- 1Department of Psychiatry, School of Medicine, University of Maryland, Baltimore, MD, United States
- 2Department of Pharmacology, School of Medicine, University of Maryland, Baltimore, MD, United States
- 3Department of Anatomy & Neurobiology, School of Medicine, University of Maryland, Baltimore, MD, United States
- 4Veterans Affairs Maryland Health Care System, Baltimore, MD, United States
Fluctuating hormone levels, such as estradiol might underlie the difference in the prevalence of psychiatric disorders observed in women vs. men. Estradiol exert its effects primarily through binding on the two classical estrogen receptor subtypes, alpha (ERα) and beta (ERβ). Both receptors have been suggested to a have role in the development of psychiatric disorders, however, most of the current literature is limited to their role in females. We investigated the role of estrogen receptors on cognition (novel-object recognition), anxiety (open-field test, elevated-plus maze, and light/dark box), stress-responsive behaviors (forced-swim test, learned helplessness following inescapable shock, and sucrose preference), pre-pulse inhibition (PPI) and amphetamine-induced hyperlocomotion in both male and female mice either lacking the ERα or ERβ receptor. We found that female Esr1−/− mice have attenuated pre-pulse inhibition, whereas female Esr2−/− mice manifested enhanced pre-pulse inhibition. No pre-pulse inhibition difference was observed in male Esr1−/− and Esr2−/− mice. Moreover, amphetamine-induced hyperlocomotion was decreased in male Esr1−/−, but not Esr2−/− mice, while female Esr1−/− and Esr2−/− mice showed an enhanced response. Genetic absence of ERα did not alter the escape capability or sucrose preference following inescapable shock in both male and female mice. In contrast, female, but not male Esr2−/− mice, manifested decreased escape failures compared with controls. Lack of Esr2 gene in male mice was associated with decreased sucrose preference following inescapable shock, suggesting susceptibility for development of anhedonia following stress. No sucrose preference differences were found in female Esr2−/− mice following inescapable shock stress. Lastly, we demonstrated that lack of Esr1 or Esr2 genes had no effect on memory and anxiety-like behaviors in both male and female mice. Our findings indicate a differential sex-specific involvement of estrogen receptors in the development of stress-mediated maladaptive behaviors as well as psychomotor activation responses suggesting that these receptors might act as potential treatment targets in a sex-specific manner.
Introduction
Mental disorders are extremely common, affecting approximately 18.3% of the U.S. adult population (see 2016 National Survey on Drug Use and Health). The prevalence of many mental disorders, including anxiety and depressive disorders, are higher among women than men (see 2016 National Survey on Drug Use and Health). These gender differences have been attributed, at least partly, to fluctuations of the ovarian hormone estradiol [see (1)]. Specifically, an increase in estradiol occurring during female puberty has been associated with increased prevalence of mood disorders [see (2, 3)]. Additionally, several studies showed that the incidence of depression (4–6) and anxiety (7) increases in women during the menopausal transition, a period that is characterized by robust fluctuations in estrogen levels, before overall levels drop to approximately 10% of estrogen levels during the pre-menopausal period. Although estradiol treatment was shown to alleviate depressive symptoms in women (8, 9), the mechanistic relationship between estrogen and depression remains unclear.
Estradiol exerts its effects through binding to two classical estrogen receptor subtypes, the estrogen receptor alpha (ERα) and beta (ERβ) and the non-classical G-protein coupled estrogen receptor, GPR30 (10). These receptors have been suggested to play a role in the pathophysiology of mood disorders. Specifically, Esr1 gene variants, which code for ERα, have been associated with increased risk of developing depression in women (11–15). In rats, estradiol, via acting at the ERα, normalized postpartum-induced anxiety- and depressive-like behavior, measured in the elevated-plus maze (EPM) and the forced-swim tests (FST), respectively (16). Moreover, knockdown of the ERα selectively in the posterior-dorsal amygdala of female mice decreased anxiety-like behavior as demonstrated by the increase time spend in the light compartment of the light/dark box (L/D box) (17), suggesting a possible role of ERα in regulating anxiety behaviors. Although, the role of ERα has been extensively investigated in women and female animals, its role in male depression and anxiety has received limited attention. However, a genetic association study identified a possible link between Esr1 polymorphisms and depression in men (18).
Polymorphisms in Esr2, which codes for ERβ have been associated with moderate depressive symptoms in women (18), whereas there are no studies investigating the role of Esr2 polymorphisms in male depression. In rodent studies, selective ERβ ligands (19), as well as estradiol (20) decrease immobility time in the FST in wild-type, but not Esr2 knockout female mice. In addition, ERβ, but not ERα, agonists decreased immobility time in the FST in ovariectomized rats (21), suggesting that activation of ERβ induces antidepressant effects in female rodents. There is a report suggesting antidepressant efficacy of an ERβ agonist in the tail-suspension test in male mice (22). Administration of the ERβ agonist diarylpropionitrile decreased anxiety-like behaviors in female wild-type mice but not in mice lacking the ERβ receptor gene (23).
Overall, most of the existing studies that have been published and investigated the role of the estrogen receptors in anxiety and depressive behaviors mainly concentrate on a single sex, as estradiol is considered a “female” hormone. Furthermore, limited studies assessed for the involvement of either ERβ or ERα in behavioral responses to stress. Therefore, in the present study, we sought to understand the role of estrogen receptors in anxiety, as well as in depressive-related behavioral responses following stress in both female and male mice.
Methods
Mice
Esr1 and Esr2 breeding pairs were obtained from Jackson laboratories. Wild-type, heterozygous and homozygous Esr1 mice were bred in-house by breeding heterozygous males and females. Heterozygous and homozygous Esr2 mice were bred in-house by breeding heterozygous females and homozygous males. Both Esr1 and Esr2 mice were bred on a C57BL/6J background. At the time of behavioral testing, the age of the animals was 8–12 weeks. Mice were grouped-housed and maintained under a 12 h light–dark cycle (lights on at 7:00 a.m.). Water and food was available ad libitum. All mice were housed in the same room in individually ventilated cages. All experimental procedures were approved by the University of Maryland Animal Care and Use Committee and were conducted in full accordance with the NIH Guide for the Care and Use of Laboratory Animals. Tail samples were obtained prior to weaning and genotyped by TransnetYX, Inc. (Cordova, TN, USA). The primer sequences are as follows:
Esr1 genotype: Wild-type-Forward: TCGGGCATCGCCTACG; Reverse: GGCGACACGCTGTTGAG. Esr1-Forward: CATTCTCAGTATTGTTTTGCCAAGTTCT; Reverse: GGCGACACGCTGTTGAG
Esr2 genotype: Wild-type-Forward: CCAAGAGGGATGCTCACTTCT: Reverse: CAGACACCGTAATGATACCCAGATG. Esr2-Forward: GCCAAGAGGGATGCTCACTTC; Reverse: TCCATCAGAAGCTGACTCTAGAACT.
Behavioral Characterization
All behavioral experiments were performed during the light phase of the light/dark cycle between 10:00 a.m. and 3:00 p.m. The order of testing within animals (Figure 1A) was determined by the degree of stressfulness of each test with the least stressful conducted first and the most potentially distressing test last (24–26). The numbers of animals used for the open-field test, light/dark box, elevated-plus maze, novel-object recognition, forced-swim test, learned helplessness, shock sensitivity and sucrose preference are as follows—Esr1+/+, Esr1+/−, Esr1−/− Females: n = 12, 20, 11; Males: n = 8, 12, 9 and Esr2+/−, Esr2−/− Females: n = 9, 8; Males: n = 13, 11. The numbers of animals used for the pre-pulse inhibition and amphetamine-induced hyperlocomotion are as follows—Esr1+/+, Esr1+/−, Esr1−/− Females: n = 11, 11, 10; Males: n = 10, 10, 8 and Esr2+/−, Esr2−/− Females: n = 11, 9; Males: n = 7, 8.
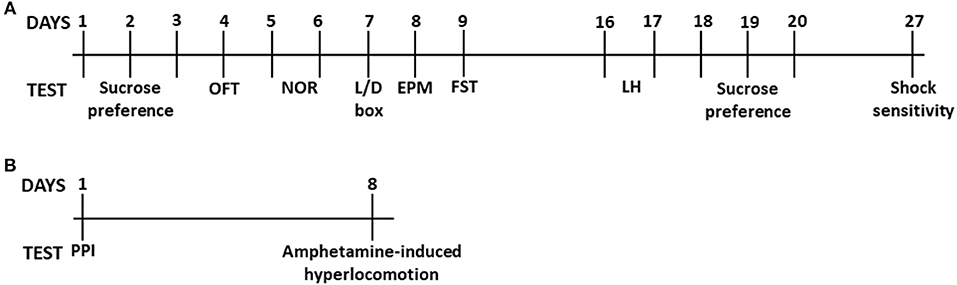
Figure 1. Timeline of behavioral experiments. Timeline of (A) the sucrose preference, open-field test, novel-object recognition, light/dark box, elevated-plus maze, forced-swim test, learned helplessness and shock sensitivity. Timeline of (B) pre-pulse inhibition and amphetamine-induced hyperlocomotion.
Open-Field Test (OFT)
The OFT was performed under 300 Lux white lighting. Mice were individually placed into open-field arenas (100 × 100 × 38 cm; San Diego Instruments, San Diego, CA) for a 10-min period. The sessions were recorded using an overhead, digital video-camera. Distanced traveled and time spent in the center of the arena was analyzed using TopScan v2.0 (CleverSys, Inc., Reston VA).
Light/Dark Box (L/D box)
The L/D box was used as previously described (26), with minor modifications. Briefly, mice were placed in the illuminated compartment of the L/D box (35 × 35 cm), facing the wall opposite to the dark compartment, and allowed to explore the whole apparatus for 5 min. The sessions were recorded using a video-camera and the time spent in the illuminated and dark compartment was scored using TopScan v2.0 (CleverSys, Inc., Reston VA).
Elevated-Plus Maze (EPM)
The EPM was carried in dim white lighting conditions (~5 lux). The apparatus consisted of 2 closed arms and 2 open arms (39 × 5 cm each) and was elevated 50 cm above the floor (Stoelting, Woodale, IL). The experiment was carried out as previously described (27). The time spent in the open and closed arms of the EPM during the 5-min test was recorded by an over-head digital video-camera and scored using TopScan v2.0 (CleverSys, Inc., Reston VA). Amount of time spent in the open arms was used as the primary outcome for the anxiety behavioral assessment.
Novel-Object Recognition (NOR)
Short-term recognition memory was assessed using the novel object recognition task protocol, as previously described (28, 29). The NOR was carried in dim white lighting conditions (~10–15 lux). The apparatus and objects used here has been previously described by Zanos et al. (28). The test was conducted over two days. On the first day, the habituation phase, the animals were allowed to explore an empty novel object recognition apparatus (40 × 9 × 23 cm) for 30 min and then returned to their home cages. On the second day, the mice were re-introduced into the same apparatus, but this time containing two identical objects fixed on the floor, which they were allowed to explore for 30 min. After this familiarization phase, mice were immediately returned to their home-cages for 30 min. The mice were then placed back into the novel object recognition apparatus, in which one of the “familiar” objects was replaced by a “novel” object (retention phase) for 4 min. All three phases of the novel object recognition test were recorded via an overhead video-camera and analyzed using TopScan v2.0 automated scoring software (CleverSys, Inc., Reston VA). The time spent interacting with the familiar and novel objects during the retention phase was measured. A discrimination ratio was calculated by dividing the time of interaction with the novel object by the total time of interaction with both objects during the retention phase.
Forced-Swim Test (FST)
The FST was performed in normal white light conditions (~300 lux) and was performed as previously described (30). Briefly, mice were subjected to a 6-min swim session in clear Plexiglas cylinders (30-cm height × 20-cm diameter) filled with 15 cm of water (23 ± 1°C). Sessions were recorded using a digital video camera. Immobility time, defined as passive floating with no additional activity other than that necessary to keep the animal's head above water, was scored for the last 4 min of the 6-min test by a trained experimenter blind to the genotypes.
Learned Helplessness
The learned helplessness paradigm was performed in accordance with Dao et al. (31) and was separated in two phases: training and test. On Day 1 mice received inescapable shock training (0.3 mA, 2 s shock duration 120 trials, inter-trial interval 15 s) in one compartment of the two-compartment Coulbourn Mouse Shuttle Cage (Coulbourn Instruments, Whitehall, PA). On day 2, learned helplessness test consisted of 45 escapable shock trials (0.3 mA, duration of open door: 15 s). The average inter-trial interval was 20 s. In trials 1–5, the gate opened concomitantly with the shock initiation and stayed open for the duration of the shock. In trials 6–45, the gate with a 3 s delay after initiation of the shock. In all trials, shock was terminated if mice passed through the gate to the other compartment. Number of escape failures and escape latency was automatically measured by GraphicState 3.01 (Coulbourn Instruments, Whitehall, PA).
Shock Sensitivity Test
Mice were tested for their sensitivity to shock as previously described with minor modifications (32). Briefly, mice were subjected to increment shock intensities (0.02–0.50 mA; 0.02 mA increments from 0.02 to 0.2 mA and 0.5 mA increments from 0.2 to 0.5 mA) and testing for their flinch response. The increments occurred every 30 s and the shock delivery were automatically controlled by GraphicState 3.01 (Coulbourn Instruments, Whitehall, PA). Scoring was performed live by an experienced experimenter blind to the genotypes.
Sucrose Preference Test
For assessing the baseline sucrose preference, mice were singly housed for 72 h and presented with two identical bottles containing either tap water or 1% sucrose solution. After baseline sucrose measurement, mice were re-group housed. Following the learned helplessness testing, similar to the baseline measurements, mice were singly housed for 72 h and presented with two identical bottles containing either tap water or 1% sucrose solution. The location of the sucrose and tap water bottle was changed every day to avoid the development of side preference.
Pre-pulse Inhibition (PPI)
The pre-pulse inhibition (PPI) paradigm was performed as previously described (33), with minor modifications. Mice were individually tested in acoustic startle boxes (SR-LAB; San Diego Instruments, San Diego, CA). The animals were placed in the startle chamber for a 30-min habituation period. The experiment started with a further 5-min adaptation period during which the mice were exposed to a constant background noise (67 db), followed by five initial startle stimuli (120 db, 40-ms duration each). Subsequently, animals were exposed to five different trial types: pulse alone trials (120 db, 40-ms duration), three prepulse trials of 76, 81, and 86 db of white noise bursts (20-ms duration) preceding a 120-db pulse by 100 ms, and background (67 db) no-stimuli trials. Each of these trials was randomly presented five times. The percentage PPI was calculated using the following formula: [(magnitude on pulse alone trial—magnitude on prepulse + pulse trial)/magnitude on pulse alone trial] × 100.
Amphetamine-Induced Hyperlocomotion
The amphetamine-induced hyperlocomotion experiment was performed under white lighting conditions of ~80 lux. Mice were placed into the open-field arenas (50 × 50 × 38 cm; San Diego Instruments) for a 30-min habituation period, as described in the OFT protocol above. Following the habituation period, the locomotion response to a saline injection (5 ml/kg, i.p.) was assessed for 30-min. After that, mice were administered d-amphetamine (2 mg/kg, i.p.; Sigma-Aldrich, St. Louis, MO) and placed back to the arena for 60-min to assess their locomotion response. Distanced traveled and time spent in the center of the arena was analyzed using TopScan v2.0 (CleverSys, Inc., Reston VA).
All the behavioral assessments were performed by an experimenter blind to the genotype of animals. The OFT, novel object recognition, L/D box, EPM, FST, learned helplessness, sucrose preference and shock sensitivity were performed on the same animals starting from the least to the most stressful test (for timeline see Figure 1A). There was a 7-day gap period between the FST and learned helplessness and a 7-day gap between sucrose preference post-stress and shock sensitivity, when mice remained undisturbed in their home cages. The PPI and amphetamine-induced hyperlocomotion was performed on the same animals with at least a 7-day gap between these tests (for timeline see Figure 1B).
Statistical Analysis
The OFT, L/D box, EPM, novel object recognition, FST, and acoustic startle as well as, the total escape failures in the learned helplessness test with the wild-type, heterozygous and homozygous Esr1 mice were analyzed using one-way ANOVA. The OFT, L/D box, EPM, novel object recognition, FST, and acoustic startle as well as, the total escape failures in the learned helplessness test with the heterozygous and homozygous Esr2 mice were analyzed with an unpaired Student's t-test. The average escape latency, escape failures, sucrose preference, pre-pulse inhibition and amphetamine-induced hyperlocomotion for both Esr1 and Esr2 were analyzed with a repeated measure two-way ANOVA. Datasets that fail to pass normality test as tested by Shapiro-Wilk test, were analyzed by Kruskal-Wallis and Mann-Whitney test. Fisher's exact test was used as an additional analysis for % pre-pulse inhibition to test whether the proportion of mice decreased their PPI was significantly different between genotypes. The male and female mice experiments were performed in separate cohorts, and thus are not combined on the same graphs and statistical analyses. Holm-Sidak post-hoc test was performed when a significant interaction effect was observed in the ANOVAs. Statistical significance was set at p < 0.05. All analyses were performed using GraphPad Prism v 6.01. All values are expressed as mean ± SEM. Statistical details are summarized in Table 1.
Results
Effects of Esr1 and Esr2 Genes on Anxiety-Related Behaviors in Male and Female Mice
Open-Field Test
We first assessed OFT behavior in male and female heterozygous (Esr1+/−) and homozygous (Esr1−/−) Esr1 mice, as well as their littermate wild-type controls (Esr1+/+). No difference was observed in the total distance traveled between the groups in both male (Figure 2A) and female mice (Figure 2K). Similarly, no difference was observed in the time-spent in the center of the open field arena in male (Figure 2B) and female mice (Figure 2L).
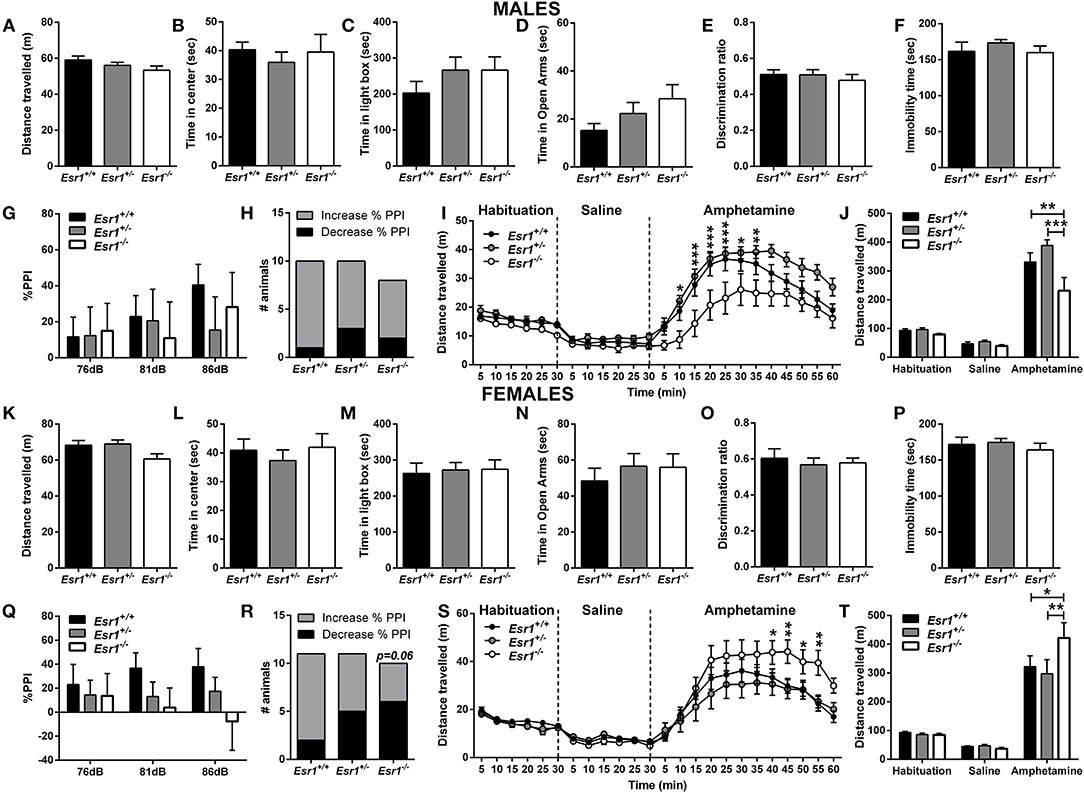
Figure 2. Baseline behavioral characterization of male and female Esr1+/+, Esr1+/− and Esr1−/− mice. In male mice, no effect of genotype was observed between in (A,B) the open-field test, (C) light/dark box, (D) elevated-plus maze, (E) novel-object recognition, (F) forced-swim test, (G) pre-pulse inhibition and (H) contingency representation of pre-pulse inhibition. (I,J) An attenuated amphetamine response was observed in the male Esr1−/− mice compared with Esr1+/+ and Esr1+/− mice. In female mice, no genotype difference was observed in (K,L) the open-field test, (M) light/dark box, (N) elevated-plus maze, (O) novel-object recognition, (P) forced-swim test, (Q) pre-pulse inhibition and (R) contingency representation of pre-pulse inhibition. (S,T) An enhanced amphetamine response was observed in the male Esr1−/− mice compared with Esr1+/+ and Esr1+/− mice. *p < 0.05, **p < 0.01, ***p < 0.001; n = 8, 12, 9 for (A–F), n = 10, 10, 8 for (G–J), n = 12, 20, 11 for (K–P), n = 11, 11, 10 for (Q–T).
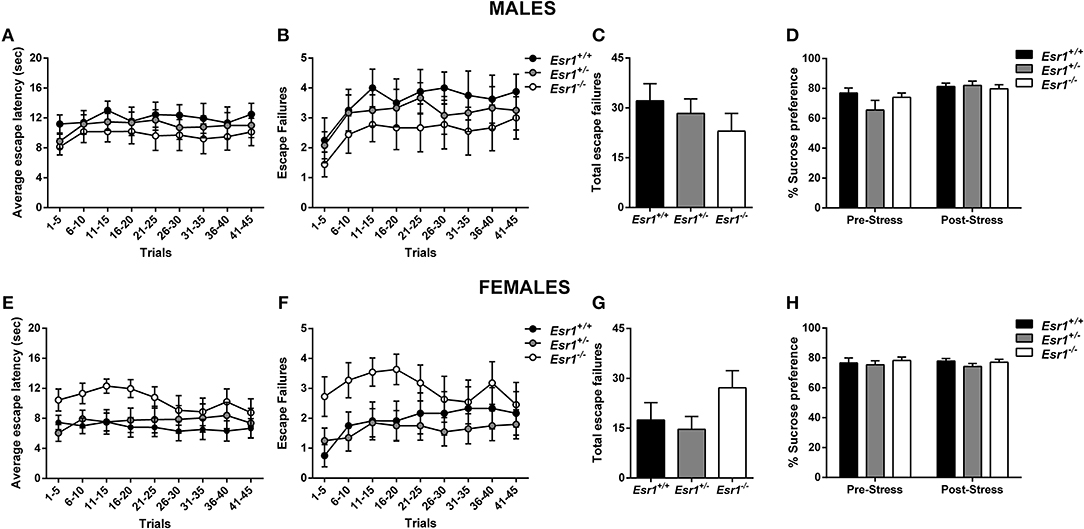
Figure 3. Effects of stress in male and female Esr1+/+, Esr1+/− and Esr1−/− mice. In male mice no effect of genotype was observed following inescapable shock training in (A) escape latency, (B,C) escape failures and (D) sucrose preference. In female mice no effect of genotype was observed following inescapable schok training in (E) escape latency (F,G) escape failures and (H) sucrose preference. n = 8, 12, 9 for (A–D), n = 12, 20, 11 for (E–H).
Moreover, OFT behavior was assessed in male and female heterozygous (Esr2+/−) and homozygous (Esr2−/−) Esr2 mice. No difference was observed in the total distance traveled between the genotype groups in both male (Figure 4A) and female mice (Figure 4K). Similarly, no difference was observed in the time-spent in the center of the open field arena in male (Figure 4B) and female mice (Figure 4L).
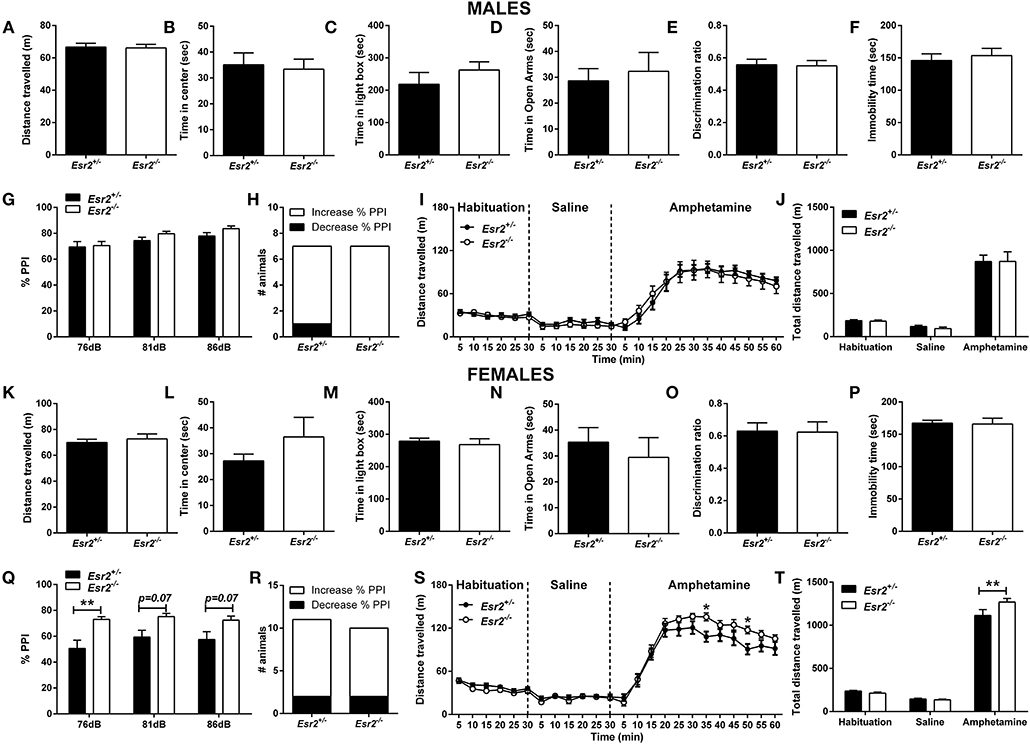
Figure 4. Baseline behavioral characterization of male and female Esr2+/− and Esr2−/− mice. No difference was observed between the different genotypes in male mice in (A,B) the open-field test, (C) light/dark box, (D) elevated-plus maze, (E) novel-object recognition, (F) forced-swim test, (G) pre-pulse inhibition, (H) contingency representation of pre-pulse inhibition and (I,J) amphetamine-induced hyperlocomotion. No difference was observed between the different genotypes in female mice in (K,L) the open-field test, (M) light/dark box, (N) elevated-plus maze, (O) novel-object recognition and (P) forced-swim test. (Q) Female Esr2−/− mice showed higher pre-pulse inhibition compared with Esr2+/− mice. (R) No difference was observed in contingency representation of pre-pulse inhibition. (S,T) An enhanced amphetamine response was observed in the male Esr2−/− mice compared with Esr2+/− mice. *p < 0.05, **p < 0.01; n = 13, 11 for (A–F), n = 7, 8 for (G–J), n = 9, 8 for (K–P), n = 11, 9 for (Q–T).
Light/Dark Box
We measured the time that mice choose to spend in the brightly illuminated area of the L/D box. No effect of Esr1 knocked-down (neither Esr1+/− or Esr1−/−) was observed in the in male (Figure 2C) or female (Figure 2M) mice compared with their wild-type littermates.
In addition, both male (Figure 4C) and female (Figure 4M) Esr2−/− and Esr2+/− mice spent similar time in the illuminated area of the L/D box.
Elevated-Plus Maze
We measured the time mice choose to spend in the open arms of the EPM. No difference was observed between the different genotypes (Esr1+/+, Esr1+/−, or Esr1−/−) in male (Figure 2D) and female (Figure 2N) mice.
Also, no difference was observed between the different genotypes in male (Figure 4D) and female (Figure 4N) Esr2 mice.
Effects of Esr1 and Esr2 Deletion on Novel Object Memory in Male and Female Mice
Short-term recognition memory was assessed using the novel object recognition test. Neither male (Figure 2E) or female (Figure 2O) Esr1−/− mice manifest object recognition impairment, since there was no difference in the novel object recognition discrimination ratio compared with the wild-type controls.
Moreover, both male (Figure 4E) and female (Figure 4O) Esr2 mice did not show any genotype dependent object recognition impairment as assessed with a novel object recognition discrimination ratio.
Effect of Esr1 and Esr2 Deletion on Sensorimotor Gating in Male and Female Mice
Sensorimotor gating deficits were assessed using the PPI paradigm. No statistically significant difference was observed in either male (Figures 2G,H) or female (Figures 2Q,R) Esr1−/− mice in the % PPI. However, Fisher's exact test revealed a near significant difference between female Esr1+/+ and Esr1−/− (Figure 2R). No difference was observed between the genotypes in the startle amplitude (Male mice-Esr1+/+: 250.8 ± 53.14, Esr1+/−: 235.9 ± 74.03, Esr1−/−: 270.5 ± 59.86; Female mice-Esr1+/+: 156.0 ± 30.29, Esr1+/−: 148.9 ± 32.97, Esr1−/−: 265.2 ± 63.61).
No difference was observed in male Esr2−/− mice in the % PPI (Figures 4G,H) and in the startle amplitude (Esr2+/−: 231.1 ± 36.26, Esr2−/−: 205.3 ± 26.94) compared with their littermate controls. However, an increase in the % PPI was observed in female Esr2−/− compared with Esr2+/− mice (Figure 4Q). No difference was observed in the contingency analysis (Figure 4R) and startle response (Esr2+/−: 197.3 ± 30.16, Esr2−/−: 166.6 ± 34.57).
Effect of Esr1 and Esr2 Deletion on d-Amphetamine-Induced Hyperlocomotion in Male and Female Mice
An increase in locomotor activity was observed in both male [F(46, 667) = 2.866, p < 0.001; Figure 2I- F(4, 58) = 5.628, p < 0.001; Figure 2J] and female mice [F(46, 667) = 2.552, p < 0.001; Figure 2S- F(4, 58) = 2.259, p = 0.074; Figure 2T] from all the genotypes. However, male Esr1−/− mice showed a lower response to d-amphetamine as indicated by the lower distance traveled compared with Esr1+/+ (p < 0.001) and Esr1+/− (p < 0.01) mice (Figures 2I,J). In contrast, female Esr1−/− mice had a greater response to the d-amphetamine compared with Esr1+/+ and Esr1+/− mice (Figures 2S,T).
Following administration of d-amphetamine, an increase in locomotor activity was observed in both male (Figures 4I,J) and female mice (Figures 4S,T) from all the genotypes. No difference was observed in the d-amphetamine-induced hyperlocomotion between male Esr2+/− and Esr2−/− mice (Figures 4I,J). In contrast, female Esr2−/− mice had a greater locomotor response to d-amphetamine as shown by the higher distance traveled compared with Esr2+/− mice [F(23, 299) = 2.367, p < 0.001; Figure 4S- F(2, 26) = 4.787, p = 0.017; Figure 4T].
Effects of Esr1 and Esr2 Deletion on Behavioral Despair and Anhedonia in Male and Female Mice
Forced-Swim Test
Behavioral despair was assessed using the FST in male and female Esr1+/− and Esr1−/− mice, as well as their littermate wild-type controls. Under baseline conditions, no effect of the deletion of Esr1 was observed in the FST in either male (Figure 2E) or female mice (Figure 2P).
No difference was also observed between Esr2+/− and Esr2−/− in the immobility time in male (Figure 4F) and female mice (Figure 4P).
Learned Helplessness
Development of helpless behavior was tested following inescapable shock. No difference in the escape latency (Figure 3A), and escape failures (Figures 3B,C) was identified between male Esr1+/−, or Esr1−/− mice compared with their littermate wild-type controls. In agreement, there was no difference in escape latency (Figure 3E) and escape failures (Figures 3F,G) between Esr1−/−, Esr1+/− and Esr1+/+ mice. The learned helplessness response was not affected by differences in shock perception since no differences were observed in the flinch response in the shock sensitivity test by either male (Esr1+/+: 0.045 ± 0.005, Esr1+/−: 0.037 ± 0.028, Esr1−/−: 0.055 ± 0.027) or female (Esr1+/+: 0.047 ± 0.006, Esr1+/−: 0.054 ± 0.026, Esr1−/−: 0.038 ± 0.023) mice.
No difference in the escape latency (Figure 5A), and escape failures (Figures 5B,C) between the male Esr2+/− and Esr2−/−; while female Esr2−/− mice had lower escape latency [F(8, 112) = 2.116, p = 0.04; Figure 5E] and lower total escape failures [t(14) = 2.274, p = 0.039; Figure 5G] compared with Esr2+/− mice. Although, there was a statistical significance difference in the average escape latency and total escape failures, the breakdown of escape failures does not reach statistical significance (Figure 5F). The learned helplessness response was not affected by any differences in shock perception since no differences were observed in the flinch response in the shock sensitivity test by both male (Esr2+/−: 0.057 ± 0.007, Esr2−/−: 0.062 ± 0.007) and female (Esr2+/−: 0.091 ± 0.005, Esr2−/−: 0.090 ± 0.007) mice.
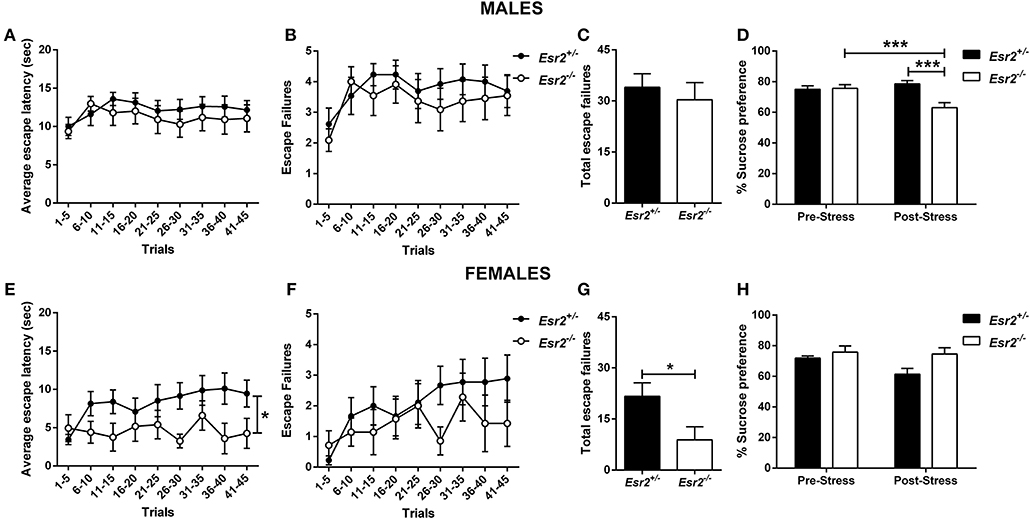
Figure 5. Effects of stress in male and female Esr2+/− and Esr2−/− mice. No difference was observed between the different genotypes following inescapable shock training in male mice in the (A) escape latency and (B,C) escape failures. (D) Male Esr2−/− mice decreased their sucrose preference following stress compared with their baseline and Esr2+/− mice. Female Esr2−/− mice demonstrated decreased (E) escape latency. Although no statistically significant difference was observed in the (F) escape failures timeline, (G) female Esr2−/− mice had lower total escape failures compared with Esr2+/− mice. (H) No difference was observed in the pre- and post-stress sucrose preference. *p < 0.05, ***p < 0.001; n = 13, 11 for (A–D), n = 9, 8 for (E–H).
Sucrose Preference
The development of anhedonia was tested at baseline (stress-naïve) conditions and following footshock stress (as described in the learned helplessness experiment) in male and female Esr1+/− and Esr1−/− mice, as well as wild-type control mice. No differences were observed between the different genotypes at baseline sucrose preference following stress in either male (Figure 3D) or female mice (Figure 3H).
In addition, the development of anhedonia was tested at baseline and following the learned helplessness procedure in male and female Esr2+/− and Esr2−/− mice. Although no difference was observed in sucrose preference prior to learned helplessness, following stress, male Esr2−/− mice decreased their sucrose preference compared with controls as well as compared with their pre-stress sucrose preference [F(1, 22) = 17.63, p < 0.001; Figure 5D]. No difference was observed in female Esr2−/− and Esr2+/− mice was observed both before and after stress (Figure 5H).
Discussion
In the present study, we demonstrate that the lack of ERα and ERβ are differentially involved in the development of helplessness and anhedonia in male and female mice following stress. To our knowledge, this is the first study to investigate the effects ERα and ERβ using knockout mice in the development of helplessness, i.e., increase in escape failures following inescapable shock training, as well as the development of anhedonia following stress in both male and female mice. We demonstrate that female Esr2−/− mice manifested significantly lower escape failures in the learned helplessness test, as well as an overall higher sucrose preference prior and after the learned helplessness stress compared with the heterozygous controls suggesting that deletion of Esr2 gene might be beneficial against the development of maladaptive behaviors following stress. Our finding is not in line with previous findings that administration of ERβ agonists in ovariectomized female rats decrease immobility time in the FST (21, 34). The different approaches used to investigate the role of ERβ in responses to stress between the aforementioned and present study might account for these differences. For example, here we investigated the development of helplessness following the exposure of mice to stress (inescapable shock), whereas the previously published reports used the FST in stress-naïve rats as a measure of antidepressant efficacy of ERβ agonists. Moreover, our use of intact mice vs. the use of ovariectomized rats in this earlier study might also contribute to these differences.
While we demonstrate that deletion of Esr2 gene in female mice has a protective effect against the development of helplessness, this is not the case in male Esr2−/− mice, which had similar escape failures compared to the Esr2+/− mice. However, we observed a decrease in sucrose preference following inescapable shock stress in male Esr2−/− compared with Esr2+/− control mice, suggesting that Esr2−/− male mice are more susceptible to stress-induced anhedonia. To our knowledge this is the first study to demonstrate a role of Esr2 gene in male mice in the development of stress-induced anhedonia, a core symptom of depression in humans, and further investigation is warranted to identify the specific role of this receptor in male depression.
In contrast with the effects of Esr2 deletion, following inescapable shock, we did not observe any statistically significant differences in either male or female Esr1−/− mice compared with either wild-type and heterozygous littermates. Moreover, neither male nor female Esr1−/− mice showed any anhedonia symptoms as measured by sucrose preference prior or after the inescapable shock stress suggesting that Esr1 is not substantially involved in the development of these behavioral responses. These conclusions are also in agreement with our finding that both male and female Esr1−/− mice do not manifest any differences in the FST compared with their wild-type controls. It has been recently reported that ERα in the nucleus accumbens drives a pro-resilient phenotype in both male and female mice (35). This is in apparent contrast with our findings demonstrating that lack of Esr1−/− does not induce susceptibility to develop learned helplessness or result in changes in sucrose preference following stress in either sex. It may be that while increased ERa driven transcription mediates resilience, lack of the gene does not modulate susceptibility.
Although we demonstrated differential effects of both estrogen receptors in male and female mice in response to stress, we did not observe any effects of genetic deletion of estrogen receptors in anxiety-related tests. In line with our findings, Krezel et al. (36) demonstrated that both male and female Esr1−/− mice had similar thigmotaxis and spent similar time in open arms of the EPM compared with wild-type mice. While, our findings in male Esr2−/− mice are also in agreement with other reports (36, 37), their finding that female Esr2−/− mice have higher anxiety-like behaviors compared with wild-type mice, as measured by the EPM and OFT, are in contrast with the results presented here. In line with our findings, other reports demonstrated that female Esr2−/− have similar performance on anxiety behavioral tests as wild-type mice (23, 38). Interestingly, Walf et al. (38) also demonstrated that female wild-type as well as in Esr2−/− mice during proestrous compared with diestrous had higher open field-central entries, which was interpreted as an anxiolytic effect. Therefore, future studies should address this limitation and further investigate the effects of the estrous cycle and estrogen receptors in anxiety-like behaviors.
Moreover, another limitation of the current study is the use of heterozygous mice for Esr2 instead of wild-type as controls. This might be particularly important when negative results are obtained, such as for the anxiety-related behaviors, since heterozygous mice might have different phenotypes than wild-types. However, it was previously shown that Esr2+/− behave in a similar manner as wild-type mice in the elevated plus-mice (39), a test assessing anxiety-like behaviors. Considering this, and the fact that our findings are also in agreement with other studies (20, 36, 37, 40) provides confidence to our results. However, for this reason the negative results comparing Esr2+/− to Esr2−/− should be interpreted cautiously as a full wild-type control was not included in the experimental design. In addition, since we are using conventional knockout mice, the absence of differences in these behaviors could be due to compensatory mechanisms.
Sex differences have been reported in patients with major depression, with male, but not female, patients demonstrating decreased PPI compared with healthy controls (41, 42). In order to test if estrogen receptors might be implicated in these sex differences, we assessed male and female Esr1 and Esr2 knockout mice in the PPI paradigm. Although we observed no significant difference with the male Esr1−/− and Esr2−/− mice, deletion of estrogen receptors in female mice exerted differential effects. Specifically, contingency analysis demonstrated a near significant decrease in % PPI in Esr1−/− compared with Esr1+/+. This may be related to the finding that a decrease in % PPI is observed in rodents following ovariectomy, an effect that is normalized with estradiol replacement (43, 44); thus, considering our results we postulate that this decrease is attributed to the actions of estradiol through ERα. In contrast with the effects of Esr1 deletion, female Esr2−/− mice manifested higher PPI than their littermate controls suggesting an enhanced sensorimotor gating response. In combination with our findings that deletion of Esr2 gene in female mice results in decreased escape failures, and the literature demonstrating stress-induced decreases in PPI (45–47), these results suggest that deletion of Esr2 gene in females might result in stress resilience. Enhanced PPI in this case might be also associated with an improved ability of these mice to deal with information processing. Moreover, considering the literature supporting a protective effect of estradiol in women with schizophrenia [see (48)], our findings cannot preclude that the differential regulation of PPI response in Esr1 and Esr2 knockout female mice might have a functional relevance to schizophrenia. However, further investigation is warranted for better understanding this finding and the possible implications of estrogen receptors in stress resilience and/or schizophrenia.
Furthermore, we observed that male Esr1−/− mice showed attenuated response to amphetamine, as measured by hyperlocomotion, compared with Esr1+/− and Esr1+/+, suggesting a possible interaction between Esr1−/− and the dopaminergic signaling. The possible interaction between Esr1 and the dopaminergic system is also supported by the findings that male mice lacking Esr1 have decreased tyrosine hydroxylase (TH) mRNA and protein levels in the midbrain (49), which might also explain our findings that male Esr1−/− mice manifest attenuated response to amphetamine-induced hyperlocomotion compared with WT mice. A possible interaction between these two systems is further supported by our findings in female mice; however, in this instance lack of Esr1 in females enhanced the amphetamine-induced hyperlocomotion compared with controls. A decrease in TH mRNA and protein levels in the midbrain was also observed in female mice lacking Esr1−/− (49). These differences in the amphetamine-induced locomotion in Esr1−/− mice could be related to this finding, or could be influenced by differences in their hormonal status compared with WT mice. Both male and female Esr1−/− mice display highly atrophied reproductive organs and are infertile (50–53) and female Esr1−/− mice are anovulatory and acyclic (54, 55). Considering that gonadal hormones can affect dopamine response [see (56–58)], the difference in the hormonal status of these mice could contribute to the observed differences in amphetamine-induced hyperlocomotion. An enhancement of amphetamine-induced hyperlocomotion was also observed in female Esr2 knockout mice compared with their respective controls, whereas no difference was observed in male Esr2−/− mice. Female Esr2−/− have been reported to have similar levels of TH immunoreactive cells in the midbrain compared to WT mice (59), though this does not preclude differences in dopamine neuron activity or release. Interestingly, ERα and ERβ agonists have been shown to reverse the amphetamine-induced disruption of PPI (60), which further supports a role of these receptors in modulating response to amphetamine. In addition, estradiol is known to modulate several dopamine-related behaviors such as sexual motivation (61), as well as to increase the rewarding effects of d-amphetamine (62, 63); however, the exact role of estrogen receptors needs to be further investigated.
Overall, we demonstrate that deletion of either Esr1 or Esr2 differentially affects the development of stress-related responses as well as psychomotor responses in male and female mice. Specifically, deletion of Esr2 in male mice led to increased susceptibility for the development of stress-related maladaptive behaviors, whereas deletion of Esr2 in female mice resulted in resilience against the development of such behaviors. Also the amphetamine locomotor response was attenuated in male Esr1−/− mice, while female Esr1−/− and Esr2−/− showed enhanced response. The present findings suggest that differential manipulation of Esr1 and Esr2 in males and females might have potential applications for the treatment of mood disorders.
Author Contributions
PG and TG designed the experiment. PG, PZ, and CJ performed the experiments presented in this manuscript. PG analyzed all the results included in this manuscript. PG and TG drafted the paper. PZ and CJ critically reviewed the manuscript.
Conflict of Interest Statement
The authors declare that the research was conducted in the absence of any commercial or financial relationships that could be construed as a potential conflict of interest.
Acknowledgments
This work was supported by National Institute of Health grant MH100700 to TG. This material is the result of work in part supported with resources and the use of facilities at the VA Maryland Health Care System, Baltimore, Maryland. The contents do not represent the views of the U.S. Department of Veterans Affairs or the United States Government.
References
1. Wharton W, Gleason CE, Olson SR, Carlsson CM, Asthana S. Neurobiological underpinnings of the estrogen - mood relationship. Curr Psychiatry Rev. (2012) 8:247–56. doi: 10.2174/157340012800792957
2. Altemus M, Sarvaiya N, Neill Epperson C. Sex differences in anxiety and depression clinical perspectives. Front Neuroendocrinol. (2014) 35:320–30. doi: 10.1016/j.yfrne.2014.05.004
3. Balzer BW, Duke SA, Hawke CI, Steinbeck KS. The effects of estradiol on mood and behavior in human female adolescents: a systematic review. Eur J Pediatr. (2015) 174:289–98. doi: 10.1007/s00431-014-2475-3
4. Matthews KA. Myths and realities of the menopause. Psychosom Med. (1992) 54:1–9. doi: 10.1097/00006842-199201000-00001
5. Hardy R, Kuh D. Change in psychological and vasomotor symptom reporting during the menopause. Soc Sci Med. (2002) 55:1975–88. doi: 10.1016/S0277-9536(01)00326-4
6. Woods NF, Mariella A, Mitchell ES. Patterns of depressed mood across the menopausal transition: approaches to studying patterns in longitudinal data. Acta Obstet Gynecol Scand. (2002) 81:623–32. doi: 10.1034/j.1600-0412.2002.810708.x
7. Paoletti AM, Floris S, Mannias M, Orru M, Crippa D, Orlandi R, et al. Evidence that cyproterone acetate improves psychological symptoms and enhances the activity of the dopaminergic system in postmenopause. J Clin Endocrinol Metab. (2001) 86:608–12. doi: 10.1210/jcem.86.2.7179
8. Schmidt PJ, Nieman L, Danaceau MA, Tobin MB, Roca CA, Murphy JH, et al. Estrogen replacement in perimenopause-related depression: a preliminary report. Am J Obstet Gynecol. (2000) 183:414–20. doi: 10.1067/mob.2000.106004
9. Soares CN, Almeida OP, Joffe H, Cohen LS. Efficacy of estradiol for the treatment of depressive disorders in perimenopausal women: a double-blind, randomized, placebo-controlled trial. Arch Gen Psychiatry (2001) 58:529–34. doi: 10.1001/archpsyc.58.6.529
10. Yasar P, Ayaz G, User SD, Gupur G, Muyan M. Molecular mechanism of estrogen-estrogen receptor signaling. Reprod Med Biol. (2017) 16:4–20. doi: 10.1002/rmb2.12006
11. Mill J, Kiss E, Baji I, Kapornai K, Daroczy G, Vetro A, et al. Association study of the estrogen receptor alpha gene (ESR1) and childhood-onset mood disorders. Am J Med Genet B Neuropsychiatr Genet. (2008) 147B:1323–6. doi: 10.1002/ajmg.b.30751
12. Kim JJ, Pae CU, Kim MR, Min JA, Kim KH, Lee CU, et al. Association between estrogen receptor gene polymorphisms and depression in post-menopausal women: a preliminary study. Psychiatry Investig. (2010) 7:224–7. doi: 10.4306/pi.2010.7.3.224
13. Ryan J, Scali J, Carriere I, Peres K, Rouaud O, Scarabin PY, et al. Oestrogen receptor polymorphisms and late-life depression. Br J Psychiatry (2011) 199:126–31. doi: 10.1192/bjp.bp.111.091751
14. Ryan J, Scali J, Carriere I, Peres K, Rouaud O, Scarabin PY, et al. Estrogen receptor alpha gene variants and major depressive episodes. J Affect Disord. (2012) 136:1222–6. doi: 10.1016/j.jad.2011.10.010
15. Pinsonneault JK, Sullivan D, Sadee W, Soares CN, Hampson E, Steiner M. Association study of the estrogen receptor gene ESR1 with postpartum depression–a pilot study. Arch Womens Ment Health (2013) 16:499–509. doi: 10.1007/s00737-013-0373-8
16. Furuta M, Numakawa T, Chiba S, Ninomiya M, Kajiyama Y, Adachi N, et al. Estrogen, predominantly via estrogen receptor alpha, attenuates postpartum-induced anxiety- and depression-like behaviors in female rats. Endocrinology (2013) 154:3807–16. doi: 10.1210/en.2012-2136
17. Spiteri T, Musatov S, Ogawa S, Ribeiro A, Pfaff DW, Agmo A. The role of the estrogen receptor alpha in the medial amygdala and ventromedial nucleus of the hypothalamus in social recognition, anxiety and aggression. Behav Brain Res. (2010) 210:211–20. doi: 10.1016/j.bbr.2010.02.033
18. Ryan J, Ancelin ML. Polymorphisms of estrogen receptors and risk of depression: therapeutic implications. Drugs (2012) 72:1725–38. doi: 10.2165/11635960-000000000-00000
19. Clark JA, Alves S, Gundlah C, Rocha B, Birzin ET, Cai SJ, et al. Selective estrogen receptor-beta (SERM-beta) compounds modulate raphe nuclei tryptophan hydroxylase-1 (TPH-1) mRNA expression and cause antidepressant-like effects in the forced swim test. Neuropharmacology (2012) 63:1051–63. doi: 10.1016/j.neuropharm.2012.07.004
20. Rocha BA, Fleischer R, Schaeffer JM, Rohrer SP, Hickey GJ. 17 Beta-estradiol-induced antidepressant-like effect in the forced swim test is absent in estrogen receptor-beta knockout (BERKO) mice. Psychopharmacology (2005) 179:637–43. doi: 10.1007/s00213-004-2078-1
21. Walf AA, Rhodes ME, Frye CA. Antidepressant effects of ERbeta-selective estrogen receptor modulators in the forced swim test. Pharmacol Biochem Behav. (2004) 78:523–9. doi: 10.1016/j.pbb.2004.03.023
22. Hughes ZA, Liu F, Platt BJ, Dwyer JM, Pulicicchio CM, Zhang G, et al. WAY-200070, a selective agonist of estrogen receptor beta as a potential novel anxiolytic/antidepressant agent. Neuropharmacology (2008) 54:1136–42. doi: 10.1016/j.neuropharm.2008.03.004
23. Walf AA, Koonce CJ, Frye CA. Estradiol or diarylpropionitrile decrease anxiety-like behavior of wildtype, but not estrogen receptor beta knockout, mice. Behav Neurosci. (2008) 122:974–81. doi: 10.1037/a0012749
24. Crawley JN, Paylor R. A proposed test battery and constellations of specific behavioral paradigms to investigate the behavioral phenotypes of transgenic and knockout mice. Horm Behav. (1997) 31:197–211. doi: 10.1006/hbeh.1997.1382
25. Crawley JN. What's Wrong With My Mouse? Behavioral Phenotyping of Transgenic and Knockout Mice. New York, NY: John Wiley & Sons, Inc., (2006).
26. Georgiou P, Zanos P, Hourani S, Kitchen I, Bailey A. Cocaine abstinence induces emotional impairment and brain region-specific upregulation of the oxytocin receptor binding. Eur J Neurosci. (2016) 44:2446–54. doi: 10.1111/ejn.13348
27. Georgiou P, Zanos P, Garcia-Carmona JA, Hourani S, Kitchen I, Laorden ML, et al. Methamphetamine abstinence induces changes in mu-opioid receptor, oxytocin and CRF systems: association with an anxiogenic phenotype. Neuropharmacology (2016) 105:520–32. doi: 10.1016/j.neuropharm.2016.02.012
28. Zanos P, Bhat S, Terrillion CE, Smith RJ, Tonelli LH, Gould TD. Sex-dependent modulation of age-related cognitive decline by the L-type calcium channel gene Cacna1c (Cav 1.2). Eur J Neurosci. (2015) 42:2499–507. doi: 10.1111/ejn.12952
29. Le NT, Chang L, Kovlyagina I, Georgiou P, Safren N, Braunstein KE, et al. Motor neuron disease, TDP-43 pathology, and memory deficits in mice expressing ALS-FTD-linked UBQLN2 mutations. Proc Natl Acad Sci USA. (2016) 113:E7580–9. doi: 10.1073/pnas.1608432113
30. Can A, Dao DT, Arad M, Terrillion CE, Piantadosi SC, Gould TD. The mouse forced swim test. J Vis Exp. (2012) 59:e3638. doi: 10.3791/3638
31. Dao DT, Mahon PB, Cai X, Kovacsics CE, Blackwell RA, Arad M, et al. Mood disorder susceptibility gene CACNA1C modifies mood-related behaviors in mice and interacts with sex to influence behavior in mice and diagnosis in humans. Biol Psychiatry (2010) 68:801–10. doi: 10.1016/j.biopsych.2010.06.019
32. Kovacsics CE, Gould TD. Shock-induced aggression in mice is modified by lithium. Pharmacol Biochem Behav. (2010) 94:380–6. doi: 10.1016/j.pbb.2009.09.020
33. Zanos P, Piantadosi SC, Wu HQ, Pribut HJ, Dell MJ, Can A, et al. The prodrug 4-chlorokynurenine causes ketamine-like antidepressant effects, but not side effects, by NMDA/GlycineB-site inhibition. J Pharmacol Exp Ther. (2015) 355:76–85. doi: 10.1124/jpet.115.225664
34. Weiser MJ, Wu TJ, Handa RJ. Estrogen receptor-beta agonist diarylpropionitrile: biological activities of R- and S-enantiomers on behavior and hormonal response to stress. Endocrinology (2009) 150:1817–25. doi: 10.1210/en.2008-1355
35. Lorsch ZS, Loh Y-HE, Purushothaman I, Walker DM, Parise EM, Salery M, et al. Estrogen receptor α drives pro-resilient transcription in mouse models of depression. Nature Commun. (2018) 9:1116. doi: 10.1038/s41467-018-03567-4
36. Krezel W, Dupont S, Krust A, Chambon P, Chapman PF. Increased anxiety and synaptic plasticity in estrogen receptor beta -deficient mice. Proc Natl Acad Sci USA. (2001) 98:12278–82. doi: 10.1073/pnas.221451898
37. Tsuda MC, Yamaguchi N, Nakata M, Ogawa S. Modification of female and male social behaviors in estrogen receptor beta knockout mice by neonatal maternal separation. Front Neurosci. (2014) 8:274. doi: 10.3389/fnins.2014.00274
38. Walf AA, Koonce C, Manley K, Frye CA. Proestrous compared to diestrous wildtype, but not estrogen receptor beta knockout, mice have better performance in the spontaneous alternation and object recognition tasks and reduced anxiety-like behavior in the elevated plus and mirror maze. Behav Brain Res. (2009) 196:254–60. doi: 10.1016/j.bbr.2008.09.016
39. Walf AA, Frye CA. A review and update of mechanisms of estrogen in the hippocampus and amygdala for anxiety and depression behavior. Neuropsychopharmacology (2006) 31:1097–111. doi: 10.1038/sj.npp.1301067
40. Oyola MG, Portillo W, Reyna A, Foradori CD, Kudwa A, Hinds L, et al. Anxiolytic effects and neuroanatomical targets of estrogen receptor-beta (ERbeta) activation by a selective ERbeta agonist in female mice. Endocrinology (2012) 153:837–46. doi: 10.1210/en.2011-1674
41. Matsuo J, Ota M, Hidese S, Hori H, Teraishi T, Ishida I, et al. Sexually dimorphic deficits of prepulse inhibition in patients with major depressive disorder and their relationship to symptoms: a large single ethnicity study. J Affect Disord. (2017) 211:75–82. doi: 10.1016/j.jad.2017.01.012
42. Matsuo J, Ota M, Hidese S, Teraishi T, Hori H, Ishida I, et al. Sensorimotor gating in depressed and euthymic patients with bipolar disorder: analysis on prepulse inhibition of acoustic startle response stratified by gender and state. Front Psychiatry (2018) 9:123. doi: 10.3389/fpsyt.2018.00123
43. Charitidi K, Meltser I, Canlon B. Estradiol treatment and hormonal fluctuations during the estrous cycle modulate the expression of estrogen receptors in the auditory system and the prepulse inhibition of acoustic startle response. Endocrinology (2012) 153:4412–21. doi: 10.1210/en.2012-1416
44. Sbisa A, Van Den Buuse M, Gogos A. The effect of estrogenic compounds on psychosis-like behaviour in female rats. PLoS ONE (2018) 13:e0193853. doi: 10.1371/journal.pone.0193853
45. Pijlman FT, Herremans AH, Van De Kieft J, Kruse CG, Van Ree JM. Behavioural changes after different stress paradigms: prepulse inhibition increased after physical, but not emotional stress. Eur Neuropsychopharmacol. (2003) 13:369–80. doi: 10.1016/S0924-977X(03)00040-3
46. Sutherland JE, Conti LH. Restraint stress-induced reduction in prepulse inhibition in Brown Norway rats: role of the CRF2 receptor. Neuropharmacology (2011) 60:561–71. doi: 10.1016/j.neuropharm.2010.12.022
47. De La Casa LG, Mena A, Ruiz-Salas JC. Effect of stress and attention on startle response and prepulse inhibition. Physiol Behav. (2016) 165:179–86. doi: 10.1016/j.physbeh.2016.07.022
48. Gogos A, Sbisa AM, Sun J, Gibbons A, Udawela M, Dean B. A role for estrogen in schizophrenia: clinical and preclinical findings. Int J Endocrinol. (2015) 2015:615356. doi: 10.1155/2015/615356
49. Kuppers E, Krust A, Chambon P, Beyer C. Functional alterations of the nigrostriatal dopamine system in estrogen receptor-alpha knockout (ERKO) mice. Psychoneuroendocrinology (2008) 33:832–8. doi: 10.1016/j.psyneuen.2008.03.007
50. Lubahn DB, Moyer JS, Golding TS, Couse JF, Korach KS, Smithies O. Alteration of reproductive function but not prenatal sexual development after insertional disruption of the mouse estrogen receptor gene. Proc Natl Acad Sci USA. (1993) 90:11162–6. doi: 10.1073/pnas.90.23.11162
51. Couse JF, Korach KS. Estrogen receptor null mice: what have we learned and where will they lead us? Endocr Rev. (1999) 20:358–417. doi: 10.1210/edrv.20.3.0370
52. Hewitt SC, Korach KS. Estrogen receptors: structure, mechanisms and function. Rev Endocr Metab Disord. (2002) 3:193–200. doi: 10.1023/A:1020068224909
53. Couse JF, Yates MM, Walker VR, Korach KS. Characterization of the hypothalamic-pituitary-gonadal axis in estrogen receptor (ER) Null mice reveals hypergonadism and endocrine sex reversal in females lacking ERalpha but not ERbeta. Mol Endocrinol. (2003) 17:1039–53. doi: 10.1210/me.2002-0398
54. Lindzey J, Korach KS. Developmental and physiological effects of estrogen receptor gene disruption in mice. Trends Endocrinol Metab. (1997) 8:137–45. doi: 10.1016/S1043-2760(97)00007-6
55. Sanchez-Andrade G, Kendrick KM. Roles of alpha- and beta-estrogen receptors in mouse social recognition memory: effects of gender and the estrous cycle. Horm Behav. (2011) 59:114–22. doi: 10.1016/j.yhbeh.2010.10.016
56. Kuhn C, Johnson M, Thomae A, Luo B, Simon SA, Zhou G, et al. The emergence of gonadal hormone influences on dopaminergic function during puberty. Horm Behav. (2010) 58:122–37. doi: 10.1016/j.yhbeh.2009.10.015
57. Barth C, Villringer A, Sacher J. Sex hormones affect neurotransmitters and shape the adult female brain during hormonal transition periods. Front Neurosci. (2015) 9:37. doi: 10.3389/fnins.2015.00037
58. Karpinski M, Mattina GF, Steiner M. Effect of gonadal hormones on neurotransmitters implicated in the pathophysiology of obsessive-compulsive disorder: a critical review. Neuroendocrinology (2017) 105:1–16. doi: 10.1159/000453664
59. Johnson ML, Ho CC, Day AE, Walker QD, Francis R, Kuhn CM. Oestrogen receptors enhance dopamine neurone survival in rat midbrain. J Neuroendocrinol. (2010) 22:226–37. doi: 10.1111/j.1365-2826.2010.01964.x
60. Labouesse MA, Langhans W, Meyer U. Effects of selective estrogen receptor alpha and beta modulators on prepulse inhibition in male mice. Psychopharmacology (2015) 232:2981–94. doi: 10.1007/s00213-015-3935-9
61. Yoest KE, Cummings JA, Becker JB. Estradiol, dopamine and motivation. Cent Nerv Syst Agents Med Chem. (2014) 14:83–9. doi: 10.2174/1871524914666141226103135
62. Justice AJ, De Wit H. Acute effects of d-amphetamine during the follicular and luteal phases of the menstrual cycle in women. Psychopharmacology (1999) 145:67–75. doi: 10.1007/s002130051033
Keywords: estrogen receptor alpha, estrogen receptor beta, depressive-like behaviors, mood disorders, sex differences, stress, amphetamine, psychomotor activation
Citation: Georgiou P, Zanos P, Jenne CE and Gould TD (2019) Sex-Specific Involvement of Estrogen Receptors in Behavioral Responses to Stress and Psychomotor Activation. Front. Psychiatry 10:81. doi: 10.3389/fpsyt.2019.00081
Received: 10 November 2018; Accepted: 06 February 2019;
Published: 26 February 2019.
Edited by:
Naguib Mechawar, McGill University, CanadaReviewed by:
Christina Dalla, National and Kapodistrian University of Athens, GreeceCaroline Menard, Laval University, Canada
Copyright © 2019 Georgiou, Zanos, Jenne and Gould. This is an open-access article distributed under the terms of the Creative Commons Attribution License (CC BY). The use, distribution or reproduction in other forums is permitted, provided the original author(s) and the copyright owner(s) are credited and that the original publication in this journal is cited, in accordance with accepted academic practice. No use, distribution or reproduction is permitted which does not comply with these terms.
*Correspondence: Todd D. Gould, Z291bGRsYWJAbWUuY29t