) that are collected by detectors. These class of searches are independent of the particle physics model, and only depend on the energy of the primary annihilation/decay event. The multimessenger approach for DM searches implies the collection of complementary information given by different cosmic rays and experiments.
In this review, we focus on a subclass of cosmic-ray experiments. We briefly introduce the reader to the fundamentals of the multimessenger approach for the indirect search of WIMPs (section 2). In particular, in section 3 we will discuss recent results of TeV DM studies. We provide very general information about brane world theory as a possibility for multi-TeV DM candidates in section 4. Finally, we will tray the main conclusions and the prospective of future studies in section 5.
2. Multimessenger Approach to Indirect Searches of DM
The multimessenger approach is the next frontier for DM searches. Signatures of DM annihilation or decay events in astrophysical sources may be observed in cosmic-ray fluxes by Cerenkov telescopes such as VERITAS (Park and VERITAS Collaboration, 2015), HESS (Gottschall et al., 2015), MAGIC(Sitarek et al., 2015), HAWC (Mostafa, 2014), and CTA (Maier et al., 2017); neutrino telescopes such as ANTARES (Bouwhuis and for the ANTARES collaboration, 2009) or IceCube (Chianese, 2017); satellites such as PAMELA (Galper and Spillantini, 2018), AMS (Cuoco et al., 2017), and Fermi (Collaboration, 2012) or balloon experiments like CAPRICE (Ambriola et al., 1999) or BESS (Mitchell, 2010) (for a general overview see e.g., Gaskins, 2016). The secondary products of annihilation and decay of DM particles contribute to the cosmic-ray differential flux as (Gammaldi, 2016a):
where:
• ηcr depends on the secondary particles of interest (cosmic rays) and their propagation; ηcr = 1 for gamma rays, otherwise it depends on neutrino oscillations or the velocity of the charged particle for antimatter studies;
• The total flux is given by decay (a = 1) and/or annihilation (a = 2) events of DM particle into the i-th SM particle (annihilation/decay channel). The ζ factor discerns between these two cases: and are, respectively the inverse of the decay time and thermal averaged annihilation cross section times velocity. The probability that DM annihilates or decays into the i-th channel depends on the nature of DM;
• The differential number of cosmic rays produced at the source by subsequent events of annihilation or decay of SM particles is simulated by means of Monte Carlo events generator software, such as PYTHIA or HERWIG. A Particle Physicist Cookbook for Dark Matter (PPPC4DM) (Cirelli et al., 2011) provides the cosmic-ray fluxes for an immediate application. In particular, electroweak corrections are important for multi-TeV events (Ciafaloni et al., 2011). Some uncertainties may be introduced in the evaluation of both the and κcr factor due to the choice of the Fortran or C++ versions of PYTHIA or HERWIG software, as discussed in Cembranos et al. (2013a) for gamma-ray fluxes;
• The κcr factor depends on the astrophysics of DM distribution as well as on the cosmic-ray propagation. For neutral cosmic-rays (n-cr) (e.g., gamma rays and neutrinos) it is the so-called astrophysical J-factor
Here, ρ(r) is the DM density distribution. The line of sight (l.o.s) l is the distance from the observer to any observed source. The radial distance r from the center of the target to a given point inside it, is related to l by r2 = l2 + d2 − 2dlcosα, where d is the distance from the Earth to the center of the target. The distance from the Earth to the edge of the DM halo in the direction α is . For neutral particles, directional observations are achievable. In this case, the flux must be averaged over the solid angle of the detector, that is typically of order of ΔΩ = 2π(1 − cosθ), being θ the angular resolution of the telescope. Further details about the calculation of the astrophysical factor can be found e.g., in Appendix B of Gammaldi et al. (2018).
For charged cosmic-rays (c-cr), directional observations are not feasible. In fact, charged particles observed in a given direction might have been produced everywhere in the sky. In this case the κc-cr factor is proportional to a diffusion term:
The diffusion factor at the position of the Sun Rc-cr(r⊙, E) for charged cosmic rays (e±, p±) is the solution of a diffusion equation that depends on the particle of interest and DM distribution. It describes the diffusion of particles in the Galaxy and the production of secondary cosmic rays due to the interaction with the Interstellar Medium (ISM). The final flux at the position of the Earth also includes Solar magnetic field effect (Perko, 1987).
Each of the previous points require further investigations, yet this is beyond the scope of this review. In the following section we will apply such a primary equation to the specific case of TeV DM candidates. We will show recent results for several cosmic rays (cr = gamma rays, neutrinos and antiprotons), focusing on annihilation events (a = 2 and ).
3. Indirect Searches and Multi-TeV DM
Multi-TeV DM candidates have been proposed in literature e.g., in order to explain the cut-off at TeV energy scale observed by the HESS telescopes at the Galactic Center (CG) (Aharonian, 2009; Abdallah et al., 2016). The interpretation of these fluxes as DM signal has been widely discussed from the very early days of the publications of the observed data (Bergström et al., 2005a; Bergstrom et al., 2005b; Profumo, 2005; Aharonian, 2006). At a first moment, it was concluded that the spectral features of these gamma rays disfavoured the DM origin (Aharonian and Neronov, 2005; Aharonian, 2006). More recently, combined analyses of Fermi LAT and HESS data have allowed new interpretations (Belikov et al., 2012; Cembranos et al., 2012b, 2013b).
Before going into further details and in order to avoid any misunderstanding, it should be noted that we do not refer to Fermi-LAT signal commonly known as the GeV-excess. In fact, Fermi LAT among other experiments (e.g., MAGIC Aleksic et al., 2011), have allowed to set stringent constraints on the DM particle mass and annihilation cross-section (Bertoni et al., 2015; Di Mauro and Donato, 2015; Ahnen et al., 2016; Caputo et al., 2016; Albert et al., 2017; Lisanti et al., 2018) as well as to increase the number of claims of gamma-ray signatures from DM (Geringer-Sameth et al., 2015; Bertoni et al., 2016; Li et al., 2016; Albert et al., 2017). However, most of these studies deal with DM particle mass between few MeV and hundreds of GeV. In particular, the GC-excess has been largely interpreted both in terms of DM signatures (Alves et al., 2014; Calore et al., 2014, 2015; Cerdeno et al., 2014; Huang et al., 2014; Achterberg et al., 2015; Bertone et al., 2016; Karwin et al., 2017) and of astrophysical signal from Millisecond Pulsars (MSPs) (Brandt and Kocsis, 2015; Cholis et al., 2015; Petrovic et al., 2015; Bartels et al., 2016; Hooper and Linden, 2016, 2018; Hooper and Mohlabeng, 2016; Lee et al., 2016; Haggard et al., 2017). On the other hand, the combined analysis of Fermi-LAT data with TeV experiments open new avenues to different interpretations. If the high energy (HE) Fermi-LAT data are assumed as the background component for the HESS observations in the GC region, the fit of the VHE HESS data improves with respect to previous works (Belikov et al., 2012; Cembranos et al., 2012b, 2013b; Gammaldi, 2015). In particular, the HESS data show a spectral cut-off within a region of tens of parsecs from the GC (Aharonian, 2009) and no spectral cut-off in a region of hundreds of parsecs from the GC. The latter emission let researchers think about the existence of a pevatron accelerator (Abramowski et al., 2016), and an astrophysical origin from GeV to TeV energy scale (Gaggero et al., 2017). Some efforts have been also pursued to explain the TeV cut-off as a combined signal of TeV DM and MSPs (Lacroix et al., 2016).
In the following, we assume the astrophysical origin for the GeV-excess, and we focus on the multi-TeV DM interpretation of the HESS data. In the upper left panel of Figure 1, we show the fit to gamma-ray spectra of Fermi-LAT and HESS data with a thermal DM particle mass of 48.8 TeV that annihilates in the W+W− boson channel (Cembranos et al., 2012b, 2013b). Similar fits can be found in Belikov et al. (2012). This multi-TeV DM candidate is still a subject matter of discussion. In fact, the TeV DM hypothesis in this region needs an enhancement factor that, in the case of thermal DM particle, could be explained as increasing DM density throughout the GC with respect to the benchmark NFW profile (Cembranos et al., 2012b, 2013b). Hydrodynamic simulations together with a Black Hole (BH) induced DM-spike may explain the required enhancement of 103 in the J-factor (Gammaldi et al., 2016), and the radial dimension of the DM-spike depends on the initial DM profile, that is a cuspy or core (Gammaldi et al., 2016, 2017; Lacroix, 2018). The DM spike that corresponds to several DM halo density profiles is shown in the upper right panel in Figure 1.
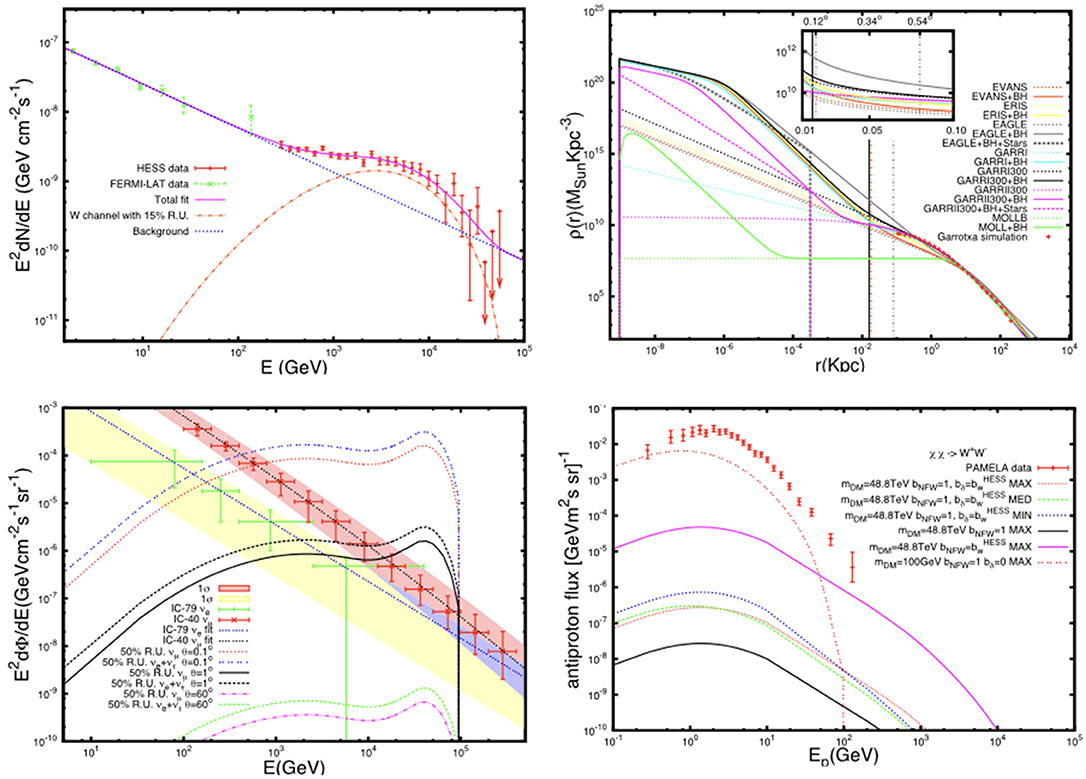
Figure 1. Upper Left: Figure from Gammaldi (2016b). The combination of the gamma-ray flux expected by DM annihilation events with a power-law background component well fits the gamma-ray spectra of Fermi-LAT and HESS data with a thermal DM particle of mass of 48.8 TeV that annihilates in the W+W− boson channel (Cembranos et al., 2012b, 2013b). Upper Right: Figure from Gammaldi et al. (2016). The enhancement of 103 required in order to fit the gamma-ray spectra can be explained by the J-factor of a BH-induced DM-spike in hydrodynamical N-body simulations with a cusp profile. Lower Left: Figure from Cembranos et al. (2014). The expected neutrino flux from 48.8 TeV DM candidate is compared with the atmospheric neutrino background detected by IceCube. Lower Right: Figure from Cembranos et al. (2015). the expected antiproton flux from 48.8 TeV DM candidate is compared with the PAMELA data. The total antiproton flux considers the diffusion of antiprotons produced by multi-TeV DM in the halo and the extra component given by the DM-spike at the GC.
In the framework of the multimessenger approach to DM searches, further investigation has been addressed in order to study the possibility to detect a neutrino signal originated by the same multi-TeV DM candidate, that may explain the observed cut-off in gamma rays. For neutrino searches, in Equation 1 ην = Pfp where Pfp are the elements of the symmetric 3 × 3 matrix which takes into account the neutrino oscillation effects from the neutrino flavor (νp) produced at the source and the neutrino flavor (νf) observed at the telescope. The astrophysical J-factor is the same as for gamma rays (for more details see Cembranos et al., 2014). In order to get a 2σ − 5σ neutrino signature from the DM candidate of ≈ 50 TeV annihilating in W+W− SM channel (with a minimum of 2-years of exposition time and 50m2 of detector effective area) the IceCube telescope should have resolution angle θ ~ 0.72° and low energy threshold ≈ 1 − 2 TeV. Currently, the IceCube resolution angle is worse than 5°, making unrealistic this kind of required observation (Aartsen et al., 2017). This is shown in the lower left panel of Figure 1.
Moreover, the same primary DM annihilation event, if constituting the origin of the observed cut-off in TeV gamma rays, may also produce leptonic or hadronic counterparts. The production of a concrete particle will induce secondary production that would affect mainly the diffuse signal through hadronic emission by inelastic proton collision with the interstellar gas, inverse Compton scattering of interstellar radiation by cosmic-ray electrons and positrons, or Bremsstrahlung. The e± and data from ATIC/PPB-BETS, PAMELA, Fermi LAT and AMS have been largely studied (Cirelli, 2012). On the one hand, low energy data are consistent with astrophysical primary sources (Serpico, 2012; Berezhko and Ksenofontov, 2014; Di Mauro et al., 2014, 2017; Giesen et al., 2015) (yet see e.g., Belotsky et al., 2014, 2017; Giesen et al., 2015 for different interpretation as DM). In this sense, antiproton data can be used to characterize diffusion models of charged particles along the Galaxy or to constrain new physics, whose antiproton flux may be identified upon the diffusion background. As discussed in the introduction, the κ-factor is given by Equation (3) for charged cosmic rays; instead ηp∝vp, where vp is the antiproton velocity (see Cembranos et al., 2015 for more details). The antiproton flux generated by ≈ 50 TeV DM candidate distributed in the halo with a possible enhancement at the GC appears to remain below the antiproton flux measured by PAMELA (lower right panel in Figure 1). Increasing the maximum energy threshold in antiproton data would allow to detect some signature on the extrapolated background.
On the other hand, the very recent AMS-02 positron data (Aguilar, 2019) are consistent with primary emission from astrophysical sources at low energy scale (Serpico, 2012), yet a finite energy cut-off at GeV is established with a significance of more than 4σ. Such a cut-off may have predominantly originated either from DM or from other astrophysics. We would like to invoke the possibility - that has not yet been explored - to study these data within the multi-TeV DM framework.
Complementary to the GC region, dwarf galaxies represent excellent targets for the indirect searches of DM. The GC represents a very appealing target, due to its closeness and the large amount of DM in the region, yet the complex nature of this area makes the identification of the source quite difficult, as we discussed so far. On the other hand, dwarf galaxies are close DM dominated structures with a low astrophysical background. Well-known dwarf spheroidal (dSph) galaxies are pressure-supported systems where the contamination from intrinsic astrophysical sources is negligible (Winter et al., 2016). In fact, they host an old stellar population of low-luminosity and do not possess gas. These objects have been studied at TeV energy scale by several gamma-ray telescopes, such as VERITAS (Archambault et al., 2017), HESS (Abramowski et al., 2014; Abdalla et al., 2018), MAGIC (Ahnen et al., 2016), and HAWC (Albert et al., 2018). The ≈ 50 TeV DM → W+W− candidate results to be compatible with all these exclusion limits. In fact, the enhancement factor required to fit the HESS spectral cut-off at the GC, has to be understood as due to the local environment. Indeed, it would not be applied to dwarf satellites unless any BH is detected (Gonzalez-Morales et al., 2014).
Recently, it has been shown that the astrophysical contamination in gamma rays is negligible also in rotationally-supported dwarf irregular (dIrr) galaxies (Gammaldi et al., 2018). Because Active Galactic Nuclei are not observed in dIrr galaxies, the background is expected to be negligible at TeV scale. In the left panel of Figure 2 we show the preliminary study of the HAWC collaboration on this new class of targets for the analysis of 1-year of data taking (Cadena et al., 2018). Although the results do not reach the thermal annihilation cross-section value and bounds are not competitive with respect to those obtained at lower WIMP mass scales, they represent new results for the TeV DM candidate by means of a previously unexplored kind of DM targets, i.e., dIrr galaxies.
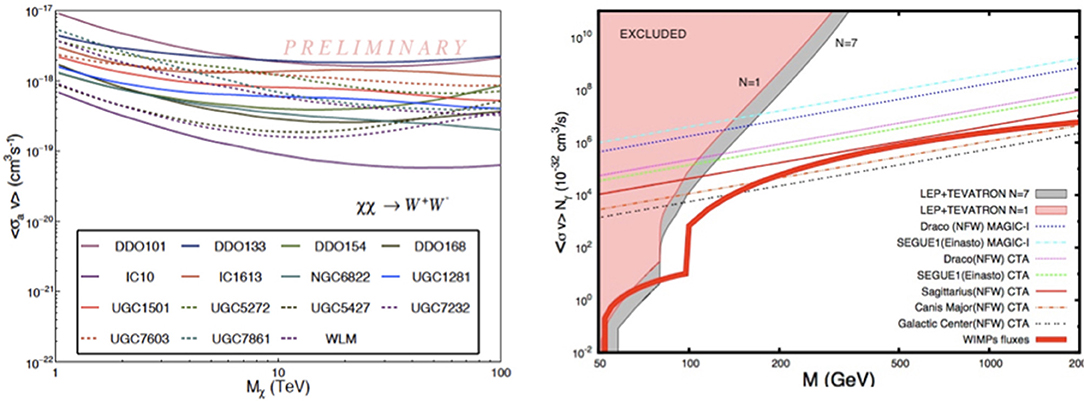
Figure 2. Left: Figure from Cadena et al. (2018). Constraints on the DM mass and annihilation cross-section by the study of several dwarf irregular galaxies at TeV energy scale, with 1-year of data of the HAWC observatory. Right: Figure from Cembranos et al. (2012a). Study of detectability of branon DM with Cherenkov telescopes.
4. The Multi-TeV DM Particle Nature
The particle nature of the prospective multi-TeV DM candidate has been investigated since the first analysis of the HESS data. Among other DM candidates (Gardner and Fuller, 2013) and beyond the SM, the largest neutralino masses appears unlikely to explain the HESS data (Profumo, 2005). Few models could naturally produce DM particle mass from a few to tens of TeV—see e.g., dark atoms (Belotsky et al., 2014, 2017) or minimal DM models (Cirelli and Strumia, 2009; Garcia-Cely et al., 2015). The Brane World Theory may naturally produce a thermal DM candidate up to masses of 100 TeV (Cembranos et al., 2003). In brief, in the framework of extra-dimensions, and in the particular case of four-dimensional effective phenomenology, massive branons are new pseudoscalar fields which can be understood as the pseudo-Goldstone bosons corresponding to the spontaneous breaking of translational invariance in the bulk space produced by the presence of the brane. They are prevented from decaying into SM particles by parity invariance on the brane. Limits on the model parameter from three-level processes in colliders are given by HERA, Tevatron, LEP-II and LHC (Cembranos et al., 2011), and prospects for ILC and CLIC can be found in Achard et al. (2004) and Creminelli and Strumia (2001). As introduced before, strong experimental limitations in direct searches and colliders affect the study of branons as multi-TeV WIMP candidates. In the right panel of Figure 2 we show the prospect of detectability of branons DM with the future Cherenkov telescope array (CTA), among the others (Cembranos et al., 2012a).
5. Conclusions
We have briefly discussed general aspects of the multimessenger approach to the indirect searches of DM focusing on the TeV energy scale. The Multi-TeV DM candidate is being considered after the advent of the last generation of gamma-ray telescopes and recent observations of the GC region. The ≈ 50 TeV DM → W+W− candidate combined with a power-law background component, fits the VHE gamma-ray spectral cut-off observed by HESS in the inner 10 parsecs at the GC combined with the HE Fermi-LAT data well. Additionally, multimessenger searches via both neutrino and antimatter fluxes have been addressed to better investigate such a heavy DM hypothesis for the GC. DSph galaxies have also been scanned at the TeV energy scale by MAGIC, HESS, VERITAS, and HAWC telescopes, resulting in compatible constraints. The next generation of both experimental (such as future CTA) and previously unexplored types of DM targets (i.e., dIrr galaxies) will increasingly improve the constraints via indirect searches. Indirect searches of DM may also be performed through the study of the synchrotron radiation, which is a radio signal emitted by the interaction of the secondary fluxes of charged particles produced in DM annihilation or decay events with the magnetic field of the target. This signal may be detected by the SKA telescope, also for the particular case of branon DM candidate in the GC (Bacon et al., 2018; Bull et al., 2018) or in dwarf galaxies (Cembranos et al. in preparation). Finally, on the theoretical side, multi-TeV branon DM candidates represent an appealing possibility, among others. Unfortunately, strong experimental limitations make it very difficult to set further constraints on the nature of the multi-TeV DM particle through direct searches and colliders. Therefore, the study of particle physics nature of multi-TeV DM candidates at underground laboratories is a challenge and represents a new frontier in physics.
Author Contributions
VG contributed to the conception and design of the study, performed the analysis, and organized the results of this mini review.
Funding
This work has been supported by JUAN DE LA CIERVA-FORMACIÓN FJCI-2016-29213, by the Spanish Agencia Estatal de Investigación through the grants FPA2015-65929-P (MINECO/FEDER, UE) and IFT Centro de Excelencia Severo Ochoa SEV-2016-0597, by INFN project QGSKY, by the Agencia Estatal de Investigación (AEI) y al Fondo Europeo de Desarrollo Regional (FEDER) FIS2016-78859-P(AEI/FEDER, UE) and partially by the H2020 CSA Twinning project No. 692194 ORBI-T-WINNINGO.
Conflict of Interest Statement
The author declares that the research was conducted in the absence of any commercial or financial relationships that could be construed as a potential conflict of interest.
Acknowledgments
The author acknowledges the support of the Spanish Red Consolider MultiDark FPA2017-90566-REDC. The section 2 Multimessenger Approach to indirect searches of DM was adapted with permission of the EPJ Web of Conferences 121, 06003 (2016), both the upper-left panel in Figure 1 and right panel in Figure 2 were adapted with permission of the Universidad Complutense de Madrid. The lower-right panel in the same Figure 1 was reused with permission of IOPscience. The license agreements between the American Physical Society (APS) and VG with licence numbers: RNP/18/DEC/009954 and RNP/18/DEC/009955, respectively allows the republication of the figures in the upper-right and lower-left panels in Figure 1 of this manuscript. VG also thanks J.A.R. Cembranos, R. Lineros, C. Muñoz, E. Roldán and M. A. Sánchez-Conde for useful discussions.
References
Aalseth, C. E., Barbeau, P. S., Cerdeo, D. G., Colaresi, J., Collar, J. I., de Lurgio, P., et al. (2008). Experimental constraints on a dark matter origin for the DAMA annual modulation effect. Phys. Rev. Lett. 101:251301. [Erratum: Phys. Rev. Lett. 102, 109903 (2009)]. doi: 10.1103/PhysRevLett.101.251301
Aartsen, M. G., Ackermann, M., Adams, J., Aguilar, J. A., Ahlers, M., Ahrens, M., et al. (2017). Search for neutrinos from dark matter self-annihilations in the center of the Milky Way with 3 years of IceCube/DeepCore. Eur. Phys. J. C77:627. doi: 10.1140/epjc/s10052-017-5213-y
Abdalla, H., Aharonian, F., Ait Benkhali, F., Angüner, E.O., Arakawa, M., Arcaro, C., et al. (2018). Searches for gamma-ray lines and 'pure WIMP' spectra from Dark Matter annihilations in dwarf galaxies with H.E.S.S. arXiv:1810.00995. doi: 10.1088/1475-7516/2018/11/037
Abdallah, H., Abramowski, A., Aharonian, F., Ait Benkhali, F., Akhperjanian, A. G., Angüner, E., et al. (2016). Search for dark matter annihilations towards the inner Galactic halo from 10 years of observations with H.E.S.S. Phys. Rev. Lett. 117:111301. doi: 10.1103/PhysRevLett.117.111301
Abramowski, A., Aharonian, F., Ait Benkhali, F., Akhperjanian, A. G., Angüner, E., Backes, M., et al. (2014). Search for dark matter annihilation signatures in H.E.S.S. observations of Dwarf Spheroidal Galaxies. Phys. Rev. D90:112012. doi: 10.1103/PhysRevD.90.112012
Abramowski, A., Aharonian, F., Ait Benkhali, F., Akhperjanian, A. G., Angüner, E., Backes, M., et al. (2016). Acceleration of petaelectronvolt protons in the Galactic Centre. Nature 531:476. doi: 10.1038/nature17147
Achard, P., Adriani, O., Aguilar-Benitez, M., Alcaraz, J., Alemanni, G., Allaby, J., et al. (2004). Search for branons at LEP. Phys. Lett. B597:145–154. doi: 10.1016/j.physletb.2004.07.014
Achterberg, A., Amoroso, S., Caron, S., Hendriks, L., Ruiz de Austri, R., and Weniger, C. (2015). A description of the Galactic Center excess in the minimal supersymmetric standard model. J. Cosmol. Astropart. Phys. 1508. doi: 10.1088/1475-7516/2015/08/006
Ade, P. A. R., Aghanim, N., Armitage-Caplan, C., Arnaud, M., Ashdown, M., Atrio-Barandela, F., et al. (2016). Planck 2015 results. XIII. Cosmological parameters. Astron. Astrophys. 594:A13. doi: 10.1051/0004-6361/201321591
Ade, P. A. R., Aghanim, N., Arnaud, M., Ashdown, M., Aumont, J., Baccigalupi, C., et al. (2014). Planck 2013 results. XVI. Cosmological parameters. Astron. Astrophys. 571:A16. doi: 10.1051/0004-6361/201525830
Aguilar, M. E. (2019). Towards understanding the origin of cosmic-ray positrons. Phys. Rev. Lett. 122:041102. doi: 10.1103/PhysRevLett.122.041102
Aharonian, E. A. (2009). Spectrum and variability of the Galactic center VHE gamma-ray source HESS J1745-290. Astron. Astrophys. 503, 817–825. doi: 10.1051/0004-6361/200811569
Aharonian, F., and Neronov, A. (2005). High-energy gamma rays from the massive black hole in the galactic center. Astrph. J. 619, 306–313. doi: 10.1086/426426
Aharonian, F. E. A. (2006). Hess observations of the galactic center region and their possible dark matter interpretation. Phys. Rev. Lett. 97:221102. doi: 10.1103/PhysRevLett.97.221102
Ahmed, Z. (2009). Search for weakly interacting massive particles with the first five-tower data from the cryogenic dark matter search at the Soudan Underground Laboratory. Phys. Rev. Lett. 102:011301. doi: 10.1103/PhysRevLett.102.011301
Ahnen, M. L., Ansoldi, S., Antonelli, L. A., Antoranz, P., Babic, A., Banerjee, B., et al. (2016). Limits to dark matter annihilation cross-section from a combined analysis of MAGIC and Fermi-LAT observations of dwarf satellite galaxies. arXiv:1601.06590 . doi: 10.1088/1475-7516/2016/02/039
Albert, A., Alfaro, R., Alvarez, C., Álvarez, J. D., Arceo, R., Arteaga-Velázquez, J. C., et al. (2018). Dark matter limits from dwarf spheroidal galaxies with The HAWC gamma-ray observatory. Astrophys. J. 853:154. doi: 10.3847/1538-4357/aaa6d8
Albert, A., Anderson, B., Bechtol, K., Drlica-Wagner, A., Meyer, M., Sanchez-Conde, M., et al. (2017). Searching for dark matter annihilation in recently discovered milky way satellites with fermi-LAT. Astrophys. J. 834:110. doi: 10.3847/1538-4357/834/2/110
Aleksic, J., Alvarez, E. A., Antonelli, L. A., Antoranz, P., Asensio, M., Backes, M., et al. (2011). Searches for dark matter annihilation signatures in the Segue 1 satellite galaxy with the MAGIC-I telescope. J. Cosmol. Astropart. Phys. 1106:035. doi: 10.1088/1475-7516/2011/06/035
Alves, A., Profumo, S., Queiroz, F. S., and Shepherd, W. (2014). Effective field theory approach to the Galactic Center gamma-ray excess. Phys. Rev. D90:115003. doi: 10.1103/PhysRevD.90.115003
Ambriola, M. L., Barbiellini, G., Bartalucci, S., Basini, G., Bellotti, R., Bergstroem, D., et al. (1999). Caprice98: a balloon borne magnetic spectrometer to study cosmic ray antimatter and composition at different atmospheric depths. Nucl. Phys. 78, 32 – 37. doi: 10.1016/S0920-5632(99)00519-8
Angle, J., Aprile, E., Arneodo, F., Baudis, L., Bernstein, A., Bolozdynya, A., et al. (2008). Limits on spin-dependent WIMP-nucleon cross-sections from the XENON10 experiment. Phys. Rev. Lett. 101:091301. doi: 10.1103/PhysRevLett.101.091301
Archambault, S., Archer, A., Benbow, W., Bird, R., Bourbeau, E., Brantseg, T., et al. (2017). Dark matter constraints from a joint analysis of dwarf spheroidal galaxy observations with VERITAS. Phys. Rev. D95:082001. doi: 10.1103/PhysRevD.95.082001
Bacon, D. J., Battye, R. A., Bull, P., Camera, S., Ferreira, P. G., Harrison, I., et al. (2018). Cosmology with phase 1 of the square kilometre array: red book 2018: technical specifications and performance forecasts. arXiv:1811.02743
Bartels, R., Krishnamurthy, S., and Weniger, C. (2016). Strong support for the millisecond pulsar origin of the Galactic center GeV excess. Phys. Rev. Lett. 116:051102. doi: 10.1103/PhysRevLett.116.051102
Baudis, L. (2014). WIMP dark matter direct-detection searches in noble gases. Phys. Dark Univ. 4, 50–59. doi: 10.1016/j.dark.2014.07.001
Baum, S., Freese, K., and Kelso, C. (2019). Dark matter implications of DAMA/LIBRA-phase2 results. Phys. Lett. B789:262–269. doi: 10.1016/j.physletb.2018.12.036
Belikov, A. V., Zaharijas, G., and Silk, J. (2012). Study of the gamma-ray spectrum from the Galactic Center in view of multi-TeV dark matter candidates. Phys. Rev. 86:083516. doi: 10.1103/PhysRevD.86.083516
Belotsky, K., Budaev, R., Kirillov, A., and Laletin, M. (2017). Fermi-LAT kills dark matter interpretations of AMS-02 data. Or not? J. Cosmol. Astropart. Phys. 1701:021. doi: 10.1088/1475-7516/2017/01/021
Belotsky, K., Khlopov, M., Kouvaris, C., and Laletin, M. (2014). Decaying dark atom constituents and cosmic positron excess. Adv. High Energy Phys. 2014:214258. doi: 10.1155/2014/214258
Berezhko, E. G., and Ksenofontov, L. T. (2014). Antiprotons produced in supernova remnants. Astrophys. J. 791:L22. doi: 10.1088/2041-8205/791/2/L22
Bergstrom, L. (2000). Nonbaryonic dark matter: observational evidence and detection methods. Rept. Prog. Phys. 63:793. doi: 10.1088/0034-4885/63/5/2r3
Bergström, L., Bringmann, T., Eriksson, M., and Gustafsson, M. (2005a). Gamma rays from heavy neutralino dark matter. Phys. Rev. Lett. 95:241301. doi: 10.1103/PhysRevLett.95.241301
Bergstrom, L., Bringmann, T., Eriksson, M., and Gustafsson, M. (2005b). Gamma rays from Kaluza-Klein dark matter. Phys. Rev. Lett. 94:131301. doi: 10.1103/PhysRevLett.94.131301
Bernabei, R. (2008). First results from DAMA/LIBRA and the combined results with DAMA/NaI. Eur. Phys. J. C56, 333–355. doi: 10.1140/epjc/s10052-008-0662-y
Bernabei, R., Belli, P., Bussolotti, A., Cappella, F., Caracciolo, V., Cerulli, R., et al. (2018). First model independent results from DAMA/LIBRA phase2. Universe 4:116.
Bernabei, R., Belli, P., Cappella, F., Cerulli, R., Dai, C. J., d'Angelo, A., et al. (2013). Final model independent result of DAMA/LIBRA-phase1. Eur. Phys. J. C73:2648. doi: 10.1140/epjc/s10052-013-2648-7
Bertone, G., Calore, F., Caron, S., Ruiz, R., Kim, J. S., Trotta, R., et al. (2016). Global analysis of the pMSSM in light of the Fermi GeV excess: prospects for the LHC Run-II and astroparticle experiments. J. Cosmol. Astropart. Phys. 2016, 1604. doi: 10.1088/1475-7516/2016/04/037
Bertone, G., Hooper, D., and Silk, J. (2005). Particle dark matter: evidence, candidates and constraints. Phys. Rept. 405, 279–390. doi: 10.1016/j.physrep.2004.08.031
Bertoni, B., Hooper, D., and Linden, T. (2015). Examining the Fermi-LAT third source catalog in search of dark matter subhalos. J. Cosmol. Astropart. Phys. 1512:035. doi: 10.1088/1475-7516/2015/12/035
Bertoni, B., Hooper, D., and Linden, T. (2016). Is the gamma-ray source 3FGL J2212.5+0703 a dark matter subhalo? J. Cosmol. Astropart. Phys. 1605:049. doi: 10.1088/1475-7516/2016/05/049
Bouwhuis, M., and for the ANTARES collaboration (2009). Concepts and performance of the Antares data acquisition system. arXiv:0908.0811.
Bradac, M., Clowe, D., Gonzalez, A. H., Marshall, P., Forman, W., Jones, C., et al. (2006). Strong and weak lensing united. 3. Measuring the mass distribution of the merging galaxy cluster 1E0657-56. Astrophys. J. 652, 937–947. doi: 10.1086/508601
Brandt, T. D., and Kocsis, B. (2015). Disrupted globular clusters can explain the galactic center gamma ray excess. Astrophys. J. 812:15. doi: 10.1088/0004-637X/812/1/15
Broeils, A. H. (1992). The mass distribution of the dwarf spiral NGC 1560. Astron. Astrophys. 256, 19–32.
Bull, P., Camera, S., Kelley, K., Padmanabhan, H., Pritchard, J., Raccanelli, A., et al. (2018). Fundamental physics with the square kilometer array. arXiv:1810.02680
Cadena, S. H., Alfaro, R., Sandoval, A., Belmont, E., Leon, H., Gammaldi, V., et al. (2018). “Searching for TeV DM evidence from dwarf irregular galaxies with the HAWC observatory,” in Presented at the 35th International Cosmic Ray Conference (ICRC2017) (Busan: Bexco).
Calore, F., Cholis, I., McCabe, C., and Weniger, C. (2015). A tale of tails: dark matter interpretations of the fermi GeV excess in light of background model systematics. Phys. Rev. D91:063003. doi: 10.1103/PhysRevD.91.063003
Calore, F., De Romeri, V., Di Mauro, M., Donato, F., Herpich, J., Maccio, A. V., et al. (2014). Gamma ray anisotropies from dark matter in the Milky Way: the role of the radial distribution. Mon. Not. Roy. Astron. Soc. 442, 1151–1156. doi: 10.1093/mnras/stu912
Caputo, R., Buckley, M. R., Martin, P., Charles, E., Brooks, A. M., Drlica-Wagner, A., et al. (2016). Search for gamma-ray emission from dark matter annihilation in the small magellanic cloud with the Fermi Large Area Telescope. Phys. Rev. D93:062004. doi: 10.1103/PhysRevD.93.062004
Cembranos, J. A. R., de la Cruz-Dombriz, A., Gammaldi, V., Lineros, R. A., and Maroto, A. L. (2013a). Reliability of Monte Carlo event generators for gamma ray dark matter searches. J. High Energ. Phys. 2013:77. doi: 10.1007/JHEP09(2013)077
Cembranos, J. A. R., de la Cruz-Dombriz, A., Gammaldi, V., and Maroto, A. L. (2012a). Detection of branon dark matter with gamma ray telescopes. Phys. Rev. D85:043505. doi: 10.1103/PhysRevD.85.043505
Cembranos, J. A. R., Diaz-Cruz, J. L., and Prado, L. (2011). Impact of DM direct searches and the LHC analyses on branon phenomenology. Phys. Rev. D84:083522. doi: 10.1103/PhysRevD.84.083522
Cembranos, J. A. R., Dobado, A., and Maroto, A. L. (2003). Brane-world dark matter. Phys. Rev. Lett. 90:241301. doi: 10.1103/PhysRevLett.90.241301
Cembranos, J. A. R., Gammaldi, V., and Maroto, A. L. (2012b). Possible dark matter origin of the gamma ray emission from the galactic center observed by HESS. Phys. Rev. D86:103506. doi: 10.1103/PhysRevD.86.103506
Cembranos, J. A. R., Gammaldi, V., and Maroto, A. L. (2013b). Spectral study of the HESS J1745-290 gamma-ray source as dark matter signal. J. Cosmol. Astropart. Phys. 1304:051. doi: 10.1088/1475-7516/2013/04/051
Cembranos, J. A. R., Gammaldi, V., and Maroto, A. L. (2014). Neutrino fluxes from Dark Matter in the HESS J1745-290 source at the Galactic Center. Phys. Rev. D90:043004. doi: 10.1103/PhysRevD.90.043004
Cembranos, J. A. R., Gammaldi, V., and Maroto, A. L. (2015). Antiproton signatures from astrophysical and dark matter sources at the galactic center. J. Cosmol. Astropart. Phys. Madrid. 1503:041. doi: 10.1088/1475-7516/2015/03/041
Cerdeno, D. G., Peiro, M., and Robles, S. (2014). Low-mass right-handed sneutrino dark matter: SuperCDMS and LUX constraints and the Galactic Centre gamma-ray excess. J. Cosmol. Astropart. Phys. 1408:005. doi: 10.1088/1475-7516/2014/08/005
Chianese, M. (2017). “Dark matter scenarios at IceCube,” in Prepared for the Proceedings of the Conference NOW2016:090 (Lecce).
Cholis, I., Hooper, D., and Linden, T. (2015). Challenges in explaining the galactic center gamma-ray excess with millisecond pulsars. J. Cosmol. Astropart. Phys. 1506:043. doi: 10.1088/1475-7516/2015/06/043
Ciafaloni, P., Comelli, D., Riotto, A., Sala, F., Strumia, A., and Urbano, A. (2011). Weak corrections are relevant for dark matter indirect detection. J. Cosmol. Astropart. Phys. 1103:019. doi: 10.1088/1475-7516/2011/03/019
Cirelli, M. (2012). Indirect searches for dark matter: a status review. Pramana 79, 1021–1043. doi: 10.1007/s12043-012-0419-x
Cirelli, M., Corcella, G., Hektor, A., Hutsi, G., Kadastik, M., Panci, P., et al. (2011). PPPC 4 DM ID: a poor particle physicist cookbook for dark matter indirect detection. J. Cosmol. Astropart. Phys. 1103:051. [Erratum: J. Cosmol. Astropart. Phys. 1210, E01 (2012)]. doi: 10.1088/1475-7516/2011/03/051
Cirelli, M., and Strumia, A. (2009). Minimal dark matter: model and results. New J. Phys. 11:105005. doi: 10.1088/1367-2630/11/10/105005
Collaboration, F. (2012). The fermi large area telescope on orbit: event classification, instrument response functions, and calibration. Astrophys. J. 203:4. doi: 10.1088/0067-0049/203/1/4
Creminelli, P., and Strumia, A. (2001). Collider signals of brane fluctuations. Nuclear Physics B 596, 125–135.
Cuoco, A., Kramer, M., and Korsmeier, M. (2017). Novel dark matter constraints from antiprotons in light of ams-02. Phys. Rev. Lett. 118:191102. doi: 10.1103/PhysRevLett.118.191102
Desai, S., Ashie, Y., Fukuda, S., Fukuda, Y., Ishihara, K., and Itow, Y. (2004). Search for dark matter WIMPs using upward through-going muons in Super-Kamiokande. Phys. Rev. D70:083523. doi: 10.1103/PhysRevD.70.083523
Di Mauro, M., and Donato, F. (2015). Composition of the Fermi-LAT isotropic gamma-ray background intensity: emission from extragalactic point sources and dark matter annihilations. Phys. Rev. D91:123001. doi: 10.1103/PhysRevD.91.123001
Di Mauro, M., Donato, F., Fornengo, N., Lineros, R., and Vittino, A. (2014). Interpretation of AMS-02 electrons and positrons data. J. Cosmol. Astropart. Phys. 1404:006. doi: 10.1088/1475-7516/2014/04/006
Di Mauro, M., Manconi, S., Vittino, A., Donato, F., Fornengo, N., Baldini, L., et al. (2017). Theoretical interpretation of Pass 8 Fermi-LAT e++e− data. Astrophys. J. 845:107. doi: 10.3847/1538-4357/aa8225
Drukier, A., and Stodolsky, L. (1984). Principles and applications of a neutral-current detector for neutrino physics and astronomy. Phys. Rev. D 30, 2295–2309.
Gaggero, D., Grasso, D., Marinelli, A., Taoso, M., and Urbano, A. (2017). Diffuse cosmic rays shining in the Galactic center: a novel interpretation of H.E.S.S. and Fermi-LAT gamma-ray data. Phys. Rev. Lett. 119:031101. doi: 10.1103/PhysRevLett.119.031101
Galper, A., and Spillantini, P. (2018). Ten years of cr physics with pamela. Adv. Space Res. 62, 2892–2901. doi: 10.1016/j.asr.2017.08.026
Gammaldi, V. (2016a). Highlights on gamma rays, neutrinos and antiprotons from TeV Dark Matter. EPJ Web Conf. 121:06003. doi: 10.1051/epjconf/201612106003
Gammaldi, V. (2016b). Indirect Searchers of TeV Dark Matter. Ph.D. thesis, Universidad Complutense Madrid (UCM).
Gammaldi, V., Avila-Reese, V., Valenzuela, O., and Gonzales-Morales, A. X. (2016). Analysis of the very inner Milky Way dark matter distribution and gamma-ray signals. Phys. Rev. D94:121301. doi: 10.1103/PhysRevD.94.121301
Gammaldi, V., Avila-Reese, V., Valenzuela, O., Gonzalez-Morales, A. X., Salucci, P., and Nesti, F. (2017). On the possible enhancement of the dark matter density distribution at the galactic center. Rev. Mex. Astron. Astrof. Ser. Conf. 49:105.
Gammaldi, V., Karukes, E., and Salucci, P. (2018). Theoretical predictions for dark matter detection in dwarf irregular galaxies with gamma rays. Phys. Rev. D 98:083008. doi: 10.1103/PhysRevD.98.083008
Garcia-Cely, C., Ibarra, A., Lamperstorfer, A. S., and Tytgat, M. H. G. (2015). Gamma-rays from heavy minimal dark matter. J. Cosmol. Astropart. Phys. 1510:058. doi: 10.1088/1475-7516/2015/10/058
Gardner, S., and Fuller, G. (2013). Dark matter studies entrain nuclear physics. Prog. Part. Nucl. Phys. 71, 167–184. doi: 10.1016/j.ppnp.2013.03.001
Gaskins, J. M. (2016). A review of indirect searches for particle dark matter. Contemp. Phys. 57, 496–525. doi: 10.1080/00107514.2016.1175160
Geringer-Sameth, A., Walker, M. G., Koushiappas, S. M., Koposov, S. E., Belokurov, V., Torrealba, G., et al. (2015). Indication of gamma-ray emission from the newly discovered dwarf galaxy reticulum II. Phys. Rev. Lett. 115:081101. doi: 10.1103/PhysRevLett.115.081101
Giesen, G., Boudaud, M., Genolini, Y., Poulin, V., Cirelli, M., Salati, P., et al. (2015). AMS-02 antiprotons, at last! Secondary astrophysical component and immediate implications for dark matter. J. Cosmol. Astropart. Phys. 2015, 1509. doi: 10.1088/1475-7516/2015/9/023
Gonzalez-Morales, A. X., Profumo, S., and Queiroz, F. S. (2014). Effect of black holes in local dwarf spheroidal galaxies on gamma-ray constraints on dark matter annihilation. Phys. Rev. D90:103508. doi: 10.1103/PhysRevD.90.103508
Goodman, M. W., and Witten, E. (1985). Detectability of certain dark-matter candidates. Phys. Rev. D 31, 3059–3063. doi: 10.1103/PhysRevD.31.3059
Gottschall, D., Forster, A., Bonardi, A., Santangelo, A., Puhlhofer, G., and for the HESS collaboration (2015). The mirror alignment and control system for CT5 of the H.E.S.S.experiment. ArXiv e-prints.
Haggard, D., Heinke, C., Hooper, D., and Linden, T. (2017). Low mass X-ray binaries in the inner galaxy: implications for millisecond pulsars and the GeV excess. J. Cosmol. Astropart. Phys. 1705:056. doi: 10.1088/1475-7516/2017/05/056
Hong, T. M. (2017). “Dark matter searches at the LHC,” in 5th Large Hadron Collider Physics Conference (LHCP 2017) (Shanghai).
Hooper, D., and Linden, T. (2016). The gamma-ray pulsar population of globular clusters: implications for the GeV excess. J. Cosmol. Astropart. Phys. 1608:018. doi: 10.1088/1475-7516/2016/08/018
Hooper, D., and Linden, T. (2018). Millisecond pulsars, TeV halos, and implications for the galactic center gamma-ray excess. Phys. Rev. D98:043005. doi: 10.1103/PhysRevD.98.043005
Hooper, D., and Mohlabeng, G. (2016). The gamma-ray luminosity function of millisecond pulsars and implications for the GeV excess. J. Cosmol. Astropart. Phys. 1603:049. doi: 10.1088/1475-7516/2016/03/049
Huang, J., Liu, T., Wang, L.-T., and Yu, F. (2014). Supersymmetric subelectroweak scale dark matter, the galactic center gamma-ray excess, and exotic decays of the 125 GeV Higgs boson. Phys. Rev. D90:115006. doi: 10.1103/PhysRevD.90.115006
Karwin, C., Murgia, S., Tait, T. M. P., Porter, T. A., and Tanedo, P. (2017). Dark matter interpretation of the fermi-LAT observation toward the galactic center. Phys. Rev. D95:103005. doi: 10.1103/PhysRevD.95.103005
Lacroix, T. (2018). Dynamical constraints on a dark matter spike at the galactic centre from stellar orbits. Astron. Astrophys. 619:A46. doi: 10.1051/0004-6361/201832652
Lacroix, T., Silk, J., Moulin, E., and Boehm, C. (2016). Connecting the new H.E.S.S. diffuse emission at the galactic center with the fermi GeV excess: a combination of millisecond pulsars and heavy dark matter? Phys. Rev. D94:123008. doi: 10.1103/PhysRevD.94.123008
Lage, C., and Farrar, G. (2014). Constrained simulation of the bullet cluster. Astrophys. J. 787:144. doi: 10.1088/0004-637X/787/2/144
Lang, R. F., and Seidel, W. (2009). Search for dark matter with CRESST. New J. Phys. 11:105017. doi: 10.1088/1367-2630/11/10/105017
Lee, S. K., Lisanti, M., Safdi, B. R., Slatyer, T. R., and Xue, W. (2016). Evidence for unresolved gamma-ray point sources in the inner galaxy. Phys. Rev. Lett. 116:051103. doi: 10.1103/PhysRevLett.116.051103
Li, S., Liang, Y.-F., Duan, K.-K., Shen, Z.-Q., Huang, X., Li, X., et al. (2016). Search for gamma-ray emission from eight dwarf spheroidal galaxy candidates discovered in year two of dark energy survey with fermi-lat data. Phys. Rev. D 93:043518. doi: 10.1103/PhysRevD.93.043518
Lin, S. T., Li, H. B., Li, X., Lin, S. K., Wong, H. T., Deniz, M., et al. (2009). New limits on spin-independent and spin-dependent couplings of low-mass WIMP dark matter with a germanium detector at a threshold of 220 eV. Phys. Rev. D79:061101. doi: 10.1103/PhysRevD.79.061101
Lisanti, M., Mishra-Sharma, S., Rodd, N. L., Safdi, B. R., and Wechsler, R. H. (2018). Mapping extragalactic dark matter annihilation with galaxy surveys: a systematic study of stacked group searches. Phys. Rev. D97:063005. doi: 10.1103/PhysRevD.97.063005
Livio, M., and Silk, J. (2014). Broaden the search for dark matter. Nature 507, 29. doi: 10.1038/507029a
Maier, G., Arrabito, L., Bernl, K., Bregeon, J., Cumani, P., Hassan, T., et al. (2017). “Performance of the cherenkov telescope array,” in International Cosmic Ray Conference (Busan), 35:846.
Marrodan Undagoitia, T., and Rauch, L. (2016). Dark matter direct detection experiments. J. Phys. G43:013001. doi: 10.1088/0954-3899/43/1/013001
Mitchell, J. E. A. (2010). “The balloon-borne experiment with a superconducting spectrometer (BESS) program,” in 38th COSPAR Scientific Assembly, Vol. 38 of COSPAR Meeting (Bremen), 3.
Munoz, C. (2004). Dark matter detection in the light of recent experimental results. Int. J. Mod. Phys. A19, 3093–3170. doi: 10.1142/S0217751X04018154
Naab, T., and Ostriker, J. P. (2017). Theoretical challenges in galaxy formation. Ann. Rev. Astronomy Astrophys. 55, 59–109. doi: 10.1146/annurev-astro-081913-040019
Park, N., and VERITAS Collaboration (2015). “Performance of the VERITAS experiment,” in 34th International Cosmic Ray Conference (ICRC2015) (The Hague), 771.
Penning, B. (2018). The pursuit of dark matter at colliders: an overview. J. Phys. G45:063001. doi: 10.1088/1361-6471/aabea7
Persic, M., Salucci, P., and Stel, F. (1996). The Universal rotation curve of spiral galaxies: 1. The Dark matter connection. Mon. Not. Roy. Astron. Soc. 281:27. doi: 10.1093/mnras/281.1.27
Petrovic, J., Serpico, P. D., and Zaharijas, G. (2015). Millisecond pulsars and the galactic center gamma-ray excess: the importance of luminosity function and secondary emission. J. Cosmol. Astropart. Phys. 1502:023. doi: 10.1088/1475-7516/2015/02/023
Profumo, S. (2005). TeV gamma-rays and the largest masses and annihilation cross sections of neutralino dark matter. Phys. Rev. D72:103521. doi: 10.1103/PhysRevD.72.103521
Rubin, V.C., Ford, W.K. Jr, and Thonnard, N. (1980). Rotational properties of 21 SC galaxies with a large range of luminosities and radii, from NGC 4605 /R = 4kpc/ to UGC 2885 /R = 122 kpc/. Astrophys. J. 238, 471–487. doi: 10.1086/158003
Savage, C., Gelmini, G., Gondolo, P., and Freese, K. (2009). Compatibility of DAMA/LIBRA dark matter detection with other searches. J. Cosmol. Astropart. Phys. 904:10. doi: 10.1088/1475-7516/2009/04/010
Serpico, P. D. (2012). Astrophysical models for the origin of the positron 'excess'. Astropart. Phys. 39–40, 2–11. doi: 10.1016/j.astropartphys.2011.08.007
Sitarek, J., Carmona, E., Colin, P., Mazin, D., and Tescaro, D. (2015). “Performance of the MAGIC telescopes after the major upgrade,” in 34th International Cosmic Ray Conference (ICRC2015) (The Hague), 981.
Winter, M., Zaharijas, G., Bechtol, K., and Vandenbroucke, J. (2016). Estimating the GeV emission of millisecond pulsars in dwarf spheroidal galaxies. Astrophys. J. 832:L6. doi: 10.3847/2041-8205/832/1/L6
Keywords: dark matter, cosmic rays, TeV energy scale, indirect searches, simulations, density distribution
Citation: Gammaldi V (2019) Multimessenger Multi-TeV Dark Matter. Front. Astron. Space Sci. 6:19. doi: 10.3389/fspas.2019.00019
Received: 29 November 2018; Accepted: 11 March 2019;
Published: 05 April 2019.
Edited by:
Marina Cobal, University of Udine, ItalyReviewed by:
Maxim Yurievich Khlopov, UMR7164 Astroparticule et Cosmologie (APC), FranceVyacheslav Ivanovich Dokuchaev, Institute for Nuclear Research (RAS), Russia
Copyright © 2019 Gammaldi. This is an open-access article distributed under the terms of the Creative Commons Attribution License (CC BY). The use, distribution or reproduction in other forums is permitted, provided the original author(s) and the copyright owner(s) are credited and that the original publication in this journal is cited, in accordance with accepted academic practice. No use, distribution or reproduction is permitted which does not comply with these terms.
*Correspondence: Viviana Gammaldi, dml2aWFuYS5nYW1tYWxkaUB1YW0uZXM=