- 1Department of Psychiatry, Yale University School of Medicine, New Haven, CT, United States
- 2Department of Neuroscience, Yale University School of Medicine, New Haven, CT, United States
- 3Interdepartmental Neuroscience Program, Yale University, New Haven, CT, United States
- 4Beijing Huilongguan Hospital, Beijing, China
The role of dopamine in cocaine misuse has been extensively documented for the mesocorticolimbic circuit. Preclinical work from earlier lesion studies to recent multidisciplinary investigations has suggested that the hypothalamus is critically involved in motivated behavior, with the lateral and medial hypothalamus each involved in waking/feeding and resting/satiety. However, little is known of hypothalamus function and dysfunction in cocaine misuse. Here, we examined resting state functional connectivity of the lateral and medial hypothalamus in 70 individuals with cocaine dependence (CD) and 70 age as well as gender matched healthy controls (HC). Image pre-processing and analyses followed published work. Compared to HC, CD showed increased lateral hypothalamic connectivity with dorsolateral prefrontal cortex and decreased functional connectivity with the ventral precuneus. CD showed increased medial hypothalamic connectivity with the inferior parietal lobule and decreased connectivity with the ventromedial prefrontal cortex, temporal gyrus, fusiform gyrus, and ventral striatum. Further, at trend level significance, the connectivity strength between lateral hypothalamus and dorsolateral prefrontal cortex was positively correlated with total amount of cocaine use in the past month (p = 0.004, r = 0.35) and the connectivity strength between medial hypothalamus and ventral striatum was negatively correlated with cocaine craving as assessed by the Tiffany Cocaine Craving Questionnaire (p = 0.008, r = −0.33). Together, the findings demonstrated altered resting state functional connectivity of the hypothalamus and may provide new insight on circuit level deficits in cocaine dependence.
Introduction
Individuals with drug addiction are characterized by severe motivation deficits (1) and under-responsiveness to natural reinforcers (2–4). The dopaminergic pathways process reinforcing stimuli and play a critical role in motivated behavior (5–8). Dopamine helps not only to establish the motivational value of extrinsic stimuli during initial conditioning but also link incentives to action through learning (1, 6). Preclinical studies showed that repeated administration of stimulants altered dopaminergic signaling and motivated behaviors such as preference for sucrose (9–12). Abundant research of dopaminergic deficits in addiction has focused on the ventral striatum, largely in the context of reward-related responses. The hypothalamus also receives extensive projections from the dopaminergic midbrain and is implicated in functions from those essential to survival to cognitive and affective processes in support of goal-directed behavior (13–18). On the other hand, it remains unclear how hypothalamus function is influenced by cocaine misuse.
Located below the thalamus, the hypothalamus lies along third ventricle walls below the hypothalamic sulcus and continues across the floor of the ventricle (19, 20). The hypothalamus regulates arousal, food intake, sexual drive, reward and affective response (21–23). Previous studies have implicated the hypothalamus in drug addiction (24, 25). In rodents the transition from controlled to compulsive cocaine self-administration was associated with substantial remodeling of hypothalamic circuitry (26–28). Compulsive cocaine use altered an array of dopamine gene expression, leading to functional reorganization of the hypothalamus (26). In humans individuals with cocaine dependence (CD) showed altered hypothalamus activation viewing erotic vs. neutral pictures, as compared to healthy controls (HC) (29). Hypothalamus response to monetary reward vs. non-reward was associated with the duration of abstinence in CD (30). HC showed increased hypothalamus activation viewing food vs. neutral pictures (31–34), whereas a recent study reported decreased hypothalamus activation in CD for the same contrast (35). Together, these findings highlighted hypothalamus dysfunction in the context of reward, food and sex drive in cocaine addiction.
Preclinical work from earlier lesion studies to recent multidisciplinary investigations has suggested that the hypothalamus could be broadly divided into lateral hypothalamus (LH) and medial hypothalamus (MH) each involved in waking/feeding and resting/satiety (36). On the other hand, imaging studies in humans have not aimed to distinguish LH and MH and delivered a more diverse picture of hypothalamus functions. For instance, on the basis of reported coordinates, both LH (37–40) and MH (32–34) appeared to respond to exposure to high caloric vs. low caloric food or non-food stimuli. Both LH (41–49) and MH (46, 49–51) responded to exposure to erotic vs. neutral visual stimulation. Similarly, both LH (52–54) and MH (52, 55–57) increased activations to gain vs. no-gain scenarios in the monetary incentive delay task. Thus, both hypothalamus divisions appeared to be engaged in behavioral challenges that involved explicit reward.
Combining T1-weighted 3D Fast Field Echo MR imaging and histology, Baroncini and colleagues identified hypothalamic nuclei and adjacent white matter fascicles (20). Gray and white matter structures within and around the hypothalamus were identified via specific landmarks on histological sections, from the optic chiasm anteriorly to the mammillary bodies posteriorly, and with reference to published work and atlas (58–65). The landmarks, including the optic tract, the floor of the diencephalon, the third ventricle, and the fornix, were readily identifiable in MR scans. Identifiability scorings between an anatomist and a neuroradiologists for 20 brains was highly in agreement (Cohen's κ = 0.96, p < 0.0001). The averaged MNI coordinates of identified hypothalamic nuclei in anatomical MNI space were provided from the 20 volunteers. Based on these coordinates, the MH (mean: ±4, −2, −12) included the arcuate nucleus, ventromedial nucleus and part of the dorsomedial nucleus and LH (mean: ±6, −9, −10) included the other hypothalamic nuclei located laterally and posteriorly. With the coordinates, a more recent study highlighted distinct resting state functional connectivity (rsFC) of the LH and MH (66). Specifically, LH was more heavily connected to the dorsomedial PFC (dmPFC), thalamus, and frontal operculum, and MH was more connected to the vmPFC and ventral striatum. These studies suggested the feasibility in examining activity and functional connectivity of the LH and MH separately.
In the current study, we examined the rsFC of the LH and MH in CD as compared to HC. We hypothesized that (1) LH and MH would show distinct functional connectivities, in replication of Kullmann et al. (66); and (2) compared to HC, CD showed altered rsFC in correlation with clinical characteristics such as cocaine craving score and duration and recent amount of cocaine use. Further, the literature supports sex differences in hypothalamus dysfunction in relation to cocaine misuse. For instance, female as compared to male rats showed greater hypothalamic-pituitary-adrenal (HPA) axis activation following administration of cocaine (67). Male but not female rats showed significant increases in cocaine- and amphetamine-regulated transcript (CART) peptide expression in the hypothalamus following forced swim stress (68). In humans, cocaine use appeared to alter hypothalamic-pituitary-gonadal function more in men than in women (69). During exposure to stress or drug cues, cocaine-dependent women demonstrated more blunted HPA axis response than did cocaine-dependent men (70, 71). Thus, we explored potential sex differences in the current study.
Materials and Methods
This is an exploratory study using an imaging data set collected earlier (72). Briefly, cocaine dependent subjects (CD) were recruited for inpatient stay at the Clinical Neuroscience Research Unit of the Connecticut Mental Health Center and healthy control subjects (HC) participated in the study as “outpatients.” The goals of the original study were to examine the component neural processes of cognitive control using a stop signal task (73, 74) and how these processes were altered in cocaine addiction. The original sample consisted of 97 CD and 96 HC. The current study was based on a subsample of the participants who were also scanned during resting state. Below is a description of the subjects, study procedures, and data analysis specific to the current work.
Subjects, Informed Consent, and Assessment
Seventy recently abstinent subjects with cocaine dependence (CD, 52 men) and 70 age- and gender-matched healthy control (HC, 46 men) subjects participated in the study (Table 1). CD met criteria for current cocaine dependence, as diagnosed by the Structured Clinical Interview for DSM-IV (75). Recent cocaine use was confirmed by urine toxicology screens. They were drug-free while staying in an inpatient unit for 7–10 days prior to the current fMRI study. All subjects were physically healthy with no major medical illnesses or current use of prescription medications. None reported having a history of head injury or neurological illness. Other exclusion criteria included dependence on another psychoactive substance (except nicotine) and current or past history of psychotic disorders. Individuals with current depressive or anxiety symptoms requiring treatment or currently being treated for these symptoms were excluded as well. The Human Investigation committee at Yale University School of Medicine approved all study procedures, and all subjects signed an informed consent prior to participation.
CD's were assessed with the Beck Depression Inventory (76) and the State-Trait Anxiety Inventory (77) at admission. The mean (±SD) BDI (10.0 ± 7.5) and STAI state (34.0 ± 8.1) and trait (37.8 ± 8.2) scores were within the range reported previously for individuals with cocaine dependence (78–81). Cocaine craving was assessed with the Cocaine Craving Questionnaire, brief version (CCQ-Brief), for all participants every 2–3 days during the inpatient stay (82). The CCQ-Brief is a 10-item questionnaire, abbreviated from the CCQ-Now (83). CCQ-Brief, CCQ-Now and other measures were highly correlated in craving assessment (82). Each item was rated on a scale from 1 to 7, with a higher total score (ranging from 10 to 70) indicating greater craving. Here, CDs averaged 22.7 ± 11.9 in CCQ score across all assessments and 19.3 ± 6.6 on the day or within 2 days of the scan.
Imaging Protocol
Conventional T1-weighted spin echo sagittal anatomical images were acquired for slice localization using a 3T scanner (Siemens Trio). Anatomical images of the functional slice locations were next obtained with spin echo imaging in the axial plane parallel to the AC–PC line with TR = 300 ms, TE = 2.5 ms, bandwidth = 300 Hz/pixel, flip angle = 60°, field of view = 220 × 220 mm, matrix = 256 × 256, 32 slices with slice thickness = 4 mm and no gap. Functional, blood oxygen level-dependent (BOLD) signals were then acquired with a single-shot gradient echo echoplanar imaging (EPI) sequence. 32 axial slices parallel to the AC–PC line covering the whole brain were acquired with TR = 2,000 ms, TE = 25 ms, bandwidth = 2,004 Hz/pixel, flip angle = 85°, field of view = 220 × 220 mm, matrix = 64 × 64, 32 slices with slice thickness = 4 mm and no gap. One 10-min resting state BOLD scan was obtained for each participant with eyes closed.
Imaging Data Preprocessing
Data were analyzed with Statistical Parametric Mapping (SPM8, Wellcome Department of Imaging Neuroscience, University College London, U.K.). Images from the first five TRs at the beginning of each trial were discarded to enable the signal to achieve steady-state equilibrium between RF pulsing and relaxation. Standard image preprocessing was performed. Images of each individual subject were first realigned (motion corrected) and corrected for slice timing. A mean functional image volume was constructed for each subject per run from the realigned image volumes. These mean images were co-registered with the high-resolution structural image and then segmented for normalization with affine registration followed by nonlinear transformation (84, 85). The normalization parameters determined for the structure volume were then applied to the corresponding functional image volumes for each subject. Finally, the images were smoothed with a Gaussian kernel of 4 mm at Full Width at Half Maximum.
Additional preprocessing was applied to reduce spurious BOLD variances that were unlikely to reflect neuronal activity (86–89). The sources of spurious variance were removed through linear regression by including the signal from the ventricular system, white matter, and whole brain, in addition to the six parameters obtained by rigid body head motion correction. First-order derivatives of the whole brain, ventricular and white matter signals were also included in the regression.
Cordes and colleagues suggested that BOLD fluctuations below a frequency of 0.1 Hz contribute to regionally specific BOLD correlations (90). Thus, we applied a temporal band-pass filter (0.009 Hz < f < 0.08 Hz) to the time course in order to obtain low-frequency fluctuations, as in previous studies (87–89, 91).
Head Motion
As extensively investigated in Van Dijk et al. (92), micro head motion (>0.1 mm) is an important source of spurious correlations in resting state functional connectivity analysis (92). Therefore, we applied a “scrubbing” method proposed by Power and colleagues (93) and successfully applied in previous studies (94, 95) to remove time points affected by head motions. Briefly, for every time point t, we computed the framewise displacement given by FD(t) = |Δdx(t)| + |Δdy(t)| + |Δdz(t)| + |Δα(t)| + |Δβ(t)| + |Δγ(t)|, where (dx, dy, dz) and (α, β, γ) are the translational and rotational movements, respectively (93). The second head movement metric was the root mean square variance (DVARS) of the differences in % BOLD intensity I(t) between consecutive time points across brain voxels, computed as follows: , where the brackets indicate the mean across brain voxels. Finally, to compute each subject's correlation map, we removed every time point that exceeded the head motion limit FD(t) >0.5 mm or DVARS(t) >0.5% (93, 95). On average, 1% of the time points were removed across subjects. CD and HC did not differ in FD (p = 0.72) or in DVARS (p = 0.52).
Seed Based Correlation and Group Analyses
The seed regions of MH (two spheres of 2 mm in radius, centered at x = ±4, y = −2, z = −12) and LH (two spheres of 2 mm in radius, centered at x = ±6, y = −9, z = −10) were generated according to previous studies (Figure 1) (20, 66). Supplementary Figure 1 shows the seed region in relation to potential locations of the hypothalamic subnuclei based on an atlas (65). The BOLD time courses were averaged spatially over each of the MH and LH seeds. For individual subjects, we computed the correlation coefficient between the averaged time course of each seed region and the time courses of all other brain voxels. To assess and compare the resting state functional connectivity, we converted these image maps, which were not normally distributed, to z score maps by Fisher's z transform (96, 97): . The Z maps were used in group random effect analyses. We performed one-sample t-test each on the Z maps of MH and LH for CD and HC and two-sample t-test with age as covariate to compare the two groups. We also performed a two-way ANOVA with age as a covariate on Z maps to verify group (CD vs. HC) main effect and examine seed (LH vs. MH) main effect and group by seed interaction. In addition, we examined main effect and interaction effect of sex differences in another two-way ANOVA (group × sex) each for LH and MH connectivity.
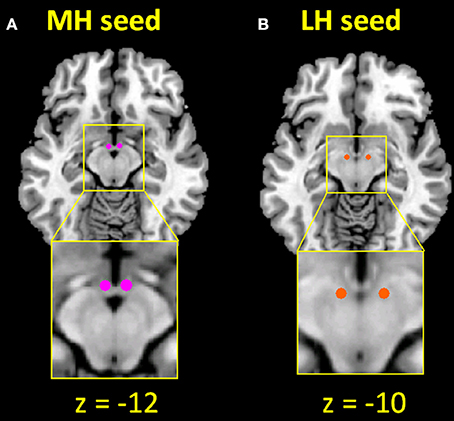
Figure 1. Seed regions of the (A) medial hypothalamus (MH; x = ±4, y = −2, z = −12) and (B) lateral hypothalamus (LH; x = ±6, y = −9, z = −10).
Results
We reported the results of one-sample t-test of whole-brain connectivity of the MH and LH each for CD and HC and of two-sample t-test of CD vs. HC. The one-sample t-test allowed us to examine whether the findings of HC replicated those reported in earlier studies. Further, with a two-way ANOVA (group: CD vs. HC × seed: LH vs. MH) we examined whether LH and MH connectivities were differentially altered in CD vs. HC. All findings were queried at a corrected threshold, according to current reporting standards.
Resting State Functional Connectivity (rsFC)
Examined at voxel p < 0.05 corrected for FWE on the basis of Gaussian random field theory, the results of a one-sample t-test of the MH and LH connectivity to the whole brain are shown in Supplementary Figure 2 for HC and CD. The patterns of whole-brain connectivity largely reflected those reported earlier (66). We then compared CD and HC in a two-sample t-test at voxel p < 0.001 uncorrected and cluster p < 0.05 FWE (Figure 2; Table 2). Because more CD than HC participants were current smokers, we included smoker status as well as age as covariates in the model. Compared to HC, CD showed increased MH rsFC with inferior parietal lobule (IPL) as well as decreased rsFC with the ventromedial prefrontal cortex (vmPFC), ventral stratum (VS), superior temporal gyrus, fusiform gyrus and cerebellum. Compared to HC, CD showed increased LH rsFC with the dorsolateral prefrontal cortex (dlPFC) and decreased LH rsFC with the ventral precuneus (PCu).
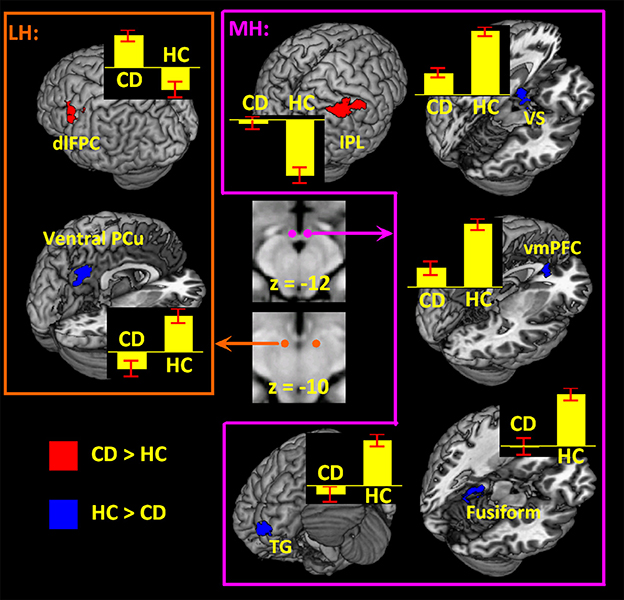
Figure 2. Hypothalamic connectivity differences between 70 CD and 70 HC (voxel p < 0.001 uncorrected and cluster p < 0.05 FWE corrected) each for LH and MH. Histograms show mean ± standard error of the connectivity z scores. dlPFC, dorsolateral prefrontal cortex; PCu, precuneus; IPL, inferior parietal lobule; VS, ventral striatum; vmPFC, ventromedial prefrontal cortex; TG, temporal gyrus.
As the LH and MH appear to support motivated behavior in opposite directions, we conducted a two-way group (CD vs. HC) × seed (LH vs. MH) ANOVA with smoker status and age as covariates to examine the interaction effects. At voxel p < 0.001, uncorrected and cluster p < 0.05 FWE, the results showed main effects of increased dlPFC and decreased vmPFC, temporal gyrus, fusiform gyrus and cerebellum connectivities in CD as compared to HC, as expected. In interaction effects, the ventral striatum (VS, x = −9, y = 8, z = −17, volume = 891 mm3, peak voxel Z = 3.92) showed increased LH connectivity (p = 0.005) but decreased MH connectivity (p = 4 × 10−8) in CD as compared to HC (Figure 3A). In contrast, a cluster in the dorsal precuneus (PCu) (x = 6, y = −73, z = 49, volume = 999 mm3, peak voxel Z = 3.85) showed decreased LH connectivity (p = 0.02) but increased MH connectivity (p = 3 × 10−5) in CD as compared to HC (Figure 3B).
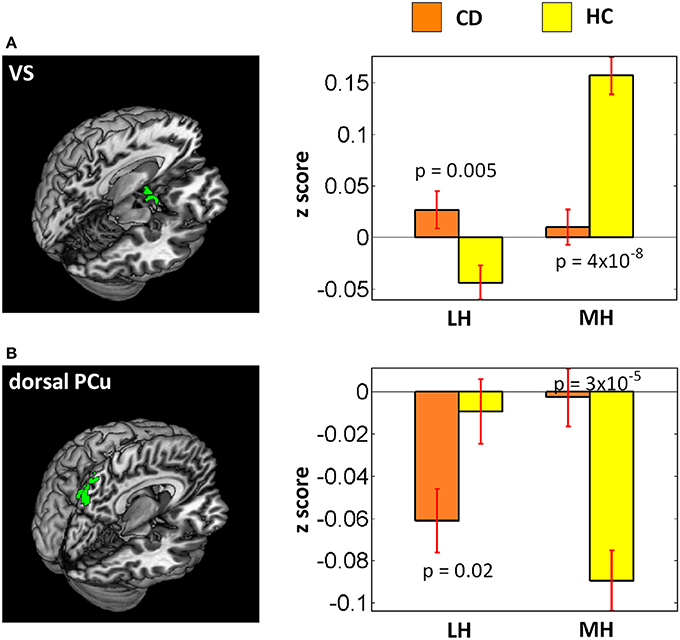
Figure 3. Results of two-way ANOVA (CD vs. HC × LH vs. MH). Left panel showed brain regions (A: ventral striatum; B: dorsal precuneus) identified under voxel p < 0.001 uncorrected and cluster-level p < 0.05 FWE corrected. Right panel showed bar plots of connectivity z scores (mean ± standard error). P-values were from planned two-sample t-tests of CD vs. HC each for LH and MH connectivity.
Relationship to Clinical Characteristics
In evaluating the relationship between the connectivity changes and cocaine use variables, we focused on cocaine craving (CCQ score), duration of use (years), and amount of recent use (grams of cocaine used in the past month). Thus, with the number (7) of ROIs tested, the results of linear regression were evaluated with a corrected p = 0.05/(3 × 7) = 0.0024. None of the Pearson regressions demonstrated a correlation at the corrected threshold. At an uncorrected threshold, across CD, the LH-dlPFC connectivity strength (z scores) was positively correlated with total amount of cocaine use in the past month (p = 0.006, r = 0.34). The MH-VS connectivity strength was negatively correlated with cocaine craving as assessed by the CCQ (p = 0.006, r = −0.34). The MH-vmPFC connectivity strength was negatively correlated with years of cocaine use (p = 0.03, r = −0.26).
Sex Differences
In a separate ANOVA, we assessed group by sex interaction each for LH and MH connectivity with smoker status and age as covariates (Figure 4). For the sex main effect women showed higher connectivity between MH and bilateral ventral tegmental area (VTA) (x = 12, y = −19, z = −14, volume = 486 mm3, peak voxel Z = 3.73; and x = −9, y = −13, z = −17, volume = 540 mm3, Z = 3.35). An interaction effect was observed for the MH—dorsomedial prefrontal cortex (dmPFC) (x = −12, y = 38, z = 46, volume = 3,321 mm3, Z = 4.30) connectivity, with HC men showing higher connectivity than HC women and CD showing the opposite pattern. At an uncorrected threshold, MH-dmPFC connectivity was negatively correlated with CCQ score in CD women (p = 0.02, r = −0.64) but not in CD men (p = 0.59, r = 0.09). A slope test confirmed the sex difference (p = 0.04).
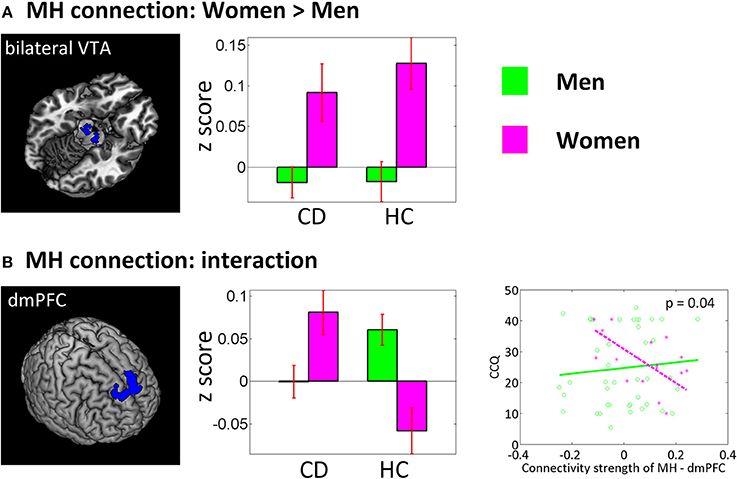
Figure 4. Sex main and sex by group interaction effects of a two-way ANOVA (CD vs. HC × men vs. women). Left panel showed brain regions identified under voxel p < 0.005 uncorrected and cluster-level p < 0.05 FWE corrected (or small volume correction at p < 0.05, FWE for the VTA). The middle panel showed corresponding bar plots of the connectivity strength (z score, mean ± S.E.). VTA, ventral tegmental area; dmPFC, dorsomedial prefrontal cortex. The lower right panel showed regression of CCQ score vs. MH-dmPFC rsFC for men vs. women.
Discussion
The ventral striatum is a major projection target of the dopaminergic midbrain and critically implicated in the etiology of cocaine addiction. Many studies have examined functional connectivities of the ventral striatum (98–102). We recently employed difference mapping to describe how ventral striatal subregions were altered in resting state connectivities in cocaine addicted vs. non-drug using individuals (103). Hypothalamus also receives heavy dopaminergic projections from the midbrain. On the other hand, no studies to date have examined whether or how functional connectivities of the hypothalamus may be influenced by chronic cocaine exposure. The current work took on this challenge and explored resting state functional connectivity (rsFC) of the lateral (LH) and medial (MH) hypothalamus in recently abstinent individuals with cocaine dependence (CD) as contrasted with age- and sex-matched healthy subjects (HC). Whole-brain rsFC of the LH and MH largely replicated the results reported earlier, with the LH connected with the midbrain, thalamus, lentiform nucleus as well as the rostral and dorsal anterior cingulate cortex and the MH connected with the ventral striatum and ventromedial prefrontal crotex (66). Compared with HC, CD showed distinct changes in LH and MH rsFC. We highlighted some of the main findings in the below.
Hypothalamus and Ventral Stratum (VS)
The VS was more heavily connected with the MH than LH, in accord with (66). Compared to HC, CD showed decreased MH-VS connectivity, with the connectivity strength negatively correlated with cocaine craving score. Thus, cocaine addiction is associated with diminished MH-VS connectivity and the extent of impairment appears to be associated with cocaine craving. The VS and hypothalamus are reciprocally connected (104). Both the VS and hypothalamus receive projections from the dopaminergic midbrain and are implicated in processing of reward-related stimuli, including those associated with drugs of abuse (6, 14, 104–106). Imaging studies showed that the extracellular concentration of dopamine in the VS increased with administration of drugs of abuse, and the experienced euphoria was positively associated with the extent of dopamine release in the VS (107–110). Hypothalamus connectivity with the VS was also observed here as a group (CD vs. HC) by seed (LH vs. MH) interaction effect, with CD showing increased LH and decreased MH-VS connectivity as compared to HC. For HC, the VS was each positively and negatively connected with the MH and LH, but this differential pattern of connectivity was not observed in CD. How disrupted hypothalamus VS connectivity may conduce to changes in motivated behavior in CD would be an important issue to address in future work.
Hypothalamus and Precuneus
Compared with HC, CD showed decreased LH rsFC with the ventral precuneus. ANOVA showed an interaction effect with CD and HC each showing stronger negative LH and MH connectivity with the dorsal precuneus. Previous studies implicated the precuneus in behavioral engagement and self-awareness, with the ventral precuneus comprising part of the default mode network (DMN) (111–114). DMN dysfunction has been widely reported in drug addiction (115–120) and hypothalamus—DMN dysconnectivity was observed in a number of neuropsychiatric disorders (121–124). The current findings may also be considered with our earlier report of the fractional amplitude of the low frequency BOLD signal of the dorsal precuenus as a neural analog of behavioral engagement (113) and studies of altered behavioral engagement in cocaine misuse (125–129).
Hypothalamus and Prefrontal Cortex (PFC)
The hypothalamus and nucleus accumbens share projections from the PFC (130), with the medial PFC projecting preferentially to the MH (131). Neurons of the subgenual anterior cingulate cortex—a subarea of the vmPFC—increased firing during rest and sleep (132), as do neurons of the MH (133, 134). Neurons in the vmPFC that project directly to the hypothalamus responded to a conditioned food cue in sated rats (135). Both the vmPFC and hypothalamus responded to emotional exposure (136), sexual arousal (42, 44, 45, 137), as well as food stimulus (138, 139). These studies suggested functional coordination between the (medial) hypothalamus and vmPFC, which, as the current findings showed, may be disrupted in CD.
The dlPFC is widely implicated in drug addiction. Severity of drug use across heroin, alcohol, MDMA, and cannabis was negatively associated with dlPFC activation in dependent individuals (140). In the current study, we observed increased functional connectivity between the LH and dlPFC, a finding that can be considered with earlier reports of hyperactive dlPFC during negative emotion processing in addicted individuals (29, 128, 141–144). In studies of depression, repetitive transcranial magnetic stimulation of the dlPFC induced neuroendocrine changes, likely via its effects on the hypothalamus-pituitary-adrenal (HPA) axis (145). Interestingly, a recent study showed increased hypothalamic connectivity with the dlPFC in depression patients after 8 weeks of sertraline treatment (146). Thus, altered LH-dlPFC connectivity may reflect an outcome of chronic exposure to catecholaminergic agents, including cocaine and many antidepressants. How cocaine misuse disrupts dlPFC regulation of the HPA axis activity warrants more research.
Hypothalamus and Inferior Parietal Lobule (IPL)
We observed increased MH rsFC with the IPL in CD as compared to HC, with HC but not CD showing significant negative connectivity. The finding was broadly consistent with attention and parietal dysfunction in chronic cocaine users (147–151). Notably, a recent study showed increased hypothalamus IPL connectivity during exposure to food vs. non-food stimuli in obese individuals (152). Further, craving and hypothalamus—IPL connectivity both decreased after leptin administration, in support of a functional role of this circuit in food “wanting.” MH-IPL circuit dysfunction may similarly be investigated with cue craving tasks or behavioral paradigms that address attentional bias to drug cues.
Limitations of the Study, Conclusions, and Future Research
Several limitations of the study need to be considered. First, the hypothalamus is a relatively small structure (~2,360 mm3, vs. amygdala ~3,744 mm3, for comparison). We drew a hypothalamus mask in MNI space based on a brain atlas (65) and marked the putative locations of hypothalamic subnuclei based on Baroncini et al. (20), as shown in Supplementary Figure 1. Our LH and MH seeds were within the range of previous reported coordinates. The connectivity we observed between LH and dmPFC/thalamus and between MH and vmPFC/ventral striatum was identical to the results reported in Kullmann et al. (66). On the other hand, image resolution as well as inter-subject variation in anatomy pose challenges to accurately identifying the hypothalamus. These findings thus remain to be substantiated. Second, CD may differ from HC in details of alcohol and nicotine use, or other clinical variables, such as history of childhood trauma, that were not assessed in the current study. Further, although candidates with clinical depression or anxiety disorders were excluded, we evaluated subclinical depression and anxiety with the BDI and STAI for CD but not for HC. Thus, we cannot rule out the possibility that the current findings may be influenced by these other clinical variables. Third, the 10-min resting state scan was conducted after four 10-min sessions of a stop signal task to address cognitive control. These task runs may influence rsFC of the default network, for instance (153). Although a previous study also showed that whole brain functional connectivity organizations were robust and stable between pre- and post-task resting states (154), we cannot rule out the possibility that the hypothalamus rsFC may be influenced by prior task runs. Fourth, as described earlier, the hypothalamus is involved in a variety of motivated behavior. Here, we related the connectivity findings to cocaine use variables but it remains to be seen how hypothalamus connectivity is disrupted in association with changes in of motivated behavior in cocaine addiction.
In summary, we demonstrated altered hypothalamus rsFC in recently abstinent cocaine addicted individuals. Cocaine misuse was associated with distinct changes in LH and MH rsFC. The findings suggested the importance in characterizing the role of hypothalamus circuit as a neural marker of cocaine addiction. Both thalamus and hypothalamus functions are disrupted in cocaine addiction (72, 155–159). Although studies have generally conceived of thalamus and hypothalamus dysfunction in terms of the respective effects of cocaine on noradrenergic and dopaminergic signaling, more work is needed to examine the neurobiological bases of thalamus-hypothalamus dysfunction in cocaine addiction.
Author Contributions
SZha and CL contributed to the conceptualization and design of the study. SZha, WW, SZho, and CL carried out the experiment and data analyses. All authors contributed to the writing and approved the final version of the manuscript.
Funding
Supported by NIH grants DA023248 and DA040032, and the Peter McManus Charitable Trust, as well as the State of Connecticut. The funding agencies otherwise have no role in the conceptualization of the study, data collection and analysis, or the decision to publish these results.
Conflict of Interest Statement
The authors declare that the research was conducted in the absence of any commercial or financial relationships that could be construed as a potential conflict of interest.
Supplementary Material
The Supplementary Material for this article can be found online at: https://www.frontiersin.org/articles/10.3389/fpsyt.2018.00344/full#supplementary-material
References
1. Blum K, Thanos PK, Oscar-Berman M, Febo M, Baron D, Badgaiyan RD, et al. Dopamine in the brain: hypothesizing surfeit or deficit links to reward and addiction. J Reward Defic Syndr. (2015) 1:95–104. doi: 10.17756/jrds.2015-016
2. Kalivas PW, Volkow ND. The neural basis of addiction: a pathology of motivation and choice. Am J Psychiatry (2005) 162:1403–13. doi: 10.1176/appi.ajp.162.8.1403
3. Koob GF, Volkow ND. Neurocircuitry of addiction. Neuropsychopharmacology (2010) 35:217–38. doi: 10.1038/npp.2009.110
4. Kopetz CE, Lejuez CW, Wiers RW, Kruglanski AW. Motivation and self-regulation in addiction: a call for convergence. Perspect Psychol Sci. (2013) 8:3–24. doi: 10.1177/1745691612457575
5. Wise RA. Brain reward circuitry: insights from unsensed incentives. Neuron (2002) 36:229–40. doi: 10.1016/S0896-6273(02)00965-0
6. Wise RA. Dopamine, learning and motivation. Nat Rev Neurosci. (2004) 5:483–94. doi: 10.1038/nrn1406
7. Pierce RC, Kumaresan V. The mesolimbic dopamine system: the final common pathway for the reinforcing effect of drugs of abuse? Neurosci Biobehav Rev. (2006) 30:215–38. doi: 10.1016/j.neubiorev.2005.04.016
8. Volkow ND, Wang GJ, Newcorn JH, Kollins SH, Wigal TL, Telang F, et al. Motivation deficit in ADHD is associated with dysfunction of the dopamine reward pathway. Mol Psychiatry (2011) 16:1147–54. doi: 10.1038/mp.2010.97
9. Henry DJ, White FJ. Repeated cocaine administration causes persistent enhancement of D1 dopamine receptor sensitivity within the rat nucleus accumbens. J Pharmacol Exp Ther. (1991) 258:882–90.
10. Sora I, Hall FS, Andrews AM, Itokawa M, Li XF, Wei HB, et al. Molecular mechanisms of cocaine reward: combined dopamine and serotonin transporter knockouts eliminate cocaine place preference. Proc Natl Acad Sci USA. (2001) 98:5300–5. doi: 10.1073/pnas.091039298
11. Lepelletier FX, Tauber C, Nicolas C, Solinas M, Castelnau P, Belzung C, et al. Prenatal exposure to methylphenidate affects the dopamine system and the reactivity to natural reward in adulthood in rats. Int J Neuropsychopharmacol. (2014) 18:1–11. doi: 10.1093/ijnp/pyu044
12. Ponchio RA, Teodorov E, Kirsten TB, Coelho CP, Oshiro A, Florio JC, et al. Repeated methylphenidate administration during lactation reduces maternal behavior, induces maternal tolerance, and increases anxiety-like behavior in pups in adulthood. Neurotoxicol Teratol. (2015) 50:64–72. doi: 10.1016/j.ntt.2015.05.008
13. Hernandez L, Paredes D, Rada P. Feeding behavior as seen through the prism of brain microdialysis. Physiol Behav. (2011) 104:47–56. doi: 10.1016/j.physbeh.2011.04.031
14. Watabe-Uchida M, Zhu L, Ogawa SK, Vamanrao A, Uchida N. Whole-brain mapping of direct inputs to midbrain dopamine neurons. Neuron (2012) 74:858–73. doi: 10.1016/j.neuron.2012.03.017
15. Tsujino N, Sakurai T. Role of orexin in modulating arousal, feeding, and motivation. Front Behav Neurosci. (2013) 7:28. doi: 10.3389/fnbeh.2013.00028
16. Verschure PF, Pennartz CM, Pezzulo G. The why, what, where, when and how of goal-directed choice: neuronal and computational principles. Philos Trans R Soc Lond B Biol Sci. (2014) 369:1655–68. doi: 10.1098/rstb.2013.0483
17. Castro DC, Cole SL, Berridge KC. Lateral hypothalamus, nucleus accumbens, and ventral pallidum roles in eating and hunger: interactions between homeostatic and reward circuitry. Front Syst Neurosci. (2015) 9:90. doi: 10.3389/fnsys.2015.00090
18. Stuber GD, Wise RA. Lateral hypothalamic circuits for feeding and reward. Nat Neurosci. (2016) 19:198–205. doi: 10.1038/nn.4220
20. Baroncini M, Jissendi P, Balland E, Besson P, Pruvo JP, Francke JP, et al. MRI atlas of the human hypothalamus. Neuroimage (2012) 59:168–80. doi: 10.1016/j.neuroimage.2011.07.013
21. Dileone RJ, Georgescu D, Nestler EJ. Lateral hypothalamic neuropeptides in reward and drug addiction. Life Sci. (2003) 73:759–68. doi: 10.1016/S0024-3205(03)00408-9
22. Sternson SM. Hypothalamic survival circuits: blueprints for purposive behaviors. Neuron (2013) 77:810–24. doi: 10.1016/j.neuron.2013.02.018
23. Poeppl TB, Langguth B, Rupprecht R, Safron A, Bzdok D, Laird AR, et al. The neural basis of sex differences in sexual behavior: a quantitative meta-analysis. Front Neuroendocrinol. (2016) 43:28–43. doi: 10.1016/j.yfrne.2016.10.001
24. Aston-Jones G, Smith RJ, Sartor GC, Moorman DE, Massi L, Tahsili-Fahadan P, et al. Lateral hypothalamic orexin/hypocretin neurons: a role in reward-seeking and addiction. Brain Res. (2010) 1314:74–90. doi: 10.1016/j.brainres.2009.09.106
25. Marchant NJ, Millan EZ, Mcnally GP. The hypothalamus and the neurobiology of drug seeking. Cell Mol Life Sci. (2012) 69:581–97. doi: 10.1007/s00018-011-0817-0
26. Ahmed SH, Lutjens R, Van Der Stap LD, Lekic D, Romano-Spica V, Morales M, et al. Gene expression evidence for remodeling of lateral hypothalamic circuitry in cocaine addiction. Proc Natl Acad Sci USA. (2005) 102:11533–8. doi: 10.1073/pnas.0504438102
27. Zhou Y, Cui CL, Schlussman SD, Choi JC, Ho A, Han JS, et al. Effects of cocaine place conditioning, chronic escalating-dose “binge” pattern cocaine administration and acute withdrawal on orexin/hypocretin and preprodynorphin gene expressions in lateral hypothalamus of Fischer and Sprague-Dawley rats. Neuroscience (2008) 153:1225–34. doi: 10.1016/j.neuroscience.2008.03.023
28. Chen J, Repunte-Canonigo V, Kawamura T, Lefebvre C, Shin W, Howell LL, et al. Hypothalamic proteoglycan syndecan-3 is a novel cocaine addiction resilience factor. Nat Commun. (2013) 4:1955. doi: 10.1038/ncomms2955
29. Asensio S, Romero MJ, Palau C, Sanchez A, Senabre I, Morales JL, et al. Altered neural response of the appetitive emotional system in cocaine addiction: an fMRI Study. Addict Biol. (2010) 15:504–16. doi: 10.1111/j.1369-1600.2010.00230.x
30. Bustamante JC, Barros-Loscertales A, Costumero V, Fuentes-Claramonte P, Rosell-Negre P, Ventura-Campos N, et al. Abstinence duration modulates striatal functioning during monetary reward processing in cocaine patients. Addict Biol. (2014) 19:885–94. doi: 10.1111/adb.12041
31. Killgore WD, Young AD, Femia LA, Bogorodzki P, Rogowska J, Yurgelun-Todd DA. Cortical and limbic activation during viewing of high- versus low-calorie foods. Neuroimage (2003) 19:1381–94. doi: 10.1016/S1053-8119(03)00191-5
32. Cornier MA. The effects of overfeeding and propensity to weight gain on the neuronal responses to visual food cues. Physiol Behav. (2009) 97:525–30. doi: 10.1016/j.physbeh.2009.03.019
33. De Araujo IE, Lin T, Veldhuizen MG, Small DM. Metabolic regulation of brain response to food cues. Curr Biol. (2013) 23:878–83. doi: 10.1016/j.cub.2013.04.001
34. English LK, Fearnbach SN, Lasschuijt M, Schlegel A, Anderson K, Harris S, et al. Brain regions implicated in inhibitory control and appetite regulation are activated in response to food portion size and energy density in children. Int J Obes (Lond). (2016) 40:1515–22. doi: 10.1038/ijo.2016.126
35. Tomasi D, Wang GJ, Wang R, Caparelli EC, Logan J, Volkow ND. Overlapping patterns of brain activation to food and cocaine cues in cocaine abusers: association to striatal D2/D3 receptors. Hum Brain Mapp. (2015) 36:120–36. doi: 10.1002/hbm.22617
36. Gao XB, Horvath T. Function and dysfunction of hypocretin/orexin: an energetics point of view. Ann Rev Neurosci. 37:101–16. doi: 10.1146/annurev-neuro-071013-013855
37. Cornier MA, Von Kaenel SS, Bessesen DH, Tregellas JR. Effects of overfeeding on the neuronal response to visual food cues. Am J Clin Nutr. (2007) 86:965–71. doi: 10.1093/ajcn/86.4.965
38. Fletcher PC, Napolitano A, Skeggs A, Miller SR, Delafont B, Cambridge VC, et al. Distinct modulatory effects of satiety and sibutramine on brain responses to food images in humans: a double dissociation across hypothalamus, amygdala, and ventral striatum. J Neurosci. (2010) 30:14346–55. doi: 10.1523/JNEUROSCI.3323-10.2010
39. Frank TC, Kim GL, Krzemien A, Van Vugt DA. Effect of menstrual cycle phase on corticolimbic brain activation by visual food cues. Brain Res. (2010) 1363:81–92. doi: 10.1016/j.brainres.2010.09.071
40. St-Onge MP, Mcreynolds A, Trivedi ZB, Roberts AL, Sy M, Hirsch J. Sleep restriction leads to increased activation of brain regions sensitive to food stimuli. Am J Clin Nutr. (2012) 95:818–24. doi: 10.3945/ajcn.111.027383
41. Beauregard M, Levesque J, Bourgouin P. Neural correlates of conscious self-regulation of emotion. J Neurosci. (2001) 21:RC165. doi: 10.1523/JNEUROSCI.21-18-j0001.2001
42. Ferretti A, Caulo M, Del Gratta C, Di Matteo R, Merla A, Montorsi F, et al. Dynamics of male sexual arousal: distinct components of brain activation revealed by fMRI. Neuroimage (2005) 26:1086–96. doi: 10.1016/j.neuroimage.2005.03.025
43. Kim SW, Sohn DW, Cho YH, Yang WS, Lee KU, Juh R, et al. Brain activation by visual erotic stimuli in healthy middle aged males. Int J Impot Res. (2006) 18:452–7. doi: 10.1038/sj.ijir.3901449
44. Ponseti J, Bosinski HA, Wolff S, Peller M, Jansen O, Mehdorn HM, et al. A functional endophenotype for sexual orientation in humans. Neuroimage (2006) 33:825–33. doi: 10.1016/j.neuroimage.2006.08.002
45. Brunetti M, Babiloni C, Ferretti A, Del Gratta C, Merla A, Olivetti Belardinelli M, et al. Hypothalamus, sexual arousal and psychosexual identity in human males: a functional magnetic resonance imaging study. Eur J Neurosci. (2008) 27:2922–7. doi: 10.1111/j.1460-9568.2008.06241.x
46. Sundaram T, Jeong GW, Kim TH, Kim GW, Baek HS, Kang HK. Time-course analysis of the neuroanatomical correlates of sexual arousal evoked by erotic video stimuli in healthy males. Korean J Radiol. (2010) 11:278–85. doi: 10.3348/kjr.2010.11.3.278
47. Wehrum S, Klucken T, Kagerer S, Walter B, Hermann A, Vaitl D, et al. Gender commonalities and differences in the neural processing of visual sexual stimuli. J Sex Med. (2013) 10:1328–42. doi: 10.1111/jsm.12096
48. Kim GW, Jeong GW. A comparative study of brain activation patterns associated with sexual arousal between males and females using 3.0-T functional magnetic resonance imaging. Sex Health (2014) 11:11–6. doi: 10.1071/SH13127
49. Lee SW, Jeong BS, Choi J, Kim JW. Sex differences in interactions between nucleus accumbens and visual cortex by explicit visual erotic stimuli: an fMRI study. Int J Impot Res. (2015) 27:161–6. doi: 10.1038/ijir.2015.8
50. Arnow BA, Desmond JE, Banner LL, Glover GH, Solomon A, Polan ML, et al. Brain activation and sexual arousal in healthy, heterosexual males. Brain (2002) 125:1014–23. doi: 10.1093/brain/awf108
51. Stark R, Schienle A, Girod C, Walter B, Kirsch P, Blecker C, et al. Erotic and disgust-inducing pictures—differences in the hemodynamic responses of the brain. Biol Psychol. (2005) 70:19–29. doi: 10.1016/j.biopsycho.2004.11.014
52. Enzi B, Edel MA, Lissek S, Peters S, Hoffmann R, Nicolas V, et al. Altered ventral striatal activation during reward and punishment processing in premanifest Huntington's disease: a functional magnetic resonance study. Exp Neurol. 235:256–64. doi: 10.1016/j.expneurol.2012.02.003
53. Montoya ER, Bos PA, Terburg D, Rosenberger LA, Van Honk J. Cortisol administration induces global down-regulation of the brain's reward circuitry. Psychoneuroendocrinology (2014) 47:31–42. doi: 10.1016/j.psyneuen.2014.04.022
54. Pfabigan DM, Seidel EM, Sladky R, Hahn A, Paul K, Grahl A, et al. P300 amplitude variation is related to ventral striatum BOLD response during gain and loss anticipation: an EEG and fMRI experiment. Neuroimage (2014) 96:12–21. doi: 10.1016/j.neuroimage.2014.03.077
55. Knutson B, Bhanji JP, Cooney RE, Atlas LY, Gotlib IH. Neural responses to monetary incentives in major depression. Biol Psychiatry (2008) 63:686–92. doi: 10.1016/j.biopsych.2007.07.023
56. Luo S, Ainslie G, Giragosian L, Monterosso JR. Striatal hyposensitivity to delayed rewards among cigarette smokers. Drug Alcohol Depend. (2011) 116:18–23. doi: 10.1016/j.drugalcdep.2010.11.012
57. Helfinstein SM, Kirwan ML, Benson BE, Hardin MG, Pine DS, Ernst M, et al. Validation of a child-friendly version of the monetary incentive delay task. Soc Cogn Affect Neurosci. (2013) 8:720–6. doi: 10.1093/scan/nss057
58. Braak H, Braak E. The hypothalamus of the human adult—chiasmatic region. Anat Embryol. (1987) 175:315–30. doi: 10.1007/BF00309845
59. Braak H, Braak E, Swaab DF, Saper CB, Josse J, Kremer HPH, et al. Anatomy of the human hypothalamus (chiasmatic and tuberal region). Prog Brain Res. (1992) 93:3–16. doi: 10.1016/S0079-6123(08)64559-8
60. Swaab DF, Hofman MA, Lucassen PJ, Purba JS, Raadsheer FC, Vandenes JAP. Functional neuroanatomy and neuropathology of the human hypothalamus. Anat Embryol. (1993) 187:317–30. doi: 10.1007/BF00185889
61. Koutcherov Y, Mai JK, Ashwell KWS, Paxinos G. Organization of the human paraventricular hypothalamic nucleus. J Comp Neurol. (2000) 423:299–318. doi: 10.1002/1096-9861(20000724)423:2<299::AID-CNE8>3.0.CO;2-A
62. Koutcherov Y, Mai JK, Ashwell KWS, Paxinos G. Organization of human hypothalamus in fetal development. J Comp Neurol. (2002) 446:301–24. doi: 10.1002/cne.10175
63. Koutcherov Y, Mai JK, Ashwel KW, Paxinos G. Organisation of the human dorsomedial hypothalamic nucleus. Neuroreport (2004) 15:107–11. doi: 10.1097/00001756-200401190-00022
64. Koutcherov Y, Paxinos G, Mai JK. Organization of the human medial preoptic nucleus. J Comp Neurol. (2007) 503:392–406. doi: 10.1002/cne.21355
66. Kullmann S, Heni M, Linder K, Zipfel S, Haring HU, Veit R, et al. Resting-state functional connectivity of the human hypothalamus. Hum Brain Mapp. (2014) 35:6088–96. doi: 10.1002/hbm.22607
67. Kuhn C, Francis R. Gender difference in cocaine-induced HPA axis activation. Neuropsychopharmacology (1997) 16:399–407. doi: 10.1016/S0893-133X(96)00278-3
68. Balkan B, Gozen O, Yararbas G, Koylu EO, Akinturk S, Kuhar MJ, et al. CART expression in limbic regions of rat brain following forced swim stress: sex differences. Neuropeptides (2006) 40:185–93. doi: 10.1016/j.npep.2006.02.001
69. Wisniewski AB, Brown TT, John M, Frankowicz JK, Cofranceso J, Golub ET, et al. Hypothalamic-pituitary-gonadal function in men and women using heroin and cocaine, stratified by HIV status. Gend Med. (2007) 4:35–44. doi: 10.1016/S1550-8579(07)80007-6
70. Fox HC, Garcia M, Kemp K, Milivojevic V, Kreek MJ, Sinha R. Gender differences in cardiovascular and corticoadrenal response to stress and drug cues in cocaine dependent individuals. Psychopharmacology (2006) 185:348–57. doi: 10.1007/s00213-005-0303-1
71. Waldrop AE, Price KL, Desantis SM, Simpson AN, Back SE, Mcrae AL, et al. Community-dwelling cocaine-dependent men and women respond differently to social stressors versus cocaine cues. Psychoneuroendocrinology (2010) 35:798–806. doi: 10.1016/j.psyneuen.2009.11.005
72. Luo X, Zhang S, Hu S, Bednarski SR, Erdman E, Farr OM, et al. Error processing and gender-shared and -specific neural predictors of relapse in cocaine dependence. Brain (2013) 136:1231–44. doi: 10.1093/brain/awt040
73. Ide JS, Li CSR. Error-related functional connectivity of the habenula in humans. Front Hum Neurosci. (2011) 5:25. doi: 10.3389/fnhum.2011.00025
74. Hu S, Li CSR. Neural processes of preparatory control for stop signal inhibition. Hum Brain Mapp. (2012) 33:2785–96. doi: 10.1002/hbm.21399
75. First M, Spitzer R, Williams J, Gibbon M. Structured Clinical Interview for DSM-IV (SCID). Washington, DC: American Psychiatric Association (1995).
76. Beck AT, Ward CH, Mendelson M, Mock J, Erbaugh J. An inventory for measuring depression. Arch Gen Psychiatry (1961) 4:561–71. doi: 10.1001/archpsyc.1961.01710120031004
77. Speilberger C, Gorsuch R, Lushene R. STAI Manual. Palo Alto, CA: Consulting Psychologist Press (1970).
78. Falck RS, Wang J, Carlson RG, Eddy M, Siegal HA. The prevalence and correlates of depressive symptomatology among a community sample of crack-cocaine smokers. J Psychoactive Drugs (2002) 34:281–288. doi: 10.1080/02791072.2002.10399964
79. Karlsgodt KH, Lukas SE, Elman I. Psychosocial stress and the duration of cocaine use in non-treatment seeking individuals with cocaine dependence. Am J Drug Alcohol Abuse (2003) 29:539–51. doi: 10.1081/ADA-120023457
80. Lopez A, Becona E. Depression and cocaine dependence. Psychol Rep. (2007) 100:520–4. doi: 10.2466/pr0.100.2.520-524
81. Rubin E, Aharonovich E, Bisaga A, Levin FR, Raby WN, Nunes EV. Early abstinence in cocaine dependence: influence of comorbid major depression. Am J Addict. (2007) 16:283–90. doi: 10.1080/10550490701389880
82. Sussner BD, Smelson DA, Rodrigues S, Kline A, Losonczy M, Ziedonis D. The validity and reliability of a brief measure of cocaine craving. Drug Alcohol Depend. (2006) 83:233–7. doi: 10.1016/j.drugalcdep.2005.11.022
83. Tiffany ST, Singleton E, Haertzen CA, Henningfield JE. The development of a cocaine craving questionnaire. Drug Alcohol Depend. (1993) 34:19–28. doi: 10.1016/0376-8716(93)90042-O
84. Friston K, Ashburner J, Frith C, Polone J, Heather J, Frackowiak R. Spatial registration and normalization of images. Hum Brain Mapp. (1995) 2:165–89. doi: 10.1002/hbm.460030303
85. Ashburner J, Friston KJ. Nonlinear spatial normalization using basis functions. Hum Brain Mapp. (1999) 7:254–66. doi: 10.1002/(SICI)1097-0193(1999)7:4<225::AID-HBM1>3.0.CO;2-P
86. Rombouts SA, Stam CJ, Kuijer JP, Scheltens P, Barkhof F. Identifying confounds to increase specificity during a “no task condition.” Evidence for hippocampal connectivity using fMRI. Neuroimage (2003) 20:1236–45. doi: 10.1016/S1053-8119(03)00386-0
87. Fox MD, Snyder AZ, Vincent JL, Corbetta M, Van Essen DC, Raichle ME. The human brain is intrinsically organized into dynamic, anticorrelated functional networks. Proc Natl Acad Sci USA. (2005) 102:9673–8. doi: 10.1073/pnas.0504136102
88. Fair DA, Schlaggar BL, Cohen AL, Miezin FM, Dosenbach NU, Wenger KK, et al. A method for using blocked and event-related fMRI data to study “resting state” functional connectivity. Neuroimage (2007) 35:396–405. doi: 10.1016/j.neuroimage.2006.11.051
89. Fox MD, Raichle ME. Spontaneous fluctuations in brain activity observed with functional magnetic resonance imaging. Nat Rev Neurosci. (2007) 8:700–11. doi: 10.1038/nrn2201
90. Cordes D, Haughton VM, Arfanakis K, Carew JD, Turski PA, Moritz CH, et al. Frequencies contributing to functional connectivity in the cerebral cortex in “resting-state” data. AJNR Am J Neuroradiol. (2001) 22:1326–1333.
91. Lowe MJ, Mock BJ, Sorenson JA. Functional connectivity in single and multislice echoplanar imaging using resting-state fluctuations. Neuroimage (1998) 7:119–32. doi: 10.1006/nimg.1997.0315
92. Van Dijk KR, Sabuncu MR, Buckner RL. The influence of head motion on intrinsic functional connectivity MRI. Neuroimage (2012) 59:431–8. doi: 10.1016/j.neuroimage.2011.07.044
93. Power JD, Barnes KA, Snyder AZ, Schlaggar BL, Petersen SE. Spurious but systematic correlations in functional connectivity MRI networks arise from subject motion. Neuroimage (2012) 59:2142–54. doi: 10.1016/j.neuroimage.2011.10.018
94. Smyser CD, Inder TE, Shimony JS, Hill JE, Degnan AJ, Snyder AZ, et al. Longitudinal analysis of neural network development in preterm infants. Cereb Cortex (2010) 20:2852–62. doi: 10.1093/cercor/bhq035
95. Tomasi D, Volkow ND. Functional connectivity of substantia nigra and ventral tegmental area: maturation during adolescence and effects of ADHD. Cereb Cortex (2014) 24:935–44. doi: 10.1093/cercor/bhs382
96. Jenkins GM, Watts DG. Spectral Analysis and Its Applications. San Francisco, CA: Holden-Day (1968).
97. Berry KJ, Mielke PW Jr. A Monte Carlo investigation of the Fisher Z transformation for normal and nonnormal distributions. Psychol Rep. (2000) 87:1101–14. doi: 10.2466/pr0.2000.87.3f.1101
98. Di Martino A, Scheres A, Margulies DS, Kelly AM, Uddin LQ, Shehzad Z, et al. Functional connectivity of human striatum: a resting state FMRI study. Cereb Cortex (2008) 18:2735–47. doi: 10.1093/cercor/bhn041
99. Tomasi D, Volkow ND, Wang R, Carrillo JH, Maloney T, Alia-Klein N, et al. Disrupted functional connectivity with dopaminergic midbrain in cocaine abusers. PLoS ONE (2010) 5:e10815. doi: 10.1371/journal.pone.0010815
100. Wilcox CE, Teshiba TM, Merideth F, Ling J, Mayer AR. Enhanced cue reactivity and fronto-striatal functional connectivity in cocaine use disorders. Drug Alcohol Depend. (2011) 115:137–44. doi: 10.1016/j.drugalcdep.2011.01.009
101. Li CS, Ide JS, Zhang S, Hu S, Chao HH, Zaborszky L. Resting state functional connectivity of the basal nucleus of Meynert in humans: in comparison to the ventral striatum and the effects of age. Neuroimage (2014) 97:321–332. doi: 10.1016/j.neuroimage.2014.04.019
102. Contreras-Rodriguez O, Albein-Urios N, Perales JC, Martinez-Gonzalez JM, Vilar-Lopez R, Fernandez-Serrano MJ, et al. Cocaine-specific neuroplasticity in the ventral striatum network is linked to delay discounting and drug relapse. Addiction (2015) 110:1953–62. doi: 10.1111/add.13076
103. Zhang S, Li CS. Ventral striatal dysfunction in cocaine dependence—difference mapping for subregional resting state functional connectivity. Transl Psychiatry. 8:119. doi: 10.1038/s41398-018-0164-0
104. Haber SN. Neuroanatomy of reward: a view from the ventral striatum. In: Gottfried JA, editor. Neurobiology of Sensation and Reward. Boca Raton, FL: CRC Press/Taylor & Francis (2011).
105. Wise RA. Dopamine and reward: the anhedonia hypothesis 30 years on. Neurotox Res. (2008) 14:169–83. doi: 10.1007/BF03033808
106. Volkow ND, Morales M. The brain on drugs: from reward to addiction. Cell (2015) 162:712–25. doi: 10.1016/j.cell.2015.07.046
107. Volkow ND, Wang GJ, Fowler JS, Logan J, Gatley SJ, Wong C, et al. Reinforcing effects of psychostimulants in humans are associated with increases in brain dopamine and occupancy of D-2 receptors. J Pharmacol Exp Therap. (1999) 291:409–15.
108. Drevets WC, Gautier C, Price JC, Kupfer DJ, Kinahan PE, Grace AA, et al. Amphetamine-induced dopamine release in human ventral striatum correlates with euphoria. Biol Psychiatry (2001) 49:81–96. doi: 10.1016/S0006-3223(00)01038-6
109. Volkow ND, Fowler JS, Wang GJ. The addicted human brain: insights from imaging studies. J Clin Invest. (2003) 111:1444–51. doi: 10.1172/JCI18533
110. Volkow ND, Fowler JS, Wang GJ, Baler R, Telang F. Imaging dopamine's role in drug abuse and addiction. Neuropharmacology (2009) 56:3–8. doi: 10.1016/j.neuropharm.2008.05.022
111. Margulies DS, Vincent JL, Kelly C, Lohmann G, Uddin LQ, Biswal BB, et al. Precuneus shares intrinsic functional architecture in humans and monkeys. Proc Natl Acad Sci USA. (2009) 106:20069–74. doi: 10.1073/pnas.0905314106
112. Cauda F, Geminiani G, D'agata F, Sacco K, Duca S, Bagshaw AP, et al. Functional connectivity of the posteromedial cortex. PLoS ONE (2010) 5:e13107. doi: 10.1371/journal.pone.0013107
113. Zhang S, Li CS. A neural measure of behavioral engagement: task-residual low-frequency blood oxygenation level-dependent activity in the precuneus. Neuroimage (2010) 49:1911–8. doi: 10.1016/j.neuroimage.2009.09.004
114. Zhang S, Li CS. Functional connectivity mapping of the human precuneus by resting state fMRI. Neuroimage (2012) 59:3548–62. doi: 10.1016/j.neuroimage.2011.11.023
115. Bednarski SR, Zhang S, Hong KI, Sinha R, Rounsaville BJ, Li CS. Deficits in default mode network activity preceding error in cocaine dependent individuals. Drug Alcohol Depend. (2011) 119:e51–7. doi: 10.1016/j.drugalcdep.2011.05.026
116. Li Z, Santhanam P, Coles CD, Lynch ME, Hamann S, Peltier S, et al. Increased “default mode” activity in adolescents prenatally exposed to cocaine. Hum Brain Mapp. (2011) 32:759–70. doi: 10.1002/hbm.21059
117. Ma N, Liu Y, Fu XM, Li N, Wang CX, Zhang H, et al. Abnormal brain default-mode network functional connectivity in drug addicts. PLoS ONE (2011) 6:e16560. doi: 10.1371/journal.pone.0016560
118. Sutherland MT, Mchugh MJ, Pariyadath V, Stein EA. Resting state functional connectivity in addiction: lessons learned and a road ahead. Neuroimage (2012) 62:2281–95. doi: 10.1016/j.neuroimage.2012.01.117
119. Ding X, Lee SW. Cocaine addiction related reproducible brain regions of abnormal default-mode network functional connectivity: a group ICA study with different model orders. Neurosci Lett. (2013) 548:110–4. doi: 10.1016/j.neulet.2013.05.029
120. Ding X, Lee SW. Changes of functional and effective connectivity in smoking replenishment on deprived heavy smokers: a resting-state FMRI study. PLoS ONE (2013) 8:e59331. doi: 10.1371/journal.pone.0059331
121. Lustig C, Snyder AZ, Bhakta M, O'brien KC, Mcavoy M, Raichle ME, et al. Functional deactivations: change with age and dementia of the Alzheimer type. Proc Natl Acad Sci USA. (2003) 100:14504–9. doi: 10.1073/pnas.2235925100
122. Kennedy DP, Redcay E, Courchesne E. Failing to deactivate: resting functional abnormalities in autism. Proc Natl Acad Sci USA. (2006) 103:8275–80. doi: 10.1073/pnas.0600674103
123. Pomarol-Clotet E, Salvador R, Sarro S, Gomar J, Vila F, Martinez A, et al. Failure to deactivate in the prefrontal cortex in schizophrenia: dysfunction of the default mode network? Psychol Med. (2008) 38:1185–93. doi: 10.1017/S0033291708003565
124. Fassbender C, Zhang H, Buzy WM, Cortes CR, Mizuiri D, Beckett L, et al. A lack of default network suppression is linked to increased distractibility in ADHD. Brain Res. (2009) 1273:114–28. doi: 10.1016/j.brainres.2009.02.070
125. Hyman SE, Malenka RC. Addiction and the brain: the neurobiology of compulsion and its persistence. Nat Rev Neurosci. (2001) 2:695–703. doi: 10.1038/35094560
126. Molitor A, Mayes LC, Ward A. Emotion regulation behavior during a separation procedure in 18-month-old children of mothers using cocaine and other drugs. Dev Psychopathol. (2003) 15:39–54. doi: 10.1017/S0954579403000038
127. Aharonovich E, Arnrhein PC, Bisaga A, Nunes EV, Hasin DS. Cognition, commitment language, and behavioral change among cocaine-dependent patients. Psychol Addict Behav. (2008) 22:557–62. doi: 10.1037/a0012971
128. Goldstein RZ, Volkow ND. Dysfunction of the prefrontal cortex in addiction: neuroimaging findings and clinical implications. Nat Rev Neurosci. (2011) 12:652–69. doi: 10.1038/nrn3119
129. Preller KH, Herdener M, Schilbach L, Stampfli P, Hulka LM, Vonmoos M, et al. Functional changes of the reward system underlie blunted response to social gaze in cocaine users. Proc Natl Acad Sci USA. (2014) 111:2842–7. doi: 10.1073/pnas.1317090111
130. Gabbott PLA, Warner TA, Jays PRL, Salway P, Busby SJ. Prefrontal cortex in the rat: projections to subcortical autonomic, motor, and limbic centers. J Comp Neurol. (2005) 492:145–77. doi: 10.1002/cne.20738
131. Bandler R, Keay KA, Floyd N, Price J. Central circuits mediating patterned autonomic activity during active vs. passive emotional coping. Brain Res Bull. (2000) 53:95–104. doi: 10.1016/S0361-9230(00)00313-0
132. Gabbott PL, Rolls ET. Increased neuronal firing in resting and sleep in areas of the macaque medial prefrontal cortex. Eur J Neurosci. (2013) 37:1737–46. doi: 10.1111/ejn.12171
133. Koyama Y, Takahashi K, Kodama T, Kayama Y. State-dependent activity of neurons in the perifornical hypothalamic area during sleep and waking. Neuroscience (2003) 119:1209–19. doi: 10.1016/S0306-4522(03)00173-8
134. Takahashi K, Lin JS, Sakai K. Characterization and mapping of sleep-waking specific neurons in the basal forebrain and preoptic hypothalamus in mice. Neuroscience (2009) 161:269–92. doi: 10.1016/j.neuroscience.2009.02.075
135. Petrovich GD, Holland PC, Gallagher M. Amygdalar and prefrontal pathways to the lateral hypothalamus are activated by a learned cue that stimulates eating. J Neurosci. (2005) 25:8295–302. doi: 10.1523/JNEUROSCI.2480-05.2005
136. Damasio AR. The Feeling of what Happens: Body, Emotion and the Making of Consciousness. New York, NY: Harcourt Brace (1999).
137. Walter M, Bermpohl F, Mouras H, Schiltz K, Tempelmann C, Rotte M, et al. Distinguishing specific sexual and general emotional effects in fMRI-subcortical and cortical arousal during erotic picture viewing. Neuroimage (2008) 40:1482–94. doi: 10.1016/j.neuroimage.2008.01.040
138. Eiler WJII, Dzemidzic M, Case KR, Soeurt CM, Armstrong CL, Mattes RD, et al. The aperitif effect: alcohol's effects on the brain's response to food aromas in women. Obes (Silver Spring) (2015) 23:1386–1393. doi: 10.1002/oby.21109
139. Thomas JM, Higgs S, Dourish CT, Hansen PC, Harmer CJ, Mccabe C. Satiation attenuates BOLD activity in brain regions involved in reward and increases activity in dorsolateral prefrontal cortex: an fMRI study in healthy volunteers. Am J Clin Nutr. (2015) 101:697–704. doi: 10.3945/ajcn.114.097543
140. Moreno-Lopez L, Stamatakis EA, Fernandez-Serrano MJ, Gomez-Rio M, Rodriguez-Fernandez A, Perez-Garcia M, et al. Neural correlates of the severity of cocaine, heroin, alcohol, MDMA and cannabis use in polysubstance abusers: a resting-PET brain metabolism study. PLoS ONE (2012) 7:e39830. doi: 10.1371/journal.pone.0039830
141. Salloum JB, Ramchandani VA, Bodurka J, Rawlings R, Momenan R, George D, et al. Blunted rostral anterior cingulate response during a simplified decoding task of negative emotional facial expressions in alcoholic patients. Alcohol Clin Exp Res. (2007) 31:1490–504. doi: 10.1111/j.1530-0277.2007.00447.x
142. Payer DE, Lieberman MD, Monterosso JR, Xue JS, Fong TW, London ED. Differences in cortical activity between methamphetamine-dependent and healthy individuals performing a facial affect matching task. Drug Alcohol Depend. (2008) 93:93–102. doi: 10.1016/j.drugalcdep.2007.09.009
143. Gruber SA, Rogowska J, Yurgelun-Todd DA. Altered affective response in marijuana smokers: an FMRI study. Drug Alcohol Depend. (2009) 105:139–53. doi: 10.1016/j.drugalcdep.2009.06.019
144. Kim YT, Lee JJ, Song HJ, Kim JH, Kwon DH, Kim MN, et al. Alterations in cortical activity of male methamphetamine abusers performing an empathy task: fMRI study. Hum Psychopharmacol Clin Exp. (2010) 25:63–70. doi: 10.1002/hup.1083
145. Meille V, Verges B, Lalanne L, Jonval L, Duvillard L, Chavet-Gelinier JC, et al. Effects of transcranial magnetic stimulation on the hypothalamic-pituitary axis in depression: results of a pilot study. J Neuropsychiatry Clin Neurosci. (2017) 29:70–3. doi: 10.1176/appi.neuropsych.16010013
146. Yang R, Zhang H, Wu X, Yang J, Ma M, Gao Y, et al. Hypothalamus-anchored resting brain network changes before and after sertraline treatment in major depression. Biomed Res Int. (2014) 2014:915026. doi: 10.1155/2014/915026
147. Garavan H, Pankiewicz J, Bloom A, Cho JK, Sperry L, Ross TJ, et al. Cue-induced cocaine craving: neuroanatomical specificity for drug users and drug stimuli. Am J Psychiatry (2000) 157:1789–98. doi: 10.1176/appi.ajp.157.11.1789
148. Hester R, Garavan H. Executive dysfunction in cocaine addiction: evidence for discordant frontal, cingulate, and cerebellar activity. J Neurosci. (2004) 24:11017–22. doi: 10.1523/JNEUROSCI.3321-04.2004
149. Kubler A, Murphy K, Garavan H. Cocaine dependence and attention switching within and between verbal and visuospatial working memory. Eur J Neurosci. (2005) 21:1984–92. doi: 10.1111/j.1460-9568.2005.04027.x
150. Tomasi D, Goldstein RZ, Telang F, Maloney T, Alia-Klein N, Caparelli EC, et al. Widespread disruption in brain activation patterns to a working memory task during cocaine abstinence. Brain Res. (2007) 1171:83–92. doi: 10.1016/j.brainres.2007.06.102
151. Bustamante JC, Barros-Loscertales A, Ventura-Campos N, Sanjuan A, Llopis JJ, Parcet MA, et al. Right parietal hypoactivation in a cocaine-dependent group during a verbal working memory task. Brain Res. (2011) 1375:111–9. doi: 10.1016/j.brainres.2010.12.042
152. Hinkle W, Cordell M, Leibel R, Rosenbaum M, Hirsch J. Effects of reduced weight maintenance and leptin repletion on functional connectivity of the hypothalamus in obese humans. PLoS ONE (2013) 8:e59114. doi: 10.1371/journal.pone.0059114
153. Grigg O, Grady CL. Task-related effects on the temporal and spatial dynamics of resting-state functional connectivity in the default network. PLoS ONE (2010) 5:e13311. doi: 10.1371/journal.pone.0013311
154. Wang ZJ, Liu JM, Zhong N, Qin YL, Zhou HY, Li KC. Changes in the brain intrinsic organization in both on-task state and post-task resting state. Neuroimage (2012) 62:394–407. doi: 10.1016/j.neuroimage.2012.04.051
155. Li CSR, Sinha R. Alexithymia and stress-induced brain activation in cocaine-dependent men and women. J Psychiatry Neurosci. (2006) 31:115–121.
156. Li CSR, Luo X, Sinha R, Rounsaville BJ, Carroll KM, Malison RT, et al. Increased error-related thalamic activity during early compared to late cocaine abstinence. Drug Alcohol Depend. (2010) 109:181–9. doi: 10.1016/j.drugalcdep.2010.01.008
157. Ide JS, Zhang S, Hu S, Sinha R, Mazure CM, Li CSR. Cerebral gray matter volumes and low-frequency fluctuation of BOLD signals in cocaine dependence: duration of use and gender difference. Drug Alcohol Depend. (2014) 134:51–62. doi: 10.1016/j.drugalcdep.2013.09.004
158. Zhang S, Hu S, Bednarski SR, Erdman E, Li CS. Error-related functional connectivity of the thalamus in cocaine dependence. Neuroimage Clin (2014) 4:585–92. doi: 10.1016/j.nicl.2014.01.015
Keywords: resting state functional connectivity, hypothalamus, cocaine, craving, fMRI
Citation: Zhang S, Wang W, Zhornitsky S and Li CR (2018) Resting State Functional Connectivity of the Lateral and Medial Hypothalamus in Cocaine Dependence: An Exploratory Study. Front. Psychiatry 9:344. doi: 10.3389/fpsyt.2018.00344
Received: 26 April 2018; Accepted: 09 July 2018;
Published: 27 July 2018.
Edited by:
Marco Diana, University of Sassari, ItalyReviewed by:
Diana Martinez, Columbia University, United StatesElliot Alan Stein, Intramural Research Program (NIDA), United States
Copyright © 2018 Zhang, Wang, Zhornitsky and Li. This is an open-access article distributed under the terms of the Creative Commons Attribution License (CC BY). The use, distribution or reproduction in other forums is permitted, provided the original author(s) and the copyright owner(s) are credited and that the original publication in this journal is cited, in accordance with accepted academic practice. No use, distribution or reproduction is permitted which does not comply with these terms.
*Correspondence: Sheng Zhang, c2hlbmcuemhhbmdAeWFsZS5lZHU=