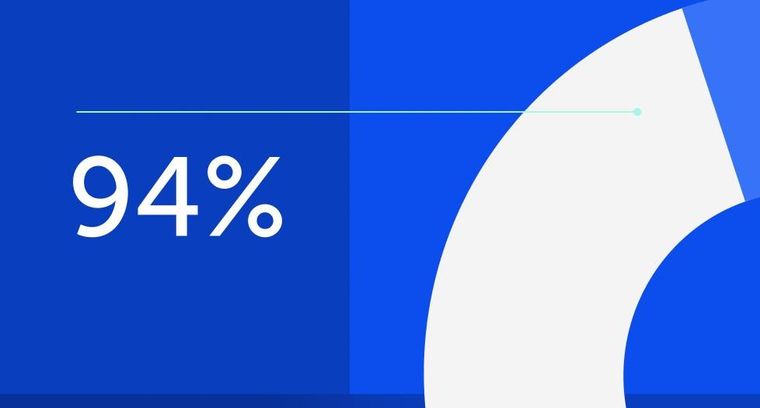
94% of researchers rate our articles as excellent or good
Learn more about the work of our research integrity team to safeguard the quality of each article we publish.
Find out more
REVIEW article
Front. Psychiatry, 27 April 2020
Sec. Molecular Psychiatry
Volume 11 - 2020 | https://doi.org/10.3389/fpsyt.2020.00315
This article is part of the Research TopicThe Search for Biomarkers in PsychiatryView all 17 articles
The high heterogeneity of psychiatric disorders leads to a lack of diagnostic precision. Therefore, the search of biomarkers is a fundamental aspect in psychiatry to reach a more personalized medicine. The endocannabinoid system (ECS) has gained increasing interest due to its involvement in many different functional processes in the brain, including the regulation of emotions, motivation, and cognition. This article reviews the role of the main components of the ECS as biomarkers in certain psychiatric disorders. Studies carried out in rodents evaluating the effects of pharmacological and genetic manipulation of cannabinoid receptors or endocannabinoids (eCBs) degrading enzymes were included. Likewise, the ECS-related alterations occurring at the molecular level in animal models reproducing some behavioral and/or neuropathological aspects of psychiatric disorders were reviewed. Furthermore, clinical studies evaluating gene or protein alterations in post-mortem brain tissue or in vivo blood, plasma, and cerebrospinal fluid (CSF) samples were analyzed. Also, the results from neuroimaging studies using positron emission tomography (PET) or functional magnetic resonance (fMRI) were included. This review shows the close involvement of cannabinoid receptor 1 (CB1r) in stress regulation and the development of mood disorders [anxiety, depression, bipolar disorder (BD)], in post-traumatic stress disorder (PTSD), as well as in the etiopathogenesis of schizophrenia, attention deficit hyperactivity disorder (ADHD), or eating disorders (i.e. anorexia and bulimia nervosa). On the other hand, recent results reveal the potential therapeutic action of the endocannabinoid tone manipulation by inhibition of eCBs degrading enzymes, as well as by the modulation of cannabinoid receptor 2 (CB2r) activity on anxiolytic, antidepressive, or antipsychotic associated effects. Further clinical research studies are needed; however, current evidence suggests that the components of the ECS may become promising biomarkers in psychiatry to improve, at least in part, the diagnosis and pharmacological treatment of psychiatric disorders.
Psychiatric disorders are one of the main causes of disability in the general population (1). According to a recent estimation, psychiatric disorders account for 32.4% of years lived with disability (YLDs) and 13% of disability adjusted life-years (DALYs), leading the global burden of disease (2). Despite this, we still have a great lack of knowledge about its neurobiological basis, and clinically applicable biomarkers have been elusive. During the last decades, an increasing effort has been made in the search of biomarkers in psychiatry to help in the diagnosis and prediction of disease progression or treatment response. However, a clinical biomarker should be validated, sensitive, specific, feasible, and easily reproducible, characteristics that make difficult the implementation in this field (3–5).
The endocannabinoid system (ECS) components (receptors, ligands, synthesizing and degrading enzymes) have gained a special interest because of their critical neuromodulatory involvement in a plethora of functional mechanisms in the central nervous system (CNS), including emotional regulation, motivational behavior, and cognitive function (6, 7). The wide distribution of ECS in the brain, together with the effects derived from its pharmacological modulation on mood or cognition with exogenous cannabinoid compounds, mainly those contained or derived from the Cannabis sativa plant, suggests that the identification of the functional role of ECS elements in certain psychiatric disorders could be a breakthrough to improve diagnosis and treatment (8–11).
Therefore, this review summarizes the findings regarding the potential involvement of ECS components as biomarkers, mainly in terms of the discovery of new therapeutic approaches, but also from the point of view of its diagnostic, prognostic and predictive application. For that purpose, studies on animal models and patients have been collected focusing on the most prevalent psychiatric conditions, including anxiety disorders (3.8%) (12), depressive disorders (3.4%) (12), schizophrenia (0.3%) (12), bipolar disorder (0.6%) (12), post-traumatic stress disorder (7.8%) (13), attention-deficit hyperactivity disorder (2.2%) (14), and eating disorders (0.2%) (12).
ECS regulates a number of physiological functions and mediates the crosstalk between different neurotransmitter systems, therefore representing a key player in the control of behavioral responses (15, 16). ECS is a ubiquitous lipid signaling system distributed throughout the organism that participates in multiple intracellular signaling pathways (17, 18). Cannabinoid receptors, endogenous ligands or endocannabinoids (eCBs), and their synthesizing and degrading enzymes are the main components of the ECS (Figure 1) present in the central and peripheral nervous system (15, 19) and in many other peripheral tissues regulating distinct functions (20).
Figure 1 Schematic representation of the main ECS components, including the metabolizing routes of the eCBs. CB1/CB2, cannabinoid receptors 1 and 2; 2-AG, 2-arachidonoylglycerol; FAAH, fatty acid amide hydrolase; MAGL, monoacylglycerol lipase; DAGL, EMT: endocannabinoid membrane transporter; NAT, N-acyl transferase; NArPE, N-arachidonoyl phosphatidylethanolamine; NAPE-PLD, N-acylphosphatidylethanolamine specific phospholipase D; DAGL, diacylglycerol lipase. Image created with BioRender.
The CB1 receptor (CB1r) is the most abundant G protein-coupled receptor in the brain (21). Physiological actions of endocannabinoids in the CNS are mediated by the activation of CB1r (22). Their expression in the CNS is widespread and heterogeneous and has crucial roles regulating brain function and disease processes (23–25). CB1r is abundant in the basal ganglia, cerebellum, in corticolimbic regions including the prefrontal cortex (PFC), nucleus accumbens (Nac), and hippocampus (Hipp), and in brain areas related to stress responses, such as the central amygdala (Amy) and the paraventricular nucleus (PVN) of the hypothalamus (Hyp) (21, 26, 27). Furthermore, CB1r is also located in terminals of peripheral neurons and glial cells, as well as in the reproductive system (i.e. uterus, ovary, testis, prostate), some glandular systems (adrenal gland), adipose tissue, heart, liver, lung, bone marrow, thymus, and the microcirculation (20, 26, 28–33).
CB2 cannabinoid receptor (CB2r) was initially considered as a peripheral cannabinoid receptor due to its high expression in the rat spleen (34) and leukocyte subpopulation in humans (32), participating in the regulation of the immune system (35). The first findings identified the presence of CB2r in the CNS only under pathological conditions such as in senile plaques in Alzheimer's disease (36), activated microglial cells/macrophages in multiple sclerosis, spinal cord in amyotrophic lateral sclerosis (37) and in the vicinity of tumors (38). However, Van Sickle and colleagues revealed that CB2r is expressed in neurons of the brainstem of mice, rats, and ferrets under normal conditions (39). This finding was key to increase the interest of CB2r in the regulation of brain function. Different studies identified CB2r in several brain regions including the frontal cortex, striatum, basal ganglia, Amy, Hipp, and the ventral tegmental area (VTA) (40–44). Interestingly, in some of these brain regions, CB2r was detected not only in the microglia (45) but also in the neurons (44, 46, 47).
The eCBs are lipid messengers acting as paracrine, autocrine, and probably endocrine mode, because their lipid nature allows them to diffuse and cross membranes (15, 17, 18, 48, 49). eCBs are agonists of CB1r and CB2r that are not accumulated in secretory vesicles but rather synthesized under tonic or phasic (on demand) modes, and released to the extracellular space following physiological and pathological stimuli (50). The two main eCBs are derivatives of polyunsatured fatty acids, N-arachidonoylethanolamine (anandamide, AEA) (51), and 2-arachidonoylglycerol (2-AG), being the most abundant eCBs in the brain (52). Firstly, AEA synthesis is produced by the N-acylphosphatidylethanolamine specific phospholipase D (NAPE-PLD) that hydrolyzes N-arachidonoyl phosphatidylethanolamine localized in cell membranes (49, 53). The AEA half-life is very short because of its quick uptake by a high affinity AEA membrane transporter distributed in the neurons and glia (54). AEA is inactivated by fatty acid amide hydrolase (FAAH) present in many organs and in the brain at postsynaptic location (55, 56). FAAH is a serine-hydrolase enzyme bound to intracellular membranes that metabolizes AEA into arachidonic acid and ethanolamine (57). Secondly, 2-AG participates in the CB1r-dependent retrograde signaling and is an intermediate metabolite for lipid synthesis providing arachidonic acid for prostaglandin synthesis (57). Neuronal membrane depolarization or the activation of Gq protein-coupled receptors (GPCRs) triggers the synthesis of 2-AG (49). The diacylglycerol precursors come from the hydrolysis of membrane phosphatidylinositol by phospholipase C, β or δ. The degradation of these precursors by diacylglycerol lipases (DAGL-α and DAGL-β) drives 2-AG synthesis (58, 59). The DAGLα isoform synthesizes the greatest amount of 2-AG, whereas DAGLβ synthesizes 2-AG under certain circumstances (54). Monoacylglycerol lipase (MAGL) is a serine-hydrolase enzyme mainly found in presynaptic terminals that catalyzes 2-AG into arachidonic acid and glycerol (55, 60). Also, the α/β-hydrolase domain 6 (ABHD6) and domain 12 (ABHD12) degrade 2-AG (49, 57).
The ECS is one of the most widely distributed neurotransmitter systems in the human brain, with a critical neuromodulatory role that motivates the interaction with other neurotransmitter and neurohormonal systems (61). Accumulating evidence points out the pivotal role of the ECS in the regulation of cognitive and behavioral functioning, suggesting its therapeutic potential in psychiatry (9, 11, 62). Furthermore, it is worth to mention that psychiatric disorders are accompanied by disturbances in the ECS components, as detailed below. Taken together, these facts suggest the potential usefulness of cannabinoid receptors, endocannabinoid ligands and degrading or synthesizing enzymes as biomarkers to move towards improved diagnostic criteria and therapeutic approaches in psychiatry.
The literature review consisted of an exhaustive search for scientific information in the Medline database (PubMed), which was always focused on the following ECS components as potential biomarkers in psychiatry: CB1r, CB2r, AEA, 2-AG, FAAH and MAGL. A total of seven search boxes were employed according to the total of psychiatric conditions included in the review: anxiety, depression, schizophrenia, bipolar disorder, post-traumatic stress disorder, attention-deficit and hyperactivity disorder, and eating disorders. These terms were combined with the term ‘cannabinoid’ by the Boolean operator ‘AND’. All the results for each search were critically analyzed by the authors to decide the inclusion or exclusion of each reference according to the adequacy of its content with the subject matter of the study. Finally, no PubMed filters were applied to maximize the selection of all the available and appropriate information.
According to the Diagnostic and Statistical Manual of Mental Disorders (DSM-5), anxiety disorders share features of excessive fear and anxiety and related behavioral disturbances. Fear is the emotional response to real or perceived imminent threat, whereas anxiety is an emotional anticipatory response to future potential threatening or stressful situations, triggering symptoms of negative affective, somatic, behavioral and cognitive components (63). The ECS plays a prominent role in the stress response and anxiety, as it is widely documented mainly by animal studies (64–67). However, our knowledge on the precise molecular mechanisms of the ECS signaling in humans is insufficient (68, 69). In the last years, compelling evidence for the involvement of ECS in anxiety has been accumulated that suggests new therapeutic leads through the discovery of potential biomarkers.
A large body of literature supports the involvement of CB1r as a potential biomarker in anxiety disorders (70–72). CB1r is widely distributed in brain areas associated with emotional regulation and stress responsiveness such as PFC, Hipp, Amy, and Hyp (19). Previous pharmacological studies evaluated the effects of different cannabinoid compounds after either systemic or intracerebral administration in rodents exposed to several animal models of anxiety (73, 74). In addition, it is important to highlight the pivotal role of CB1r in the effects of anxiolytic drugs such as benzodiazepines. Indeed, our group demonstrated that the CB1r antagonist, AM251, completely abolished the anxiolytic effects and significantly reduced the amnesic and the sedative actions induced by alprazolam (75). A very similar result was recently obtained regarding the AM251-induced blockade of the anxiolytic effects of alprazolam (76). On the other hand, the enhancement of CB1r-mediated endocannabinoid function increases the anxiolytic action of diazepam (77).
Accumulated evidence points out that CB1r manipulation produces a bidirectional effect on anxiety-related behavior (78, 79). CB1r activation decreases anxiety at lower doses (80), whereas anxiogenic effects occur at higher doses or after CB1r blockade (70, 81–87). However, several factors could modify this general assumption such as regional endogenous tone, age, sex, species differences, type of test, previous exposure to stressful situations, or dosage of cannabinoid receptor agonists or antagonists. In addition, the underlying mechanisms involved in the bidirectional effects of CB1r pharmacological modulation remain poorly understood. Among the available evidences addressing this aspect, one study revealed that CB1r in the cortical glutamatergic neurons mediates the anxiolytic effect of CP-55,940 cannabinoid agonist at low doses, whereas anxiogenic actions of higher doses are related with CB1r and GABAB receptors in GABAergic terminals (88). A growing body of evidence also suggests that the anxiogenic effects of moderate to high cannabinoid doses appear to be mediated by the interaction between endocannabinoid and endovanilloid systems, specifically through the activation of transient receptor potential cation channel subfamily V member 1 (TRPV1) vanilloid receptors (89). In this regard, the combination of high WIN-55,212 doses in the dorsolateral periaqueductal gray matter (dlPAG) with the TRPV1 antagonist capsazepine abolished the anxiogenic effect (90). Furthermore, the anxiolytic effects of high doses of the cannabinoid agonist ACEA combined with an antagonist of TRPV1 in the rat prelimbic medial prefrontal cortex (PL) suggested the critical interaction between both systems (91). Moreover, the co-administration of intra-dlPAG AEA at higher doses with a nitric oxide (NO) scavenger (carboxy-PTIO) restored the anxiolytic profile, leading to the hypothesis that the increase in anxiety-like behavior mediated by TRPV1 receptors is due to subsequent NO formation (92).
Deletion of CNR1 gene in mice (CB1−/− mice) has been another important tool to elucidate the role of this cannabinoid receptor in anxiety. Many studies have demonstrated the clear anxiety-like behavior of male CB1−/− mice (70–72, 83, 93, 94), although there are some negative results (95). Among the multiple mechanisms involved in the anxious phenotype shown by CB1−/− mice, significant age-dependent alterations in the metabolism of endocannabinoids could be pointed out (96). Interestingly, CB1−/− female mice do not have an anxious phenotype in comparison with female wild-type (WT) subjects. This finding supports an interaction between sex and the ECS at early stages of development that is critical for establishing adult anxiety-like behavior (97). Indeed, these sex-specific effects were also described under pharmacological blockade of CB1r (98). Furthermore, our group described that the effects of the anxiolytic drugs bromazepam and buspirone were missing in CB1−/− mice (99), suggesting a critical role of CB1r that was related with the control of GABAergic responses mediated by GABAA and GABAB receptors (100).
Recent studies provide relevant information regarding the specific brain regional involvement of CB1r-mediated anxiolytic actions. In this sense, the intra-dlPAG administration of AEA, ACEA (selective CB1r agonist) or AM404 (AEA reuptake inhibitor) induced anxiety-like responses that were blocked by AM251 (CB1r antagonist) (101). Similarly, AEA-mediated CB1r activation produces anxiolytic-like actions in the dlPAG employing a panic-like animal model (102, 103) or the Vogel conflict test (104). In addition, facilitation of 2-AG-mediated signaling in the dorsomedial hypothalamus (DMH) significantly reduced panic-like responses in Wistar rats, an effect that was reversed by the CB1r antagonist AM251 (105). Furthermore, activation of CB1r by 2-AG in the basolateral amygdala (BLA) has a critical role in the effects of stress-induced glucocorticoid release on suppression of synaptic GABAergic inhibition (106). Interestingly, pharmacologically-induced elevations of AEA or 2-AG in the BLA decrease anxiety in the elevated plus maze (EPM) test under conditions of low emotional arousal while are ineffective when the level of emotional arousal increased (107). Moreover, electron microscopy revealed CB1r expression in the rat lateral habenula (LHb), mediating the actions of increased 2-AG levels after acute stress exposure, while its blockade by SR141716 (rimonabant) significantly reduced anxiety-like behavior (108). In another study, WIN-55,212 was locally administered in the lateral septum (LS) of male Wistar rats, producing a CB1r-mediated anxiogenic response in the EPM paradigm since AM251 blocked this effect (109). Also, the role of CB1r functional manipulation in anxiety behavior regulation and the effects on subsequent signaling pathways in relevant corticolimbic areas such as PFC, AMY, NAc, and Hipp (110–116) have been evaluated.
A better understanding of the functional connections of the ECS with other neurotransmitter or neurohormonal systems is relevant to understand the role of ECS components as potential biomarkers in psychiatry. According to previous studies, CB1r is located in the locus coeruleus (LC) and in the dorsal raphe nucleus (DRN), and it regulates noradrenaline (NA) and serotonin (5HT) release, respectively, by the modulation of GABAergic and glutamatergic terminals (117, 118). In addition, the dopaminergic and opiodergic systems of the Amy may also be involved in the anxiolytic-like effects induced by the activation of CB1r (119, 120). Furthermore, the involvement of the ECS in the regulation of the hypothalamus–pituitary–adrenal (HPA) axis after stress exposure attracted special attention in the last years (121). Gray and cols. recently found that the stress-related neuropeptide corticotropin-releasing hormone (CRH), acting through the CRH type 1 receptor (CRHR1), reduces AEA levels in the PFC and the Amy by increasing the hydrolysis of FAAH and by increasing 2-AG levels. These data suggest that stress-related elevations in CRH signaling induce persistent changes in eCB function, impairing its tonic regulation on stress and enhancing anxiety responses (122, 123).
Genetic studies pointed out interesting results regarding the involvement of polymorphisms or epigenetic modifications of CNR1 as susceptibility/risk biomarkers to develop anxiety disorders. Lazary and cols. analyzed the interaction of the promoter regions of the serotonin transporter (5HTT; SLC6A4) and CNR1 genes on anxiety. Specific constellations of CB1r and 5HTT promoters were closely associated with high or low synaptic 5HT concentrations, which could result critically in the vulnerability to experience an anxiety disorder (124). Hay and cols. employed CRISPR/CAS9 technology to disrupt a highly conserved regulatory sequence (ECR1) of the gene encoding CB1r (CNR1). This manipulation significantly reduced CNR1 expression in the Hipp, but not in the Hyp, and induced a sex-dependent anxiogenic effect (125). In addition, a connection between ECS and epigenetic mechanisms was proposed. The exposure to immobilization stress increases anxiety-like behavior, an effect blocked by histone deacetylase (HDAC) inhibitors. Interestingly, the CB1r antagonist rimonabant attenuated the anxiolytic-like effects of the HDAC inhibitors, suggesting an association between epigenetic mechanisms and ECS signaling (126). Furthermore, in mice exposed to a chronic unpredictable stress (CUS) there were reduced levels of histone H3K9 acetylation (H3K9ac) associated with CB1r encoding gene (127).
Since the direct pharmacological modulation of CB1r has provided some disappointing results, in recent years much attention has been paid to the therapeutic role of functional manipulation of the endogenous cannabinoid ligands AEA and 2-AG by inhibiting enzymatic degradation (FAAH and MAGL, respectively) or blocking reuptake (128–132). AEA plays a crucial role in emotional control (133). Inhibition of its degradation by FAAH or its reuptake induces a robust anxiolytic effect (134–142). Indeed, stress exposure induces anxiety-like behavior and reduces AEA brain levels (143) by increasing FAAH activity in the Amy (144), a brain region closely involved in AEA-mediated emotional regulation (145). According to the effects observed in FAAH knockout mice (FAAH−/− mice), as well as with the administration of URB597 (FAAH inhibitor), preservation of CB1r function regulating GABA transmission in the striatum may be one the mechanisms involved in the anxiolytic actions of FAAH inhibition (146, 147). Environmental experimental conditions are critical to observe the anxiolytic effect of FAAH inhibition, only present under high stressful or aversive stimuli (148). Interestingly, the dual blockade of FAAH and TRPV1 represents another therapeutic approach to reduce anxiogenic behavior (149). In addition, co-administration of an ineffective dose of URB597 with an ineffective dose of diazepam led to a synergistic anxiolytic action (77).
The endocannabinoid 2-AG also presents a close involvement in emotional regulation linked with signaling in hippocampal glutamatergic neurons (150). In the last years, several evidences support the anxiolytic actions associated with the inhibition of 2-AG enzymatic degradation by means of MAGL (151–153). A link with the HPA axis has been proposed, since the elevation of 2-AG levels was accompanied by a dramatic increase in plasma corticosterone, effect that is probably mediating its anxiolytic actions (154). In addition, increased 2-AG levels in the NAc of mice previously exposed to chronic social defeat stress are associated with an anxiolytic effect and the enhancement of synaptic plasticity (155). Furthermore, the enhancement of 2-AG levels in the dlPAG by the local injection of 2-AG or the hydrolysis inhibitor, URB602, prevented NMDA-induced panic-like response in Wistar rats (156). Interestingly, genetic deletion of MAGL in mice induced an anxiety-like phenotype (157), whereas mice lacking DAGLα showed a high anxiety-like phenotype, strengthening the critical involvement of 2-AG in emotional regulation (158, 159).
Another relevant endocannabinoid biomarker is the CB2r. The first studies demonstrating the role of this receptor in the regulation of anxiety-like behavior were performed in our laboratory employing transgenic animals overexpressing CB2r in the brain (CB2xP mice). Increased expression of CB2r was significantly correlated with reduced anxiogenic-related behaviors. Interestingly, CB2xP mice presented an impaired HPA-axis response to restraint stress, as well as increased GABAAα2 and GABAAγ2 gene expression probably accounting for the lack of anxiolytic action of alprazolam in these animals (160). Furthermore, a pharmacological approach to evaluate acute and chronic effects of the activation (JWH133, CB2r selective agonist) or blockade (AM630, CB2r selective antagonist) of CB2r revealed opposite effects. Importantly, chronic CB2r blockade induced a significant anxiolytic effect that was associated with an upregulation of CB2r, GABAAα2 and GABAAγ2 in the cortex and the amygdala (160). In line with these results, the acute activation of CB2r by the administration of β-caryophyllene (BCP) induced an anxiolytic effect that was completely abolished by AM630-mediated CB2r blockade (161). Recently, Robertson and cols. described that CB2r gene expression is rapidly increased in the Hipp after social stress exposure (social defeat) (162). Genetic manipulation experiments allowed the deepening in the cell-specific functional involvement of CB2r in the Hipp, dissecting the effects of CB2r gene expression disruption in hippocampal neurons or microglia on the regulation of anxiety behavior (163). Moreover, the functional role of CB2r in VTA dopaminergic neurons was also explored. Surprisingly, deletion of CNR2 in VTA dopaminergic neurons induced a very significant anxiolytic effect (47).
In 1981, Fabre and McLendon published the first evidences regarding the anxiolytic properties of cannabinoid compounds. In this study, the synthetic cannabinoid nabilone was administered to 25 patients, producing a significant improvement in anxiety (164). Nowadays, there is a large body of evidence regarding cannabis consumption and regulation of anxiety behavior (165), although the underlying mechanisms are poorly understood. A recent study addressed this issue by combining fMRI and positron emission tomography (PET) in 14 patients following an oral dose of delta-9-tetrahydrocannabinol (THC) while they were performing a fear-processing task. The results suggested that the acute effects of cannabis on anxiety in males are mediated by the modulation of amygdalar function by THC and the extent of these effects are related to local availability of CB1r (166). On the other hand, several clinical trials using rimonabant to treat obesity showed psychiatric side effects such as increased anxiety behavior, depression or even suicidality (167). In spite of the presence of important confounding factors that probably were not appropriately taken into consideration (e.g. psychiatric comorbidity in obese patients), rimonabant was withdrawn from the market, and the enthusiasm in its therapeutic usefulness significantly decreased. Interestingly, a recent report suggested that rimonabant increases anxiety only under an aversive/anxiogenic situation (public speaking), without modifying baseline anxiety behavior (168). Alternative pharmacological approaches to modulate CB1r are now under investigation. Neutral antagonists, peripherally restricted ligands, and allosteric modulators may provide promising results [for a recent review (169)].
The elucidation of genetic variations of different endocannabinoid components involved in the vulnerability to develop anxiety-related disorders has recently gained great interest. In this regard, Gonda and cols. evaluated the interaction between four categories of stressful life events and specific genetic variations in the CNR1 rs7766029 polymorphism, for the development of depression and anxiety. The results suggested that CNR1 rs7766029 interacted significantly with financial but not with other types of life events to increase the vulnerability to develop depression and anxiety (170). In addition, allelic variants of the gene encoding FAAH have been involved in the regulation of anxiety-related behaviors. First, the disturbances of FAAH genetic variation in AEA hydrolysis appear related with alterations in frontolimbic circuits (171), with an age-dependent effect accounting for differences between the adolescence and childhood life stages (172). Second, an interaction between genetic variations of FAAH and corticotropin-releasing hormone receptor type 1 (CRHR1) has been described in relation with the risk to develop anxiety disorders (173, 174). Third, reduced FAAH activity in patients carrying the A allele of the FAAH rs324420 (C385A) polymorphism significantly increases the vulnerability to develop anxiety and depression when exposed to repetitive childhood trauma (175). Moreover, a functional variant of gene encoding CB2r (Cnr2) appears to interact with FAAH gene, increasing the sensitivity for childhood trauma when both are dysfunctional (176).
Major depressive disorder (MDD) has been one of the leading causes of years lived with disability (YLD) during the last three decades (1). According to the World Health Organization estimation for 2015, the number of people living with depression in the world is 322 million, and it is a major contributor to suicide deaths (177). DSM-5 states that the common feature of depressive disorders is the presence of sad, empty, or irritable mood, accompanied by somatic and cognitive changes that significantly affect the individual's capacity to function. MDD is being characterized by distinct changes in affect, cognition, and neurovegetative functions with episodes lasting for at least 2 weeks. Additionally, five or more symptoms have to be present during the same episode, with at least one of the symptoms being either depressed mood or anhedonia (63). Nowadays, pharmacological treatment of MDD entails relevant limitations such as delayed onset of antidepressive actions and appearance of important side effects. The limited success of drug discovery in the context of depression is ultimately linked to an inadequate understanding of the underlying biology of this disorder. In this sense, there is evidence to suggest that the ECS is impaired in MDD providing a unique opportunity to identify potential diagnostic and therapeutic biomarkers.
Martin and cols. employed the CB1−/− mice and exposed them to the CUS procedure. Their findings showed that CB1−/− were more vulnerable to CUS-induced depressive-like responses and presented an increase susceptibility to develop anhedonia (94). Some years later, it was shown that the increased despair behavior in CB1−/− mice was critically associated with down-regulated brain-derived neurotrophic factor (BDNF) levels in the Hipp. Also, local administration of BDNF in the Hipp of these animals reversed the depressive-like phenotype (178). A complete genetic screening by mRNA microarray hybridization revealed a differential gene expression pattern related to the high depressive-like behavior of CB1−/− mice at basal conditions (179). According to the results derived from the studies employing CB1−/− mice, it was proposed that CB1−/− mice could represent a validated and appropriated model to evaluate depressive-like disorders (180).
In the tail suspension test (TST) and forced swimming test (FST), acute AM251 injection induced an antidepressant effect, decreasing the immobility time in both behavioral paradigms (181). Similar results were obtained by both the acute and chronic administration of rimonabant in Wistar rats and BALB/c mice employing the FST and the chronic mild stress (CMS) paradigms, respectively (182). However, other results reveal that the activation of CB1r mediates antidepressant effects (183–187), and even that chronic rimonabant administration produces a depressogenic effect (188). Interestingly, McLaughlin and cols. showed that CB1r located in the dentate gyrus of the Hipp was responsible for the antidepressant effects of the CB1r agonist HU-210 (189). In addition, a very recent study elegantly discovered a circuit-specific CB1r-mediated modulation of glutamatergic transmission that shapes the information flow from BLA to the NAc (190). In this study, the authors consider if the reduction of CB1r in the NAc may be used as a biomarker for MDD diagnosis and point out that this aspect needs to be further determined by evaluation of CB1r levels in the NAc of MDD patients (190).
The evaluation of ECS components disturbances in animal models of depressive disorders provided relevant information. Hill and cols. showed that male Long–Evans rats exposed to the CUS presented increased CB1r binding site density in the PFC while decreased in the Hipp, Hyp and Nac, and lower levels of AEA were found in all these brain regions (191). Furthermore, sex-dependent effects of CUS were analyzed in Sprague-Dawley rats, obtaining lower and higher CB1r protein expression in males and females, respectively, whereas increased FAAH levels were present in both sexes (192). In addition, further studies employing the CUS procedure specifically focused on CB1r-mediated signaling, revealing significant loss of function disturbances in the NAc (193) and in the LHb (194). Moreover, apart from stress-related animal models, Flinders Sensitive Line (FSL) or Wistar Kyoto (WKY) rats are well-known genetic rat models of depression that were recently used to exhaustively analyze disturbances in different components of the ECS in specific brain regions and plasma (195, 196).
Enhancement of endocannabinoid signaling has been postulated as a new promising pharmacological strategy in the treatment of stress-related disorders (e.g. anxiety or depression) (197). Accordingly, a significant reduction in depressive-like behavior was found after the administration of the FAAH inhibitors URB597 (196, 198, 199) or PF3845 (200). In addition, the inhibition of MAGL by the administration of JZL194 also yielded similar antidepressant effects in the CUS animal model of depression. Interestingly, JZL194-mediated effects may be related with an enhancement of adult neurogenesis and long-term synaptic plasticity in the dentate gyrus of the Hipp, probably activating mTOR signaling pathway (201). Furthermore, a recent study evaluated the effects of JZL195, a dual inhibitor of FAAH and MAGL, in WKY rats. JZL195 elevated the endocannabinoids and BDNF levels in the ventral striatum and reduced the depressive-like phenotype in female WKY rats (202).
In spite of the limited available results, CB2r is also critically involved in emotional regulation (203). Probably, the first evidence suggesting the role of CB2r in depression was a significant reduction of these receptors in the striatum, midbrain, and Hipp in an animal model of depression (204). Afterwards, a study showed the antidepressant effects of the CB2r-seletive agonist GW405833 in rats (205). Interestingly, our group further evaluated CB2r involvement in depressive-like behavior regulation using genetic and pharmacological approaches. Mice overexpressing CB2r (CB2xP) presented decreased depressive-like behaviors under basal conditions or after the exposure to a CUS procedure. In addition, the chronic administration of AM630 blocked the CUS-induced depressogenic effect in stressed mice, effect associated with an upregulation of CB2r and BDNF in the Hipp (43). Recently, similar results were obtained by CB2r functional activation through the administration of the CB2r agonists JWH133 (206) and β-caryophyllene (207). Furthermore, the specific deletion of CB2r in midbrain DA neurons in DAT-Cnr2 conditional knockout (cKO) mice significantly increased depressive-like behavior (47).
Crosstalk of ECS with other neurotransmitter or neurohormonal systems plays a pivotal role in the effects produced by antidepressant drugs. In this regard, interactions with the serotonergic system represent a critical point due to the widely recognized clinical therapeutic usefulness of antidepressants targeting serotonin (e.g. serotonin selective reuptake inhibitors, SSRIs). SSRIs fluoxetine and escitalopram modify the concentrations of different ECS components under basal conditions (208–210) or in an animal model of depression (211). Furthermore, low doses of WIN-55,212 produced antidepressant-like actions that appeared to be mediated by 5HT (212), and CB1−/− mice have decreased levels of 5HT transporter (5HTT) (213). Moreover, co-administration of a subeffective dose of fluoxetine potentiated the effect of subeffective doses of AEA, AM404 or URB597 (214). In addition, ECS also interacts with other systems involved in emotional and stress regulation such as the HPA axis (215), glutamatergic (216), opioidergic (217), and cholinergic (218) systems.
On the other hand, it is relevant to highlight that nonpharmacological approaches such as repeated transcranial magnetic stimulation (rTMS) improve depressive-like behavior, at least in part, by modulating the ECS. Recent studies performed in rodents exposed to CUS and subsequently treated with rTMS revealed that: 1) rTMS increases BDNF production and hippocampal cell proliferation to protect against CUS-induced changes through its effect on CB1r (219); 2) rTMS antidepressive effects are at least partly mediated by increasing hippocampal 2-AG and CB1 receptor expression levels (220); and 3) high-frequency rTMS induces its antidepressant effect by upregulating DAGLα and CB1r (221). In addition, electroconvulsive therapy (ECT) significantly reduced AEA content and FAAH activity in the PFC of Sprague-Dawley rats, as well as decreased and enhanced binding site density of the CB1r in the PFC and Amy, respectively (222).
Besides the preclinical clues supporting the critical role of ECS in depression, currently there is a broad body of evidence available from clinical studies. Among them, those evaluating alterations in different ECS components in post-mortem brain tissue or plasma samples have provided compelling results. The first evidence revealed that CB1r protein expression was decreased in the anterior cingulate cortex (ACC) of patients with major depression (223). Furthermore, Choi and cols. showed that CB1r mRNA levels were higher in the PFC of major depression patients (224). However, in a recent study a lack of CB1r protein expression differences was found between depressive subjects and paired control patients (225).
In the last years, an increasing effort has been made to elucidate the alterations of ECS components (mainly the endocannabinoids AEA and 2-AG) in blood samples of patients with depression, to identify possible trait, prognosis or monitoring biomarkers that could improve the therapeutic approach. In a cohort of 28 women with diagnostic criteria for clinical depression and without medication, serum 2-AG content was significantly decreased, and this decrease was negatively correlated with duration of the depressive episode (226). Similarly, basal serum concentrations of AEA and 2-AG were significantly lower in women with nontreated major depression, and the exposure to a stressful situation significantly increased 2-AG concentrations without modifying AEA (227). However, another study described increased plasma concentrations of both AEA and 2-AG in depressed patients, and the elevation of 2-AG was significantly associated with SSRI antidepressant therapy (228). Interestingly, the antidepressant-related effects or physical exercise on eCBs levels were also analyzed. Intense exercise in control healthy patients induced a significant increase in AEA serum levels that was correlated with higher BDNF levels, whereas 2-AG concentrations remained stable (229). On the contrary, moderate exercise in women with MDD produced significant elevations in AEA but not in 2-AG, although both eCBs presented significant moderate negative associations between serum changes and mood states (230). Finally, ECT significantly elevated AEA and 2-AG levels in the cerebrospinal fluid (CSF) of patients with major depression (231).
The ECS-related polymorphic gene variant study results are relevant because of the potential diagnostic and therapeutic implications. Regarding the CNR1 and the single nucleotide polymorphism (SNP) rs1049353 (G1359A) that may contribute to the susceptibility to mood disorders (232), G-allele has been associated with higher depressive-related symptomatology (233) and increased risk of antidepressant treatment resistance in women with comorbid anxiety disorder (234). However, it provides a better response to citalopram in male depressive patients (235), whereas A-allele decreased risk to develop depression because of childhood physical abuse (236). Furthermore, several CNR1 polymorphisms appeared to be related with high neuroticism and low agreeableness personality traits, increasing the risk to develop depression (237). On the other hand, the presence of 2 long alleles of the polymorphic triplet (AAT)n of CNR1 gene was associated with reduced prevalence of depression in Parkinson's disease patients (238). In addition, the minor C allele of the CNR1 rs2023239 polymorphism may confer a protective effect against lifetime development of MDD in methadone-maintained patients (239). Despite the previous findings, a recent meta-analysis points out that CNR1 rs1049353 or AAT triplet repeat polymorphism had no association with susceptibility to depression (240).
Other relevant gene polymorphisms of the ECS are those related with FAAH and CB2r. First, variants of the FAAH gene may be related with susceptibility to mood disorders such as major depression (232). In fact, genetically reduced FAAH activity in A allele carriers of FAAH rs324420 (C385A) polymorphism constitutes a risk factor to develop anxiety and depression in patients exposed to repetitive childhood trauma. Interestingly, the authors noted that this genotype could entail pharmacogenomic consequences, namely ineffectiveness or adverse effects of FAAH inhibitors in this subpopulation (175). Second, polymorphisms of CNR2 were first studied by Onaivi and cols. in Japanese depressed patients, revealing a high incidence of Q63R but not H316Y polymorphism (204, 241). Recently, the R allele of Q63R CNR2 polymorphism, together with the A allele of FAAH C385A polymorphism were associated with increased sensitivity for childhood trauma and subsequent expression of anxious and depressive phenotypes (176). Finally, according to the previously mentioned recent meta-analysis performed by Kong and cols., CNR2 rs2501432 polymorphism might be closely associated with depression (240).
According to the DMS-5 schizophrenia is a psychotic disorder associated with a myriad of signs including positive symptoms (delusion, hallucinations, disorganized speech or grossly disorganized or catatonic behavior), negative symptoms (lack of motivation and social withdrawal), and cognitive symptoms (reduced attention and altered speech) (63, 242, 243). An extensive body of literature supports the role of ECS in schizophrenia neuropathology, a fact that is mainly sustained by the psychotic effects derived from cannabis consumption and attributed to the exogenous cannabinoid THC (244). Therefore, a great interest has been posed in the identification of specific biomarkers related with ECS functioning for preventive, diagnostic, or therapeutic purposes.
Preclinical research that focused on the role of ECS in schizophrenia relies on the evaluation of sensorimotor gating deficits by the prepulse inhibition (PPI) paradigm (245, 246). Among all the components of the ECS, CB1r is critically involved in schizophrenia. In fact, results of studies using pharmacological approaches showed that CB1r activation induces psychotic-like effects, while blockade of CB1r presents opposite actions. Decreases in startle responses together with PPI disruption were achieved by CP-55,940 administration, and rimonabant completely reversed these effects (247). A similar experiment was carried out in which CP-55,940 decreased startle response and impaired PPI, and rimonabant significantly reversed CP-55,940-induced deficits in PPI only at the lower prepulse intensity (248). This CB1r-mediated auditory gating disruption was further confirmed by measuring neuronal network oscillations in the Hipp and entorhinal cortex of Sprague-Dawley rats. CP-55,940 significantly impaired sensory gating and neuronal oscillation, an effect that was reversed by AM251 (249).
After learning that the modulation of the CB1r produced sensorimotor alterations, different animal models of schizophrenia were used to find out if CB1r blockade could be a strategy with therapeutic potential. Blockade of N-methyl-D-aspartate (NMDA) receptors (NMDAr) was used to simulate schizophrenia-like symptoms in rodents (250). Interestingly, the administration of AM251 significantly abolished phencyclidine-induced disruption of PPI in a similar way to clozapine (251), as well as impairments in recognition memory or increased behavioral despair in the FST (252). Another NMDAr antagonist used to model schizophrenia-like behavior is MK-801. The administration of the CB1r antagonist AVE1625 reversed MK801-induced cognitive impairments and decreased catalepsy and weight gain induced by clinically used antipsychotic drugs (haloperidol, olanzapine) (253). Furthermore, AM251 attenuated amnesic effects and hyperactivity induced by MK-801 (254). Therefore, it appears that blockade of CB1r may have relevant therapeutic applications for the treatment of schizophrenia.
In the so-called ‘three-hit' animal model of schizophrenia, CB1r binding and cannabinoid agonist-mediated G-protein activation decreases in the cortical, subcortical, and cerebellar brain regions (255). In a neurodevelopmental animal model of schizophrenia induced by the gestational administration of methylazoxymethanol (MAM), CB1r mRNA levels were lower in the PFC and higher in the dorsolateral striatum of adult MAM-treated Sprague-Dawley rats relative to the control group (256). Moreover, in the spontaneously hypertensive rat (SHR) strain, partially reproducing some schizophrenia-like behavioral aspects, CB1r immunoreactivity was significantly increased in the PL, cingulate cortex, and CA3 region of the Hipp (257). Recently, two studies found decreased methylation of the cannabinoid receptor interacting protein (CNR1P1) DNA promoter in the ventral Hipp (vHipp) of rats exposed to the MAM model (258, 259). CNR1P1 is an intracellular protein that interacts with the C-terminal tail of CB1r and regulates its intrinsic activity. Interestingly, a lentivirus-mediated overexpression of CNR1P1 in the vHipp of Sprague-Dawley rats induced significant schizophrenia-like cognitive and social interaction impairments, together with an increase of dopamine neuron population activity in the VTA (260).
Apart from the many preclinical studies supporting the pivotal role of CB1r, animal models of schizophrenia provided interesting results about eCBs brain level alterations. In this regard, a significant increase in 2-AG levels in the PFC of PCP-treated Lister-Hooded rats was reversed by treatment with THC, which in turn induces a large reduction of AEA in the same region (261). Furthermore, in Sprague-Dawley rats exposed to a bilateral olfactory bulbectomy, considered as an animal model of depression and schizophrenia, a significant decrease of AEA and 2-AG levels was found in the ventral striatum (262). In addition, mice with a heterozygous deletion of neuregulin 1 (Nrg 1 HET mice), a well-accepted and characterized animal model of schizophrenia (263), displayed relevant alterations in eCBs levels (264).
Finally, CB2r has also been recently involved in schizophrenia. Ishiguro and cols. studied the effects of the pharmacological blockade of CB2r in two animal models of schizophrenia induced by the administration of MK-801 or metamphetamine. The CB2r antagonist AM630 significantly exacerbated the MK-801- or metamphetamine-induced hyperlocomotion and PPI disruption, suggesting that CB2r was mediating these actions (265). Our group analyzed exhaustively the behavioral profile of CB2−/− mice to evaluate the implication of CB2r in schizophrenia-like behavior. The phenotype showed by CB2−/− mice resembled some relevant features of schizophrenia such as increased sensitivity to motor effects of cocaine, anxiety- and depressive-like behavior, disrupted short- and long-term memory consolidation and impaired PPI. These behavioral alterations were accompanied by gene expression changes in different targets from dopaminergic, noradrenergic, and serotonergic systems. Interestingly, the atypical antipsychotic risperidone significantly improved PPI disruption induced by CB2r deletion and differentially modulated some of the neurochemical disturbances compared with WT mice (266). In addition, the activation of CB2r by the agonist JWH015 reversed PPI disruptions of the MK-801-induced animal model of schizophrenia, and this effect was specifically mediated by CB2r since only AM630 but not AM251 abolished PPI improvement (267). Furthermore, activation of CB2r (JWH133) and blockade (AM630) increased MK-801-induced hyperlocomotion, although this effect was much more evident and pronounced with AM630 (268). Therefore, these results strongly suggest that CB2r functional regulation is significantly involved in schizophrenia-like behavior.
To date, an extensive and great effort has been made to elucidate the role that CB1r plays in schizophrenia. Accumulated clinical data clearly shows significant alterations of CB1r protein and gene expression levels, as well as certain CNR1 polymorphisms correlations, especially in the brain but also in the peripheral blood cells from schizophrenic patients in comparison with healthy control subjects. The information reviewed and detailed below provides important clues to further investigate the application of CB1r-related measures as potential trait, state, prognostic or even therapeutic biomarkers.
Several published studies analyzed CB1r protein and gene expression levels in different post-mortem brain regions from schizophrenic patients. Several studies examined quantitative autoradiography to evaluate CB1r availability through the binding of different radioligands. A significant increase in CB1r availability was shown in the dorsolateral prefrontal cortex (DLPFC) (269–271), although this increase was only present in paranoid schizophrenic patients (272). Interestingly, a recent study failed to show differences in CB1r-mediated functional coupling to G-proteins in the PFC of schizophrenic and control patients (273). Furthermore, higher CB1r binding levels were shown in the left ACC (274) and in superficial layers of the posterior cingulate cortex (PCC) (275), whereas no changes were found in the superior temporal gyrus (STG) (276) from schizophrenic patients. In contrast, some authors reported lower CB1r protein levels measured by immunocytochemistry (277) or Western blot (278) and decreased CB1r gene expression analyzed by in situ hybridization (277) or quantitative real time polymerase chain reaction (qRT-PCR) (273) in the PFC from schizophrenic patients compared with control subjects. Volk and cols. specifically addressed this apparent discrepancy between CB1r binding and protein or gene expression levels. In a cohort of 21 schizophrenic patients presenting lower levels of both CB1r mRNA and protein in the PFC, relative to matched healthy comparison subjects, they obtained an increased CB1r binding (271).
Neuroimaging experiments were recently carried out to obtain an in vivo approximation of the disturbances related with CB1r in schizophrenia. In this regard, PET studies yielded dissimilar results. Wong and cols. studied CB1r binding employing the novel PET tracer [11C]-OMAR (JHU 75528) in schizophrenic patients and matched controls. CB1r binding was higher in several brain regions of patients with schizophrenia, only reaching statistical significance in the pons. Interestingly, a significant correlation was found between CB1r binding and schizophrenia-related symptomatology (279). In addition, Ceccarini and cols. also showed a significant increase of CB1r binding in the NAc, insula, cingulate cortex, inferior frontal cortex, parietal and mediotemporal lobes in schizophrenic patients compared with controls measured with [18F]-MK-9470 PET. It is relevant to highlight that in the nontreated schizophrenia patients, CB1r binding was negatively correlated to negative symptoms and to depression scores, especially in the NAc (280). On the contrary, Rangathan and cols. obtained an opposite result with lower CB1r availability levels ([11C]-OMAR PET) in the Amy, caudate, PCC, Hipp, Hyp, and insula of schizophrenic patients (281). An interesting commentary on these discrepancies was published, in which several confounding factors such as symptom severity, sex, age, PET tracer, statistical analysis method or comorbid nicotine use are discussed. Overall, it could be concluded that CB1r has an important but yet complex and poorly understood role in schizophrenia (282). Finally, a very recent study examined CB1r availability by [18F]-FMPEP-d2 or [11C]-MePPEP PET, in first episode psychosis (FEP). Significant lower CB1r availability was found in patients with schizophrenia, independently of antipsychotic medication treatment. Greater reduction in CB1r availability was associated with greater symptom severity and poorer cognitive functioning (283).
The possible association between CNR1 polymorphisms and schizophrenia has been explored. In this sense, negative results were obtained with a single-base polymorphism within the first exon of the CNR1 (284), the polymorphism rs1049353 1359G/A at codon 453 in the coding region of CNR1 (285–288), or other CNR1 polymorphisms such as rs6454674 (287), AL136096 (287), rs806368 (288, 289), rs806379 (288), rs806380 (288), rs806376 (289), and rs806366 (289). However, significant associations of CNR1 polymorphisms rs7766029, rs806366, and rs1049353 were described (290). Regarding (AAT)n triplet repeat in the promoter region of the CNR1 gene, discrepant results were reported since Tsai and cols. suggested that this polymorphism was not directly involved in the pathogenesis of schizophrenia in a Chinese population (291), whereas it was significantly associated with the hebephrenic or disorganized subtype of schizophrenia (285). Interestingly, some relevant associations were identified between specific CNR1 polymorphisms and therapeutic response. Hamdani and cols. described increase G-allele frequency of the rs1049353 polymorphism in responsive schizophrenic patients, with a dose effect of the G allele. Thus, the authors proposed that the G allele of CNR1 rs1049353 polymorphism could represent a “psychopharmacogenetic” biomarker to take into consideration for the treatment of schizophrenia (288). In addition, in 65 FEP patients, TT genotype of the CNR1 rs2023239 polymorphism was associated with a better improvement of negative and positive symptoms (292). Similarly, in another group of patients with FEP, carriers of rs7766029 CC genotype presented significantly higher improvement in verbal memory and attention while carriers of rs12720071 AG genotype showed a better improvement in executive functions (293). Furthermore, minor alleles of CNR1 polymorphisms rs6928499, rs1535255, and rs2023239 might be associated with a lower risk to develop antipsychotic-induced metabolic syndrome. These relevant data could result in potential pharmacogenetic applications to optimize drug management of schizophrenic patients (294). On the contrary, one study showed that G allele carriers of the CNR1 rs1049353 (G1359A) polymorphism might be associated with a poorer therapeutic response (233).
Gene and protein analysis of CB1r in peripheral blood cells from schizophrenic patients attracted much attention. Peripheral cell (e.g. lymphocytes) changes could be mirroring, at least in part, some of the neuropathological hallmarks of the disorder. In this regard, the first published study did not detect changes in the CB1r mRNA levels in peripheral blood mononuclear cells (PBMCs) between schizophrenia and control patients (295). Similarly, no differences were observed in CB1r levels of peripheral immune cells by flow cytometry between control and schizophrenic patients, although a positive correlation between CB1r expression on monocytes and cognitive impairment was detected (296). However, an opposite result revealed an increase of CB1r in PBMCs of schizophrenic patients also evaluated by flow cytometry (297). Furthermore, there is an increase of CB1r mRNA levels in PBMCs of schizophrenic patients (298, 299) that may correlate with a reduced DNA methylation of CNR1 promoter region (299). Moreover, CB1r gene expression was correlated positively with positive and negative syndrome scale (PANSS) total symptom severity and negatively with cognitive functioning measures (298).
Besides the extensive literature evaluating the role of CB1r in schizophrenia, some efforts were done to complete the picture regarding the involvement of eCBs and its degrading and synthesizing enzymes as biomarkers. Leweke and cols reported a significant increase of AEA levels in the CSF of schizophrenic patients (300). In antipsychotic naïve first-episode paranoid schizophrenic patients, there was an eightfold increase in AEA levels in the CSF, whereas no alteration was present in patients treated with typical but not atypical antipsychotics. Furthermore, AEA levels were negatively correlated with psychotic symptoms in nonmedicated acute schizophrenics (301). Similarly, blood AEA levels were higher in patients with acute schizophrenia and were normalized with the clinical remission (295). Increased AEA levels were also detected in the CSF of schizophrenic patients who used cannabis. Interestingly, the increase of AEA was more than 10-fold higher in low-frequency compared with high-frequency cannabis users (302). In addition, higher AEA serum levels were obtained in twin-pairs discordant for schizophrenia (303), or in schizophrenic patients with substance use disorder (SUD) comorbidity, considering that baseline AEA predicted endpoint SUD scores (304). However, other studies showed different results such as no changes in serum AEA levels (305), increased 2-AG and decreased AEA in the cerebellum, Hipp, and PFC of schizophrenic patients (306).
With regard to degrading or synthesizing eCBs enzymes, the relationship of some FAAH or NAPDE-PLD polymorphisms with schizophrenia was studied, but no significant associations were obtained (290, 307). In addition, FAAH and MAGL mRNA levels were similar while FAAH activity was higher in the PFC of schizophrenic patients compared to controls (273). Interestingly, a reduction of FAAH mRNA levels correlated with clinical remission in schizophrenic patients (295). Furthermore, in FEP patients, some interesting correlations were detected between peripheral FAAH and DAGL expression and short-term verbal memory, NAPE-PLD expression and working memory, and MAGL expression and attention. Accordingly, the authors suggested the use of these ECS elements as biomarkers or pharmacological targets for FEP (308). Finally, mRNA levels of the 2-AG metabolizing enzyme, α-β-hydrolase domain 6 (ABHD6), were significantly increased in patients with schizophrenia (309).
Notwithstanding the scarce literature exploring the role of CB2r in schizophrenia, some important findings suggest its involvement and draw attention to research on its therapeutic potential. Perhaps, de Marchi and cols. published the first evidence measuring CB2r mRNA levels by semi-quantitative RT-PCR in PBMCs from schizophrenic patients in their acute phase, and when clinical remission was achieved after antipsychotic treatment with olanzapine. CB2r gene expression significantly decreased in PBMCs from patients in clinical remission (295). In FEP patients, CB2r protein expression was significantly down-regulated together with reduced levels of eCBs synthesizing enzymes (NAPE-PLD and DAGL) (310). Interestingly, increased CB2r gene expression was found in schizophrenic patients' PBMCs (298), correlating with PANSS and cognitive performance severity (296, 298), and in cells of the innate immune system (297). On the other hand, Ishiguro and cols. evaluated the implication of specific CNR2 polymorphisms in schizophrenia. R63 allele of rs2501432 (R63Q), C allele of rs12744386, and the haplotype of the R63-C allele were significantly increased in patients with schizophrenia in comparison with control subjects. Apparently, these polymorphic alterations of CNR2 are associated with loss of function. A lower response to CB2r ligands was found in cultured CHO cells transfected with the R63 allele. Reduced CB2r mRNA and protein expression levels were found in the DLPFC of schizophrenic patients independently of the diagnosis (265). In addition, the association between three CNR2 polymorphisms (rs2501432C/T, rs2229579C/T, rs2501401G/A), and schizophrenia was explored (311). However, other CNR2 polymorphisms (rs6689530 and rs34570472) were not associated with schizophrenia in a Korean population (289).
Bipolar disorder (BD) is a debilitating, lifelong neuropsychiatric illness characterized by unsteady mood states alternating from (hypo)mania to depression. According to the DSM-5, for a diagnosis of BD it is necessary to meet specific criteria for a manic episode that may be followed by hypomanic or major depressive episodes (63). Despite the availability of effective pharmacological agents, BD is inadequately treated in a subset of patients, so the identification of new therapeutic targets is necessary. In this sense, the close implication of ECS in mood regulation suggested its involvement in BD (312). This assumption is supported by the observation of the effects of high doses of cannabis and THC in healthy patients, producing psychosis, sometimes with marked hypomanic features (313). In addition, THC and cannabidiol (CBD), the main components of Cannabis sativa plant, may present mood stabilizing properties. Therefore, there is an increasing interest to evaluate ECS implication in BD.
First studies were focused on the evaluation of polymorphisms of CNR1 gene in BD pathophysiology. One study carried out in patients with BD within a Turkish population investigated the implication of three types of polymorphisms of CNR1 in this disease, demonstrating that only one of them (rs6454674) could be correlated with BD. In addition, the mean of the yearly maniac attacks was statistically higher in patients presenting heterozygote rs6454674 T/G polymorphisms compared to those with homozygote polymorphism (314). In addition, the association of CNR1 rs1049353 (1359 G/A) and FAAH rs324420 SNP (cDNA 385C to A) polymorphisms with BD was assessed in a Caucasian population. Here, the authors concluded that the distribution of CNR1 1359 G/A genotypes and alleles did not differ between BD and healthy patients, whereas the frequency of the AC genotype of FAAH (cDNA 385C to A) polymorphism was slightly higher in BD patients (232).
Nevertheless, other studies did not identify differences in ECS components between BD and healthy controls. Indeed, no differences were obtained between BD patients and healthy controls in DNA methylation of the CNR1 gene promotor region (299). Furthermore, a polymorphism of CNR1 promotor region was evaluated in another study, and no changes were observed in BD patients, concluding that it was not likely to relate with BD (315). Koethe and cols. carried out a study with post-mortem brain samples from BD patients and controls, evaluating numerical density of neurons and immunopositive glial cells for CB1r. No changes were found in these patients (223). Furthermore, in another study evaluating polymorphisms of CNR1 and FAAH, no significant differences or association were observed in BD patients (316).
Because of these contradictory results, some authors shifted their attention to the implication of CB2r in BD, with limited but promising findings. A genetic association was observed in patients with BD and CNR2 rs41311993 (524C/A) polymorphism, but not SNPs of rs2229572 (1073C/T) or rs2501432 (315A/G), suggesting that CB2r may play a role in BD (317). In addition, a genome-wide association study carried out in a population from the UK biobank, identified the association of a locus in CNR2 with distressing psychotic experiences, providing support for a shared genetic liability with BD and other neuropsychiatric disorders (318).
In summary, there is limited information about the implication of ECS in the pathophysiology of BD. Thus, more preclinical and clinical studies are needed to explore further its role in the development of this neuropsychiatric disorder and its usefulness as a therapeutic target to improve BD management.
Post-traumatic stress disorder (PTSD) is a chronic and disabling mental disease caused by the exposure to stressful, frightening or distressing events, and is included in the category of trauma- and stressor-related disorders in the DSM-5 (63). PTSD patients experience intrusion symptoms, persistent avoidance of any stimuli associated with the traumatic event, negative alterations in cognition and mood, and disturbances in arousal and reactivity, that must last more than 1 month and produce distress or functional impairment (63). The neurobiological mechanisms underlying PTSD-related symptomatology are not completely understood, being a limiting factor to identify new therapeutic targets. In this regard, a relevant association between ECS and PTSD was suggested providing interesting results about the potential development of new pharmacological approaches. Indeed, preclinical and clinical findings point out the involvement of certain ECS components in PTSD symptomatology, such as CB1r or FAAH, suggesting its potential role as biomarkers for PTSD (319, 320).
The involvement of CB1r in PTSD is supported by the presence of this receptor in brain areas regulating the response to stress and to changes observed in different animal models of PTSD. For instance, using a shock and reminder model of PTSD, higher mRNA levels of CB1r were detected in the BLA (133), and increased CB1r protein expression was found in the BLA and the CA1 region of the Hipp (321) of exposed mice as well. On the other hand, in a predator exposure-based PTSD model, anxiety-like behavior was negatively correlated with CB1r gene expression in the PFC and the amygdaloid complex, whereas no changes were observed in the Hipp (322). In addition, Xing and cols. reported in young Sprague-Dawley rats, that the exposure to an unpredictable electric shock model of PTSD induced a down-regulation of CB1r gene expression in comparison with nonstressed rats. Interestingly, the authors showed sex differences in the stress-related regulation of CB1r, showing that females presented higher mRNA levels of CB1r, as well as greater CB1r inactivation by phosphorylation. The authors concluded that these sex-related differences could lead to increased susceptibility to stress-related anxiety disorders, including PTSD, in females (323). A genetic approach was used to evaluate further the involvement of CB1r in the regulation of stress response. Repeated exposure to an acoustic stressor (high intensity bell sound) did not produce changes in adrenocorticotropin hormone (ACTH) or corticosterone (CS) levels in CB1−/− mice. These results suggested that the presence of CB1r is essential in the regulation of the stress response, and that CB1−/− mice may result appropriate to model some forms of PTSD (324).
Pharmacological manipulation approaches of the CB1r were also explored in several rodent models of PTSD and its potential usefulness as a therapeutic biomarker. The blockade of CB1r with rimonabant increased freezing behavior in a PTSD model of shock and reminder during cued expression/extinction training (325). On the other hand, several authors evaluated the effects of cannabinoid activation by WIN-55,212-2 administration into hippocampal CA1 region. The results showed a normalization of shock-induced upregulation of CB1r in the PFC and CA1 region of the Hipp (321, 326) and facilitation of inhibitory avoidance extinction in a fear-related inhibitory avoidance paradigm (327). All these effects were blocked by AM251 administration. Furthermore, WIN-55,212 administration into the BLA normalized stress-induced effects on inhibitory avoidance and acoustic startle response and facilitated fear extinction in a single prolonged stress (SPS) model of PTSD. These effects were blocked by AM251 (321, 328, 329). Similarly, the injection of WIN-55,212 in the NAc of rats exposed to a shock and reminder model of PTSD significantly facilitated the fear extinction process (330). Interestingly, Goodman and Packard demonstrated that systemic or intradorsolateral striatum (DLS) administration of WIN-55,212 could impair the consolidation of stimulus–response memory, suggesting relevant consequences for neuropsychiatric disorders such as PTSD (331). However, the intra-PFC administration of WIN-55,212 did not modulate fear extinction disturbances induced by the exposure to the SPS model (329). Finally, according to the results obtained with WIN-55,212, Reich and cols. studied the effects of a CB1r selective agonist, ACEA, in rats exposed to 3 weeks of a chronic-mild-unpredictable protocol followed by fear conditioning evaluation. In this study, ACEA administration significantly reduced freezing behavior in stressed rats, enhancing long-term extinction of fear-related memories (332).
In order to validate that cannabinoid activation improves disturbances induced by stress- or trauma-related stimuli, the effects of pharmacological endocannabinoid signaling facilitation were analyzed. In a fear conditioning paradigm, the administration of AM404 led to a dose-dependent enhancement in fear extinction, as well as a decreased shock-induced reinstatement of fear. Interestingly, the administration of rimonabant together with AM404 reversed the improvement of extinction, suggesting that AM404 effects were related to an increase in CB1r activation during extinction training (333). In addition, the injection of the FAAH inhibitor, URB597, normalized the upregulation of CB1r in the CA1 of Hipp and BLA of rats exposed to a shock and reminder model of PTSD (334) and attenuated startle response and anxiety-like behavior in a predator exposure animal model of PTSD (335). Interestingly, these effects were abolished by CB1r blockade, suggesting the implication of CB1r on URB597 effects (335, 335). Similar results were obtained with the administration of URB597 into CA1 (Hipp) and BLA brain regions, showing a facilitation of extinction processes and attenuation of startle response, anxiety- and depression-like behaviors mediated by CB1r activation (133, 336, 337). Furthermore, URB597 administration additionally prevented the increase of CB1r levels in CA1 and BLA after rodent exposure to shock and reminder model of PTSD (321).
FAAH inhibition significantly facilitates CB1r-mediated signaling of AEA and can produce a greater beneficial spectrum of biological effects than those caused by direct CB1r activation. Interestingly, the role of FAAH in learning and memory was evaluated by using another FAAH inhibitor, OL-135. The administration of this drug increased acquisition and extinction rates in mice exposed to fixed platform water maze test. Rimonabant blocked OL-135-induced effects on both acquisition and extinction levels (338). In the same study, the authors revealed that FAAH−/− mice phenotype was similar to that obtained after OL-135 administration, suggesting that the increase in AEA levels facilitates extinction processes, and that CB1r would be critically involved (338). FAAH inhibition and the consequent increase of AEA in the brain regions involved in the regulation of stress and anxiety seem to restore dysfunctional homeostasis of AEA signaling because of stress exposure. Thus, FAAH must be strongly considered as a target for PTSD management (139).
According to the involvement of ECS components in several behavioral traits of PTSD in animal models, various studies explored alterations in different biological samples (post-mortem brain tissue, blood, hair) collected from PTSD patients and adequately paired controls. At the peripheral level, some authors studied the possible correlation between CNR1 polymorphisms and PTSD symptoms. The rs1049353 polymorphism of CNR1 was studied in PTSD patients to correlate specific alleles or genotypes with fear and/or dysphoric symptoms of PTSD. This study suggested that rs1049353 polymorphism interacts with childhood physical abuse to increase fear but not dysphoric symptoms in PTSD (339). In another study carried out in a Caucasian population, the association between variants of CNR1 gene haplotypes and diagnosis of PTSD was studied. The authors reported that the variant C-A was more common in PTSD cases compared to non-PTSD controls, and the variant C-G was less common in PTSD compared to non-PTSD patients (340).
A different approach was the measurement of plasmatic eCBs in a selected cohort of patients that suffer the terroristic attacks of the World Trade Center in 2001 and met the diagnostic criteria for PTSD. 2-AG, AEA, and cortisol concentrations were measured. Only 2-AG was significantly reduced in PTSD patients, while no significant differences were found in AEA or cortisol concentrations (341). Another study showed reduced AEA and cortisol concentrations in PTSD patients compared to healthy controls with lifetime clinical histories of trauma (342). Despite these contradictory results, the fluctuations in plasmatic concentrations of eCBs may affect the reproducibility of the evaluation. Thus, the assessment of eCBs alterations in hair samples provides a more stable measurement. Hair concentrations of PEA (palmitoylethanolamide), OEA (oleoylethanolamide) and SEA (steraoylethanolamide) were measured in war survivors with and without PTSD. A regression analysis revealed a strong negative relationship between these endocannabinoids and the severity of PTSD symptoms. OEA concentrations were significantly reduced in hair samples from PTSD patients (343).
Only one human study analyzed CB1r binding using the CB1r-selective radioligand [11C]OMAR by PET. Results showed elevated CB1r binding values, especially in women, together with lower AEA and cortisol in PTSD patients. The authors suggested that abnormal CB1r-mediated AEA signaling is involved in the etiology of PTSD (342). In addition, fMRI was also used to evaluate FAAH implication in PTSD symptomatology. A common SNP (C385A) in the human FAAH gene was correlated with the quicker habituation of amygdala reactivity to threat and lower score on stress-reactivity. This variant reduced FAAH activity and possibly increased AEA-induced endocannabinoid signaling (344–346). Furthermore, Rabinak and cols. conducted an fMRI study with healthy volunteers and patients receiving acute dronabinol (synthetic THC) oral administration in a standard Pavlovian fear extinction paradigm. Interestingly, dronabinol enhanced extinction learning, providing the first evidence about the feasibility of pharmacological enhancement of extinction learning in humans using cannabinoid system modulators (347, 348). Some clinical trials with PTSD patients suggested the usefulness of dronabinol for improving the global PTSD symptom severity, sleep quality, frequency of nightmares, and PTSD hyperarousal symptoms (349). Similar results were obtained with nabilone, since its administration to PTSD patients improved insomnia, PTSD symptoms, and global assessment of functioning, reducing the frequency and intensity of nightmares (350, 351). Nevertheless, more randomized and controlled clinical trials are needed to confirm dronabinol or nabilone potential therapeutic application in the management of PTSD.
ADHD is a neuropsychiatric disorder characterized by persistent pattern of inattention and/or hyperactivity-impulsivity that interferes or reduces the quality of social, academic, or occupational functioning in accordance with DSM-5 (63). In the last years, the identification of different components of the ECS that are potentially involved in ADHD pathophysiological mechanisms has attracted much attention as shown below.
An experiment carried out in SHR rats (an animal model reproducing some features of ADHD) evaluated the modulating effects of the cannabinoid system on impulsivity, using a delay reinforcement task and the administration of WIN55212-2 or AM251 (352). This study concluded that treatment with WIN55212-2 decreased whereas AM251 increased the choices of the large reward, suggesting that CB1r plays a relevant role in impulsive behavior. Furthermore, basal gene and protein expression of CB1r in the brainstem of SHR rats was significantly lower in comparison with their normotensive counterpart, Wistar rats (353). Moreover, the overexpression of four genes, between them CNR1, was strongly associated with overall poor performance on mice during their gestational growth because of a malnutrition via high-fat or low-protein diets on the dam (354). These abnormal disturbances on diet in the gestational period are linked to the etiology of multiple neurodevelopmental disorders, including ADHD (355).
The psychostimulant drug amphetamine is often prescribed to treat ADHD. The administration of amphetamine increases monoamine neurotransmission in the brain regions as NAc and medial PFC. Accumulating reports supported the role of CB1r in the regulation of monoamine release, suggesting its possible involvement in ADHD. The administration of rimonabant did not affect monoamine release whereas dose-dependently abolished amphetamine-induced dopamine release in the NAc. This result suggested that CB1r is essential to reach the therapeutic effect of amphetamine, mediated at least in part, by the enhancement of dopaminergic signaling in the mesolimbic system in the NAc (356).
As previously stated, there is large available evidence regarding the role of different variants of CNR1 gene in psychiatry. In relation to ADHD, SNP variants at the CNR1 gene were tested on a family-based sample of trios (an ADHD child and their parents) and on an unselected adolescent sample from Northern Finland. The study detected a significant association of a SNP haplotype (C-G) with ADHD suggesting a greater risk in males than females (340). Another study reported the interaction of the two most studied CNR1 polymorphisms, rs806379 and rs1049353, that are involved with early psychosocial adversity (357). These polymorphisms of the CB1 receptor are highly associated with impulsivity representing an usual phenotype involved in ADHD (358).
The most common eating disorders are anorexia nervosa (AN) and bulimia nervosa (BN). According to DSM-5, AN is characterized by distorted body image and excessive dieting leading to severe weight loss with a pathological fear of becoming fat, whereas BN is characterized by recurrent episodes of binge eating alternated with recurrent inappropriate compensatory behavior to prevent weight gain (63). Importantly, ECS plays a major regulatory role on feeding behaviors and energy balance (359) that has drawn attention to its relationship with ADHD neurobiology. This can be confirmed by rodents with diet-induced or genetic obesity promoting an increase of endocannabinoid hypothalamic levels (360).
The participation of CB1r in the regulation of feeding behavior is well established. The acute administration of rimonabant decreased food intake and body weight gain and reduced CB1r gene expression in the PVN of male Wistar rats. However, a chronic treatment led to tolerance to the hypophagic effects of CB1r blockade without changes in food intake, body weight, or hypothalamic mRNA gene expression (361). Another study on rimonabant-treated male Sprague-Dawley rats showed that the colocalized Fos labeling of hypothalamic regions with anorexigenic and orexigenic peptides had decreased neuropeptide Y (NPY) levels (362). More recently, another study was performed in CB1r conditional and CB2−/− mice. The hypothalamic neuropeptide expression pattern displayed a marked decrease of proopiomelanocortin (POMC) and cocaine-amphetamine-regulated transcript (CART) expression in the arcuate nucleus of the hypothalamus (ARC), both neuropeptides involved on anorexigenic and behavioral changes in food intake (363).
C57BL/6J mice were treated with naltrexone (opioid receptor antagonist), rimonabant, and BD-1063 (sigma-1 receptor antagonist) on an intermittent maladaptive feeding animal model. All the treatments reduced overconsumption of a palatable food (364). In addition, the administration of other cannabinoid compounds such as CBD or CB1r antagonists significantly reduced food intake and body weight gain (365–368). Recently, a study based on the activity-based anorexia (ABA) model reproducing key aspects of human AN, measured levels of AEA, 2-AG, and the CB1r in different brain regions of female ABA Sprague-Dawley rats. 2-AG significantly decreased in various brain areas but not in the caudate putamen, whereas no changes were observed in AEA. Density of CB1r was reduced in the dentate gyrus of Hipp and in the lateral Hyp (369). These results suggested that ECS is involved in the contribution and maintenance of some aspects in the pathophysiology of AN.
Although there is some progress in the understanding of the mechanisms underlying eating disorders and body weight regulation, there is still lack of information to suggest cannabinoid related treatment for patients with AN and BN. However, some studies suggest that the ECS, primarily CB1r, plays a key role in the reward areas and metabolic patterns involved in food intake and weight gain. In this regard, a PET study on 54 patients with food intake disorders (FID, including AN and BN) revealed an inverse association between regional CB1r availability and body mass index (BMI) in the Hyp and brainstem areas in both patients with FID and healthy individuals. However, FID patients negatively correlated with BMI throughout the mesolimbic reward system (370). Also, global CB1r availability is significantly increased in the cortical and subcortical brain areas in AN patients compared with healthy controls, maybe due to a compensatory mechanism of an underactive ECS in these patients (371). Finally, eating disorder female patients presented lower CB1r mRNA levels in PBMCs (372).
The close involvement of the ECS in the etiology and neuropathology of neuropsychiatric disorders is undeniable. Considering the urgent need to identify new and better biomarkers in psychiatry, the evidence included in this review provides an overview of the opportunities that cannabinoid receptors (Table 1), endogenous cannabinoid ligands (Table 2), or their metabolizing enzymes (Table 3) offer as potential biomarkers in the clinical setting. The large number of pharmacological studies with various cannabinoid compounds, mainly conducted in animal models, reported interesting and promising information to design new therapeutic strategies that, alone or in combination with the drugs currently used in psychiatry, may improve the efficacy and safety of psychiatric disorders treatment.
Table 1 Main findings from human studies supporting the role of CB1r and CB2r as biomarkers in psychiatric disorders.
Table 2 Main findings from human studies supporting the role of AEA and 2-AG as biomarkers in psychiatric disorders.
Table 3 Main findings from human studies supporting the role of FAAH as a biomarker in psychiatric disorders.
According to the information gathered in the present review from pharmacological and genetic approaches mainly performed in rodents, some general conclusions can be drawn regarding the usefulness of ECS components as therapeutic biomarkers (Figure 2). The blockade or genetic deletion of CB1r is closely associated with the worsening of emotional behavioral traits, as revealed principally in animal models of anxiety, depression or PTSD, whereas CB1r pharmacological activation induces an improvement effect. On the other hand, CB1r activation induces psychotic symptoms, while CB1r blockade presents antipsychotic effects. Regarding CB2r, its pharmacological activation or its overexpression by means of genetic manipulation or chronic treatment induced upregulation improving anxiety- and depressive-like behaviors, as well as schizophrenia-like traits. In contrast, all these behaviors worsen by CB2r blockade or gene deletion. Interestingly, the strengthening of the endocannabinoid tone, by the inhibition of enzymatic degradation or the blockade of reuptake mechanisms, is closely related with an improvement, particularly in emotional regulation as explored in animal models of anxiety, depression, and PTSD.
Figure 2 Main findings regarding the therapeutic potential of the functional manipulation of the endocannabinoid system (ECS) components by pharmacological and genetic approaches in anxiety, depression, schizophrenia, and post-traumatic stress disorder (PTSD). CB1r, cannabinoid receptor 1; CB2r, cannabinoid receptor 2; AEA, anandamide; 2-AG, 2-arachidonoylglycerol; FAAH, fatty acid amide hydrolase; MAGL, monoacylglycerol lipase; 5-HT, serotonin; NA, noradrenaline; DA, dopamine; AcH: acetylcholine; GABA, gamma-aminobutyric acid; Glu, glutamate; HPA axis, Hypothalamus–Pituitary–Adrenal axis; *, chronic treatment.
Therefore, the available evidence points out that the functional manipulation of the ECS components presents a great therapeutic potential. However, the close interaction of the ECS with other neurotransmitter or neurohormonal systems, as well as the specific and differential neuroanatomical distribution of the ECS components, provides a complex scenario not only from a therapeutic point of view but also considering the occurrence of side effects. In this sense, some aspects should be critically addressed, especially from a pharmacological perspective. The dosing, duration, and mechanism of action involved in the manipulation of the ECS are crucial to reach an improvement and limit adverse reactions. Apart from the widely explored role of CB1r, it should be noted that in recent years an increasing emphasis is being placed on the design of strategies to regulate endogenous cannabinoid tone, through inhibitors of the degradation or reuptake of eCBs. Since this approach provided negative results, particularly regarding the inhibition of FAAH (373), current trends focused on the combination of different mechanisms of action to enhance the endocannabinoid tone (374). Moreover, the pharmacological modulation of CB2r has also attracted much attention given its safety profile and the wide range of properties attributed to it, including mood and cognitive regulation. Thus, future priorities for both human and animal research would be the potentiation of both endocannabinoid tone and CB2r-mediated actions.
The trials carried out on patients show alterations of the ECS components at different levels, which in certain cases are related to risk or predictive factors regarding the evolution of the disease, or the degree of response to drug treatment. It is relevant to highlight the underlying sex-dependent effects in terms of sexual dimorphism of the ECS (375) and sex differences in prevalence rates and presentation of the psychiatric disorders (376). These could be involved not only in the changes of the ECS components to provide sex-related diagnostic or prognostic biomarkers, but also in the pharmacological actions derived from the treatment with cannabinoid compounds (377). Finally, it is important to note that more in vivo clinical studies are recently being carried out employing blood samples (PBMCs, plasma) or neuroimaging techniques (PET, fMRI) to identify ECS-related alterations, providing very relevant data. In this sense, a higher effort is required to design and perform more clinical studies, especially increasing the sample sizes to achieve greater significance, representativeness and reproducibility, finally making possible to identify some of the ECS components as useful biomarkers applicable to clinical practice in psychiatry.
FN and JM designed the sections and contents of the review manuscript. FN oversaw the organization to distribute the writing tasks among the authors and participated in manuscript writing. MG-G, RJ-B, GR, AG, and AA-O perform the literature searches and participated in the manuscript writing. All the authors critically reviewed and approved the final version of the manuscript.
The authors declare that the research was conducted in the absence of any commercial or financial relationships that could be construed as a potential conflict of interest.
The preparation of the manuscript was supported by ‘Instituto de Salud Carlos III, Fondos FEDER, Red de Trastornos Adictivos’ (RTA, RD16/0017/0014 to J.M. and RD16/0017/0017 to G.R.) and ‘Ministerio de Sanidad, Delegación del Gobierno para el Plan Nacional Sobre Drogas’ (PNSD, 2019/012 to J.M.).
ECS, endocannabinoid system; eCBs, endocannabinois; CB1r, cannabinoid receptor 1; CB2r, cannabinoid receptor 2; CSF, cerebrospinal fluid; PET, positron emission tomography; fMRI, functional magnetic resonance; BD, bipolar disorder; PTSD, posttraumatic stress disorder; ADHD, attention deficit hyperactivity disorder; YLDs, years lived with disability; DALYs, disability adjusted life-years; CNS, central nervous system; PFC, prefrontal cortex; NAc, nucleus accumbens; Hipp, hippocampus; Amy, amygdala; PVN, paraventricular nucleus; Hyp, hypothalamus; VTA, ventral tegmental area; AEA, anandamide; 2-AG, 2-arachidonoylglycerol; NAPE-PLD, N-acylphosphatidylethanolamine specific phospholipase D; FAAH, fatty acid amide hydrolase; GPCRs, Gq protein-coupled receptors; DAGL, diacylglycerol; MAGL, monoacylglycerol lipase; ABHD, alpha/beta-hydrolase domain; DSM-5, diagnostic and statistical manual of mental disorders, 5th version; TRPV1, transient receptor potential cation channel subfamily V member 1; dlPAG, dorsolateral periaqueductal gray matter; NO, nitric oxide; WT, wild-type; DMH, dorsomedial hypothalamus; BLA, basolateral amygdala; EPM, elevated plus maze; LHb, lateral habenula; LC, locus coeruleus; DRN, dorsal raphe nucleus; NA, noradrenaline; 5-HT, serotonin; HPA axis, hypothalamus–pituitary–adrenal axis; CRH, corticotropin-releasing hormone; CRHR1, corticotropin-releasing hormone type 1 receptor; 5HTT, serotonin transporter; HDAC, histone deacetylase; CUS, chronic unpredictable stress; CB2xP, transgenic mice overexpression CB2r in the brain; BCP, beta-caryophyllene; THC, tetrahydrocannabinol; MDD, major depressive disorder; BDNF, brain-derived neurotrophic factor; TST, tail suspension test; FST, forced swimming test; CMS, chronic mild stress; FSL, Flinders sensitive line rats; WKY, Wistar Kyoto rats; cKO, conditional knockout; SSRIs, serotonin selective reuptake inhibitors; 5HTT, serotonin transporter; rTMS, repeated transcranial magnetic stimulation; ECT, electroconvulsive therapy; ACC, anterior cingulate cortex; PPI, prepulse inhibition; NMDAr, N-methyl-D-aspartate receptors; MAM, methylazoxymethanol; SHR, spontaneously hypertensive rat; CNR1P1, cannabinoid receptor interacting protein; Nrg1, neuregulin 1; DLPFC, dorsolateral prefrontal cortex; STG, superior temporal gyrus; qRT-PCR, quantitative real time polymerase chain reaction; FEP, first episode psychosis; PBMCs, peripheral blood mononuclear cells; PANSS, positive and negative syndrome scale; SUD, substance use disorder; CBD, cannabidiol; ACTH, adrenocorticotropin hormone; CS, corticosterone; SPS, single prolonged stress; DLS, dorsolateral striatum; PEA, palmitoylethanolamide; OEA, oleoylethanolamide; SEA, steraoylethanolamide; AN, anorexia nervosa; BN, bulimia nervosa; NPY, neuropeptide Y; POMC, proopiomelanocortin; CART, cocaine-amphetamine-regulated transcript; ARC, arcuate nucleus; FID, food intake disorders; BMI, body mass index.
1. G.B.D. Disease, I. Injury, and C. Prevalence. Global, regional, and national incidence, prevalence, and years lived with disability for 354 diseases and injuries for 195 countries and territories, 1990-2017: a systematic analysis for the Global Burden of Disease Study 2017. Lancet (2018) 392:1789–858. doi: 10.1016/S0140-6736(18)32279-7
2. Vigo D, Thornicroft G, Atun R. Estimating the true global burden of mental illness. Lancet Psychiatry (2016) 3:171–8. doi: 10.1016/S2215-0366(15)00505-2
3. Krystal JH, State MW. Psychiatric disorders: diagnosis to therapy. Cell (2014) 157:201–14. doi: 10.1016/j.cell.2014.02.042
4. Scarr E, Millan MJ, Bahn S, Bertolino A, Turck CW, Kapur S, et al. Biomarkers for Psychiatry: The Journey from Fantasy to Fact, a Report of the 2013 CINP Think Tank. Int J Neuropsychopharmacol (2015) 18:pyv042. doi: 10.1093/ijnp/pyv042
5. Venkatasubramanian G, Keshavan MS. Biomarkers in Psychiatry - A Critique. Ann Neurosci (2016) 23:3–5. doi: 10.1159/000443549
6. Mechoulam R, Parker LA. The endocannabinoid system and the brain. Annu Rev Psychol (2013) 64:21–47. doi: 10.1146/annurev-psych-113011-143739
7. Ashton JC, Dowie MJ, Glass M. The endocannabinoid system and human brain functions: insight from memory, motor, and mood pathologies, The endocananbinoid system: genetics, biochemistry, brain disorders, and therapy. Cambridge (Massachusetts), USA (2017) p. 115–86.
8. Manzanares J, Uriguen L, Rubio G, Palomo T. Role of endocannabinoid system in mental diseases. Neurotox Res (2004) 6:213–24. doi: 10.1007/BF03033223
9. Marco EM, Garcia-Gutierrez MS, Bermudez-Silva FJ, Moreira FA, Guimaraes F, Manzanares J, et al. Endocannabinoid system and psychiatry: in search of a neurobiological basis for detrimental and potential therapeutic effects. Front Behav Neurosci (2011) 5:63. doi: 10.3389/fnbeh.2011.00063
10. Parolaro D, Realini N, Vigano D, Guidali C, Rubino T. The endocannabinoid system and psychiatric disorders. Exp Neurol (2010) 224:3–14. doi: 10.1016/j.expneurol.2010.03.018
11. Katzman MA, Furtado M, Anand L. Targeting the Endocannabinoid System in Psychiatric Illness. J Clin Psychopharmacol (2016) 36:691–703. doi: 10.1097/JCP.0000000000000581
12. Ritchie H, Roser M. (2020). Published online at OurWorldInData.org. Retrieved from: https://ourworldindata.org/mental-health Online Resource.
13. Bromet E, Karam E, Koenen K, Stein D. The Global Epidemiology of Trauma Exposure and Posttraumatic Stress Disorder. Cambridge: Cambridge University Press (2018).
14. Fayyad J, Sampson NA, Hwang I, Adamowski T, Aguilar-Gaxiola S, Al-Hamzawi A, et al. The descriptive epidemiology of DSM-IV Adult ADHD in the World Health Organization World Mental Health Surveys. Atten Defic Hyperact Disord (2017) 9:47–65. doi: 10.1007/s12402-016-0208-3
15. Katona I, Freund TF. Multiple functions of endocannabinoid signaling in the brain. Annu Rev Neurosci (2012) 35:529–58. doi: 10.1146/annurev-neuro-062111-150420
16. Atkinson DL, Abbott JK. Cannabinoids and the brain: the effects of endogenous and exogenous cannabinoids on brain systems and function, The complex connection between cannabis and schizophrenia. Cambridge (Massachusetts), USA (2018) p. 37–74.
17. Piomelli D. The molecular logic of endocannabinoid signalling. Nat Rev Neurosci (2003) 4:873–84. doi: 10.1038/nrn1247
18. Zou S, Kumar U. Cannabinoid Receptors and the Endocannabinoid System: Signaling and Function in the Central Nervous System. Int J Mol Sci (2018) 19: 833. doi: 10.3390/ijms19030833
19. Mackie K. Distribution of cannabinoid receptors in the central and peripheral nervous system. Handb Exp Pharmacol (2005), 168: 299–325. doi: 10.1007/3-540-26573-2_10
20. Rodriguez de Fonseca F, Del Arco I, Bermudez-Silva FJ, Bilbao A, Cippitelli A, Navarro M. The endocannabinoid system: physiology and pharmacology. Alcohol Alcohol (2005) 40:2–14. doi: 10.1093/alcalc/agh110
21. Tsou K, Brown S, Sanudo-Pena MC, Mackie K, Walker JM. Immunohistochemical distribution of cannabinoid CB1 receptors in the rat central nervous system. Neuroscience (1998) 83:393–411. doi: 10.1016/s0306-4522(97)00436-3
22. Matsuda LA, Lolait SJ, Brownstein MJ, Young AC, Bonner TI. Structure of a cannabinoid receptor and functional expression of the cloned cDNA. Nature (1990) 346:561–4. doi: 10.1038/346561a0
23. Gutierrez-Rodriguez A, Puente N, Elezgarai I, Ruehle S, Lutz B, Reguero L, et al. Anatomical characterization of the cannabinoid CB1 receptor in cell-type-specific mutant mouse rescue models. J Comp Neurol (2017) 525:302–18. doi: 10.1002/cne.24066
24. Piazza PV, Cota D, Marsicano G. The CB1 Receptor as the Cornerstone of Exostasis. Neuron (2017) 93:1252–74. doi: 10.1016/j.neuron.2017.02.002
25. Busquets-Garcia A, Bains J, Marsicano G. CB1 Receptor Signaling in the Brain: Extracting Specificity from Ubiquity. Neuropsychopharmacology (2018) 43:4–20. doi: 10.1038/npp.2017.206
26. Howlett AC, Bidaut-Russell M, Devane WA, Melvin LS, Johnson MR, Herkenham M. The cannabinoid receptor: biochemical, anatomical and behavioral characterization. Trends Neurosci (1990) 13:420–3. doi: 10.1016/0166-2236(90)90124-S
27. Hu SS, Mackie K. Distribution of the Endocannabinoid System in the Central Nervous System. Handb Exp Pharmacol (2015) 231:59–93. doi: 10.1007/978-3-319-20825-1_3
28. Batkai S, Jarai Z, Wagner JA, Goparaju SK, Varga K, Liu J, et al. Endocannabinoids acting at vascular CB1 receptors mediate the vasodilated state in advanced liver cirrhosis. Nat Med (2001) 7:827–32. doi: 10.1038/89953
29. Wagner JA, Varga K, Ellis EF, Rzigalinski BA, Martin BR, Kunos G. Activation of peripheral CB1 cannabinoid receptors in haemorrhagic shock. Nature (1997) 390:518–21. doi: 10.1038/37371
30. Pertwee RG. Pharmacology of cannabinoid CB1 and CB2 receptors. Pharmacol Ther (1997) 74:129–80. doi: 10.1016/S0163-7258(97)82001-3
31. Pertwee RG, Ross RA. Cannabinoid receptors and their ligands. Prostaglandins Leukot Essent Fatty Acids (2002) 66:101–21. doi: 10.1054/plef.2001.0341
32. Galiegue S, Mary S, Marchand J, Dussossoy D, Carriere D, Carayon P, et al. Expression of central and peripheral cannabinoid receptors in human immune tissues and leukocyte subpopulations. Eur J Biochem (1995) 232:54–61. doi: 10.1111/j.1432-1033.1995.tb20780.x
33. Guindon J, Hohmann AG. The endocannabinoid system and pain. CNS Neurol Disord Drug Targets (2009) 8:403–21. doi: 10.2174/187152709789824660
34. Munro S, Thomas KL, Abu-Shaar M. Molecular characterization of a peripheral receptor for cannabinoids. Nature (1993) 365:61–5. doi: 10.1038/365061a0
35. Cabral GA, Griffin-Thomas L. Emerging role of the cannabinoid receptor CB2 in immune regulation: therapeutic prospects for neuroinflammation. Expert Rev Mol Med (2009) 11:e3. doi: 10.1017/S1462399409000957
36. Benito C, Nunez E, Tolon RM, Carrier EJ, Rabano A, Hillard CJ, et al. Cannabinoid CB2 receptors and fatty acid amide hydrolase are selectively overexpressed in neuritic plaque-associated glia in Alzheimer's disease brains. J Neurosci (2003) 23:11136–41. doi: 10.1523/JNEUROSCI.23-35-11136.2003
37. Yiangou Y, Facer P, Durrenberger P, Chessell IP, Naylor A, Bountra C, et al. COX-2, CB2 and P2X7-immunoreactivities are increased in activated microglial cells/macrophages of multiple sclerosis and amyotrophic lateral sclerosis spinal cord. BMC Neurol (2006) 6:12. doi: 10.1186/1471-2377-6-12
38. Guzman M, Sanchez C, Galve-Roperh I. Control of the cell survival/death decision by cannabinoids. J Mol Med (Berl) (2001) 78:613–25. doi: 10.1007/s001090000177
39. Van Sickle MD, Duncan M, Kingsley PJ, Mouihate A, Urbani P, Mackie K, et al. Identification and functional characterization of brainstem cannabinoid CB2 receptors. Science (2005) 310:329–32. doi: 10.1126/science.1115740
40. Gong JP, Onaivi ES, Ishiguro H, Liu QR, Tagliaferro PA, Brusco A, et al. Cannabinoid CB2 receptors: immunohistochemical localization in rat brain. Brain Res (2006) 1071:10–23. doi: 10.1016/j.brainres.2005.11.035
41. Onaivi ES. Neuropsychobiological evidence for the functional presence and expression of cannabinoid CB2 receptors in the brain. Neuropsychobiology (2006) 54:231–46. doi: 10.1159/000100778
42. Onaivi ES, Ishiguro H, Gong JP, Patel S, Perchuk A, Meozzi PA, et al. Discovery of the presence and functional expression of cannabinoid CB2 receptors in brain. Ann N Y Acad Sci (2006) 1074:514–36. doi: 10.1196/annals.1369.052
43. Garcia-Gutierrez MS, Perez-Ortiz JM, Gutierrez-Adan A, Manzanares J. Depression-resistant endophenotype in mice overexpressing cannabinoid CB(2) receptors. Br J Pharmacol (2010) 160:1773–84. doi: 10.1111/j.1476-5381.2010.00819.x
44. Zhang HY, Gao M, Liu QR, Bi GH, Li X, Yang HJ, et al. Cannabinoid CB2 receptors modulate midbrain dopamine neuronal activity and dopamine-related behavior in mice. Proc Natl Acad Sci U S A (2014) 111:E5007–15. doi: 10.1073/pnas.1413210111
45. Cabral GA, Raborn ES, Griffin L, Dennis J, Marciano-Cabral F. CB2 receptors in the brain: role in central immune function. Br J Pharmacol (2008) 153:240–51. doi: 10.1038/sj.bjp.0707584
46. Garcia-Gutierrez MS, Navarrete F, Navarro G, Reyes-Resina I, Franco R, Lanciego JL, et al. Alterations in Gene and Protein Expression of Cannabinoid CB2 and GPR55 Receptors in the Dorsolateral Prefrontal Cortex of Suicide Victims. Neurotherapeutics (2018) 15:796–806. doi: 10.1007/s13311-018-0610-y
47. Liu QR, Canseco-Alba A, Zhang HY, Tagliaferro P, Chung M, Dennis E, et al. Cannabinoid type 2 receptors in dopamine neurons inhibits psychomotor behaviors, alters anxiety, depression and alcohol preference. Sci Rep (2017) 7:17410. doi: 10.1038/s41598-017-17796-y
48. Pertwee RG, Howlett AC, Abood ME, Alexander SP, Di Marzo V, Elphick MR, et al. Cannabinoid receptors and their ligands: beyond CB(1) and CB(2). Pharmacol Rev (2010) 62:588–631. doi: 10.1124/pr.110.003004
49. Kano M, Ohno-Shosaku T, Hashimotodani Y, Uchigashima M, Watanabe M. Endocannabinoid-mediated control of synaptic transmission. Physiol Rev (2009) 89:309–80. doi: 10.1152/physrev.00019.2008
50. Alger BE, Kim J. Supply and demand for endocannabinoids. Trends Neurosci (2011) 34:304–15. doi: 10.1016/j.tins.2011.03.003
51. Devane WA, Hanus L, Breuer A, Pertwee RG, Stevenson LA, Griffin G, et al. Isolation and structure of a brain constituent that binds to the cannabinoid receptor. Science (1992) 258:1946–9. doi: 10.1126/science.1470919
52. Mechoulam R, Ben-Shabat S, Hanus L, Ligumsky M, Kaminski NE, Schatz AR, et al. Identification of an endogenous 2-monoglyceride, present in canine gut, that binds to cannabinoid receptors. Biochem Pharmacol (1995) 50:83–90. doi: 10.1016/0006-2952(95)00109-D
53. Okamoto Y, Morishita J, Tsuboi K, Tonai T, Ueda N. Molecular characterization of a phospholipase D generating anandamide and its congeners. J Biol Chem (2004) 279:5298–305. doi: 10.1074/jbc.M306642200
54. Di Marzo V, Stella N, Zimmer A. Endocannabinoid signalling and the deteriorating brain. Nat Rev Neurosci (2015) 16:30–42. doi: 10.1038/nrn3876
55. Ueda N. Endocannabinoid hydrolases. Prostaglandins Other Lipid Mediat (2002) 68-69:521–34. doi: 10.1016/S0090-6980(02)00053-9
56. Egertova M, Cravatt BF, Elphick MR. Comparative analysis of fatty acid amide hydrolase and cb(1) cannabinoid receptor expression in the mouse brain: evidence of a widespread role for fatty acid amide hydrolase in regulation of endocannabinoid signaling. Neuroscience (2003) 119:481–96. doi: 10.1016/S0306-4522(03)00145-3
57. Fezza F, Bari M, Florio R, Talamonti E, Feole M, Maccarrone M. Endocannabinoids, related compounds and their metabolic routes. Molecules (2014) 19:17078–106. doi: 10.3390/molecules191117078
58. Gao Y, Vasilyev DV, Goncalves MB, Howell FV, Hobbs C, Reisenberg M, et al. Loss of retrograde endocannabinoid signaling and reduced adult neurogenesis in diacylglycerol lipase knock-out mice. J Neurosci (2010) 30:2017–24. doi: 10.1523/JNEUROSCI.5693-09.2010
59. Tanimura A, Yamazaki M, Hashimotodani Y, Uchigashima M, Kawata S, Abe M, et al. The endocannabinoid 2-arachidonoylglycerol produced by diacylglycerol lipase alpha mediates retrograde suppression of synaptic transmission. Neuron (2010) 65:320–7. doi: 10.1016/j.neuron.2010.01.021
60. Dinh TP, Carpenter D, Leslie FM, Freund TF, Katona I, Sensi SL, et al. Brain monoglyceride lipase participating in endocannabinoid inactivation. Proc Natl Acad Sci U S A (2002) 99:10819–24. doi: 10.1073/pnas.152334899
61. Marsicano G, Lutz B. Neuromodulatory functions of the endocannabinoid system. J Endocrinol Invest (2006) 29:27–46.
62. Ibarra-Lecue I, Pilar-Cuellar F, Muguruza C, Florensa-Zanuy E, Diaz A, Uriguen L, et al. The endocannabinoid system in mental disorders: Evidence from human brain studies. Biochem Pharmacol American Psychiatric Association (2018) 157:97–107. doi: 10.1016/j.bcp.2018.07.009
63. American Psychiatric Association. (2013). Diagnostic and Statistical Manual of Mental Disorders (DSM), 5th edition.
64. Marco EM, Echeverry-Alzate V, Lopez-Moreno JA, Gine E, Penasco S, Viveros MP. Consequences of early life stress on the expression of endocannabinoid-related genes in the rat brain. Behav Pharmacol (2014) 25:547–56. doi: 10.1097/FBP.0000000000000068
65. Boero G, Pisu MG, Biggio F, Muredda L, Carta G, Banni S, et al. Impaired glucocorticoid-mediated HPA axis negative feedback induced by juvenile social isolation in male rats. Neuropharmacology (2018) 133:242–53. doi: 10.1016/j.neuropharm.2018.01.045
66. Sutt S, Raud S, Areda T, Reimets A, Koks S, Vasar E. Cat odour-induced anxiety–a study of the involvement of the endocannabinoid system. Psychopharmacol (Berl) (2008) 198:509–20. doi: 10.1007/s00213-007-0927-4
67. Cagni P, Barros M. Cannabinoid type 1 receptor ligands WIN 55,212-2 and AM 251 alter anxiety-like behaviors of marmoset monkeys in an open-field test. Behav Brain Res (2013) 240:91–4. doi: 10.1016/j.bbr.2012.11.018
68. Morena M, Patel S, Bains JS, Hill MN. Neurobiological Interactions Between Stress and the Endocannabinoid System. Neuropsychopharmacology (2016) 41:80–102. doi: 10.1038/npp.2015.166
69. Lutz B, Marsicano G, Maldonado R, Hillard CJ. The endocannabinoid system in guarding against fear, anxiety and stress. Nat Rev Neurosci (2015) 16:705–18. doi: 10.1038/nrn4036
70. Haller J, Bakos N, Szirmay M, Ledent C, Freund TF. The effects of genetic and pharmacological blockade of the CB1 cannabinoid receptor on anxiety. Eur J Neurosci (2002) 16:1395–8. doi: 10.1046/j.1460-9568.2002.02192.x
71. Haller J, Varga B, Ledent C, Freund TF. CB1 cannabinoid receptors mediate anxiolytic effects: convergent genetic and pharmacological evidence with CB1-specific agents. Behav Pharmacol (2004) 15:299–304. doi: 10.1097/01.fbp.0000135704.56422.40
72. Haller J, Varga B, Ledent C, Barna I, Freund TF. Context-dependent effects of CB1 cannabinoid gene disruption on anxiety-like and social behaviour in mice. Eur J Neurosci (2004) 19:1906–12. doi: 10.1111/j.1460-9568.2004.03293.x
73. Witkin JM, Tzavara ET, Nomikos GG. A role for cannabinoid CB1 receptors in mood and anxiety disorders. Behav Pharmacol (2005) 16:315–31. doi: 10.1097/00008877-200509000-00005
74. Valverde O. Participation of the cannabinoid system in the regulation of emotional-like behaviour. Curr Pharm Des (2005) 11:3421–9. doi: 10.2174/138161205774370780
75. Garcia-Gutierrez MS, Manzanares J. The cannabinoid CB1 receptor is involved in the anxiolytic, sedative and amnesic actions of benzodiazepines. J Psychopharmacol (2010) 24:757–65. doi: 10.1177/0269881109106910
76. Batista LA, Moreira FA. Cannabinoid CB1 receptors mediate the anxiolytic effects induced by systemic alprazolam and intra-periaqueductal gray 5-HT1A receptor activation. Neurosci Lett (2019) 703:5–10. doi: 10.1016/j.neulet.2019.03.010
77. Naderi N, Haghparast A, Saber-Tehrani A, Rezaii N, Alizadeh AM, Khani A, et al. Interaction between cannabinoid compounds and diazepam on anxiety-like behaviour of mice. Pharmacol Biochem Behav (2008) 89:64–75. doi: 10.1016/j.pbb.2007.11.001
78. Viveros MP, Marco EM, File SE. Endocannabinoid system and stress and anxiety responses. Pharmacol Biochem Behav (2005) 81:331–42. doi: 10.1016/j.pbb.2005.01.029
79. Komaki A, Hashemi-Firouzi N, Shojaei S, Souri Z, Heidari S, Shahidi S. Study the Effect of Endocannabinoid System on Rat Behavior in Elevated Plus-Maze. Basic Clin Neurosci (2015) 6:147–53.
80. Lisboa SF, Niraula A, Resstel LB, Guimaraes FS, Godbout JP, Sheridan JF. Repeated social defeat-induced neuroinflammation, anxiety-like behavior and resistance to fear extinction were attenuated by the cannabinoid receptor agonist WIN55,212-2. Neuropsychopharmacology (2018) 43:1924–33. doi: 10.1038/s41386-018-0064-2
81. Arevalo C, de Miguel R, Hernandez-Tristan R. Cannabinoid effects on anxiety-related behaviours and hypothalamic neurotransmitters. Pharmacol Biochem Behav (2001) 70:123–31. doi: 10.1016/S0091-3057(01)00578-0
82. Navarro M, Hernandez E, Munoz RM, del Arco I, Villanua MA, Carrera MR, et al. Acute administration of the CB1 cannabinoid receptor antagonist SR 141716A induces anxiety-like responses in the rat. Neuroreport (1997) 8:491–6. doi: 10.1097/00001756-199701200-00023
83. Degroot A, Nomikos GG. Genetic deletion and pharmacological blockade of CB1 receptors modulates anxiety in the shock-probe burying test. Eur J Neurosci (2004) 20:1059–64. doi: 10.1111/j.1460-9568.2004.03556.x
84. Genn RF, Tucci S, Marco EM, Viveros MP, File SE. Unconditioned and conditioned anxiogenic effects of the cannabinoid receptor agonist CP 55,940 in the social interaction test. Pharmacol Biochem Behav (2004) 77:567–73. doi: 10.1016/j.pbb.2003.12.019
85. Hill MN, Gorzalka BB. Enhancement of anxiety-like responsiveness to the cannabinoid CB(1) receptor agonist HU-210 following chronic stress. Eur J Pharmacol (2004) 499:291–5. doi: 10.1016/j.ejphar.2004.06.069
86. Rodgers RJ, Evans PM, Murphy A. Anxiogenic profile of AM-251, a selective cannabinoid CB1 receptor antagonist, in plus-maze-naive and plus-maze-experienced mice. Behav Pharmacol (2005) 16:405–13. doi: 10.1097/00008877-200509000-00013
87. Sink KS, Segovia KN, Sink J, Randall PA, Collins LE, Correa M, et al. Potential anxiogenic effects of cannabinoid CB1 receptor antagonists/inverse agonists in rats: comparisons between AM4113, AM251, and the benzodiazepine inverse agonist FG-7142. Eur Neuropsychopharmacol (2010) 20:112–22. doi: 10.1016/j.euroneuro.2009.11.002
88. Rey AA, Purrio M, Viveros MP, Lutz B. Biphasic effects of cannabinoids in anxiety responses: CB1 and GABA(B) receptors in the balance of GABAergic and glutamatergic neurotransmission. Neuropsychopharmacology (2012) 37:2624–34. doi: 10.1038/npp.2012.123
89. Faraji N, Komaki A, Salehi I. Interaction Between the Cannabinoid and Vanilloid Systems on Anxiety in Male Rats. Basic Clin Neurosci (2017) 8:129–37. doi: 10.18869/nirp.bcn.8.2.129
90. Campos AC, Guimaraes FS. Evidence for a potential role for TRPV1 receptors in the dorsolateral periaqueductal gray in the attenuation of the anxiolytic effects of cannabinoids. Prog Neuropsychopharmacol Biol Psychiatry (2009) 33:1517–21. doi: 10.1016/j.pnpbp.2009.08.017
91. Fogaca MV, Aguiar DC, Moreira FA, Guimaraes FS. The endocannabinoid and endovanilloid systems interact in the rat prelimbic medial prefrontal cortex to control anxiety-like behavior. Neuropharmacology (2012) 63:202–10. doi: 10.1016/j.neuropharm.2012.03.007
92. Batista PA, Fogaca MV, Guimaraes FS. The endocannabinoid, endovanilloid and nitrergic systems could interact in the rat dorsolateral periaqueductal gray matter to control anxiety-like behaviors. Behav Brain Res (2015) 293:182–8. doi: 10.1016/j.bbr.2015.07.019
93. Litvin Y, Phan A, Hill MN, Pfaff DW, McEwen BS. CB1 receptor signaling regulates social anxiety and memory. Genes Brain Behav (2013) 12:479–89. doi: 10.1111/gbb.12045
94. Martin M, Ledent C, Parmentier M, Maldonado R, Valverde O. Involvement of CB1 cannabinoid receptors in emotional behaviour. Psychopharmacol (Berl) (2002) 159:379–87. doi: 10.1007/s00213-001-0946-5
95. Thiemann G, Watt CA, Ledent C, Molleman A, Hasenohrl RU. Modulation of anxiety by acute blockade and genetic deletion of the CB(1) cannabinoid receptor in mice together with biogenic amine changes in the forebrain. Behav Brain Res (2009) 200:60–7. doi: 10.1016/j.bbr.2008.12.035
96. Maccarrone M, Valverde O, Barbaccia ML, Castane A, Maldonado R, Ledent C, et al. Age-related changes of anandamide metabolism in CB1 cannabinoid receptor knockout mice: correlation with behaviour. Eur J Neurosci (2002) 15:1178–86. doi: 10.1046/j.1460-9568.2002.01957.x
97. Bowers ME, Ressler KJ. Sex-dependence of anxiety-like behavior in cannabinoid receptor 1 (Cnr1) knockout mice. Behav Brain Res (2016) 300:65–9. doi: 10.1016/j.bbr.2015.12.005
98. Simone JJ, Baumbach JL, McCormick CM. Sex-specific effects of CB1 receptor antagonism and stress in adolescence on anxiety, corticosterone concentrations, and contextual fear in adulthood in rats. Int J Dev Neurosci (2018) 69:119–31. doi: 10.1016/j.ijdevneu.2018.07.011
99. Uriguen L, Perez-Rial S, Ledent C, Palomo T, Manzanares J. Impaired action of anxiolytic drugs in mice deficient in cannabinoid CB1 receptors. Neuropharmacology (2004) 46:966–73. doi: 10.1016/j.neuropharm.2004.01.003
100. Uriguen L, Garcia-Gutierrez MS, Manzanares J. Decreased GABAA and GABAB receptor functional activity in cannabinoid CB1 receptor knockout mice. J Psychopharmacol (2011) 25:105–10. doi: 10.1177/0269881109358204
101. Moreira FA, Aguiar DC, Guimaraes FS. Anxiolytic-like effect of cannabinoids injected into the rat dorsolateral periaqueductal gray. Neuropharmacology (2007) 52:958–65. doi: 10.1016/j.neuropharm.2006.10.013
102. Batista LA, Bastos JR, Moreira FA. Role of endocannabinoid signalling in the dorsolateral periaqueductal grey in the modulation of distinct panic-like responses. J Psychopharmacol (2015) 29:335–43. doi: 10.1177/0269881114566259
103. Viana TG, Hott SC, Resstel LB, Aguiar DC, Moreira FA. Anti-aversive role of the endocannabinoid system in the periaqueductal gray stimulation model of panic attacks in rats. Psychopharmacol (Berl) (2015) 232:1545–53. doi: 10.1007/s00213-014-3793-x
104. Lisboa SF, Resstel LB, Aguiar DC, Guimaraes FS. Activation of cannabinoid CB1 receptors in the dorsolateral periaqueductal gray induces anxiolytic effects in rats submitted to the Vogel conflict test. Eur J Pharmacol (2008) 593:73–8. doi: 10.1016/j.ejphar.2008.07.032
105. Viana TG, Bastos JR, Costa RB, Hott SC, Mansur FS, Coimbra CC, et al. Hypothalamic endocannabinoid signalling modulates aversive responses related to panic attacks. Neuropharmacology (2019) 148:284–90. doi: 10.1016/j.neuropharm.2019.01.022
106. Di S, Itoga CA, Fisher MO, Solomonow J, Roltsch EA, Gilpin NW, et al. Acute Stress Suppresses Synaptic Inhibition and Increases Anxiety via Endocannabinoid Release in the Basolateral Amygdala. J Neurosci (2016) 36:8461–70. doi: 10.1523/JNEUROSCI.2279-15.2016
107. Morena M, Leitl KD, Vecchiarelli HA, Gray JM, Campolongo P, Hill MN. Emotional arousal state influences the ability of amygdalar endocannabinoid signaling to modulate anxiety. Neuropharmacology (2016) 111:59–69. doi: 10.1016/j.neuropharm.2016.08.020
108. Berger AL, Henricks AM, Lugo JM, Wright HR, Warrick CR, Sticht MA, et al. The Lateral Habenula Directs Coping Styles Under Conditions of Stress via Recruitment of the Endocannabinoid System. Biol Psychiatry (2018) 84:611–23. doi: 10.1016/j.biopsych.2018.04.018
109. Hajizadeh Moghaddam A, Bigdellu R, Fatemi Tabatabaei SR, Roohbakhsh A. Cannabinoid system of the lateral septum in the modulation of anxiety-like behaviors in rats. Arch Iran Med (2013) 16:711–6. doi: 0131612/AIM.006
110. Rubino T, Sala M, Vigano D, Braida D, Castiglioni C, Limonta V, et al. Cellular mechanisms underlying the anxiolytic effect of low doses of peripheral Delta9-tetrahydrocannabinol in rats. Neuropsychopharmacology (2007) 32:2036–45. doi: 10.1038/sj.npp.1301330
111. Rubino T, Realini N, Castiglioni C, Guidali C, Vigano D, Marras E, et al. Role in anxiety behavior of the endocannabinoid system in the prefrontal cortex. Cereb Cortex (2008) 18:1292–301. doi: 10.1016/j.neuropharm.2007.06.024
112. Hartmann A, Fassini A, Scopinho A, Correa FM, Guimaraes FS, Lisboa SF, et al. Role of the endocannabinoid system in the dorsal hippocampus in the cardiovascular changes and delayed anxiety-like effect induced by acute restraint stress in rats. J Psychopharmacol (2019) 33:606–14. doi: 10.1177/0269881119827799
113. Kochenborger L, Levone BR, da Silva ES, Taschetto AP, Terenzi MG, Paschoalini MA, et al. The microinjection of a cannabinoid agonist into the accumbens shell induces anxiogenesis in the elevated plus-maze. Pharmacol Biochem Behav (2014) 124:160–6. doi: 10.1016/j.pbb.2014.05.017
114. Lisboa SF, Borges AA, Nejo P, Fassini A, Guimaraes FS, Resstel LB. Cannabinoid CB1 receptors in the dorsal hippocampus and prelimbic medial prefrontal cortex modulate anxiety-like behavior in rats: additional evidence. Prog Neuropsychopharmacol Biol Psychiatry (2015) 59:76–83. doi: 10.1016/j.pnpbp.2015.01.005
115. Remmers F, Lange MD, Hamann M, Ruehle S, Pape HC, Lutz B. Addressing sufficiency of the CB1 receptor for endocannabinoid-mediated functions through conditional genetic rescue in forebrain GABAergic neurons. Brain Struct Funct (2017) 222:3431–52. doi: 10.1007/s00429-017-1411-5
116. Rubino T, Guidali C, Vigano D, Realini N, Valenti M, Massi P, et al. CB1 receptor stimulation in specific brain areas differently modulate anxiety-related behaviour. Neuropharmacology (2008) 54:151–60. doi: 10.1016/j.neuropharm.2007.06.024
117. Mendiguren A, Aostri E, Pineda J. Regulation of noradrenergic and serotonergic systems by cannabinoids: relevance to cannabinoid-induced effects. Life Sci (2018) 192:115–27. doi: 10.1016/j.lfs.2017.11.029
118. Haring M, Enk V, Aparisi Rey A, Loch S, Ruiz de Azua I, Weber T, et al. Cannabinoid type-1 receptor signaling in central serotonergic neurons regulates anxiety-like behavior and sociability. Front Behav Neurosci (2015) 9:235. doi: 10.3389/fnbeh.2015.00235
119. Zarrindast MR, Mahboobi S, Sadat-Shirazi MS, Ahmadi S. Anxiolytic-like effect induced by the cannabinoid CB1 receptor agonist, arachydonilcyclopropylamide (ACPA), in the rat amygdala is mediated through the D1 and D2 dopaminergic systems. J Psychopharmacol (2011) 25:131–40. doi: 10.1177/0269881110376688
120. Zarrindast MR, Sarahroodi S, Arzi A, Khodayar MJ, Taheri-Shalmani S, Rezayof A. Cannabinoid CB1 receptors of the rat central amygdala mediate anxiety-like behavior: interaction with the opioid system. Behav Pharmacol (2008) 19:716–23. doi: 10.1097/FBP.0b013e3283123c83
121. Lee TT, Gorzalka BB. Evidence for a Role of Adolescent Endocannabinoid Signaling in Regulating HPA Axis Stress Responsivity and Emotional Behavior Development. Int Rev Neurobiol (2015) 125:49–84. doi: 10.1016/bs.irn.2015.09.002
122. Gray JM, Vecchiarelli HA, Morena M, Lee TT, Hermanson DJ, Kim AB, et al. Corticotropin-releasing hormone drives anandamide hydrolysis in the amygdala to promote anxiety. J Neurosci (2015) 35:3879–92. doi: 10.1523/JNEUROSCI.2737-14.2015
123. Gray JM, Wilson CD, Lee TT, Pittman QJ, Deussing JM, Hillard CJ, et al. Sustained glucocorticoid exposure recruits cortico-limbic CRH signaling to modulate endocannabinoid function. Psychoneuroendocrinology (2016) 66:151–8. doi: 10.1016/j.psyneuen.2016.01.004
124. Lazary J, Lazary A, Gonda X, Benko A, Molnar E, Hunyady L, et al. Promoter variants of the cannabinoid receptor 1 gene (CNR1) in interaction with 5-HTTLPR affect the anxious phenotype. Am J Med Genet B Neuropsychiatr Genet (2009) 150B:1118–27. doi: 10.1002/ajmg.b.31024
125. Hay EA, McEwan A, Wilson D, Barrett P, D'Agostino G, Pertwee RG, et al. Disruption of an enhancer associated with addictive behaviour within the cannabinoid receptor-1 gene suggests a possible role in alcohol intake, cannabinoid response and anxiety-related behaviour. Psychoneuroendocrinology (2019) 109:104407. doi: 10.1016/j.psyneuen.2019.104407
126. Hayase T. Putative Epigenetic Involvement of the Endocannabinoid System in Anxiety- and Depression-Related Behaviors Caused by Nicotine as a Stressor. PloS One (2016) 11:e0158950. doi: 10.1371/journal.pone.0158950
127. Lomazzo E, Konig F, Abassi L, Jelinek R, Lutz B. Chronic stress leads to epigenetic dysregulation in the neuropeptide-Y and cannabinoid CB1 receptor genes in the mouse cingulate cortex. Neuropharmacology (2017) 113:301–13. doi: 10.1016/j.neuropharm.2016.10.008
128. Bedse G, Hartley ND, Neale E, Gaulden AD, Patrick TA, Kingsley PJ, et al. Functional Redundancy Between Canonical Endocannabinoid Signaling Systems in the Modulation of Anxiety. Biol Psychiatry (2017) 82:488–99. doi: 10.1016/j.biopsych.2017.03.002
129. Bedse G, Bluett RJ, Patrick TA, Romness NK, Gaulden AD, Kingsley PJ, et al. Therapeutic endocannabinoid augmentation for mood and anxiety disorders: comparative profiling of FAAH, MAGL and dual inhibitors. Transl Psychiatry (2018) 8:92. doi: 10.1038/s41398-018-0141-7
130. Chicca A, Nicolussi S, Bartholomaus R, Blunder M, Aparisi Rey A, Petrucci V, et al. Chemical probes to potently and selectively inhibit endocannabinoid cellular reuptake. Proc Natl Acad Sci U S A (2017) 114:E5006–15. doi: 10.1073/pnas.1704065114
131. Kinsey SG, O'Neal ST, Long JZ, Cravatt BF, Lichtman AH. Inhibition of endocannabinoid catabolic enzymes elicits anxiolytic-like effects in the marble burying assay. Pharmacol Biochem Behav (2011) 98:21–7. doi: 10.1016/j.pbb.2010.12.002
132. Busquets-Garcia A, Puighermanal E, Pastor A, de la Torre R, Maldonado R, Ozaita A. Differential role of anandamide and 2-arachidonoylglycerol in memory and anxiety-like responses. Biol Psychiatry (2011) 70:479–86. doi: 10.1016/j.biopsych.2011.04.022
133. Zimmermann T, Bartsch JC, Beer A, Lomazzo E, Guggenhuber S, Lange MD, et al. Impaired anandamide/palmitoylethanolamide signaling in hippocampal glutamatergic neurons alters synaptic plasticity, learning, and emotional responses. Neuropsychopharmacology (2019) 44:1377–88. doi: 10.1038/s41386-018-0274-7
134. Aisenberg N, Serova L, Sabban EL, Akirav I. The effects of enhancing endocannabinoid signaling and blocking corticotrophin releasing factor receptor in the amygdala and hippocampus on the consolidation of a stressful event. Eur Neuropsychopharmacol (2017) 27:913–27. doi: 10.1016/j.euroneuro.2017.06.006
135. Bortolato M, Campolongo P, Mangieri RA, Scattoni ML, Frau R, Trezza V, et al. Anxiolytic-like properties of the anandamide transport inhibitor AM404. Neuropsychopharmacology (2006) 31:2652–9. doi: 10.1038/sj.npp.1301061
136. Campos AC, Ferreira FR, Guimaraes FS, Lemos JI. Facilitation of endocannabinoid effects in the ventral hippocampus modulates anxiety-like behaviors depending on previous stress experience. Neuroscience (2010) 167:238–46. doi: 10.1016/j.neuroscience.2010.01.062
137. Duan T, Gu N, Wang Y, Wang F, Zhu J, Fang Y, et al. Fatty acid amide hydrolase inhibitors produce rapid anti-anxiety responses through amygdala long-term depression in male rodents. J Psychiatry Neurosci (2017) 42:230–41. doi: 10.1503/jpn.160116
138. El-Alfy AT, Abourashed EA, Patel C, Mazhari N, An H, Jeon A. Phenolic compounds from nutmeg (Myristica fragrans Houtt.) inhibit the endocannabinoid-modulating enzyme fatty acid amide hydrolase. J Pharm Pharmacol (2019) 71:1879–89. doi: 10.1111/jphp.13174
139. Griebel G, Stemmelin J, Lopez-Grancha M, Fauchey V, Slowinski F, Pichat P, et al. The selective reversible FAAH inhibitor, SSR411298, restores the development of maladaptive behaviors to acute and chronic stress in rodents. Sci Rep (2018) 8:2416. doi: 10.1038/s41598-018-20895-z
140. Kathuria S, Gaetani S, Fegley D, Valino F, Duranti A, Tontini A, et al. Modulation of anxiety through blockade of anandamide hydrolysis. Nat Med (2003) 9:76–81. doi: 10.1038/nm803
141. Marco EM, Rapino C, Caprioli A, Borsini F, Laviola G, Maccarrone M. Potential Therapeutic Value of a Novel FAAH Inhibitor for the Treatment of Anxiety. PloS One (2015) 10:e0137034. doi: 10.1371/journal.pone.0137034
142. Scherma M, Medalie J, Fratta W, Vadivel SK, Makriyannis A, Piomelli D, et al. The endogenous cannabinoid anandamide has effects on motivation and anxiety that are revealed by fatty acid amide hydrolase (FAAH) inhibition. Neuropharmacology (2008) 54:129–40. doi: 10.1016/j.neuropharm.2007.08.011
143. Bluett RJ, Gamble-George JC, Hermanson DJ, Hartley ND, Marnett LJ, Patel S. Central anandamide deficiency predicts stress-induced anxiety: behavioral reversal through endocannabinoid augmentation. Transl Psychiatry (2014) 4:e408. doi: 10.1038/tp.2014.53
144. Hill MN, Kumar SA, Filipski SB, Iverson M, Stuhr KL, Keith JM, et al. Disruption of fatty acid amide hydrolase activity prevents the effects of chronic stress on anxiety and amygdalar microstructure. Mol Psychiatry (2013) 18:1125–35. doi: 10.1038/mp.2012.90
145. Morena M, Aukema RJ, Leitl KD, Rashid AJ, Vecchiarelli HA, Josselyn SA, et al. Upregulation of Anandamide Hydrolysis in the Basolateral Complex of Amygdala Reduces Fear Memory Expression and Indices of Stress and Anxiety. J Neurosci (2019) 39:1275–92. doi: 10.1523/JNEUROSCI.2251-18.2018
146. Rossi S, De Chiara V, Musella A, Sacchetti L, Cantarella C, Castelli M, et al. Preservation of striatal cannabinoid CB1 receptor function correlates with the antianxiety effects of fatty acid amide hydrolase inhibition. Mol Pharmacol (2010) 78:260–8. doi: 10.1124/mol.110.064196
147. Moreira FA, Kaiser N, Monory K, Lutz B. Reduced anxiety-like behaviour induced by genetic and pharmacological inhibition of the endocannabinoid-degrading enzyme fatty acid amide hydrolase (FAAH) is mediated by CB1 receptors. Neuropharmacology (2008) 54:141–50. doi: 10.1016/j.neuropharm.2007.07.005
148. Haller J, Barna I, Barsvari B, Gyimesi Pelczer K, Yasar S, Panlilio LV, et al. Interactions between environmental aversiveness and the anxiolytic effects of enhanced cannabinoid signaling by FAAH inhibition in rats. Psychopharmacol (Berl) (2009) 204:607–16. doi: 10.1007/s00213-009-1494-7
149. Micale V, Cristino L, Tamburella A, Petrosino S, Leggio GM, Drago F, et al. Anxiolytic effects in mice of a dual blocker of fatty acid amide hydrolase and transient receptor potential vanilloid type-1 channels. Neuropsychopharmacology (2009) 34:593–606. doi: 10.1038/npp.2008.98
150. Guggenhuber S, Romo-Parra H, Bindila L, Leschik J, Lomazzo E, Remmers F, et al. Impaired 2-AG Signaling in Hippocampal Glutamatergic Neurons: Aggravation of Anxiety-Like Behavior and Unaltered Seizure Susceptibility. Int J Neuropsychopharmacol (2015) 19: pyv091. doi: 10.1093/ijnp/pyv091
151. Almeida-Santos AF, Gobira PH, Rosa LC, Guimaraes FS, Moreira FA, Aguiar DC. Modulation of anxiety-like behavior by the endocannabinoid 2-arachidonoylglycerol (2-AG) in the dorsolateral periaqueductal gray. Behav Brain Res (2013) 252:10–7. doi: 10.1016/j.bbr.2013.05.027
152. Almeida-Santos AF, Moreira FA, Guimaraes FS, Aguiar DC. 2-Arachidonoylglycerol endocannabinoid signaling coupled to metabotropic glutamate receptor type-5 modulates anxiety-like behavior in the rat ventromedial prefrontal cortex. J Psychopharmacol (2017) 31:740–9. doi: 10.1177/0269881117704986
153. Sciolino NR, Zhou W, Hohmann AG. Enhancement of endocannabinoid signaling with JZL184, an inhibitor of the 2-arachidonoylglycerol hydrolyzing enzyme monoacylglycerol lipase, produces anxiolytic effects under conditions of high environmental aversiveness in rats. Pharmacol Res (2011) 64:226–34. doi: 10.1016/j.phrs.2011.04.010
154. Aliczki M, Zelena D, Mikics E, Varga ZK, Pinter O, Bakos NV, et al. Monoacylglycerol lipase inhibition-induced changes in plasma corticosterone levels, anxiety and locomotor activity in male CD1 mice. Horm Behav (2013) 63:752–8. doi: 10.1016/j.yhbeh.2013.03.017
155. Bosch-Bouju C, Larrieu T, Linders L, Manzoni OJ, Laye S. Endocannabinoid-Mediated Plasticity in Nucleus Accumbens Controls Vulnerability to Anxiety after Social Defeat Stress. Cell Rep (2016) 16:1237–42. doi: 10.1016/j.celrep.2016.06.082
156. Gobira PH, Almeida-Santos AF, Guimaraes FS, Moreira FA, Aguiar DC. Role of the endocannabinoid 2-arachidonoylglycerol in aversive responses mediated by the dorsolateral periaqueductal grey. Eur Neuropsychopharmacol (2016) 26:15–22. doi: 10.1016/j.euroneuro.2015.11.014
157. Imperatore R, Morello G, Luongo L, Taschler U, Romano R, De Gregorio D, et al. Genetic deletion of monoacylglycerol lipase leads to impaired cannabinoid receptor CB(1)R signaling and anxiety-like behavior. J Neurochem (2015) 135:799–813. doi: 10.1111/jnc.13267
158. Jenniches I, Ternes S, Albayram O, Otte DM, Bach K, Bindila L, et al. Anxiety, Stress, and Fear Response in Mice With Reduced Endocannabinoid Levels. Biol Psychiatry (2016) 79:858–68. doi: 10.1016/j.biopsych.2015.03.033
159. Shonesy BC, Bluett RJ, Ramikie TS, Baldi R, Hermanson DJ, Kingsley PJ, et al. Genetic disruption of 2-arachidonoylglycerol synthesis reveals a key role for endocannabinoid signaling in anxiety modulation. Cell Rep (2014) 9:1644–53. doi: 10.1016/j.celrep.2014.11.001
160. Garcia-Gutierrez MS, Manzanares J. Overexpression of CB2 cannabinoid receptors decreased vulnerability to anxiety and impaired anxiolytic action of alprazolam in mice. J Psychopharmacol (2011) 25:111–20. doi: 10.1177/0269881110379507
161. Bahi A, Al Mansouri S, Al Memari E, Al Ameri M, Nurulain SM, Ojha S. beta-Caryophyllene, a CB2 receptor agonist produces multiple behavioral changes relevant to anxiety and depression in mice. Physiol Behav (2014) 135:119–24. doi: 10.1016/j.physbeh.2014.06.003
162. Robertson JM, Achua JK, Smith JP, Prince MA, Staton CD, Ronan PJ, et al. Anxious behavior induces elevated hippocampal Cb2 receptor gene expression. Neuroscience (2017) 352:273–84. doi: 10.1016/j.neuroscience.2017.03.061
163. Li Y, Kim J. Distinct roles of neuronal and microglial CB2 cannabinoid receptors in the mouse hippocampus. Neuroscience (2017) 363:11–25. doi: 10.1016/j.neuroscience.2017.08.053
164. Fabre LF, McLendon D. The efficacy and safety of nabilone (a synthetic cannabinoid) in the treatment of anxiety. J Clin Pharmacol (1981) 21:377S–82S. doi: 10.1002/j.1552-4604.1981.tb02617.x
165. Crippa JA, Zuardi AW, Martin-Santos R, Bhattacharyya S, Atakan Z, McGuire P, et al. Cannabis and anxiety: a critical review of the evidence. Hum Psychopharmacol (2009) 24:515–23. doi: 10.1002/hup.1048
166. Bhattacharyya S, Egerton A, Kim E, Rosso L, Riano Barros D, Hammers A, et al. Acute induction of anxiety in humans by delta-9-tetrahydrocannabinol related to amygdalar cannabinoid-1 (CB1) receptors. Sci Rep (2017) 7:15025. doi: 10.1038/s41598-017-14203-4
167. Christensen R, Kristensen PK, Bartels EM, Bliddal H, Astrup A. Efficacy and safety of the weight-loss drug rimonabant: a meta-analysis of randomised trials. Lancet (2007) 370:1706–13. doi: 10.1016/S0140-6736(07)61721-8
168. Bergamaschi MM, Queiroz RH, Chagas MH, Linares IM, Arrais KC, de Oliveira DC, et al. Rimonabant effects on anxiety induced by simulated public speaking in healthy humans: a preliminary report. Hum Psychopharmacol (2014) 29:94–9. doi: 10.1002/hup.2374
169. Nguyen T, Thomas BF, Zhang Y. Overcoming the Psychiatric Side Effects of the Cannabinoid CB1 Receptor Antagonists: Current Approaches for Therapeutics Development. Curr Top Med Chem (2019) 19:1418–35. doi: 10.2174/1568026619666190708164841
170. Gonda X, Petschner P, Eszlari N, Sutori S, Gal Z, Koncz S, et al. Effects of Different Stressors Are Modulated by Different Neurobiological Systems: The Role of GABA-A Versus CB1 Receptor Gene Variants in Anxiety and Depression. Front Cell Neurosci (2019) 13:138. doi: 10.3389/fncel.2019.00138
171. Gartner A, Dorfel D, Diers K, Witt SH, Strobel A, Brocke B. Impact of FAAH genetic variation on fronto-amygdala function during emotional processing. Eur Arch Psychiatry Clin Neurosci (2019) 269:209–21. doi: 10.1007/s00406-018-0944-9
172. Gee DG, Fetcho RN, Jing D, Li A, Glatt CE, Drysdale AT, et al. Individual differences in frontolimbic circuitry and anxiety emerge with adolescent changes in endocannabinoid signaling across species. Proc Natl Acad Sci U S A (2016) 113:4500–5. doi: 10.1073/pnas.1600013113
173. Harris BN, Hohman ZP, Campbell CM, King KS, Tucker CA, Garrison Institute on A. FAAH genotype, CRFR1 genotype, and cortisol interact to predict anxiety in an aging, rural Hispanic population: A Project FRONTIER study. Neurobiol Stress (2019) 10:100154. doi: 10.1016/j.ynstr.2019.100154
174. Demers CH, Drabant Conley E, Bogdan R, Hariri AR. Interactions Between Anandamide and Corticotropin-Releasing Factor Signaling Modulate Human Amygdala Function and Risk for Anxiety Disorders: An Imaging Genetics Strategy for Modeling Molecular Interactions. Biol Psychiatry (2016) 80:356–62. doi: 10.1016/j.biopsych.2015.12.021
175. Lazary J, Eszlari N, Juhasz G, Bagdy G. Genetically reduced FAAH activity may be a risk for the development of anxiety and depression in persons with repetitive childhood trauma. Eur Neuropsychopharmacol (2016) 26:1020–8. doi: 10.1016/j.euroneuro.2016.03.003
176. Lazary J, Eszlari N, Juhasz G, Bagdy G. A functional variant of CB2 receptor gene interacts with childhood trauma and FAAH gene on anxious and depressive phenotypes. J Affect Disord (2019) 257:716–22. doi: 10.1016/j.jad.2019.07.083
177. World Health Organization. (2017). Depression and other common mental disorders. Global health estimates. World Health Organization.
178. Aso E, Ozaita A, Valdizan EM, Ledent C, Pazos A, Maldonado R, et al. BDNF impairment in the hippocampus is related to enhanced despair behavior in CB1 knockout mice. J Neurochem (2008) 105:565–72. doi: 10.1111/j.1471-4159.2007.05149.x
179. Aso E, Ozaita A, Serra MA, Maldonado R. Genes differentially expressed in CB1 knockout mice: involvement in the depressive-like phenotype. Eur Neuropsychopharmacol (2011) 21:11–22. doi: 10.1016/j.euroneuro.2010.06.007
180. Valverde O, Torrens M. CB1 receptor-deficient mice as a model for depression. Neuroscience (2012) 204:193–206. doi: 10.1016/j.neuroscience.2011.09.031
181. Shearman LP, Rosko KM, Fleischer R, Wang J, Xu S, Tong XS, et al. Antidepressant-like and anorectic effects of the cannabinoid CB1 receptor inverse agonist AM251 in mice. Behav Pharmacol (2003) 14:573–82. doi: 10.1097/00008877-200312000-00001
182. Griebel G, Stemmelin J, Scatton B. Effects of the cannabinoid CB1 receptor antagonist rimonabant in models of emotional reactivity in rodents. Biol Psychiatry (2005) 57:261–7. doi: 10.1016/j.biopsych.2004.10.032
183. Adamczyk P, Golda A, McCreary AC, Filip M, Przegalinski E. Activation of endocannabinoid transmission induces antidepressant-like effects in rats. J Physiol Pharmacol (2008) 59:217–28.
184. Ebrahimi-Ghiri M, Nasehi M, Zarrindast MR. Anxiolytic and antidepressant effects of ACPA and harmaline co-treatment. Behav Brain Res (2019) 364:296–302. doi: 10.1016/j.bbr.2019.02.034
185. Elbatsh MM, Moklas MA, Marsden CA, Kendall DA. Antidepressant-like effects of Delta(9)-tetrahydrocannabinol and rimonabant in the olfactory bulbectomised rat model of depression. Pharmacol Biochem Behav (2012) 102:357–65. doi: 10.1016/j.pbb.2012.05.009
186. Haj-Mirzaian A, Amini-Khoei H, Haj-Mirzaian A, Amiri S, Ghesmati M, Zahir M, et al. Activation of cannabinoid receptors elicits antidepressant-like effects in a mouse model of social isolation stress. Brain Res Bull (2017) 130:200–10. doi: 10.1016/j.brainresbull.2017.01.018
187. Segev A, Rubin AS, Abush H, Richter-Levin G, Akirav I. Cannabinoid receptor activation prevents the effects of chronic mild stress on emotional learning and LTP in a rat model of depression. Neuropsychopharmacology (2014) 39:919–33. doi: 10.1038/npp.2013.292
188. Beyer CE, Dwyer JM, Piesla MJ, Platt BJ, Shen R, Rahman Z, et al. Depression-like phenotype following chronic CB1 receptor antagonism. Neurobiol Dis (2010) 39:148–55. doi: 10.1016/j.nbd.2010.03.020
189. McLaughlin RJ, Hill MN, Morrish AC, Gorzalka BB. Local enhancement of cannabinoid CB1 receptor signalling in the dorsal hippocampus elicits an antidepressant-like effect. Behav Pharmacol (2007) 18:431–8. doi: 10.1097/FBP.0b013e3282ee7b44
190. Shen CJ, Zheng D, Li KX, Yang JM, Pan HQ, Yu XD, et al. Cannabinoid CB1 receptors in the amygdalar cholecystokinin glutamatergic afferents to nucleus accumbens modulate depressive-like behavior. Nat Med (2019) 25:337–49. doi: 10.1038/s41591-018-0299-9
191. Hill MN, Carrier EJ, McLaughlin RJ, Morrish AC, Meier SE, Hillard CJ, et al. Regional alterations in the endocannabinoid system in an animal model of depression: effects of concurrent antidepressant treatment. J Neurochem (2008) 106:2322–36. doi: 10.1111/j.1471-4159.2008.05567.x
192. Reich CG, Taylor ME, McCarthy MM. Differential effects of chronic unpredictable stress on hippocampal CB1 receptors in male and female rats. Behav Brain Res (2009) 203:264–9. doi: 10.1016/j.bbr.2009.05.013
193. Wang W, Sun D, Pan B, Roberts CJ, Sun X, Hillard CJ, et al. Deficiency in endocannabinoid signaling in the nucleus accumbens induced by chronic unpredictable stress. Neuropsychopharmacology (2010) 35:2249–61. doi: 10.1038/npp.2010.99
194. Park H, Rhee J, Lee S, Chung C. Selectively Impaired Endocannabinoid-Dependent Long-Term Depression in the Lateral Habenula in an Animal Model of Depression. Cell Rep (2017) 20:289–96. doi: 10.1016/j.celrep.2017.06.049
195. Kirkedal C, Elfving B, Muller HK, Moreira FA, Bindila L, Lutz B, et al. Hemisphere-dependent endocannabinoid system activity in prefrontal cortex and hippocampus of the Flinders Sensitive Line rodent model of depression. Neurochem Int (2019) 125:7–15. doi: 10.1016/j.neuint.2019.01.023
196. Vinod KY, Xie S, Psychoyos D, Hungund BL, Cooper TB, Tejani-Butt SM. Dysfunction in fatty acid amide hydrolase is associated with depressive-like behavior in Wistar Kyoto rats. PloS One (2012) 7:e36743. doi: 10.1371/journal.pone.0036743
197. Mangieri RA, Piomelli D. Enhancement of endocannabinoid signaling and the pharmacotherapy of depression. Pharmacol Res (2007) 56:360–6. doi: 10.1016/j.phrs.2007.09.003
198. Bortolato M, Mangieri RA, Fu J, Kim JH, Arguello O, Duranti A, et al. Antidepressant-like activity of the fatty acid amide hydrolase inhibitor URB597 in a rat model of chronic mild stress. Biol Psychiatry (2007) 62:1103–10. doi: 10.1016/j.biopsych.2006.12.001
199. Realini N, Vigano D, Guidali C, Zamberletti E, Rubino T, Parolaro D. Chronic URB597 treatment at adulthood reverted most depressive-like symptoms induced by adolescent exposure to THC in female rats. Neuropharmacology (2011) 60:235–43. doi: 10.1016/j.neuropharm.2010.09.003
200. Wang Y, Zhang X. FAAH inhibition produces antidepressant-like efforts of mice to acute stress via synaptic long-term depression. Behav Brain Res (2017) 324:138–45. doi: 10.1016/j.bbr.2017.01.054
201. Zhang Z, Wang W, Zhong P, Liu SJ, Long JZ, Zhao L, et al. Blockade of 2-arachidonoylglycerol hydrolysis produces antidepressant-like effects and enhances adult hippocampal neurogenesis and synaptic plasticity. Hippocampus (2015) 25:16–26. doi: 10.1002/hipo.22344
202. Dong B, Shilpa BM, Shah R, Goyal A, Xie S, Bakalian MJ, et al. Dual pharmacological inhibitor of endocannabinoid degrading enzymes reduces depressive-like behavior in female rats. J Psychiatr Res (2020) 120:103–12. doi: 10.1016/j.jpsychires.2019.10.010
203. Chen DJ, Gao M, Gao FF, Su QX, Wu J. Brain cannabinoid receptor 2: expression, function and modulation. Acta Pharmacol Sin (2017) 38:312–6. doi: 10.1038/aps.2016.149
204. Onaivi ES, Ishiguro H, Gong JP, Patel S, Meozzi PA, Myers L, et al. Brain neuronal CB2 cannabinoid receptors in drug abuse and depression: from mice to human subjects. PloS One (2008) 3:e1640. doi: 10.1371/journal.pone.0001640
205. Hu B, Doods H, Treede RD, Ceci A. Depression-like behaviour in rats with mononeuropathy is reduced by the CB2-selective agonist GW405833. Pain (2009) 143:206–12. doi: 10.1016/j.pain.2009.02.018
206. Wang S, Sun H, Liu S, Wang T, Guan J, Jia J. Role of hypothalamic cannabinoid receptors in post-stroke depression in rats. Brain Res Bull (2016) 121:91–7. doi: 10.1016/j.brainresbull.2016.01.006
207. Hwang ES, Kim HB, Lee S, Kim MJ, Kim KJ, Han G, et al. Antidepressant-like effects of beta-caryophyllene on restraint plus stress-induced depression. Behav Brain Res (2019) 380:112439. doi: 10.1016/j.bbr.2019.112439
208. Smaga I, Bystrowska B, Gawlinski D, Pomierny B, Stankowicz P, Filip M. Antidepressants and changes in concentration of endocannabinoids and N-acylethanolamines in rat brain structures. Neurotox Res (2014) 26:190–206. doi: 10.1007/s12640-014-9465-0
209. Hill MN, Ho WS, Hillard CJ, Gorzalka BB. Differential effects of the antidepressants tranylcypromine and fluoxetine on limbic cannabinoid receptor binding and endocannabinoid contents. J Neural Transm (Vienna) (2008) 115:1673–9. doi: 10.1007/s00702-008-0131-7
210. Smaga I, Zaniewska M, Gawlinski D, Faron-Gorecka A, Szafranski P, Cegla M, et al. Changes in the cannabinoids receptors in rats following treatment with antidepressants. Neurotoxicology (2017) 63:13–20. doi: 10.1016/j.neuro.2017.08.012
211. Rodriguez-Gaztelumendi A, Rojo ML, Pazos A, Diaz A. Altered CB receptor-signaling in prefrontal cortex from an animal model of depression is reversed by chronic fluoxetine. J Neurochem (2009) 108:1423–33. doi: 10.1111/j.1471-4159.2009.05898.x
212. Bambico FR, Katz N, Debonnel G, Gobbi G. Cannabinoids elicit antidepressant-like behavior and activate serotonergic neurons through the medial prefrontal cortex. J Neurosci (2007) 27:11700–11. doi: 10.1523/JNEUROSCI.1636-07.2007
213. Burokas A, Martin-Garcia E, Gutierrez-Cuesta J, Rojas S, Herance JR, Gispert JD, et al. Relationships between serotonergic and cannabinoid system in depressive-like behavior: a PET study with [11C.-DASB. J Neurochem (2014) 130:126–35. doi: 10.1111/jnc.12716
214. Umathe SN, Manna SS, Jain NS. Involvement of endocannabinoids in antidepressant and anti-compulsive effect of fluoxetine in mice. Behav Brain Res (2011) 223:125–34. doi: 10.1016/j.bbr.2011.04.031
215. Hill MN, Ho WS, Sinopoli KJ, Viau V, Hillard CJ, Gorzalka BB. Involvement of the endocannabinoid system in the ability of long-term tricyclic antidepressant treatment to suppress stress-induced activation of the hypothalamic-pituitary-adrenal axis. Neuropsychopharmacology (2006) 31:2591–9. doi: 10.1038/sj.npp.1301092
216. Khakpai F, Ebrahimi-Ghiri M, Alijanpour S, Zarrindast MR. Ketamine-induced antidepressant like effects in mice: A possible involvement of cannabinoid system. BioMed Pharmacother (2019) 112:108717. doi: 10.1016/j.biopha.2019.108717
217. Ostadhadi S, Haj-Mirzaian A, Nikoui V, Kordjazy N, Dehpour AR. Involvement of opioid system in antidepressant-like effect of the cannabinoid CB1 receptor inverse agonist AM-251 after physical stress in mice. Clin Exp Pharmacol Physiol (2016) 43:203–12. doi: 10.1111/1440-1681.12518
218. Kruk-Slomka M, Michalak A, Biala G. Antidepressant-like effects of the cannabinoid receptor ligands in the forced swimming test in mice: mechanism of action and possible interactions with cholinergic system. Behav Brain Res (2015) 284:24–36. doi: 10.1016/j.bbr.2015.01.051
219. Wang HN, Wang L, Zhang RG, Chen YC, Liu L, Gao F, et al. Anti-depressive mechanism of repetitive transcranial magnetic stimulation in rat: the role of the endocannabinoid system. J Psychiatr Res (2014) 51:79–87. doi: 10.1016/j.jpsychires.2014.01.004
220. Fang G, Wang Y. Effects of rTMS on Hippocampal Endocannabinoids and Depressive-like Behaviors in Adolescent Rats. Neurochem Res (2018) 43:1756–65. doi: 10.1007/s11064-018-2591-y
221. Xue SS, Xue F, Ma QR, Wang SQ, Wang Y, Tan QR, et al. Repetitive high-frequency transcranial magnetic stimulation reverses depressive-like behaviors and protein expression at hippocampal synapses in chronic unpredictable stress-treated rats by enhancing endocannabinoid signaling. Pharmacol Biochem Behav (2019) 184:172738. doi: 10.1016/j.pbb.2019.172738
222. Hill MN, Barr AM, Ho WS, Carrier EJ, Gorzalka BB, Hillard CJ. Electroconvulsive shock treatment differentially modulates cortical and subcortical endocannabinoid activity. J Neurochem (2007) 103:47–56. doi: 10.1111/j.1471-4159.2007.04688.x
223. Koethe D, Llenos IC, Dulay JR, Hoyer C, Torrey EF, Leweke FM, et al. Expression of CB1 cannabinoid receptor in the anterior cingulate cortex in schizophrenia, bipolar disorder, and major depression. J Neural Transm (Vienna) (2007) 114:1055–63. doi: 10.1007/s00702-007-0660-5
224. Choi K, Le T, McGuire J, Xing G, Zhang L, Li H, et al. Expression pattern of the cannabinoid receptor genes in the frontal cortex of mood disorder patients and mice selectively bred for high and low fear. J Psychiatr Res (2012) 46:882–9. doi: 10.1016/j.jpsychires.2012.03.021
225. Rodriguez-Munoz M, Sanchez-Blazquez P, Callado LF, Meana JJ, Garzon-Nino J. Schizophrenia and depression, two poles of endocannabinoid system deregulation. Transl Psychiatry (2017) 7:1291. doi: 10.1038/s41398-017-0029-y
226. Hill MN, Miller GE, Ho WS, Gorzalka BB, Hillard CJ. Serum endocannabinoid content is altered in females with depressive disorders: a preliminary report. Pharmacopsychiatry (2008) 41:48–53. doi: 10.1055/s-2007-993211
227. Hill MN, Miller GE, Carrier EJ, Gorzalka BB, Hillard CJ. Circulating endocannabinoids and N-acyl ethanolamines are differentially regulated in major depression and following exposure to social stress. Psychoneuroendocrinology (2009) 34:1257–62. doi: 10.1016/j.psyneuen.2009.03.013
228. Romero-Sanchiz P, Nogueira-Arjona R, Pastor A, Araos P, Serrano A, Boronat A, et al. Plasma concentrations of oleoylethanolamide in a primary care sample of depressed patients are increased in those treated with selective serotonin reuptake inhibitor-type antidepressants. Neuropharmacology (2019) 149:212–20. doi: 10.1016/j.neuropharm.2019.02.026
229. Heyman E, Gamelin FX, Goekint M, Piscitelli F, Roelands B, Leclair E, et al. Intense exercise increases circulating endocannabinoid and BDNF levels in humans–possible implications for reward and depression. Psychoneuroendocrinology (2012) 37:844–51. doi: 10.1016/j.psyneuen.2011.09.017
230. Meyer JD, Crombie KM, Cook DB, Hillard CJ, Koltyn KF. Serum Endocannabinoid and Mood Changes after Exercise in Major Depressive Disorder. Med Sci Sports Exerc (2019) 51:1909–17. doi: 10.1249/MSS.0000000000002006
231. Kranaster L, Hoyer C, Aksay SS, Bumb JM, Leweke FM, Janke C, et al. Electroconvulsive therapy enhances endocannabinoids in the cerebrospinal fluid of patients with major depression: a preliminary prospective study. Eur Arch Psychiatry Clin Neurosci (2017) 267:781–6. doi: 10.1007/s00406-017-0789-7
232. Monteleone P, Bifulco M, Maina G, Tortorella A, Gazzerro P, Proto MC, et al. Investigation of CNR1 and FAAH endocannabinoid gene polymorphisms in bipolar disorder and major depression. Pharmacol Res (2010) 61:400–4. doi: 10.1016/j.phrs.2010.01.002
233. Schennach R, Zill P, Obermeier M, Hauer D, Dehning S, Cerovecki A, et al. The CNR1 gene in depression and schizophrenia - is there an association with early improvement and response? Psychiatry Res (2012) 196:160. doi: 10.1016/j.psychres.2011.11.021
234. Domschke K, Dannlowski U, Ohrmann P, Lawford B, Bauer J, Kugel H, et al. Cannabinoid receptor 1 (CNR1) gene: impact on antidepressant treatment response and emotion processing in major depression. Eur Neuropsychopharmacol (2008) 18:751–9. doi: 10.1016/j.euroneuro.2008.05.003
235. Mitjans M, Gasto C, Catalan R, Fananas L, Arias B. Genetic variability in the endocannabinoid system and 12-week clinical response to citalopram treatment: the role of the CNR1, CNR2 and FAAH genes. J Psychopharmacol (2012) 26:1391–8. doi: 10.1177/0269881112454229
236. Agrawal A, Nelson EC, Littlefield AK, Bucholz KK, Degenhardt L, Henders AK, et al. Cannabinoid receptor genotype moderation of the effects of childhood physical abuse on anhedonia and depression. Arch Gen Psychiatry (2012) 69:732–40. doi: 10.1001/archgenpsychiatry.2011.2273
237. Juhasz G, Chase D, Pegg E, Downey D, Toth ZG, Stones K, et al. CNR1 gene is associated with high neuroticism and low agreeableness and interacts with recent negative life events to predict current depressive symptoms. Neuropsychopharmacology (2009) 34:2019–27. doi: 10.1038/npp.2009.19
238. Barrero FJ, Ampuero I, Morales B, Vives F, de Dios Luna Del Castillo J, Hoenicka J, et al. Depression in Parkinson's disease is related to a genetic polymorphism of the cannabinoid receptor gene (CNR1). Pharmacogenomics J (2005) 5:135–41. doi: 10.1038/sj.tpj.6500301
239. Icick R, Peoc'h K, Karsinti E, Ksouda K, Hajj A, Bloch V, et al. A cannabinoid receptor 1 polymorphism is protective against major depressive disorder in methadone-maintained outpatients. Am J Addict (2015) 24:613–20. doi: 10.1111/ajad.12273
240. Kong X, Miao Q, Lu X, Zhang Z, Chen M, Zhang J, et al. The association of endocannabinoid receptor genes (CNR1 and CNR2) polymorphisms with depression: A meta-analysis. Med (Baltimore) (2019) 98:e17403. doi: 10.1097/MD.0000000000017403
241. Onaivi ES, Ishiguro H, Gong JP, Patel S, Meozzi PA, Myers L, et al. Functional expression of brain neuronal CB2 cannabinoid receptors are involved in the effects of drugs of abuse and in depression. Ann N Y Acad Sci (2008) 1139:434–49. doi: 10.1196/annals.1432.036
242. Owen MJ, Sawa A, Mortensen PB. Schizophrenia. Lancet (2016) 388:86–97. doi: 10.1016/S0140-6736(15)01121-6
243. Marder SR, Cannon TD. Schizophrenia. N Engl J Med (2019) 381:1753–61. doi: 10.1056/NEJMra1808803
244. Sherif M, Radhakrishnan R, D'Souza DC, Ranganathan M. Human Laboratory Studies on Cannabinoids and Psychosis. Biol Psychiatry (2016) 79:526–38. doi: 10.1016/j.biopsych.2016.01.011
245. Powell SB, Zhou X, Geyer MA. Prepulse inhibition and genetic mouse models of schizophrenia. Behav Brain Res (2009) 204:282–94. doi: 10.1016/j.bbr.2009.04.021
246. Braff DL. Prepulse inhibition of the startle reflex: a window on the brain in schizophrenia. Curr Top Behav Neurosci (2010) 4:349–71. doi: 10.1007/7854_2010_61
247. Mansbach RS, Rovetti CC, Winston EN, Lowe 3. , Effects of the cannabinoid CB1 receptor antagonist SR141716A on the behavior of pigeons and rats. Psychopharmacol (Berl) (1996) 124:315–22. doi: 10.1007/BF02247436
248. Martin RS, Secchi RL, Sung E, Lemaire M, Bonhaus DW, Hedley LR, et al. Effects of cannabinoid receptor ligands on psychosis-relevant behavior models in the rat. Psychopharmacol (Berl) (2003) 165:128–35. doi: 10.1007/s00213-002-1240-x
249. Hajos M, Hoffmann WE, Kocsis B. Activation of cannabinoid-1 receptors disrupts sensory gating and neuronal oscillation: relevance to schizophrenia. Biol Psychiatry (2008) 63:1075–83. doi: 10.1016/j.biopsych.2007.12.005
250. Lee G, Zhou Y. NMDAR Hypofunction Animal Models of Schizophrenia. Front Mol Neurosci (2019) 12:185. doi: 10.3389/fnmol.2019.00185
251. Ballmaier M, Bortolato M, Rizzetti C, Zoli M, Gessa G, Heinz A, et al. Cannabinoid receptor antagonists counteract sensorimotor gating deficits in the phencyclidine model of psychosis. Neuropsychopharmacology (2007) 32:2098–107. doi: 10.1038/sj.npp.1301344
252. Guidali C, Vigano D, Petrosino S, Zamberletti E, Realini N, Binelli G, et al. Cannabinoid CB1 receptor antagonism prevents neurochemical and behavioural deficits induced by chronic phencyclidine. Int J Neuropsychopharmacol (2011) 14:17–28. doi: 10.1017/S1461145710000209
253. Black MD, Stevens RJ, Rogacki N, Featherstone RE, Senyah Y, Giardino O, et al. AVE1625, a cannabinoid CB1 receptor antagonist, as a co-treatment with antipsychotics for schizophrenia: improvement in cognitive function and reduction of antipsychotic-side effects in rodents. Psychopharmacol (Berl) (2011) 215:149–63. doi: 10.1007/s00213-010-2124-0
254. Kruk-Slomka M, Budzynska B, Slomka T, Banaszkiewicz I, Biala G. The Influence of the CB1 Receptor Ligands on the Schizophrenia-Like Effects in Mice Induced by MK-801. Neurotox Res (2016) 30:658–76. doi: 10.1007/s12640-016-9662-0
255. Szucs E, Dvoracsko S, Tomboly C, Buki A, Kekesi G, Horvath G, et al. Decreased CB receptor binding and cannabinoid signaling in three brain regions of a rat model of schizophrenia. Neurosci Lett (2016) 633:87–93. doi: 10.1016/j.neulet.2016.09.020
256. Gomes FV, Edelson JR, Volk DW, Grace AA. Altered brain cannabinoid 1 receptor mRNA expression across postnatal development in the MAM model of schizophrenia. Schizophr Res (2018) 201:254–60. doi: 10.1016/j.schres.2018.04.030
257. Almeida V, Levin R, Peres FF, Suiama MA, Vendramini AM, Santos CM, et al. Role of the endocannabinoid and endovanilloid systems in an animal model of schizophrenia-related emotional processing/cognitive deficit. Neuropharmacology (2019) 155:44–53. doi: 10.1016/j.neuropharm.2019.05.015
258. Neary JL, Perez SM, Peterson K, Lodge DJ, Carless MA. Comparative analysis of MBD-seq and MeDIP-seq and estimation of gene expression changes in a rodent model of schizophrenia. Genomics (2017) 109:204–13. doi: 10.1016/j.ygeno.2017.03.004
259. Perez SM, Aguilar DD, Neary JL, Carless MA, Giuffrida A, Lodge DJ. Schizophrenia-Like Phenotype Inherited by the F2 Generation of a Gestational Disruption Model of Schizophrenia. Neuropsychopharmacology (2016) 41:477–86. doi: 10.1038/npp.2015.169
260. Perez SM, Donegan JJ, Boley AM, Aguilar DD, Giuffrida A, Lodge DJ. Ventral hippocampal overexpression of Cannabinoid Receptor Interacting Protein 1 (CNRIP1) produces a schizophrenia-like phenotype in the rat. Schizophr Res (2019) 206:263–70. doi: 10.1016/j.schres.2018.11.006
261. Vigano D, Guidali C, Petrosino S, Realini N, Rubino T, Di Marzo V, et al. Involvement of the endocannabinoid system in phencyclidine-induced cognitive deficits modelling schizophrenia. Int J Neuropsychopharmacol (2009) 12:599–614. doi: 10.1017/S1461145708009371
262. Eisenstein SA, Clapper JR, Holmes PV, Piomelli D, Hohmann AG. A role for 2-arachidonoylglycerol and endocannabinoid signaling in the locomotor response to novelty induced by olfactory bulbectomy. Pharmacol Res (2010) 61:419–29. doi: 10.1016/j.phrs.2009.12.013
263. Karl T. Neuregulin 1: a prime candidate for research into gene-environment interactions in schizophrenia? Insights from genetic rodent models. Front Behav Neurosci (2013) 7:106. doi: 10.3389/fnbeh.2013.00106
264. Clarke DJ, Stuart J, McGregor IS, Arnold JC. Endocannabinoid dysregulation in cognitive and stress-related brain regions in the Nrg1 mouse model of schizophrenia. Prog Neuropsychopharmacol Biol Psychiatry (2017) 72:9–15. doi: 10.1016/j.pnpbp.2016.08.006
265. Ishiguro H, Horiuchi Y, Ishikawa M, Koga M, Imai K, Suzuki Y, et al. Brain cannabinoid CB2 receptor in schizophrenia. Biol Psychiatry (2010) 67:974–82. doi: 10.1016/j.biopsych.2009.09.024
266. Ortega-Alvaro A, Aracil-Fernandez A, Garcia-Gutierrez MS, Navarrete F, Manzanares J. Deletion of CB2 cannabinoid receptor induces schizophrenia-related behaviors in mice. Neuropsychopharmacology (2011) 36:1489–504. doi: 10.1038/npp.2011.34
267. Khella R, Short JL, Malone DT. CB2 receptor agonism reverses MK-801-induced disruptions of prepulse inhibition in mice. Psychopharmacol (Berl) (2014) 231:3071–87. doi: 10.1007/s00213-014-3481-x
268. Kruk-Slomka M, Banaszkiewicz I, Biala G. The Impact of CB2 Receptor Ligands on the MK-801-Induced Hyperactivity in Mice. Neurotox Res (2017) 31:410–20. doi: 10.1007/s12640-017-9702-4
269. Dean B, Sundram S, Bradbury R, Scarr E, Copolov D. Studies on [3H.CP-55940 binding in the human central nervous system: regional specific changes in density of cannabinoid-1 receptors associated with schizophrenia and cannabis use. Neuroscience (2001) 103:9–15. doi: 10.1016/S0306-4522(00)00552-2
270. Jenko KJ, Hirvonen J, Henter ID, Anderson KB, Zoghbi SS, Hyde TM, et al. Binding of a tritiated inverse agonist to cannabinoid CB1 receptors is increased in patients with schizophrenia. Schizophr Res (2012) 141:185–8. doi: 10.1016/j.schres.2012.07.021
271. Volk DW, Eggan SM, Horti AG, Wong DF, Lewis DA. Reciprocal alterations in cortical cannabinoid receptor 1 binding relative to protein immunoreactivity and transcript levels in schizophrenia. Schizophr Res (2014) 159:124–9. doi: 10.1016/j.schres.2014.07.017
272. Dalton VS, Long LE, Weickert CS, Zavitsanou K. Paranoid schizophrenia is characterized by increased CB1 receptor binding in the dorsolateral prefrontal cortex. Neuropsychopharmacology (2011) 36:1620–30. doi: 10.1038/npp.2011.43
273. Muguruza C, Morentin B, Meana JJ, Alexander SP, Callado LF. Endocannabinoid system imbalance in the postmortem prefrontal cortex of subjects with schizophrenia. J Psychopharmacol (2019) 33:1132–40. doi: 10.1177/0269881119857205
274. Zavitsanou K, Garrick T, Huang XF. Selective antagonist [3H.SR141716A binding to cannabinoid CB1 receptors is increased in the anterior cingulate cortex in schizophrenia. Prog Neuropsychopharmacol Biol Psychiatry (2004) 28:355–60. doi: 10.1016/j.pnpbp.2003.11.005
275. Newell KA, Deng C, Huang XF. Increased cannabinoid receptor density in the posterior cingulate cortex in schizophrenia. Exp Brain Res (2006) 172:556–60. doi: 10.1007/s00221-006-0503-x
276. Dong R, Liu X, Liu Y, Deng Z, Nie X, Wang X, et al. Enrichment of epidermal stem cells by rapid adherence and analysis of the reciprocal interaction of epidermal stem cells with neighboring cells using an organotypic system. Cell Biol Int (2007) 31:733–40. doi: 10.1016/j.cellbi.2007.01.007
277. Eggan SM, Hashimoto T, Lewis DA. Reduced cortical cannabinoid 1 receptor messenger RNA and protein expression in schizophrenia. Arch Gen Psychiatry (2008) 65:772–84. doi: 10.1001/archpsyc.65.7.772
278. Uriguen L, Garcia-Fuster MJ, Callado LF, Morentin B, La Harpe R, Casado V, et al. Immunodensity and mRNA expression of A2A adenosine, D2 dopamine, and CB1 cannabinoid receptors in postmortem frontal cortex of subjects with schizophrenia: effect of antipsychotic treatment. Psychopharmacol (Berl) (2009) 206:313–24. doi: 10.1007/s00213-009-1608-2
279. Wong DF, Kuwabara H, Horti AG, Raymont V, Brasic J, Guevara M, et al. Quantification of cerebral cannabinoid receptors subtype 1 (CB1) in healthy subjects and schizophrenia by the novel PET radioligand [11C.OMAR. Neuroimage (2010) 52:1505–13. doi: 10.1016/j.neuroimage.2010.04.034
280. Ceccarini J, De Hert M, Van Winkel R, Peuskens J, Bormans G, Kranaster L, et al. Increased ventral striatal CB1 receptor binding is related to negative symptoms in drug-free patients with schizophrenia. Neuroimage (2013) 79:304–12. doi: 10.1016/j.neuroimage.2013.04.052
281. Ranganathan M, Cortes-Briones J, Radhakrishnan R, Thurnauer H, Planeta B, Skosnik P, et al. Reduced Brain Cannabinoid Receptor Availability in Schizophrenia. Biol Psychiatry (2016) 79:997–1005. doi: 10.1016/j.biopsych.2015.08.021
282. Mihov Y. Positron Emission Tomography Studies on Cannabinoid Receptor Type 1 in Schizophrenia. Biol Psychiatry (2016) 79:e97–9. doi: 10.1016/j.biopsych.2016.04.015
283. Borgan F, Laurikainen H, Veronese M, Marques TR, Haaparanta-Solin M, Solin O, et al. In Vivo Availability of Cannabinoid 1 Receptor Levels in Patients With First-Episode Psychosis. JAMA Psychiatry (2019). 76:1074–84. doi: 10.1001/jamapsychiatry.2019.1427
284. Leroy S, Griffon N, Bourdel MC, Olie JP, Poirier MF, Krebs MO. Schizophrenia and the cannabinoid receptor type 1 (CB1): association study using a single-base polymorphism in coding exon 1. Am J Med Genet (2001) 105:749–52. doi: 10.1002/ajmg.10038
285. Ujike H, Takaki M, Nakata K, Tanaka Y, Takeda T, Kodama M, et al. CNR1, central cannabinoid receptor gene, associated with susceptibility to hebephrenic schizophrenia. Mol Psychiatry (2002) 7:515–8. doi: 10.1038/sj.mp.4001029
286. Zammit S, Spurlock G, Williams H, Norton N, Williams N, O'Donovan MC, et al. Genotype effects of CHRNA7, CNR1 and COMT in schizophrenia: interactions with tobacco and cannabis use. Br J Psychiatry (2007) 191:402–7. doi: 10.1192/bjp.bp.107.036129
287. Seifert J, Ossege S, Emrich HM, Schneider U, Stuhrmann M. No association of CNR1 gene variations with susceptibility to schizophrenia. Neurosci Lett (2007) 426:29–33. doi: 10.1016/j.neulet.2007.08.008
288. Hamdani N, Tabeze JP, Ramoz N, Ades J, Hamon M, Sarfati Y, et al. The CNR1 gene as a pharmacogenetic factor for antipsychotics rather than a susceptibility gene for schizophrenia. Eur Neuropsychopharmacol (2008) 18:34–40. doi: 10.1016/j.euroneuro.2007.05.005
289. Bae JS, Kim JY, Park BL, Kim JH, Kim B, Park CS, et al. Genetic association analysis of CNR1 and CNR2 polymorphisms with schizophrenia in a Korean population. Psychiatr Genet (2014) 24:225–9. doi: 10.1097/YPG.0000000000000047
290. Costa M, Squassina A, Congiu D, Chillotti C, Niola P, Galderisi S, et al. Investigation of endocannabinoid system genes suggests association between peroxisome proliferator activator receptor-alpha gene (PPARA) and schizophrenia. Eur Neuropsychopharmacol (2013) 23:749–59. doi: 10.1016/j.euroneuro.2012.07.007
291. Tsai SJ, Wang YC, Hong CJ. Association study of a cannabinoid receptor gene (CNR1) polymorphism and schizophrenia. Psychiatr Genet (2000) 10:149–51. doi: 10.1097/00041444-200010030-00008
292. Suarez-Pinilla P, Roiz-Santianez R, Ortiz-Garcia de la Foz V, Guest PC, Ayesa-Arriola R, Cordova-Palomera A, et al. Brain structural and clinical changes after first episode psychosis: Focus on cannabinoid receptor 1 polymorphisms. Psychiatry Res (2015) 233:112–9. doi: 10.1016/j.pscychresns.2015.05.005
293. Rojnic Kuzman M, Bosnjak Kuharic D, Ganoci L, Makaric P, Kekin I, Rossini Gajsak L, et al. Association of CNR1 genotypes with changes in neurocognitive performance after eighteen-month treatment in patients with first-episode psychosis. Eur Psychiatry (2019) 61:88–96. doi: 10.1016/j.eurpsy.2019.07.004
294. Yu W, De Hert M, Moons T, Claes SJ, Correll CU, van Winkel R. CNR1 gene and risk of the metabolic syndrome in patients with schizophrenia. J Clin Psychopharmacol (2013) 33:186–92. doi: 10.1097/JCP.0b013e318283925e
295. De Marchi N, De Petrocellis L, Orlando P, Daniele F, Fezza F, Di Marzo V. Endocannabinoid signalling in the blood of patients with schizophrenia. Lipids Health Dis (2003) 2:5. doi: 10.1186/1476-511X-2-5
296. Ferretjans R, de Campos SM, Ribeiro-Santos R, Guimaraes FC, de Oliveira K, Cardoso AC, et al. Cognitive performance and peripheral endocannabinoid system receptor expression in schizophrenia. Schizophr Res (2014) 156:254–60. doi: 10.1016/j.schres.2014.04.028
297. de Campos-Carli SM, Araujo MS, de Oliveira Silveira AC, de Rezende VB, Rocha NP, Ferretjans R, et al. Cannabinoid receptors on peripheral leukocytes from patients with schizophrenia: Evidence for defective immunomodulatory mechanisms. J Psychiatr Res (2017) 87:44–52. doi: 10.1016/j.jpsychires.2016.12.001
298. Chase KA, Feiner B, Rosen C, Gavin DP, Sharma RP. Characterization of peripheral cannabinoid receptor expression and clinical correlates in schizophrenia. Psychiatry Res (2016) 245:346–53.doi: 10.1016/j.psychres.2016.08.055
299. D'Addario C, Micale V, Di Bartolomeo M, Stark T, Pucci M, Sulcova A, et al. A preliminary study of endocannabinoid system regulation in psychosis: Distinct alterations of CNR1 promoter DNA methylation in patients with schizophrenia. Schizophr Res (2017) 188:132–40. doi: 10.1016/j.schres.2017.01.022
300. Leweke FM, Giuffrida A, Wurster U, Emrich HM, Piomelli D. Elevated endogenous cannabinoids in schizophrenia. Neuroreport (1999) 10:1665–9. doi: 10.1097/00001756-199906030-00008
301. Giuffrida A, Leweke FM, Gerth CW, Schreiber D, Koethe D, Faulhaber J, et al. Cerebrospinal anandamide levels are elevated in acute schizophrenia and are inversely correlated with psychotic symptoms. Neuropsychopharmacology (2004) 29:2108–14. doi: 10.1038/sj.npp.1300558
302. Leweke FM, Giuffrida A, Koethe D, Schreiber D, Nolden BM, Kranaster L, et al. Anandamide levels in cerebrospinal fluid of first-episode schizophrenic patients: impact of cannabis use. Schizophr Res (2007) 94:29–36. doi: 10.1016/j.schres.2007.04.025
303. Koethe D, Pahlisch F, Hellmich M, Rohleder C, Mueller JK, Meyer-Lindenberg A, et al. Familial abnormalities of endocannabinoid signaling in schizophrenia. World J Biol Psychiatry (2019) 20:117–25. doi: 10.1080/15622975.2018.1449966
304. Potvin S, Kouassi E, Lipp O, Bouchard RH, Roy MA, Demers MF, et al. Endogenous cannabinoids in patients with schizophrenia and substance use disorder during quetiapine therapy. J Psychopharmacol (2008) 22:262–9. doi: 10.1177/0269881107083816
305. Desfosses J, Stip E, Bentaleb LA, Lipp O, Chiasson JP, Furtos A, et al. Plasma Endocannabinoid Alterations in Individuals with Substance Use Disorder are Dependent on the “Mirror Effect” of Schizophrenia. Front Psychiatry (2012) 3:85. doi: 10.3389/fpsyt.2012.00085
306. Muguruza C, Lehtonen M, Aaltonen N, Morentin B, Meana JJ, Callado LF. Quantification of endocannabinoids in postmortem brain of schizophrenic subjects. Schizophr Res (2013) 148:145–50. doi: 10.1016/j.schres.2013.06.013
307. Morita Y, Ujike H, Tanaka Y, Uchida N, Nomura A, Ohtani K, et al. A nonsynonymous polymorphism in the human fatty acid amide hydrolase gene did not associate with either methamphetamine dependence or schizophrenia. Neurosci Lett (2005) 376:182–7. doi: 10.1016/j.neulet.2004.11.050
308. Bioque M, Cabrera B, Garcia-Bueno B, Mac-Dowell KS, Torrent C, Saiz PA, et al. Dysregulated peripheral endocannabinoid system signaling is associated with cognitive deficits in first-episode psychosis. J Psychiatr Res (2016) 75:14–21. doi: 10.1016/j.jpsychires.2016.01.002
309. Volk DW, Siegel BI, Verrico CD, Lewis DA. Endocannabinoid metabolism in the prefrontal cortex in schizophrenia. Schizophr Res (2013) 147:53–7. doi: 10.1016/j.schres.2013.02.038
310. Bioque M, Garcia-Bueno B, Macdowell KS, Meseguer A, Saiz PA, Parellada M, et al. Peripheral endocannabinoid system dysregulation in first-episode psychosis. Neuropsychopharmacology (2013) 38:2568–77. doi: 10.1038/npp.2013.165
311. Tong D, He S, Wang L, Jin L, Si P, Cheng X. Association of single-nucleotide polymorphisms in the cannabinoid receptor 2 gene with schizophrenia in the Han Chinese population. J Mol Neurosci (2013) 51:454–60. doi: 10.1007/s12031-013-0062-0
312. Arjmand S, Behzadi M, Kohlmeier KA, Mazhari S, Sabahi A, Shabani M. Bipolar disorder and the endocannabinoid system. Acta Neuropsychiatr (2019) 31:193–201. doi: 10.1017/neu.2019.21
313. Ashton CH, Moore PB. Endocannabinoid system dysfunction in mood and related disorders. Acta Psychiatr Scand (2011) 124:250–61. doi: 10.1111/j.1600-0447.2011.01687.x
314. Alpak G, Copoglu S, Geyik E, Unal A, Igci M, Igci Y, et al. Rs6454674, Rs806368 and Rs1049353 CNR1 Gene Polymorphisms in Turkish Bipolar Disorder Patients: A Preliminary Study. Dis Mol Med (2014) 2:4. doi: 10.5455/dmm.20140428011918
315. Tsai SJ, Wang YC, Hong CJ. Association study between cannabinoid receptor gene (CNR1) and pathogenesis and psychotic symptoms of mood disorders. Am J Med Genet (2001) 105:219–21. doi: 10.1002/ajmg.1259
316. Pisanu C, Congiu D, Costa M, Sestu M, Chillotti C, Ardau R, et al. No association of endocannabinoid genes with bipolar disorder or lithium response in a Sardinian sample. Psychiatry Res (2013) 210:887–90. doi: 10.1016/j.psychres.2013.09.025
317. Minocci D, Massei J, Martino A, Milianti M, Piz L, Di Bello D, et al. Genetic association between bipolar disorder and 524A>C (Leu133Ile) polymorphism of CNR2 gene, encoding for CB2 cannabinoid receptor. J Affect Disord (2011) 134:427–30. doi: 10.1016/j.jad.2011.05.023
318. Legge SE, Jones HJ, Kendall KM, Pardinas AF, Menzies G, Bracher-Smith M, et al. Association of Genetic Liability to Psychotic Experiences With Neuropsychotic Disorders and Traits. JAMA Psychiatry (2019) 76:1256–65. doi: 10.1001/jamapsychiatry.2019.2508
319. Berardi A, Schelling G, Campolongo P. The endocannabinoid system and Post Traumatic Stress Disorder (PTSD): From preclinical findings to innovative therapeutic approaches in clinical settings. Pharmacol Res (2016) 111:668–78. doi: 10.1016/j.phrs.2016.07.024
320. Bassir Nia A, Bender R, Harpaz-Rotem I. Endocannabinoid System Alterations in Posttraumatic Stress Disorder: A Review of Developmental and Accumulative Effects of Trauma. Chronic Stress (Thousand Oaks) (2019) 3: 1–23. doi: 10.1177/2470547019864096
321. Shoshan N, Akirav I. The effects of cannabinoid receptors activation and glucocorticoid receptors deactivation in the amygdala and hippocampus on the consolidation of a traumatic event. Neurobiol Learn Mem (2017) 144:248–58. doi: 10.1016/j.nlm.2017.08.004
322. Campos AC, Ferreira FR, da Silva WA Jr., Guimaraes FS. Predator threat stress promotes long lasting anxiety-like behaviors and modulates synaptophysin and CB1 receptors expression in brain areas associated with PTSD symptoms. Neurosci Lett (2013) 533:34–8. doi: 10.1016/j.neulet.2012.11.016
323. Xing G, Carlton J, Zhang L, Jiang X, Fullerton C, Li H, et al. Cannabinoid receptor expression and phosphorylation are differentially regulated between male and female cerebellum and brain stem after repeated stress: implication for PTSD and drug abuse. Neurosci Lett (2011) 502:5–9. doi: 10.1016/j.neulet.2011.05.013
324. Fride E, Suris R, Weidenfeld J, Mechoulam R. Differential response to acute and repeated stress in cannabinoid CB1 receptor knockout newborn and adult mice. Behav Pharmacol (2005) 16:431–40. doi: 10.1097/00008877-200509000-00016
325. Bowers ME, Ressler KJ. Interaction between the cholecystokinin and endogenous cannabinoid systems in cued fear expression and extinction retention. Neuropsychopharmacology (2015) 40:688–700. doi: 10.1038/npp.2014.225
326. Korem N, Akirav I. Cannabinoids prevent the effects of a footshock followed by situational reminders on emotional processing. Neuropsychopharmacology (2014) 39:2709–22. doi: 10.1038/npp.2014.132
327. Abush H, Akirav I. Cannabinoids modulate hippocampal memory and plasticity. Hippocampus (2010) 20:1126–38. doi: 10.1002/hipo.20711
328. Ganon-Elazar E, Akirav I. Cannabinoids prevent the development of behavioral and endocrine alterations in a rat model of intense stress. Neuropsychopharmacology (2012) 37:456–66. doi: 10.1038/npp.2011.204
329. Ganon-Elazar E, Akirav I. Cannabinoids and traumatic stress modulation of contextual fear extinction and GR expression in the amygdala-hippocampal-prefrontal circuit. Psychoneuroendocrinology (2013) 38:1675–87. doi: 10.1016/j.psyneuen.2013.01.014
330. Korem N, Lange R, Hillard CJ, Akirav I. Role of beta-catenin and endocannabinoids in the nucleus accumbens in extinction in rats exposed to shock and reminders. Neuroscience (2017) 357:285–94. doi: 10.1016/j.neuroscience.2017.06.015
331. Goodman J, Packard MG. Peripheral and intra-dorsolateral striatum injections of the cannabinoid receptor agonist WIN 55,212-2 impair consolidation of stimulus-response memory. Neuroscience (2014) 274:128–37. doi: 10.1016/j.neuroscience.2014.05.007
332. Reich CG, Iskander AN, Weiss MS. Cannabinoid modulation of chronic mild stress-induced selective enhancement of trace fear conditioning in adolescent rats. J Psychopharmacol (2013) 27:947–55. doi: 10.1177/0269881113499207
333. Chhatwal JP, Davis M, Maguschak KA, Ressler KJ. Enhancing cannabinoid neurotransmission augments the extinction of conditioned fear. Neuropsychopharmacology (2005) 30:516–24. doi: 10.1038/sj.npp.1300655
334. Danandeh A, Vozella V, Lim J, Oveisi F, Ramirez GL, Mears D, et al. Effects of fatty acid amide hydrolase inhibitor URB597 in a rat model of trauma-induced long-term anxiety. Psychopharmacol (Berl) (2018) 235:3211–21. doi: 10.1007/s00213-018-5020-7
335. Fidelman S, Mizrachi Zer-Aviv T, Lange R, Hillard CJ, Akirav I. Chronic treatment with URB597 ameliorates post-stress symptoms in a rat model of PTSD. Eur Neuropsychopharmacol (2018) 28:630–42. doi: 10.1016/j.euroneuro.2018.02.004
336. Maymon N, Mizrachi Zer-Aviv T, Sabban EL, Akirav I, Neuropeptide Y. and cannabinoids interaction in the amygdala after exposure to shock and reminders model of PTSD. Neuropharmacology (2020) 162:107804. doi: 10.1016/j.neuropharm.2019.107804
337. Segev A, Korem N, Mizrachi Zer-Aviv T, Abush H, Lange R, Sauber G, et al. Role of endocannabinoids in the hippocampus and amygdala in emotional memory and plasticity. Neuropsychopharmacology (2018) 43:2017–27. doi: 10.1038/s41386-018-0135-4
338. Varvel SA, Wise LE, Niyuhire F, Cravatt BF, Lichtman AH. Inhibition of fatty-acid amide hydrolase accelerates acquisition and extinction rates in a spatial memory task. Neuropsychopharmacology (2007) 32:1032–41. doi: 10.1038/sj.npp.1301224
339. Mota N, Sumner JA, Lowe SR, Neumeister A, Uddin M, Aiello AE, et al. The rs1049353 polymorphism in the CNR1 gene interacts with childhood abuse to predict posttraumatic threat symptoms. J Clin Psychiatry (2015) 76:e1622–3. doi: 10.4088/JCP.15l10084
340. Lu AT, Ogdie MN, Jarvelin MR, Moilanen IK, Loo SK, McCracken JT, et al. Association of the cannabinoid receptor gene (CNR1) with ADHD and post-traumatic stress disorder. Am J Med Genet B Neuropsychiatr Genet (2008) 147B:1488–94. doi: 10.1002/ajmg.b.30693
341. Hill MN, Bierer LM, Makotkine I, Golier JA, Galea S, McEwen BS, et al. Reductions in circulating endocannabinoid levels in individuals with post-traumatic stress disorder following exposure to the World Trade Center attacks. Psychoneuroendocrinology (2013) 38:2952–61. doi: 10.1016/j.psyneuen.2013.08.004
342. Neumeister A, Normandin MD, Pietrzak RH, Piomelli D, Zheng MQ, Gujarro-Anton A, et al. Elevated brain cannabinoid CB1 receptor availability in post-traumatic stress disorder: a positron emission tomography study. Mol Psychiatry (2013) 18:1034–40. doi: 10.1038/mp.2013.61
343. Wilker S, Pfeiffer A, Elbert T, Ovuga E, Karabatsiakis A, Krumbholz A, et al. Endocannabinoid concentrations in hair are associated with PTSD symptom severity. Psychoneuroendocrinology (2016) 67:198–206. doi: 10.1016/j.psyneuen.2016.02.010
344. Hariri AR, Gorka A, Hyde LW, Kimak M, Halder I, Ducci F, et al. Divergent effects of genetic variation in endocannabinoid signaling on human threat- and reward-related brain function. Biol Psychiatry (2009) 66:9–16. doi: 10.1016/j.biopsych.2008.10.047
345. Gunduz-Cinar O, MacPherson KP, Cinar R, Gamble-George J, Sugden K, Williams B, et al. Convergent translational evidence of a role for anandamide in amygdala-mediated fear extinction, threat processing and stress-reactivity. Mol Psychiatry (2013) 18:813–23. doi: 10.1038/mp.2012.72
346. Dincheva I, Drysdale AT, Hartley CA, Johnson DC, Jing D, King EC, et al. FAAH genetic variation enhances fronto-amygdala function in mouse and human. Nat Commun (2015) 6:6395. doi: 10.1038/ncomms7395
347. Rabinak CA, Angstadt M, Sripada CS, Abelson JL, Liberzon I, Milad MR, et al. Cannabinoid facilitation of fear extinction memory recall in humans. Neuropharmacology (2013) 64:396–402. doi: 10.1016/j.neuropharm.2012.06.063
348. Rabinak CA, Angstadt M, Lyons M, Mori S, Milad MR, Liberzon I, et al. Cannabinoid modulation of prefrontal-limbic activation during fear extinction learning and recall in humans. Neurobiol Learn Mem (2014) 113:125–34. doi: 10.1016/j.nlm.2013.09.009
349. Roitman P, Mechoulam R, Cooper-Kazaz R, Shalev A. Preliminary, open-label, pilot study of add-on oral Delta9-tetrahydrocannabinol in chronic post-traumatic stress disorder. Clin Drug Invest (2014) 34:587–91. doi: 10.1007/s40261-014-0212-3
350. Jetly R, Heber A, Fraser G, Boisvert D. The efficacy of nabilone, a synthetic cannabinoid, in the treatment of PTSD-associated nightmares: A preliminary randomized, double-blind, placebo-controlled cross-over design study. Psychoneuroendocrinology (2015) 51:585–8. doi: 10.1016/j.psyneuen.2014.11.002
351. Cameron C, Watson D, Robinson J. Use of a synthetic cannabinoid in a correctional population for posttraumatic stress disorder-related insomnia and nightmares, chronic pain, harm reduction, and other indications: a retrospective evaluation. J Clin Psychopharmacol (2014) 34:559–64. doi: 10.1097/JCP.0000000000000180
352. Leffa DT, Ferreira SG, Machado NJ, Souza CM, Rosa FD, de Carvalho C, et al. Caffeine and cannabinoid receptors modulate impulsive behavior in an animal model of attentional deficit and hyperactivity disorder. Eur J Neurosci (2019) 49:1673–83. doi: 10.1111/ejn.14348
353. Haspula D, Clark MA. Heterologous regulation of the cannabinoid type 1 receptor by angiotensin II in astrocytes of spontaneously hypertensive rats. J Neurochem (2016) 139:523–36. doi: 10.1111/jnc.13776
354. Grissom NM, Herdt CT, Desilets J, Lidsky-Everson J, Reyes TM. Dissociable deficits of executive function caused by gestational adversity are linked to specific transcriptional changes in the prefrontal cortex. Neuropsychopharmacology (2015) 40:1353–63. doi: 10.1038/npp.2014.313
355. Van Lieshout RJ, Taylor VH, Boyle MH. Pre-pregnancy and pregnancy obesity and neurodevelopmental outcomes in offspring: a systematic review. Obes Rev (2011) 12:e548–59. doi: 10.1111/j.1467-789X.2010.00850.x
356. Kleijn J, Wiskerke J, Cremers TI, Schoffelmeer AN, Westerink BH, Pattij T. Effects of amphetamine on dopamine release in the rat nucleus accumbens shell region depend on cannabinoid CB1 receptor activation. Neurochem Int (2012) 60:791–8. doi: 10.1016/j.neuint.2012.03.002
357. Buchmann AF, Hohm E, Witt SH, Blomeyer D, Jennen-Steinmetz C, Schmidt MH, et al. Role of CNR1 polymorphisms in moderating the effects of psychosocial adversity on impulsivity in adolescents. J Neural Transm (Vienna) (2015) 122:455–63. doi: 10.1007/s00702-014-1266-3
358. Ponce G, Hoenicka J, Rubio G, Ampuero I, Jimenez-Arriero MA, Rodriguez-Jimenez R, et al. Association between cannabinoid receptor gene (CNR1) and childhood attention deficit/hyperactivity disorder in Spanish male alcoholic patients. Mol Psychiatry (2003) 8:466–7. doi: 10.1038/sj.mp.4001278
359. Bermudez-Silva FJ, Cardinal P, Cota D. The role of the endocannabinoid system in the neuroendocrine regulation of energy balance. J Psychopharmacol (2012) 26:114–24. doi: 10.1177/0269881111408458
360. Quarta C, Mazza R, Obici S, Pasquali R, Pagotto U. Energy balance regulation by endocannabinoids at central and peripheral levels. Trends Mol Med (2011) 17:518–26. doi: 10.1016/j.molmed.2011.05.002
361. Rorato R, Miyahara C, Antunes-Rodrigues J, Elias LL. Tolerance to hypophagia induced by prolonged treatment with a CB1 antagonist is related to the reversion of anorexigenic neuropeptide gene expression in the hypothalamus. Regul Pept (2013) 182:12–8. doi: 10.1016/j.regpep.2012.12.004
362. Verty AN, Boon WM, Mallet PE, McGregor IS, Oldfield BJ. Involvement of hypothalamic peptides in the anorectic action of the CB receptor antagonist rimonabant (SR 141716). Eur J Neurosci (2009) 29:2207–16. doi: 10.1111/j.1460-9568.2009.06750.x
363. Lage R, Parisi C, Seoane-Collazo P, Ferno J, Mazza R, Bosch F, et al. Lack of Hypophagia in CB1 Null Mice is Associated to Decreased Hypothalamic POMC and CART Expression. Int J Neuropsychopharmacol (2015) 18: pyv011. doi: 10.1093/ijnp/pyv011
364. Moore CF, Schlain GS, Mancino S, Sabino V, Cottone P. A behavioral and pharmacological characterization of palatable diet alternation in mice. Pharmacol Biochem Behav (2017) 163:1–8. doi: 10.1016/j.pbb.2017.10.013
365. Riedel G, Fadda P, McKillop-Smith S, Pertwee RG, Platt B, Robinson L. Synthetic and plant-derived cannabinoid receptor antagonists show hypophagic properties in fasted and non-fasted mice. Br J Pharmacol (2009) 156:1154–66. doi: 10.1111/j.1476-5381.2008.00107.x
366. Wiley JL, Burston JJ, Leggett DC, Alekseeva OO, Razdan RK, Mahadevan A, et al. CB1 cannabinoid receptor-mediated modulation of food intake in mice. Br J Pharmacol (2005) 145:293–300. doi: 10.1038/sj.bjp.0706157
367. Sofia RD, Knobloch LC. Comparative effects of various naturally occurring cannabinoids on food, sucrose and water consumption by rats. Pharmacol Biochem Behav (1976) 4:591–9. doi: 10.1016/0091-3057(76)90202-1
368. Wierucka-Rybak M, Wolak M, Bojanowska E. The effects of leptin in combination with a cannabinoid receptor 1 antagonist, AM 251, or cannabidiol on food intake and body weight in rats fed a high-fat or a free-choice high sugar diet. J Physiol Pharmacol (2014) 65:487–96.
369. Collu R, Scherma M, Piscitelli F, Giunti E, Satta V, Castelli MP, et al. Impaired brain endocannabinoid tone in the activity-based model of anorexia nervosa. Int J Eat Disord (2019) 52:1251–62. doi: 10.1002/eat.23157
370. Monteleone P, Matias I, Martiadis V, De Petrocellis L, Maj M, Di Marzo V. Blood levels of the endocannabinoid anandamide are increased in anorexia nervosa and in binge-eating disorder, but not in bulimia nervosa. Neuropsychopharmacology (2005) 30:1216–21. doi: 10.1038/sj.npp.1300695
371. Gerard N, Pieters G, Goffin K, Bormans G, Van Laere K. Brain type 1 cannabinoid receptor availability in patients with anorexia and bulimia nervosa. Biol Psychiatry (2011) 70:777–84. doi: 10.1016/j.biopsych.2011.05.010
372. Schroeder M, Eberlein C, de Zwaan M, Kornhuber J, Bleich S, Frieling H. Lower levels of cannabinoid 1 receptor mRNA in female eating disorder patients: association with wrist cutting as impulsive self-injurious behavior. Psychoneuroendocrinology (2012) 37:2032–6. doi: 10.1016/j.psyneuen.2012.03.025
373. Dider S, Ji J, Zhao Z, Xie L. Molecular mechanisms involved in the side effects of fatty acid amide hydrolase inhibitors: a structural phenomics approach to proteome-wide cellular off-target deconvolution and disease association. NPJ Syst Biol Appl (2016) 2:16023. doi: 10.1038/npjsba.2016.23
374. Toczek M, Malinowska B. Enhanced endocannabinoid tone as a potential target of pharmacotherapy. Life Sci (2018) 204:20–45. doi: 10.1016/j.lfs.2018.04.054
375. Rubino T, Parolaro D. Sexually dimorphic effects of cannabinoid compounds on emotion and cognition. Front Behav Neurosci (2011) 5:64. doi: 10.3389/fnbeh.2011.00064
376. Green T, Flash S, Reiss AL. Sex differences in psychiatric disorders: what we can learn from sex chromosome aneuploidies. Neuropsychopharmacology (2019) 44:9–21. doi: 10.1038/s41386-018-0153-2
Keywords: endocannabinoid system, cannabinoid receptor (CB1r, CB2r), endocannabinoid, biomarker, sychiatry, diagnosis, treatment
Citation: Navarrete F, García-Gutiérrez MS, Jurado-Barba R, Rubio G, Gasparyan A, Austrich-Olivares A and Manzanares J (2020) Endocannabinoid System Components as Potential Biomarkers in Psychiatry. Front. Psychiatry 11:315. doi: 10.3389/fpsyt.2020.00315
Received: 15 January 2020; Accepted: 30 March 2020;
Published: 27 April 2020.
Edited by:
Helge Frieling, Hannover Medical School, GermanyReviewed by:
Kirsten R. Müller-Vahl, Hannover Medical School, GermanyCopyright © 2020 Navarrete, García-Gutiérrez, Jurado-Barba, Rubio, Gasparyan, Austrich-Olivares and Manzanares. This is an open-access article distributed under the terms of the Creative Commons Attribution License (CC BY). The use, distribution or reproduction in other forums is permitted, provided the original author(s) and the copyright owner(s) are credited and that the original publication in this journal is cited, in accordance with accepted academic practice. No use, distribution or reproduction is permitted which does not comply with these terms.
*Correspondence: Jorge Manzanares, am1hbnphbmFyZXNAdW1oLmVz
Disclaimer: All claims expressed in this article are solely those of the authors and do not necessarily represent those of their affiliated organizations, or those of the publisher, the editors and the reviewers. Any product that may be evaluated in this article or claim that may be made by its manufacturer is not guaranteed or endorsed by the publisher.
Research integrity at Frontiers
Learn more about the work of our research integrity team to safeguard the quality of each article we publish.