- 1Centro de Biotecnología y Genómica de Plantas (CBGP, UPM-INIA), Universidad Politécnica de Madrid (UPM) – Instituto Nacional de Investigación y Tecnología Agraria y Alimentaria (INIA), Madrid, Spain
- 2Departamento de Biotecnología-Biología Vegetal. Escuela Técnica Superior de Ingeniería Agronómica, Alimentaria y de Biosistemas, Universidad Politécnica de Madrid (UPM), Madrid, Spain
- 3Facultad de Ciencias Ambientales y Bioquímica, Universidad de Castilla-La Mancha, Toledo, Spain
- 4Plant Response Biotech, Centro de Empresas, Madrid, Spain
- 5Semillas Fitó, Barcelona, Spain
- 6Plant Molecular Genetics Department, Centro Nacional de Biotecnología/CSIC, Madrid, Spain
Mitogen-activated protein kinases (MAPK) play pivotal roles in transducing developmental cues and environmental signals into cellular responses through pathways initiated by MAPK kinase kinases (MAP3K). AtYODA is a MAP3K of Arabidopsis thaliana that controls stomatal development and non-canonical immune responses. Arabidopsis plants overexpressing a constitutively active YODA protein (AtCA-YDA) show broad-spectrum disease resistance and constitutive expression of defensive genes. We tested YDA function in crops immunity by heterologously overexpressing AtCA-YDA in Solanum lycopersicum. We found that these tomato AtCA-YDA plants do not show developmental phenotypes and fitness alterations, except a reduction in stomatal index, as reported in Arabidopsis AtCA-YDA plants. Notably, AtCA-YDA tomato plants show enhanced resistance to the bacterial pathogen Pseudomonas syringae pv. tomato DC3000 and constitutive upregulation of defense-associated genes, corroborating the functionality of YDA in tomato immunity. This function was further supported by generating CRISPR/Cas9-edited tomato mutants impaired in the closest orthologs of AtYDA [Solyc08g081210 (SlYDA1) and Solyc03g025360 (SlYDA2)]. Slyda1 and Slyda2 mutants are highly susceptible to P. syringae pv. tomato DC3000 in comparison to wild-type plants but only Slyda2 shows altered stomatal index. These results indicate that tomato orthologs have specialized functions and support that YDA also regulates immune responses in tomato and may be a trait for breeding disease resistance.
Introduction
Plants rely on a two-tiered immune system to cope with the countless attempts of pathogens and pests to attack and colonize them. The first layer of this immune system consists of an extensive battery of membrane bound receptors, mainly receptor like kinases (RLKs) and receptor like proteins (RLPs), that are classified as pattern recognition receptors (PRRs). Successful recognition by these PRRs of highly conserved molecules from the pathogens (so-called, microbe-associated molecular patterns, MAMPs) activates MAMP-triggered immunity (MTI) that contributes to basal resistance by inhibiting pathogens colonization (Boutrot and Zipfel, 2017). Notably, PRRs can also perceive host (plant-self) derived molecules synthesized (e.g., peptides) or released upon pathogen infection or herbivory damage on plant tissues (e.g., plant cell wall derived glycans), that are referred as damage-associated molecular patterns (DAMPs), and activate complementary immune responses (DAMP-Triggered Immunity, DTI; Li et al., 2020). Pathogens have evolved mechanisms to inhibit immune responses by injecting effector proteins in plant cells to abolish defense signaling and resistance activation. To cope with this, plants have developed a second surveillance mechanism, named effector-triggered immunity (ETI), to sense these pathogen effectors through cytoplasmic PRRs encoded by the known resistance genes, which activate a more durable resistance response, that has been extensively used in crop protection breeding programs (Thordal-Christensen, 2020).
During MTI, DTI, and ETI activation, defense signaling is transmitted from the receptors to the nucleus, where a profound transcriptomic reprograming takes place, leading to the expression of genes that contributes to different resistance mechanisms [e.g., synthesis of antimicrobial metabolites and peptides/proteins, like pathogenesis related (PR) proteins, cell wall remodeling, and callose deposition; Albert et al., 2020]. Among the early downstream defense responses that are activated upon recognition of MAMP/DAMPs or effectors by PRRs are the production of reactive oxygen species (ROS), membrane ion fluxes, and activation of mitogen-activated protein kinases cascades (MAPKs or MPKs; Boutrot and Zipfel, 2017). MAPK cascades are central hubs in the plant immune signaling transduction since phosphorylation of proteins is one of the post-translational modifications, playing a major role in immune regulation and cell transcriptional reprograming (Bi and Zhou, 2017). Three levels of hierarchy operate in MAPK activation: MAPK kinase kinases (MAP3K/MEKKs) phosphorylate and activate MAPK kinases (MAPKK/MKKs) that later phosphorylate MAPKs. Activation of MAPKs will lead to phosphorylation of downstream targets, such as transcription factors or metabolic enzymes like those involved in synthesis of defensive phytohormones [e.g., salicylic acid (SA); Thulasi Devendrakumar et al., 2018].
In Arabidopsis and tomato, few PRR-MAPK modules involved in immunity have been described. For example, the FLAGELLIN-SENSITIVE 2 receptor (FLS2) that specifically recognizes the immunogenic MAMP peptide flg22, derived from the bacterial flagellin protein, activates a conserved cascade that involves the MAPKKK5, MKK4/MKK5, and MPK3/MPK6 (Liang and Zhou, 2018). Also, the signaling module composed by MEKK1, MKK1/MKK2, and MPK4 has been identified to be relevant in MTI (Ichimura et al., 2006; Suarez-Rodriguez et al., 2007; Gao et al., 2008; Kong et al., 2012). This cascade contributes to basal resistance and is guarded by the resistance (R) protein, SUMM2 (Zhang et al., 2012). Remarkably, this last mechanism highlights the relevance of MAPK cascades in immunity, as plants have developed a surveillance system to monitor that phosphorylation cascades are functional.
YODA (YDA) is a MAP3K from Arabidopsis thaliana that belongs to the MEKK family and together with MKK4/MKK5 and MPK3/MPK6 constitute a regulatory module controlling stomatal development (Bergmann et al., 2004). Perception of the extracellular peptides EPF1 and EPF2 by the receptor complex formed by the RLKs ERECTA (ER), ER-like family members ERL1 and ERL2, and brassinosteroid-associated kinase 1 (BAK1), and by the RLP Too Many Mouths (TMM), leads to a inhibition of stomatal development through the activation of YDA MAPK module (Lee et al., 2015; Meng et al., 2015). Accordingly, Arabidopsis yda mutants show an increase of leaf stomata and stomatal indexes (Bergmann et al., 2004; Sopeña-Torres et al., 2018). Remarkably, this ER/ERLs/TMM/BAK1-YDA signaling cascade has been demonstrated recently to operate also in Arabidopsis immunity, as mutants impaired in any of these molecular components are more susceptible to pathogens and are impaired in the activation of non-canonical immune pathways (Jordá et al., 2016; Sopeña-Torres et al., 2018). ER also controls in crops (e.g., tomato) other developmental processes, like internode length, rapid cycling, and compact plant size (Kwon et al., 2020), and some responses to stresses, such as thermotolerance (Shen et al., 2015).
N-terminal domain of some MAP3K has been shown to have a regulatory function on MAPKs activity. In fact, deletions of the N-terminal amino acids result in the constitutive activation of MAP3K (CA-MAP3K) based on the phenotypes observed in CA-MAP3K overexpressor lines. Tobacco and tomato plants expressing CA-MAP3Ks, such as tobacco MPK3α, show increased resistance to some pathogens, like the bacterium Pseudomonas syringae, but this phenotype was associated to the activation of programed cell death (PCD) that impacted negatively on plant fitness (Asai et al., 2002; del Pozo et al., 2004). Similarly, deletion of 184–322 amino acids of the N-terminal domain in Arabidopsis YDA (AtCA-YDA), leads to its constitutive activation and to a reduction of stomatal production in Arabidopsis plants (Lukowitz et al., 2004). Notably, AtCA-YDA plants are also highly resistant to a wide range of pathogens, including necrotrophic fungus, hemibiotrophic bacteria, and biotrophic oomycetes. Arabidopsis AtCA-YDA plants show constitutive expression of defensive genes that are not regulated by canonical immune pathways, like those mediated by defensive phytohormones, such as SA, jasmonic acid (JA), ethylene (ET), or MTI. Notably, AtCA-YDA plants do not show activation of PCD. These data led to suggest that YDA and their upstream PRRs (ER, ERL1, ERL2, TMM, and BAK1) regulate a novel non-canonical immune pathway conferring broad-spectrum disease resistance in Arabidopsis that could be of interest for breeding broad-spectrum disease resistance in crops (Molina et al., 2016; Sopeña-Torres et al., 2018).
To test whether YDA might play similar functions in crops immunity and to determine its biotechnological potential in breeding disease resistance, we overexpressed AtCA-YDA (35S::AtCA-YDA) in tomato (Solanum lycopersicum). Here, we show that constitutive expression of AtCA-YDA in tomato confers enhanced resistance against the bacterium P. syringae pv. tomato (Pto), supporting that YDA modulates immune responses in other plant species. The stomatal index was also found to be reduced in tomato 35S::AtCA-YDA lines further corroborating the functionality of YDA in stomatal regulation in crops. We also proved YDA function in tomato by generating CRISPR/Cas mutant lines in SlYDA1 and SlYDA2, the two closest orthologs of AtYDA, which were found to be highly susceptible to Pto, further demonstrating the relevant role of YDA in crops immunity.
Materials and Methods
Plant Growth Conditions
The Moneymaker (MM) cultivar of tomato (S. lycopersicum) was used as wild type in all the assays performed. Seeds were sown individually in pots with a mixture of peat moss:vermiculite (3:1). Plants grew at 24°C in the greenhouse with a light regime of 12 h. For in vitro growth assays, seedlings were surface sterilized by incubating them in bleach (100%) for 4 min, washed with sterilized water four times, and then plated onto MS media (Murashige and Skoog basal salt medium; Duchefa Biochemie).
Stable Transformation of Solanum lycopersicum
Moneymaker cultivar was transformed with Agrobacterium tumefaciens carrying the binary vector pGWB2 for AtYDA (CaMV35S::AtYDA; Nakagawa et al., 2007) and pB2GW7 for AtCA-YDA (CaMV35S::AtCA-YDA; Karimi et al., 2002) at the transformation service of University of California at Davis (United States) using its standard protocol.1 AtYDA tomato transgenic plants were selected on kanamycin/hygromycin plates (50 and 10 mg/l, respectively; Duchefa Biochemie), while AtCA-YDA plants were selected based on their resistance to the glufosinate herbicide. Homozygous lines were selected by PCR and the levels of transgene expression were also measured by qRT-PCR using the oligonucleotides depicted on Supplementary Table S9. Two independent homozygous lines transformed with AtCA-YDA were selected (#3 and #7) and two for AtYDA (#6 and #5). AtYDA line #5 does not show expression of the transgene and was used as control line. All the assays were performed with T3 plants.
Generation of CRISPR/Cas9 Lines of Solanum lycopersicum
Tandem gRNAs targeting two closely located sites were designed for both SlYDA1 and SlYDA2 under control of different U6 promoters. gRNAs were designed using the “Breaking Cas” online tool (Oliveros et al., 2016).2 SlYDA1 gRNAs (5'-CGAGCTGTCAGGAGCACTGT-3' and 5'-TGAACCCTGGTTTCTGGCTA-3') had an aggregated score of 98.9 (100 maximum score) and are targeting the third exon. SlYDA2 gRNAs (5'-CCTTCTTTTAGGAGATACGG-3' and 5'-CTGCAAAAGGCTCCAGGAGG-3') had an aggregated score of 97.5 and 99.5, respectively, and are targeting the second exon. All the potential off-targets had an individual score ≤0.5 with at least four mismatches within the 20-nt gRNA sequence selected. The tandem gRNAs for each gene were cloned together with the UBQ10/CAS9 sequence through triple LR cloning (Gateway system, Life Invitrogen) into the pB7m34GW,0 vector, which allow selection of the transgenic plants through BASTA/PPT. Both constructs have been used to transform MM cultivar using A. tumefaciens at the transformation service of the Universidad Politécnica de Valencia (UPV) following their standard transformation protocol. Transformants were selected on BASTA resistance, transplanted, and selfed for T2 seed production. T2 seeds were sown and selected in plantlet state by HRM PCR (High Resolution Melting; Roche LightCycler 480) for the presence of CRISPR induced mutations, based on the sequences of the gRNAs. Additionally, PCR analysis was performed to test the absence of the transgene. Selected plants were transplanted and selfed for T3 seed production. T3 plants were analyzed by Sanger sequencing (Macrogen sequencing Service) to confirm the homozygosity of the mutation and to characterize the modification. Based on the CRISPR/Cas9 induced mutations, CRISPR mutants #36 and #37 (two independent Slyda1 mutant lines of SlYDA1) and #23, #52, and #313 (three independent Slyda2 mutant lines of SlYDA2) were selected for further analysis.
Morphometric and Stomatal Abundance Parameters
As the fruits grew and ripened, they were picked and immediately their fresh weight was determined. Pictures of the tomato plants were taken at 18, 25, and 35 days after germination. Stomatal and pavement cell densities and stomatal indexes were scored in the terminal leaflet of the third leaf of 25-day-old plants. Leaflets were hand-excised, fixed, and clarified as indicated in Morales-Navarro et al. (2018) with minor modifications. Plant material was fixed in ethanol:acetic acid 9:1 (v/v) for 48 h, rehydrated through ethanol series, and cleared in a chloral hydrate:glycerol:water solution (8:1:2, w/v/v) for 48 h. The abaxial epidermis was inspected with differential interference contrast (DIC) under a Nikon Eclipse 90i upright microscope and a DXM1200C camera for image acquisition. Micrographs were analyzed with the free software Fiji Image J (Schindelin et al., 2012). Epidermal cell counts of each individual were an average from two areas of 0.4 mm2. Stomatal and pavement cell density were calculated as number of stomata or pavement cells per area unit (cell number mm−2), respectively, and the stomatal index as percentage of epidermal cells that were stomata (number of stomata/total number of epidermal cells × 100).
Pathogenicity Assays
Pseudomonas syringae pv. tomato DC3000 was grown for 36 h in King Agar B (KB) plates under dark conditions. Cells were scraped from the plates and suspended in 10 mM MgCl2 at a final concentration of 108 colony forming units (CFU)·ml−1, and 0.002% (vol/vol) Silwet L-77 was added prior inoculation. Three-week-old plants of wild-type genotype and of each transgenic (#3 and #7 of AtCA-YDA, #5 and #6 of AtYDA) or CRISPR/Cas9 mutant lines (#36 and #37 of Slyda1 and #23, #52, and #313 of Slyda2) were spray-inoculated with the bacterial suspension. Bacterial growth was determined at 3 h post inoculation (time 0) and 5 days post infection by averaging the CFU·cm−2 isolated from four infected plants. Leaf disks were homogenized in 10 mM MgCl2, and serial dilutions were plated on KB solid medium containing rifampicin (25 μg/ml; Duchefa Biochemie). These assays were repeated in triplicate.
RNA Extraction for Gene Expression Analyses
Total RNA was isolated from tomato leaves of at least three plants at the indicated days after sowing using an extraction buffer (0.2 M Tris pH 9; 0.4 M LiCl; 25 mM EDTA, and 1% SDS), followed by two phenol extraction steps and an 8 M LiCl precipitation. Two milligram of total RNA resuspended in milli-Q water was treated with DNaseI following the manufacturer’s protocol (TURBO DNAfree, Ambion). First-strand cDNA synthesis was performed with oligo-dT using Transcriptor First Strand cDNA Synthesis (Roche) following manufacturer instructions. SYBRGreen master mix (Roche) was used for qRT-PCR reactions that were performed in the LightCycler® 480 Instrument II (Roche) as reported previously (Jordá et al., 2016). UBC (Solyc03g123660) was used as tomato housekeeping gene. Primers used for expression analysis are listed in Supplementary Table S9. All the gene expression assays were performed at least three times.
RNA-seq Analyses
The terminal leaflet of the third leaf of 25-day-old plants was used to extract RNA. Total RNA was isolated as described in the previous section, from four independent individuals of MM, AtCA-YDA#3, AtCA-YDA#7, and AtYDA#6 lines, from two independent experiments, and subjected to RNA sequencing (n = 4 for AtCA-YDA lines and n = 2 for AtYDA lines). RNA was further purified using the RNeasy Mini Kit following manufacturer instructions (Qiagen). RNA samples were sequenced in the sequencing Core Facilities of the Center for Regulatory Genomics, CRG (Barcelona, Spain). Prior to sequencing quality control of RNA samples was performed with a Bioanalizer. Samples were sequenced with the Illumina HiSeq3000 (high output mode) with x50 tomato genome coverage using 1 × 50 bp reads. Raw data were aligned to the newly annotated S. lycopersicum genome with HISAT2 v2.1.0 (Kim et al., 2015). Then it was sorted with Samtools (Li et al., 2009) and differential expression was obtained with StringTie (Frazee et al., 2015; Pertea et al., 2015). Functional categories of the missregulated genes were obtained using Blast2Go pipeline (Götz et al., 2008) of the whole published annotation (data base from NCBI December 06, 2017). Gene ontology categories were done using the BINGO program (Maere et al., 2005).
Phylogenetic Analyses/Ortholog Search
MEGA5 program (Tamura et al., 2011) was used to perform the evolutionary analysis. The full length protein sequence of Ar. thaliana AtYODA (At1g63700), the putative orthologs from S. lycopersicum (SlYDA1, Solyc08g081210; SlYDA2, Solyc03g025360; and SlYDA3, Solyc06g03680) and some Solanum MAP3K (Solyc12g088940, Solyc09g047920, Solyc04g079400, Solyc02g090430, Solyc02g065110, Solyc04g064590, and Solyc03g117640) were aligned using MUSCLE and inferred using the neighbor-joining method. The protein sequence of Solyc06g005910 (tubulin β-chain) was used to root the tree. Identity and similarity calculations between the protein sequences were determined using Sequence Identity And Similarity (SIAS) tool and BLOSUM62 matrix.3
Statistical Methods
Normality of distributions was analyzed using Shapiro-Wilk normality test. Student’s t-test or the Mann-Whitney test analyses were performed with STATGRAPHICS CENTURION XVI (Statpoint Technologies, Warrenton, VA, USA).
Accession Numbers
RNA-seq read data have been submitted to the NCBI Sequence Read Archive (SRA) under accession PRJNA635132.
Results
Expression of AtCA-YDA in Tomato Does Not Affect Plant Fitness
Since overexpression of AtCA-YDA in Arabidopsis confers broad-spectrum disease resistance to pathogens and activates specific immune responses (Sopeña-Torres et al., 2018), we explored if CA-YDA protein could be also a positive regulator of immune responses in other plant species, like tomato (S. lycopersicum). We first heterologously expressed AtCA-YDA in tomato (MM) by generating plants constitutively overexpressing AtCA-YDA that were obtained by cloning AtCA-YDA coding sequence (harboring a deletion of the amino acids 184–322 at the N-terminal region) into the binary vector pB2GW7 (Karimi et al., 2002) under the control of the 35S cauliflower mosaic virus promoter (35SCaMV; 35S::AtCA-YDA lines; Figure 1A). Among the plant transformants generated, we selected for further characterization two independent AtCA-YDA tomato lines (AtCA-YDA #3 and AtCA-YDA #7) harboring a single insertion event based on segregation analyses. We determined the presence of the AtCA-YDA transgene on the transformant lines by PCR (Figure 1B), and we proved by qRT-PCR that AtCA-YDA was expressed in leaves of the transgenic lines at different developmental stages (Figure 1C). Since expression levels of MAP3K are fine-tuned, we assessed whether overexpression of AtCA-YDA could impact on the expression of the three S. lycopersicum YDA ortholog genes (Solyc03g025360, Solyc06g036080, and Solyc08g081210; Supplementary Figure S1). Phylogenetic analysis showed that Solyc08g081210 (SlYDA1), Solyc03g025360 (SlYDA2), and Solyc06g036080 (SlYDA3) are the closest AtYDA orthologs based on the alignment of full length proteins (Supplementary Figure S1), and their similarities to AtYDA ranged from 67.5% of SlYDA1 to 65.23% of SlYDA3 (Supplementary Figure S2). Solyc03g025360 and Solyc06g036080 (SlYDA2 and SlYDA3) seem to result from a gene duplication (Supplementary Figures S1, S2A). The N-terminal sequence of SlYDA2 showed higher similarity with the N-terminal of AtYDA than that of SlYDA1 that has some amino acid deletions in comparison to AtYDA sequence (Supplementary Figure S2A).
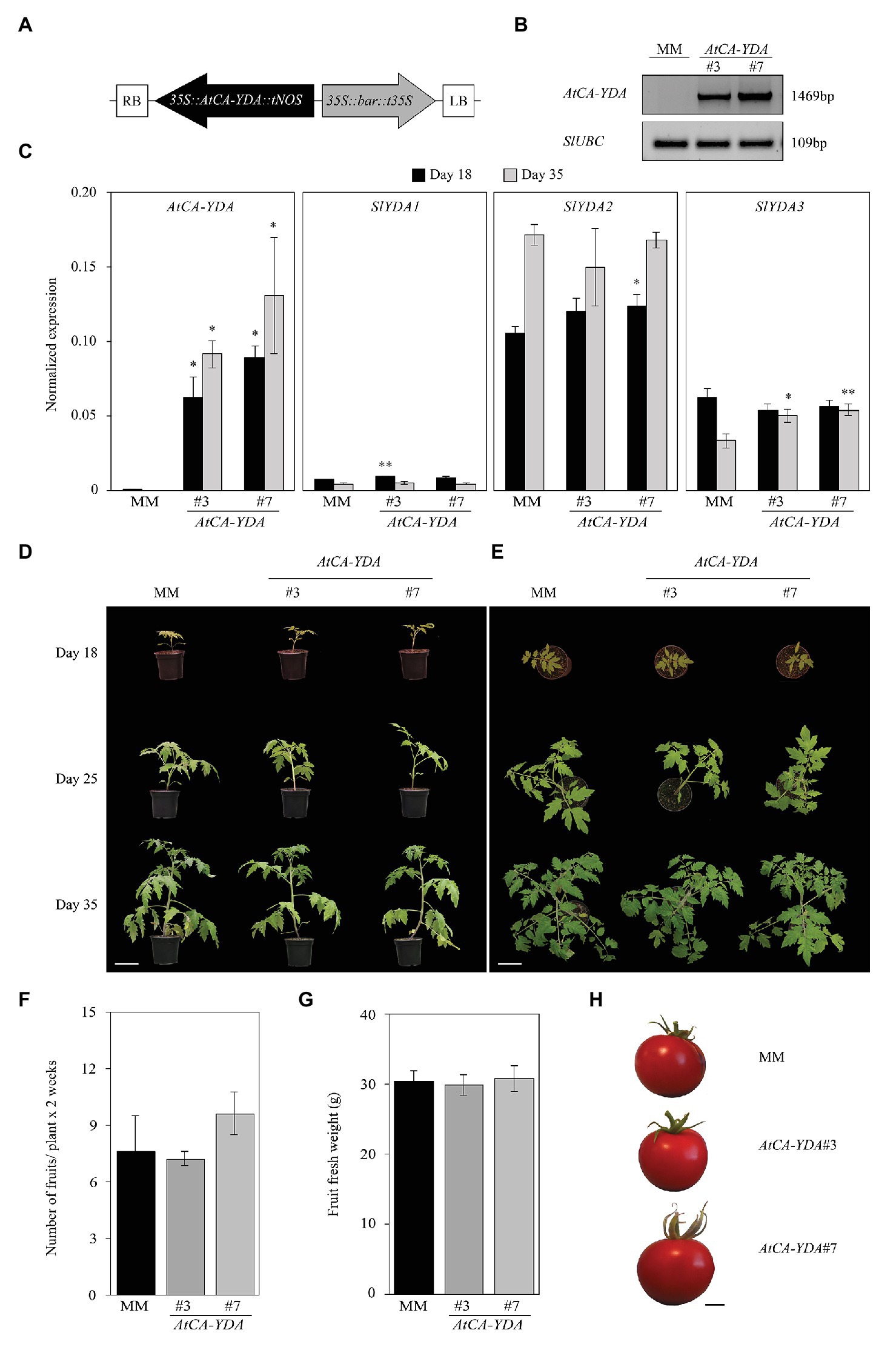
Figure 1. Expression of AtCA-YDA in tomato does not have deleterious effect on plant development. (A) Scheme of construction used for transformation of tomato plants with AtCA-YDA in pB2GW7 vector, driven by the CaMV 35S promoter (35S) and nopaline synthase terminator (tNOS). RB, right border sequence of T-DNA; LB, left border sequence of T-DNA; bar cassette to express the DL-phosphinothricin resistance gene driven by 35S and terminator t35S. (B) PCR detection of the transgene on Moneymaker (MM) wild-type plants and transgenic lines AtCA-YDA #3 and #7. SlUBC gene was PCR amplified and used as equal DNA loading control. (C) Expression of AtCA-YDA, SlYDA1 (Solyc08g081210), SlYDA2 (Solyc03g025360), and SlYDA3 (Solyc06g036080) in S. lycopersicum MM and transgenic AtCA-YDA plants (lines #3 and #7) at 18 and 35 days after sowing. Shown data are average expression of three independent experiments (n = 3) ± SE. Asterisks indicate statistical significance with respect to MM plants (Student’s t-test; *p < 0.05 and **p < 0.01). (D,E) Morphology of the indicated genotypes at 18, 25, and 35 days after sowing. Bar = 10 cm. (F) Number of fruits collected from the indicated lines (n = 12). (G) Fresh weight (grams/fruit) of the tomato fruits produced by AtCA-YDA #3 (n = 36) and #7 (n = 48) and MM plants (n = 58). These results are representative of at least three different experiments performed that gave similar results. (H) Representative phenotypes of fruits of MM and AtCA-YDA #3 and #7 plants. Bar = 1 cm.
Expression levels of these three genes, determined by qRT-PCR, were found to be very low in tomato leaves of wild-type MM plants. Overexpression of AtCA-YDA in the transgenic lines has minor effect on the transcription levels of SlYDA1 or SlYDA2, at any of the developmental stages analyzed, whereas SlYDA3 gene exhibited slight higher expression levels in these lines at 35 days post seed germination than in wild-type plants (Figure 1C).
We next assessed whether AtCA-YDA expression had any impact on tomato development. For that purpose, we monitored the growth of these plants and MM throughout their development and evaluated the production of fruits. We found that neither of the two tomato AtCA-YDA lines showed a penalty on plant or fruit development nor reduction in fruit yield per plant compared to wild-type (MM) plants (Figures 1D–H). We also tested the impact of AtCA-YDA overexpression on in vitro seed germination since YDA has been described to regulate Arabidopsis embryo development (Bergmann et al., 2004). We observed that seeds of the two independent AtCA-YDA lines showed a slight delay in reaching 100% germination in comparison to wild-type (MM) seeds (Supplementary Figure S3A), but they did not show any alteration in root or leaf development (Supplementary Figure S3B).
We also generated for comparison 35S::AtYDA tomato transgenic lines expressing the full-length protein by cloning the AtYDA full sequence into pGWB2 vector under the control of 35SCaMV (35S::AtYDA lines; Nakagawa et al., 2007; Supplementary Figure S4A). Several transformed lines were selected for further characterization, but after extensive analyses of AtYDA expression, we only identified one independent 35S::AtYDA line (AtYDA #6) with detectable expression levels of the transgene (Supplementary Figure S4C). Also, we selected as negative control for the analyses, an stable transformant line (AtYDA #5) that did not express the transgene (Supplementary Figures S4B,C). The expression of tomato SlYDA1-SlYDA3 genes in the 35S::AtYDA lines was not affected, with the exception of SlYDA3, whose expression was, like in the 35S::AtCA-YDA tested (Figure 1C), slightly higher in the transgenic line than in wild-type plants (Supplementary Figure S4C). Besides, no developmental phenotypic alterations and fruit yield penalties were found on the AtYDA #6 line expressing the transgene (Supplementary Figures S4D–G).
AtCA-YDA Expression Modulates Stomatal Development in Solanum lycopersicum
AtYDA regulates stomatal development and pattern in Arabidopsis. Guard cell formation is completely abolished in Arabidopsis AtCA-YDA homozygous plants (Lukowitz et al., 2004). To unveil, whether AtCA-YDA overexpression could lead to similar phenotypes on tomato, we analyzed stomatal abundance in the lines transformed with AtCA-YDA (Figure 2; Supplementary Table S1). The stomatal index (percentage of epidermal cells that are stomata) was reduced in the AtCA-YDA lines compared to the MM control plants (Figure 2A), indicating that expression of a constitutively active YDA protein was able to control the cell fate of tomato epidermal cells, repressing stomatal production as reported in Arabidopsis (Lukowitz et al., 2004). Significantly, the AtCA-YDA lines showed increased stomatal and pavement cell density compared to MM control plants (Figures 2B,C), indicating additional effects of AtCA-YDA on epidermal cell size and probably in the specification of epidermal cell fate. These results confirm that AtCA-YDA is functional in S. lycopersicum as stomatal development is affected by its constitutive overexpression.
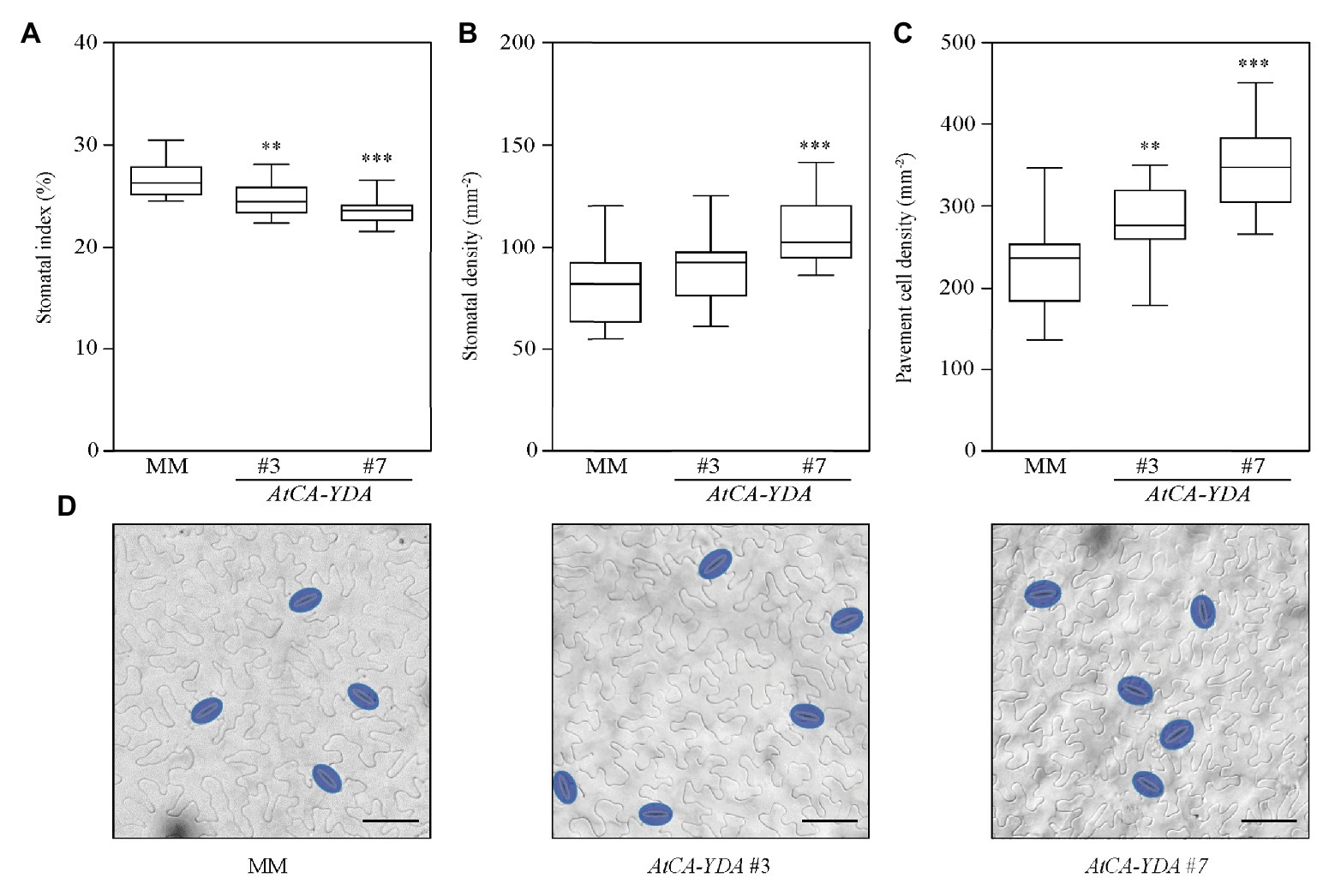
Figure 2. Tomato plants overexpressing AtCA-YDA show reduced stomatal index and higher epidermal cell density. (A–C) Stomatal index, stomatal density, and pavement cell density were analyzed in the abaxial epidermis of the mature third leaf from wild-type (MM) and transgenic AtCA-YDA plants (lines #3 and #7). The box plot diagram shows the median of the distribution as a center line, and the 25th and 75th percentiles are indicated by box limits. Whiskers show the minimum and maximum values. Data were obtained from 11 to 16 plants of each genotype. Asterisks indicate statistical significance with respect to MM plants (Student’s t-test; **p ≤ 0.01 and ***p ≤ 0.001). (D) Representative differential interference contrast (DIC) images of the epidermis scored in panels (A–C) leaves of plants from MM and two independent AtCA-YDA lines (#3 and #7), as indicated in the panels. Stomata are false-colored in blue. Bar = 50 μm. This experiment was performed twice and gave similar results. See also Supplementary Table S1.
AtCA-YDA Tomato Plants Are More Resistant Than Wild-Type Plants to the Bacterium Pseudomonas syringae pv. tomato
We next tested whether constitutive activation of AtYDA on tomato plants can enhance resistance, as it has been described in AtCA-YDA Arabidopsis plants (Sopeña-Torres et al., 2018). With that purpose, we spray-inoculated 3-week-old plants of two AtCA-YDA tomato lines and wild-type (MM) genotype with a suspension of the virulent bacteria P. syringae pv. tomato DC3000 (Pto), and bacterial growth was monitored at 3 h post inoculation (0 days post-inoculation, dpi), for determining equal bacterial inoculation in the tested genotypes, and at 5 dpi to characterize bacterial growth and infection. Remarkably, bacterial colony forming units (cfu/cm2) in the two AtCA-YDA tomato lines were significantly lower than in the wild-type plants (Figure 3A). Decrease in the bacterial growth was also associated with a reduction of disease symptoms caused by bacterial infection, which were lower in tomato AtCA-YDA lines than in MM (Figure 3B). This enhanced resistance phenotype of AtCA-YDA tomato lines was not due to a constitutive activation of canonical defense signaling pathways required for resistance to Pto (Betsuyaku et al., 2018; Liu et al., 2019), like those mediated by SA or ethylene (ET) or jasmonic acid (JA), since expression of tomato marker genes of these pathways (e.g., SlPR1, SlERF1, and SlPI-II, respectively) was not constitutively upregulated in mock-treated plants, as determined by qRT-PCR (Figure 3C). However, expression levels of SlPR1 and SlPI-II were higher at 24 hpi in AtCA-YDA lines than in inoculated wild-type plants (Figure 3C). Remarkably, 35S::AtYDA line #6 overexpressing AtYDA was also found to be slightly more resistant to Pto than wild-type plants or the 35S::AtYDA #5 line (not expressing the transgene) used as negative control (Supplementary Figure S5). Together, our results indicate that heterologous expression of AtCA-YDA has a significant impact on tomato immune responses and that YDA-mediated immune pathway is also conserved in this crop, as previously reported in Arabidopsis (Sopeña-Torres et al., 2018).
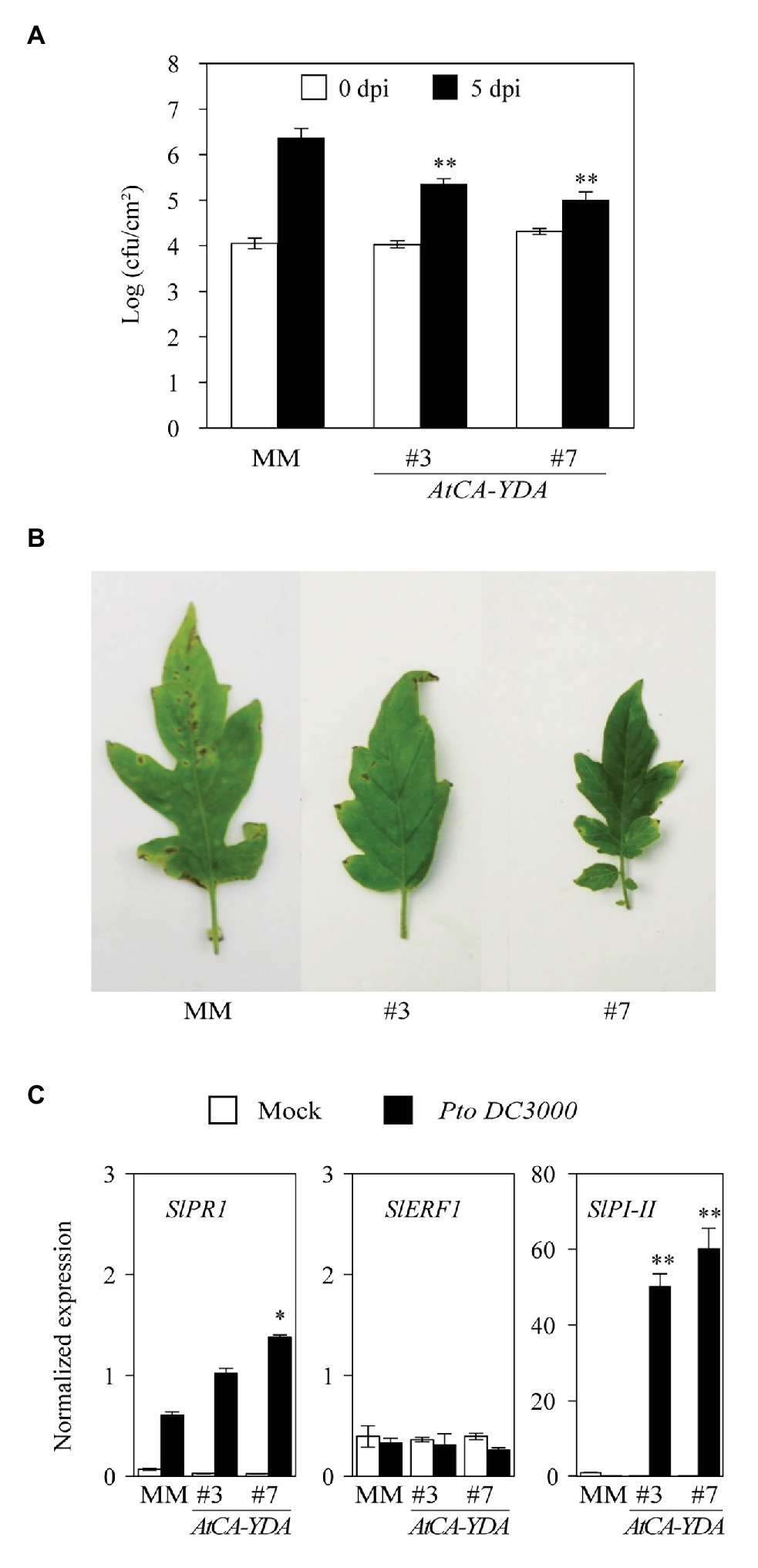
Figure 3. Tomato plants expressing AtCA-YDA show enhanced resistant to the pathogenic bacteria Pseudomonas syringae pv. tomato DC3000. (A) Quantification of bacterial growth at 0‐ and 5-day post-inoculation (dpi) with 108 cfu/ml of the bacterium. Values represent the mean (n = 12 ± SE) of three independent experiments. Asterisks indicate statistical significance with respect MM inoculated plants (Student’s t-test; **p < 0.01). (B) Macroscopic symptoms of bacteria inoculated leaves of the indicated genetic genotypes at 8 dpi. (C) Expression of SlPR1, SlERF1, and SlPI-II in response to Pto DC3000 inoculation (108 cfu/ml) or mock treatment (10 mM MgCl2). Leaf samples were collected at 24 hours post inoculation (hpi) and gene expression determined. SlUBC (Solyc03g123660) was used as internal reference gene. Data are the means (n = 4 ± standard errors) of the normalized gene expression. Statistical significance compared with MM plants was determined by Student’s t-tests: plants (*p < 0.05 and **p < 0.01). This experiment has been performed at least three times with similar results.
AtCA-YDA Expression in Tomato Induces the Transcriptional Activation of Defense Genes
To determine the basis of YDA-mediated resistance in tomato, we analyzed the transcriptomic reprograming that occurred in AtCA-YDA tomato transgenic lines. We performed a global RNA sequencing analysis (RNA-seq) on 4-week-old leaves from the two AtCA-YDA lines selected (#3 and #7) and wild-type plants. We identified a set of commonly differentially expressed genes (DEGs, n = 375, p ≤ 0.05, fold change ≥ 2) in the two AtCA-YDA lines tested in comparison to wild-type plants. Specifically, 324 DEGs were upregulated, while 51 were downregulated in both AtCA-YDA lines (Figures 4A,D; Supplementary Tables S2, S3). Functional classification of the DEGs identified some similarities between the functional categories of the genes upregulated both in tomato and Arabidopsis CA-YDA plants (Sopeña-Torres et al., 2018), like those related to translation, or RNA and ribosomal biogenesis, and response to stimuli (Figure 4C). Interestingly, other important category that is overrepresented in AtCA-YDA lines included defense-related genes, such as those encoding subtilisins, antimicrobial peptides, or resistance proteins (Figure 4C). qRT-PCR assays performed in independent experiments validated the differential expression of the upregulated genes tested, that encode proteins related with immunity, such as TIR-NBS-LRR disease resistance protein (SlDRP, Solyc02g077940) and Transducin (SlWD40, Solyc05g013880; Figure 4B). We also found 51 DEGs downregulated in both AtCA-YDA transgenic lines (Figure 4D), and two of them were validated by qRT-PCR (SlTPSI, Solyc03g098010 and SlAT, Solyc02g079490; Figure 4E). The classification into functional categories of the downregulated DEGs in AtCA-YDA lines identified genes involved in response to stimuli, signaling, and lipid metabolism (Figure 4F).
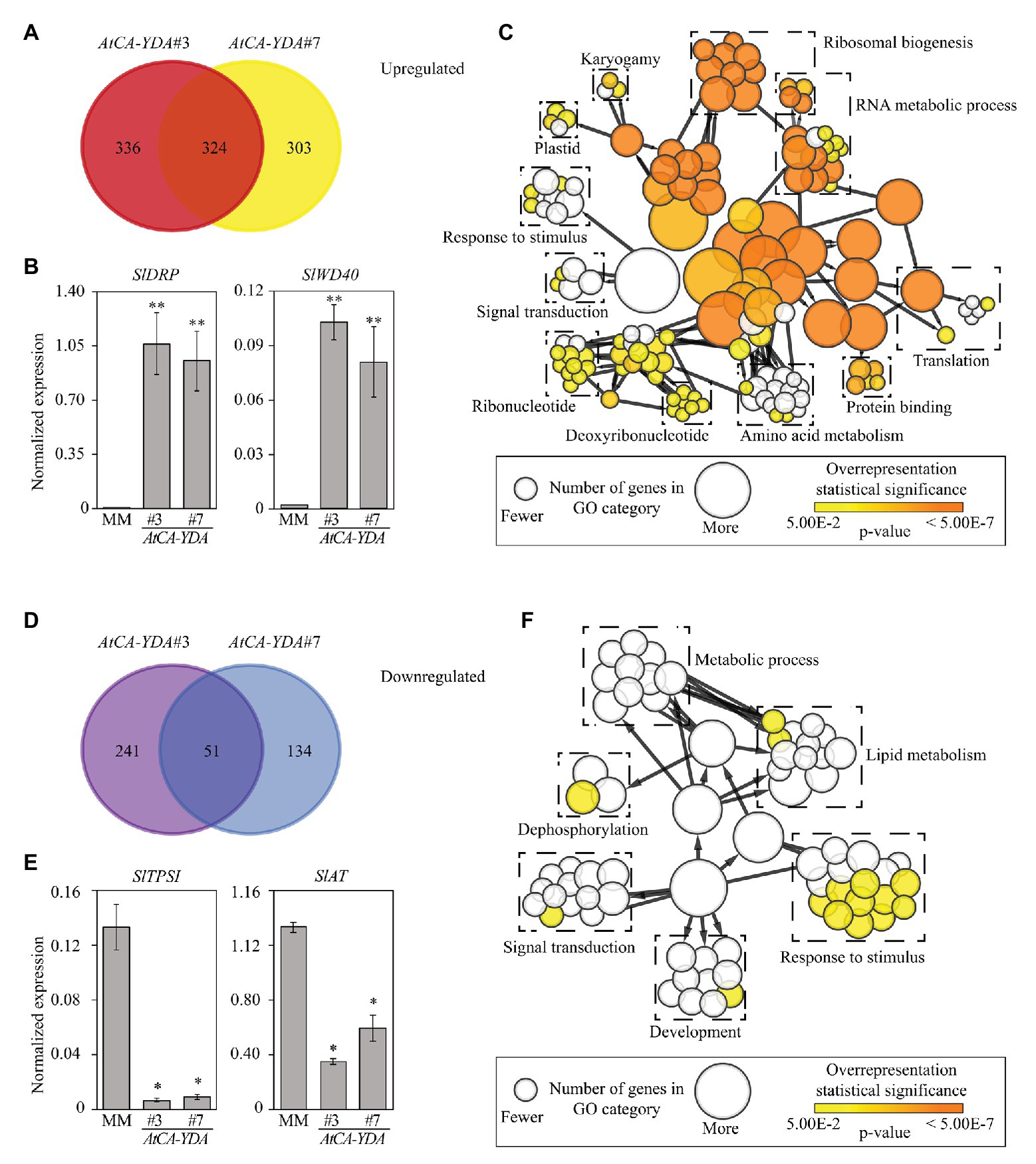
Figure 4. Expression of AtCA-YDA in tomato triggers the transcriptional regulation of defense genes. (A) Venn diagram showing overlapping of genes upregulated in AtCA-YDA lines (#3 and #7) compared to MM. Differential expression was defined as at least 2-fold change when compared with the non-transformed controls (MM). A minimal gene coverage value of 20 for each gene in RNAseq data was considered for selecting DEGs. (B) qRT-PCR analyses to validate the expression of upregulated genes in RNAseq data of AtCA-YDA plants. Expression levels of a disease resistance protein encoding gene (Solyc02g077940) and a transducin (Solyc05g013880) were analyzed and relativized to the SlUBC gene expression. Values are means (n = 6 ± SE) of the normalized gene expression. Statistical significance compared with MM plants was determined by Student’s t-tests (*p < 0.05 and **p < 0.01). (C) Functional category classification of the 324 DEGs upregulated in AtCA-YDA tomato lines. BINGO pipeline was used to assign the categories. Each significantly enriched GO term is represented with a circle, and the contribution of which is related to its diameter as indicated at the bottom inset. Significant enrichments according to the value of p are depicted in orange using the color code shown at the bottom right inset. Supplementary Table S2 depicts the genes included in this study. (D) Venn diagram of the DEGs downregulated in AtCA-YDA lines (#3 and #7) vs. wild-type (MM) plants. (E) qRT-PCR analyses to validate the expression of downregulated genes in RNAseq data of AtCA-YDA plants: phosphate starvation-induced gene (SlTPSI1, Solyc03g098010) and acetyl transferase encoding gene (SlAT, Solyc02g079490). Expression levels are normalized to the SlUBC (Solyc03g123660) gene. Values are means (n = 4 ± SE). Asterisks indicate mean values significantly different from wild-type plants (MM; Student’s t-test; *p < 0.05 and **p < 0.01). All expression analyses were performed at least three times with similar results. (F) Functional category classification of the 51 DEGs downregulated in the AtCA-YDA tomato lines using BINGO pipeline. Diameter of the circles indicates number of genes on each GO cellular component category enrichment and orange color indicates that the overrepresentation is statistically significant (p < 0.05). Supplementary Table S3 shows the genes downregulated in AtCA-YDA lines.
We took advantage of the generation of the 35S::AtYDA #6 line to determine if transcriptional reprograming regulated by AtCA-YDA and AtYDA proteins were similar, since this comparison has not been reported so far in Arabidopsis. Global RNA sequencing analysis (RNA-seq) was performed with the AtYDA #6 line. Of 934 genes found to be differentially expressed (777 up-expressed and 157 down-expressed) in this line (Supplementary Tables S4, S5) only 77 genes (43 upregulated and 34 downregulated) showed similar pattern of expression in AtCA-YDA lines, indicating that the transcriptional activation regulated by these two protein versions might be quite different (Supplementary Tables S6, S7). A core of 43 genes were found to be upregulated in AtCA-YDA and AtYDA lines, which were mainly grouped into gene ontology terms related with translation and defense, but also included genes with unknown function (Supplementary Figures S6A,B; Supplementary Table S5). Among the 34 genes downregulated in AtCA-YDA and AtYDA lines, we could identify a set of genes involved in stress responses, like phosphate starvation and defense response, including a gene of MLO family. This family has been demonstrated to have a negative function in the modulation of crops resistance to powdery mildew, that is enhanced in crops harboring mlo mutations (Kusch and Panstruga, 2017; Supplementary Figures S6C,D; Supplementary Table S7).
Disruption of the Two Closest Orthologs of AtYDA Genes in Tomato (SlYDA1 and SlYDA2) Leads to Enhanced Susceptibility to Pseudomonas syringae pv. tomato DC3000
To further confirm the relevance of YDA-mediated pathway in tomato disease resistance responses, we next assessed the role of the two closest AtYDA orthologs in tomato, SlYDA1 and SlYDA2 (Supplementary Figures S1, S2). For that purpose, we generated null mutants using CRISPR/Cas9 genome editing-technology in the Moneymaker tomato variety. Two independent mutant lines were obtained for SlYDA1 (Slyda1 Lines #36 and #37), while three CRISPR alleles were identified for SlYDA2 (Slyda2 Lines #23, #52, and #313). DNA sequence analysis allowed to confirm the mutations in these lines that lead to a shift in the open reading frame that resulted in premature stop codons and a truncated YDA protein in all the CRISPR/Cas9 lines generated (Figure 5A). Further analysis of Slyda lines showed that all mutations were stably transmitted to the offspring. Notably, mutations in SlYDA1 led to plants with reduced height and biomass at early stages of their developmental cycle in comparison to wild-type plants, although Slyda1 mutants were able to complete their cycle and set viable fruits. In contrast, mutations in SlYDA2 had no detrimental impact on tomato development and fitness (Supplementary Figure S7).
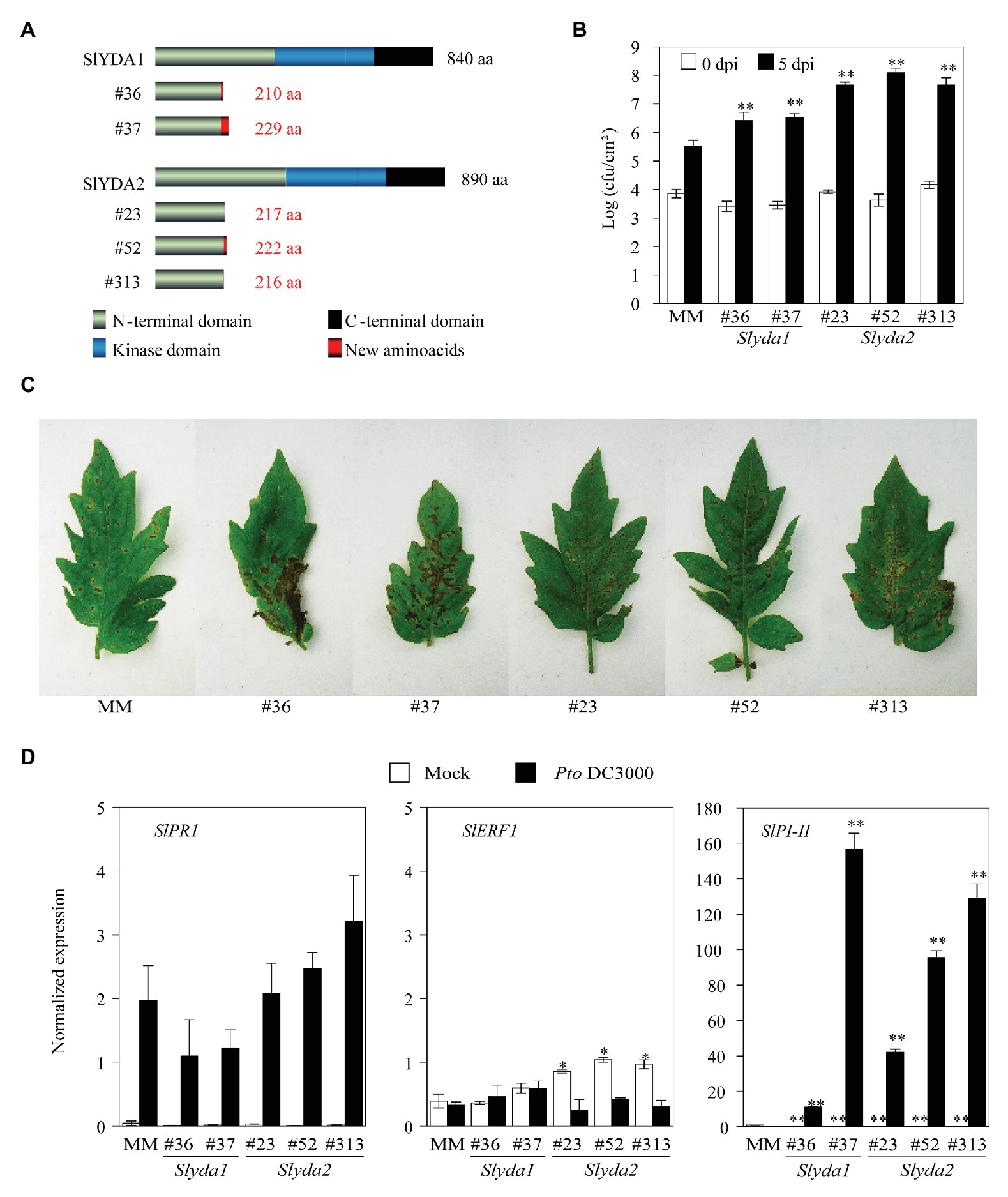
Figure 5. Mutations of SlYDA orthologs lead to enhanced susceptibility to Pseudomonas syringae. (A) Diagram of mutations generated in SlYDA1 and SlYDA2 genes by CRISPR/Cas9. (B) Growth of spray-inoculated P. syringae pv. tomato (Pto) DC3000 (108 CFU ml−1) in 21-day-old tomato plants of the indicated genotypes. Bacterial numbers were determined at 0 and 5 dpi. Values represent the mean (n = 12) ± SE of three independent experiments. Asterisks indicate mean values significantly different from MM control plants (Student’s t-test; *p < 0.05 and **p < 0.01). (C) Macroscopic symptoms of Pto DC3000-inoculated leaves at 8 dpi. Experiments were performed three times with similar results. (D) Defense gene expression analysis in MM, Slyda1, and Slyda2 plants mock‐ or bacterial-inoculated. Leaf samples were collected at 24 hpi and expression of genes was analyzed by qRT-PCR. Expression levels are relative to SlUBC gene. Values represent means (n = 6) ± SE from three independent biological replicates. Asterisks indicate mean values significantly different from expression in MM plants (Student’s t-test; *p < 0.05 and **p < 0.01).
We next evaluated the defense response of these Slyda1 and Slyda2 mutant lines to the bacterial pathogen Pto DC3000. Notably, mutations in both tomato YDA genes resulted in plants highly susceptible to this bacterium (Figure 5B) since the number of cfu/cm2, as well as leaf disease symptoms, were significantly enhanced in these mutants in comparison to wild-type plants (Figures 5B,C). This enhanced susceptibility was not due to an impairment in the induction of the expression of SA, ET, or JA-mediated defense genes, since the expression of the JA marker gene SlPI-II was enhanced in the Slyda1/Slyda2 lines upon infection, probably due to a faster bacterial colonization of these plants in comparison to wild-type plants, whereas the expression of SlPR1 and SlERF1 upon infection did not differ from that of wild-type plants (Figure 5D). Moreover, the expression of these defensive marker genes in non-inoculated mutants did not differ from that of wild-type plants with the exception of SlERF1 that showed a slightly higher expression in Slyda2 lines (Figure 5D).
To further confirm the functionality of the tomato YDA genes, we determined the impact of SlYDA1 and SlYDA2 loss of function on stomatal development, and we found that Slyda2, but not Slyda1 lines, showed a higher stomatal index and occasional stomatal clusters (mainly paired stomata) in comparison to wild-type plants (Figure 6; Supplementary Table S8), indicating that SlYDA2 is a negative regulator of stomatal formation and enforces correct stomatal patterning, as it has been described for AtYDA (Bergmann et al., 2004; Lukowitz et al., 2004). In the Slyda2 lines, the higher stomatal index phenotype was associated with a significantly higher stomatal density, without changes on pavement cell density. In contrast, mutations in SlYDA1 led to increased pavement cell density, which was translated into a higher stomatal density, but not in a higher stomatal index. These results suggest that SlYDA1 and SlYDA2 play distinct and specialized roles in stomatal development in tomato and that Slyda2 mutants show similar developmental/disease resistance phenotypes to those described in Arabidopsis yda mutants (e.g., yda11; Sopeña-Torres et al., 2018).
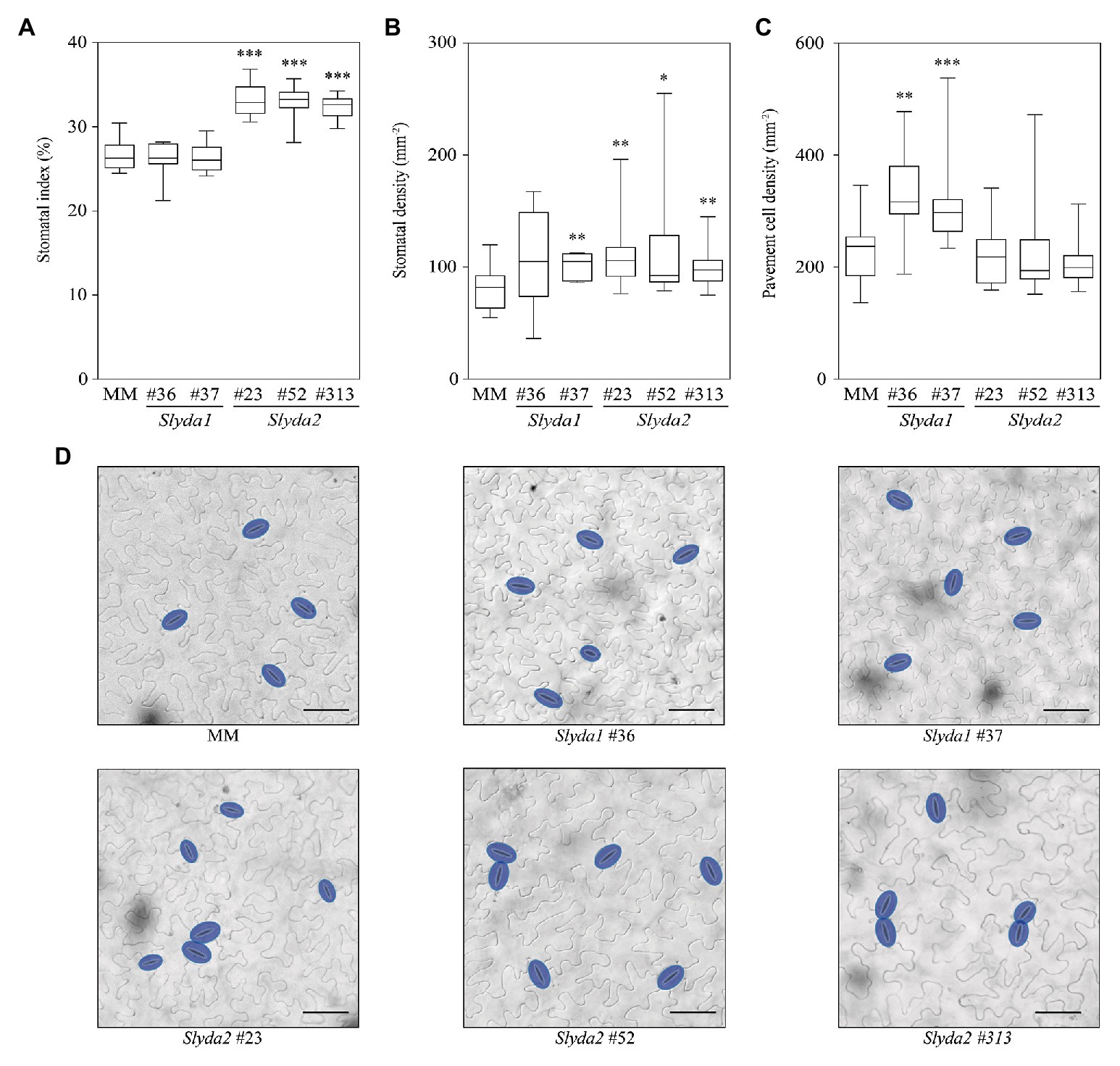
Figure 6. SlYDA2 regulates stomatal development in tomato. (A–C) Stomatal index, stomatal density, and pavement cell density were determined in the abaxial epidermis of the mature third leaf from wild-type (MM) and Slyda1 and Slyda2 plants. The box plot diagram shows the median of the distribution as a center line, and the 25th and 75th percentiles are indicated by box limits. Whiskers show the minimum and maximum values. Data were obtained from 13 to 16 plants of each genotype, except for Slyda1 lines #36 (n = 7) and #37 (n = 9). Pairwise phenotypic differences were analyzed by Mann-Whitney test, since data did not meet parametric test assumptions. Asterisks indicate statistical significance with respect to MM plants (*p ≤ 0.05, **p ≤ 0.01, and ***p ≤ 0.001). (D) Representative differential interference contrast (DIC) images of the epidermis scored in panels (A–C) of the indicated genotypes. Stomata are false colored in blue. Bar = 50 μm. See also Supplementary Table S8.
Discussion
Plants have a robust innate immune system that relies on several layers of pathogens perception that active defensive response to cope with pathogens colonization. PRRs, that are responsible of perceiving microbial MAMPs and host derived DAMPs, are widely distributed in the genomes of plant species. However, some PRRs are only present in the genomes of certain plant families, such as it occurs with the elongation factor receptor (EFR), an RLK involved in the perception of the bacterial EF-Tu MAMP that is exclusively present in Brassicaceae genomes (Lacombe et al., 2010). Due to the relevant roles of PRRs in pathogen perception and immune activation, the genetic transfer among plant species of PRRs, mainly RLKs, by transgenic overexpression has resulted in a successful biotechnological strategy to engineer broad-spectrum disease resistance in crops (Lacombe et al., 2010; Lu et al., 2015). Also, it has been shown that overexpression in some crops of PRRs of receptor like proteins (RLP) type, lacking the cytoplasmic kinase domain, from other species confers enhanced resistance to pathogens, such as it occurs in potato (Solanum tuberosum) expressing the Arabidopsis receptor-like protein 23 that recognizes the MAMP nlp20 from Phytophthora infestans (Albert et al., 2015). The success of these crop protection strategies relies on the highly conserved downstream components regulating MTI signaling pathways among plant species, which are activated upon MAMPs perception by heterologous overexpressed PRRs. In this context, MAPK cascades play a relevant role as they are convergent nodes of the signaling events that take place upon MAMP/DAMP recognition (Zhang et al., 2018). Arabidopsis genome has 80 annotated MAP3K, while tomato has 89 (Wu et al., 2014). MAPK signaling cascades are of paramount importance in activation of plant immunity, and accordingly they are major targets of pathogen effectors, such as the P. syringae HopAI1 and HopF2 or P. infestans PexRD2 effectors, to abolish the activation of plant defense responses (Zhang et al., 2007; King et al., 2014).
YDA, that encodes a MAP3K with very high similarity to members of the sterile 11/MAP/ERK kinase (STE11/MEKK) family, regulates several developmental processes (Bergmann et al., 2004) and a non-canonical immune pathway in Arabidopsis that upon activation can confer broad-spectrum disease resistance (Sopeña-Torres et al., 2018). In Arabidopsis, YDA functions downstream of the PRR complex formed by ER/ERLs/TMM/BAK1 to regulate both stomatal development and immune responses (Bergmann et al., 2004; Sopeña-Torres et al., 2018). The function of YDA in other plant species has not been determined, whereas it has been shown that ER RLK controls in crops, like tomato and rice, similar developmental processes to those regulated in Arabidopsis, but also some responses to stresses, like thermotolerance, making ER an attractive trait for breeding (Shen et al., 2015; Kwon et al., 2020). Moreover, the transcriptional factors (e.g., SPCH and MUTE) controlling ER-mediated responses (e.g., stomatal development) are conserved in crops like tomato, and the orthologs from Arabidopsis are functional in tomato (Ortega et al., 2019). Based on these previous data, it could be anticipated that YDA might regulate ER-mediated pathway in other plant species. This hypothesis has been corroborated here by overexpressing AtCA-YDA in tomato and by demonstrating the role of tomato orthologs of AtYDA (SlYDA1 and SlYDA2) in regulating immune responses (i.e., SlYDA1 and SlYDA2) and stomatal development (i.e., SlYDA2) in tomato plants.
To characterize the function of YDA in the regulation of crops immune responses, we have generated tomato lines that express the constitutive active MAP3K YDA from Arabidopsis (CA-YDA) that has been previously reported to confer broad-spectrum disease resistance to pathogens in Arabidopsis (Sopeña-Torres et al., 2018). Here, we demonstrate that heterologous expression of AtCA-YDA renders tomato plants more resistant to the bacterial pathogen Pto (Figure 3). These results strongly support the described function of YDA in plant immunity (Sopeña-Torres et al., 2018) that has been questioned by some previous results that described the antagonistic interaction of YDA on the immune pathway mediated by MAP3K3/5 (Sun et al., 2018). Remarkably, AtCA-YDA tomato lines (#3 and #7) showed a significant reduction in the stomatal index compared to wild-type plants (Figure 2), in accordance with the phenotype observed in Arabidopsis CA-YDA plants (Bergmann et al., 2004). All these results indicate that AtCA-YDA is functional in tomato plants and that all the downstream and upstream molecular components needed for the proper activation of the signaling pathway mediated by YDA, to control immune responses and stomatal development and patterning, are conserved between tomato and Arabidopsis.
Approaches to overexpress MAP3Ks in different plant species have been conducted previously to obtain plants with enhanced resistance to pathogens. However, many of these transgenic lines showed constitutive programed cell death activation, associated to the expression of SA-marker genes (e.g., SlPR1), and developed necrotic lesions on leaves that make unfeasible its possible biotechnological application as a strategy to obtain plants with enhanced resistance (Asai et al., 2002; del Pozo et al., 2004; Yamada et al., 2016). Notably, the tomato lines overexpressing AtCA-YDA protein do not show either developmental alterations or spontaneous necrotic lesions, and in accordance these lines do not have fitness or fruit yield penalties (Figure 1; Supplementary Figure S3). Therefore, overexpression of YDA in tomato might potentially be used as a strategy to increase disease resistance.
In Arabidopsis, deletion of certain amino acids from the N-terminal domain causes the constitutive activation of the MAP3K YDA (Lukowitz et al., 2004), and consequently a deep transcriptional reprograming and the constitutively upregulation of non-canonical defensive genes (Sopeña-Torres et al., 2018). Among the genes whose expression is upregulated by YDA activation in Arabidopsis, there are genes encoding antimicrobial peptides or R proteins (Sopeña-Torres et al., 2018). In tomato AtCA-YDA plants, at least 10.5% (34 genes) of the upregulated DEGs have a direct contribution to plant immune responses, and this set of DEGs includes some genes encoding antimicrobial peptides, such as thionins, lipid transfer proteins, chitinases, or cathepsin D inhibitors (García-Olmedo et al., 1998; Lisón et al., 2006). Besides, AtCA-YDA heterologous expression in tomato triggered the induction of three subtilases (e.g., P69C; Supplementary Table S2), which are extracellular proteases that have been described to exert a relevant role in responses to biotic stresses (Jordá et al., 1999; Schaller et al., 2018). In fact, overexpression of tomato P69C ortholog in Arabidopsis (AtSBT3.3) results in chromatin remodeling that concomitantly leads to an immune priming state (Ramírez et al., 2013). Interestingly, expression of some R genes was also upregulated on AtCA-YDA tomato plants (Figure 4; Supplementary Table S2). Though, it has been previously described that the constitutive overexpression of some R genes can result in constitutive upregulation of defense responses (Stokes and Richards, 2002), we did not find among the tomato AtCA-YDA DEGs defensive markers of canonical resistance pathways, like those regulated by the main defensive hormones (e.g., SA, ET, or JA). This is in line with our previous finding that the broad-spectrum resistance of Arabidopsis AtCA-YDA plants was not due to the activation of canonical immune pathways (Sopeña-Torres et al., 2018), further supporting that the ER-YDA signaling regulates a novel immune pathway. Notably, among the upregulated DEGs in tomato AtCA-YDA lines, there is a relevant number of genes that belongs to a functional category related with translation and protein folding (38.6% of genes), suggesting that the constitutive expression of AtCA-YDA might have an impact on cells reprograming for efficient protein translation. These data might support a function of YDA in the control of translation initiation in plants under biotic stress conditions, as it has been recently described for heat stress through YDA interaction with HSP90.1 protein (Samakovli et al., 2020). The transcriptional reprograming observed in tomato AtCA-YDA lines would explain the enhanced disease resistance observed in the transgenic tomato lines.
Arabidopsis has a unique YODA gene, while in rice and Brachypodium at least two YDA homologues have been identified (McKown and Bergmann, 2020). Tomato has at least three YODA like MAP3K close orthologs (Supplementary Figure S1) probably arisen by gene duplication and subsequent specialization. Using CRISPR strategy, we have been able to determine that SlYDA1 is mainly involved in meristem development, as lines impaired in this member of the family showed aberrant phenotypes at early stages of development that are attenuated at later developmental stages (Supplementary Figure S7), while SlYDA2 has functions, non-redundant with SlYDA1, in regulating stomatal development (Figure 6). Interestingly, mutations in SlYDA1 and SlYDA2 render plants highly susceptible to the bacterium Pto (Figure 5), indicating that both genes are relevant to activate a proper immune response that is independent of the SA, JA, and ET-mediated responses that are not defective in tomato CRISPR lines as revealed by the expression levels of marker genes of these canonical defensive pathways (Figure 5). However, the contribution of SlYDA1 and SlYDA2 to resistance seems to be different since Slyda2 mutants are significantly more susceptible to Pto than Slyda1 mutants. Future work will be required to unveiling their specific roles in tomato immunity, as well as the contribution of these two genes to tomato resistance to pathogens with other colonization styles. The stomatal development and immunity-associated phenotypes of Slyda2 mutant lines resemble those of Arabidopsis yda11 hypomorphic allele (Sopeña-Torres et al., 2018), suggesting that SlYDA2, like AtYDA, regulates stomatal formation and immunity. This would indicate that SlYDA2 has in tomato a very similar function than AtYDA in Arabidopsis, which is in line with similarities of their N-terminal regulatory domains (Supplementary Figure S2A). We have previously suggested that regulation of these two processes (immune responses and stomatal development) was co-opted during evolution since appearance of stomata increased the potential entry points of pathogens in plants (Sopeña-Torres et al., 2018). The results obtained with Slyda2 mutants and AtCA-YDA overexpressing lines are in accordance with this hypothesis.
Arabidopsis plants with different levels of expression of AtYDA show detrimental phenotypes, since yda null mutants (e.g., yda 1) are embryo lethal and do not complete life cycle, and plants overexpressing AtCA-YDA are viable just in heterozygosis (Bergmann et al., 2004; Sopeña-Torres et al., 2018). Slyda1 mutant lines show some phenotype alterations at early stages of development (Supplementary Figure S7). Remarkably, tomato Slyda2 and AtCA-YDA do not show alterations in fitness and fruit yield under our growth conditions, indicating that YDA might be a target trait for breeding crop protection, particularly in crops harboring several YDA orthologs in their genomes. Also, the identification of natural variants of YDA allele with increased activity of SlYDA2 might have technological impact since we could obtain tomato plants with broad-spectrum disease resistance without fitness alterations and yield penalties.
Data Availability Statement
RNA-seq read data have been submitted to the NCBI Sequence Read Archive (SRA) 283 under accession PRJNA635132.
Author Contributions
AMo and LJ designed the study and wrote the manuscript with input from all authors. SS-T generated the 35S::AtCA-YDA and 35S::AtYDA constructs for tomato transformation. Plant Response Biotech (RP, YS, and MB) generated the Solanum transformed with 35S::AtCA-YDA and 35S::AtYDA. Semillas Fitó (TJ and AMa) generated the tomato CRISPR/Cas9 lines in collaboration with MN, who did the constructs. JT performed the phenotypic characterization of the transgenic lines and the validation of the RNA seq. JT and LJ did the pathogen infection experiments. LJ did the gene expression analysis after Pto inoculations. AM-F, AO, and MM performed the stomata counting assays. AM-B analyzed the RNAseq data. All authors contributed to the article and approved the submitted version.
Funding
This work was supported by grants BIO2015-64077-R of the Spanish Ministry of Economy and Competitiveness (MINECO), RTI2018-096975-B-I00 of Spanish Ministry of Science, Innovation and Universities, and grant P190050072 of Plant Response Biotech SL to AMo. Research in the Montaña Mena’s laboratory is supported by the MINECO (PPII10-0194-4164) and the Junta de Comunidades de Castilla-La Mancha (SBPLY/17/180501/000394), complemented with EU FEDER funds. JT was financially supported by a PhD fellow of the FPI program from MINECO (BES-2016-076708). AM-F and AO were recipients of research and PhD fellowships from JCCM.
Conflict of Interest
AMo, LJ, and SS-T are inventors on the patent (US14/652,285) claiming the use of YDA for improving crop resistance. AMo is member of the scientific advisory board of Plant Response Biotech S.L. Authors RP, YS, and MB were employed by the company Plant Response Biotech, and AMa and TJ were employed by the company Semillas Fitó.
The remaining authors declare that the research was conducted in the absence of any commercial or financial relationships that could be construed as a potential conflict of interest.
Supplementary Material
The Supplementary Material for this article can be found online at: https://www.frontiersin.org/articles/10.3389/fpls.2020.584471/full#supplementary-material
Footnotes
References
Albert, I., Böhm, H., Albert, M., Feiler, C. E., Imkampe, J., Wallmeroth, N., et al. (2015). An RLP23-SOBIR1-BAK1 complex mediates NLP-triggered immunity. Nat. Plants 1:15140. doi: 10.1038/nplants.2015.140
Albert, I., Hua, C., Nürnberger, T., Pruitt, R. N., and Zhang, L. (2020). Surface sensor systems in plant immunity. Plant Physiol. 182, 1582–1596. doi: 10.1104/pp.19.01299
Asai, T., Tena, G., Plotnikova, J., Willmann, M. R., Chiu, W. L., Gomez-Gomez, L., et al. (2002). MAP kinase signalling cascade in Arabidopsis innate immunity. Nature 415, 977–983. doi: 10.1038/415977a
Bergmann, D. C., Lukowitz, W., and Somerville, C. R. (2004). Stomatal development and pattern controlled by a MAPKK kinase. Science 304, 1494–1497. doi: 10.1126/science.1096014
Betsuyaku, S., Katou, S., Takebayashi, Y., Sakakibara, H., Nomura, N., and Fukuda, H. (2018). Salicylic acid and jasmonic acid pathways are activated in spatially different domains around the infection site during effector-triggered immunity in Arabidopsis thaliana. Plant Cell Physiol. 59:439. doi: 10.1093/pcp/pcy008
Bi, G., and Zhou, J. M. (2017). MAP kinase signaling pathways: a hub of plant-microbe interactions. Cell Host Microbe 21, 270–273. doi: 10.1016/j.chom.2017.02.004
Boutrot, F., and Zipfel, C. (2017). Function, discovery, and exploitation of plant pattern recognition receptors for broad-spectrum disease resistance. Annu. Rev. Phytopathol. 55, 257–286. doi: 10.1146/annurev-phyto-080614-120106
del Pozo, O., Pedley, K. F., and Martin, G. B. (2004). MAPKKKalpha is a positive regulator of cell death associated with both plant immunity and disease. EMBO J. 23, 3072–3082. doi: 10.1038/sj.emboj.7600283
Frazee, A. C., Pertea, G., Jaffe, A. E., Langmead, B., Salzberg, S. L., and Leek, J. T. (2015). Ballgown bridges the gap between transcriptome assembly and expression analysis. Nat. Biotechnol. 33, 243–246. doi: 10.1038/nbt.3172
Gao, M., Liu, J., Bi, D., Zhang, Z., Cheng, F., Chen, S., et al. (2008). MEKK1, MKK1/MKK2 and MPK4 function together in a mitogen-activated protein kinase cascade to regulate innate immunity in plants. Cell Res. 18, 1190–1198. doi: 10.1038/cr.2008.300
García-Olmedo, F., Molina, A., Alamillo, J. M., and Rodríguez-Palenzuéla, P. (1998). Plant defense peptides. Biopolymers 47, 479–491.
Götz, S., García-Gómez, J. M., Terol, J., Williams, T. D., Nagaraj, S. H., Nueda, M. J., et al. (2008). High-throughput functional annotation and data mining with the Blast2GO suite. Nucleic Acids Res. 36, 3420–3435. doi: 10.1093/nar/gkn176
Ichimura, K., Casais, C., Peck, S. C., Shinozaki, K., and Shirasu, K. (2006). MEKK1 is required for MPK4 activation and regulates tissue-specific and temperature-dependent cell death in Arabidopsis. J. Biol. Chem. 281, 36969–36976. doi: 10.1074/jbc.M605319200
Jordá, L., Coego, A., Conejero, V., and Vera, P. (1999). Genomic cluster containing four differentially regulated subtilisin-like processing protease genes is in tomato plants. J. Biol. Chem. 274, 2360–2365.
Jordá, L., Sopeña-Torres, S., Escudero, V., Nuñez-Corcuera, B., Delgado-Cerezo, M., Torii, K. U., et al. (2016). ERECTA and BAK1 receptor like kinases interact to regulate immune responses in Arabidopsis. Front. Plant Sci. 7:897. doi: 10.3389/fpls.2016.00897
Karimi, M., Inzé, D., and Depicker, A. (2002). GATEWAY vectors for agrobacterium-mediated plant transformation. Trends Plant Sci. 7, 193–195. doi: 10.1016/s1360-1385(02)02251-3
Kim, D., Langmead, B., and Salzberg, S. L. (2015). HISAT: a fast spliced aligner with low memory requirements. Nat. Methods 12, 357–360. doi: 10.1038/nmeth.3317
King, S. R., Mclellan, H., Boevink, P. C., Armstrong, M. R., Bukharova, T., Sukarta, O., et al. (2014). Phytophthora infestans RXLR effector PexRD2 interacts with host MAPKKK ε to suppress plant immune signaling. Plant Cell 26, 1345–1359. doi: 10.1105/tpc.113.120055
Kong, Q., Qu, N., Gao, M., Zhang, Z., Ding, X., Yang, F., et al. (2012). The MEKK1-MKK1/MKK2-MPK4 kinase cascade negatively regulates immunity mediated by a mitogen-activated protein kinase kinase kinase in Arabidopsis. Plant Cell 24, 2225–2236. doi: 10.1105/tpc.112.097253
Kusch, S., and Panstruga, R. (2017). mlo-based resistance: an apparently universal “weapon” to defeat powdery mildew disease. Mol. Plant-Microbe Interact. 30, 179–189. doi: 10.1094/MPMI-12-16-0255-CR
Kwon, C. T., Heo, J., Lemmon, Z. H., Capua, Y., Hutton, S. F., Van Eck, J., et al. (2020). Rapid customization of Solanaceae fruit crops for urban agriculture. Nat. Biotechnol. 38, 182–188. doi: 10.1038/s41587-019-0361-2
Lacombe, S., Rougon-Cardoso, A., Sherwood, E., Peeters, N., Dahlbeck, D., Van Esse, H. P., et al. (2010). Interfamily transfer of a plant pattern-recognition receptor confers broad-spectrum bacterial resistance. Nat. Biotechnol. 28, 365–369. doi: 10.1038/nbt.1613
Lee, J. S., Hnilova, M., Maes, M., Lin, Y. C., Putarjunan, A., Han, S. K., et al. (2015). Competitive binding of antagonistic peptides fine-tunes stomatal patterning. Nature 522, 439–443. doi: 10.1038/nature14561
Li, H., Handsaker, B., Wysoker, A., Fennell, T., Ruan, J., Homer, N., et al. (2009). The sequence alignment/map format and SAMtools. Bioinformatics 25, 2078–2079. doi: 10.1093/bioinformatics/btp352
Li, Q., Wang, C., and Mou, Z. (2020). Perception of damaged self in plants. Plant Physiol. 182, 1545–1565. doi: 10.1104/pp.19.01242
Liang, X., and Zhou, J. M. (2018). Receptor-like cytoplasmic kinases: central players in plant receptor kinase-mediated signaling. Annu. Rev. Plant Biol. 69, 267–299. doi: 10.1146/annurev-arplant-042817-040540
Lisón, P., Rodrigo, I., and Conejero, V. (2006). A novel function for the cathepsin D inhibitor in tomato. Plant Physiol. 142, 1329–1339. doi: 10.1104/pp.106.086587
Liu, S., Wang, J., Jiang, S., Wang, H., Gao, Y., Zhang, H., et al. (2019). Tomato SlSAP3, a member of the stress-associated protein family, is a positive regulator of immunity against Pseudomonas syringae pv. tomato DC3000. Mol. Plant Pathol. 20, 815–830. doi: 10.1111/mpp.12793
Lu, F., Wang, H., Wang, S., Jiang, W., Shan, C., Li, B., et al. (2015). Enhancement of innate immune system in monocot rice by transferring the dicotyledonous elongation factor Tu receptor EFR. J. Integr. Plant Biol. 57, 641–652. doi: 10.1111/jipb.12306
Lukowitz, W., Roeder, A., Parmenter, D., and Somerville, C. (2004). A MAPKK kinase gene regulates extra-embryonic cell fate in Arabidopsis. Cell 116, 109–119. doi: 10.1016/s0092-8674(03)01067-5
Maere, S., Heymans, K., and Kuiper, M. (2005). BiNGO: a Cytoscape plugin to assess overrepresentation of gene ontology categories in biological networks. Bioinformatics 21, 3448–3449. doi: 10.1093/bioinformatics/bti551
McKown, K. H., and Bergmann, D. C. (2020). Stomatal development in the grasses: lessons from models and crops (and crop models). New Phytol. doi: 10.1111/nph.16450 [Epub ahead of print]
Meng, X., Chen, X., Mang, H., Liu, C., Yu, X., Gao, X., et al. (2015). Differential function of Arabidopsis SERK family receptor-like kinases in stomatal patterning. Curr. Biol. 25, 2361–2372. doi: 10.1016/j.cub.2015.07.068
Molina, A., Jordá, L., Sánchez-Rodriguez, C., Sopeña-Torres, S., López, G., Miedes, E., et al. (2016). Method for increasing pathogen resistance in plants. US14/652,285.
Morales-Navarro, S., Pérez-Díaz, R., Ortega, A., De Marcos, A., Mena, M., Fenoll, C., et al. (2018). Overexpression of a SDD1-Like gene from wild tomato decreases stomatal density and enhances dehydration avoidance in Arabidopsis and cultivated tomato. Front. Plant Sci. 9:940. doi: 10.3389/fpls.2018.00940
Nakagawa, T., Kurose, T., Hino, T., Tanaka, K., Kawamukai, M., Niwa, Y., et al. (2007). Development of series of gateway binary vectors, pGWBs, for realizing efficient construction of fusion genes for plant transformation. J. Biosci. Bioeng. 104, 34–41. doi: 10.1263/jbb.104.34
Oliveros, J. C., Franch, M., Tabas-Madrid, D., San-León, D., Montoliu, L., Cubas, P., et al. (2016). Breaking-Cas-interactive design of guide RNAs for CRISPR-Cas experiments for ENSEMBL genomes. Nucleic Acids Res. 44, W267–W271. doi: 10.1093/nar/gkw407
Ortega, A., De Marcos, A., Illescas-Miranda, J., Mena, M., and Fenoll, C. (2019). The tomato genome encodes SPCH, MUTE, and FAMA candidates that can replace the endogenous functions of their. Front. Plant Sci. 10:1300. doi: 10.3389/fpls.2019.01300
Pertea, M., Pertea, G. M., Antonescu, C. M., Chang, T. C., Mendell, J. T., and Salzberg, S. L. (2015). StringTie enables improved reconstruction of a transcriptome from RNA-seq reads. Nat. Biotechnol. 33, 290–295. doi: 10.1038/nbt.3122
Ramírez, V., López, A., Mauch-Mani, B., Gil, M. J., and Vera, P. (2013). An extracellular subtilase switch for immune priming in Arabidopsis. PLoS Pathog. 9:e1003445. doi: 10.1371/journal.ppat.1003445
Samakovli, D., Tichá, T., Vavrdová, T., Ovečka, M., Luptovčiak, I., Zapletalová, V., et al. (2020). YODA-HSP90 module regulates phosphorylation-dependent inactivation of SPEECHLESS to control stomatal development under acute heat stress in Arabidopsis. Mol. Plant 13, 612–633. doi: 10.1016/j.molp.2020.01.001
Schaller, A., Stintzi, A., Rivas, S., Serrano, I., Chichkova, N. V., Vartapetian, A. B., et al. (2018). From structure to function—a family portrait of plant subtilases. New Phytol. 218, 901–915. doi: 10.1111/nph.14582
Schindelin, J., Arganda-Carreras, I., Frise, E., Kaynig, V., Longair, M., Pietzsch, T., et al. (2012). Fiji: an open-source platform for biological-image analysis. Nat. Methods 9, 676–682. doi: 10.1038/nmeth.2019
Shen, H., Zhong, X., Zhao, F., Wang, Y., Yan, B., Li, Q., et al. (2015). Overexpression of receptor-like kinase ERECTA improves thermotolerance in rice and tomato. Nat. Biotechnol. 33, 996–1003. doi: 10.1038/nbt.3321
Sopeña-Torres, S., Jorda, L., Sanchez-Rodriguez, C., Miedes, E., Escudero, V., Swami, S., et al. (2018). YODA MAP3K kinase regulates plant immune responses conferring broad-spectrum disease resistance. New Phytol. 218, 661–680. doi: 10.1111/nph.15007
Stokes, T. L., and Richards, E. J. (2002). Induced instability of two Arabidopsis constitutive pathogen-response alleles. Proc. Natl. Acad. Sci. U. S. A. 99, 7792–7796. doi: 10.1073/pnas.112040999
Suarez-Rodriguez, M. C., Adams-Phillips, L., Liu, Y., Wang, H., Su, S. H., Jester, P. J., et al. (2007). MEKK1 is required for flg22-induced MPK4 activation in Arabidopsis plants. Plant Physiol. 143, 661–669. doi: 10.1104/pp.106.091389
Sun, T., Nitta, Y., Zhang, Q., Wu, D., Tian, H., Lee, J. S., et al. (2018). Antagonistic interactions between two MAP kinase cascades in plant development and immune signaling. EMBO Rep. 19:e45324. doi: 10.15252/embr.201745324
Tamura, K., Peterson, D., Peterson, N., Stecher, G., Nei, M., and Kumar, S. (2011). MEGA5: molecular evolutionary genetics analysis using maximum likelihood, evolutionary distance, and maximum parsimony methods. Mol. Biol. Evol. 28, 2731–2739. doi: 10.1093/molbev/msr121
Thordal-Christensen, H. (2020). A holistic view on plant effector-triggered immunity presented as an iceberg model. Cell. Mol. Life Sci. doi: 10.1007/s00018-020-03515-w [Epub ahead of print]
Thulasi Devendrakumar, K., Li, X., and Zhang, Y. (2018). MAP kinase signalling: interplays between plant PAMP‐ and effector-triggered immunity. Cell. Mol. Life Sci. 75, 2981–2989. doi: 10.1007/s00018-018-2839-3
Wu, J., Wang, J., Pan, C., Guan, X., Wang, Y., Liu, S., et al. (2014). Genome-wide identification of MAPKK and MAPKKK gene families in tomato and transcriptional profiling analysis during development and stress response. PLoS One 9:e103032. doi: 10.1371/journal.pone.0103032
Yamada, K., Yamaguchi, K., Shirakawa, T., Nakagami, H., Mine, A., Ishikawa, K., et al. (2016). The Arabidopsis CERK1-associated kinase PBL27 connects chitin perception to MAPK activation. EMBO J. 35, 2468–2483. doi: 10.15252/embj.201694248
Zhang, J., Shao, F., Li, Y., Cui, H., Chen, L., Li, H., et al. (2007). A Pseudomonas syringae effector inactivates MAPKs to suppress PAMP-induced immunity in plants. Cell Host Microbe 1, 175–185. doi: 10.1016/j.chom.2007.03.006
Zhang, M., Su, J., Zhang, Y., Xu, J., and Zhang, S. (2018). Conveying endogenous and exogenous signals: MAPK cascades in plant growth and defense. Curr. Opin. Plant Biol. 45, 1–10. doi: 10.1016/j.pbi.2018.04.012
Keywords: Mitogen-activated protein kinases cascades, disease resistance, YDA, breeding, tomato, bacteria, plant immunity, stomata
Citation: Téllez J, Muñoz-Barrios A, Sopeña-Torres S, Martín-Forero AF, Ortega A, Pérez R, Sanz Y, Borja M, de Marcos A, Nicolas M, Jahrmann T, Mena M, Jordá L and Molina A (2020) YODA Kinase Controls a Novel Immune Pathway of Tomato Conferring Enhanced Disease Resistance to the Bacterium Pseudomonas syringae. Front. Plant Sci. 11:584471. doi: 10.3389/fpls.2020.584471
Edited by:
Vincenzo Lionetti, Sapienza University of Rome, ItalyReviewed by:
Brian H. Kvitko, University of Georgia, United StatesHidenori Matsui, Okayama University, Japan
Copyright © 2020 Téllez, Muñoz-Barrios, Sopeña-Torres, Martín-Forero, Ortega, Pérez, Sanz, Borja, de Marcos, Nicolas, Jahrmann, Mena, Jordá and Molina. This is an open-access article distributed under the terms of the Creative Commons Attribution License (CC BY). The use, distribution or reproduction in other forums is permitted, provided the original author(s) and the copyright owner(s) are credited and that the original publication in this journal is cited, in accordance with accepted academic practice. No use, distribution or reproduction is permitted which does not comply with these terms.
*Correspondence: Antonio Molina, YW50b25pby5tb2xpbmFAdXBtLmVz; Lucía Jordá, bHVjaWEuam9yZGFAdXBtLmVz